
13 minute read
CRISPR, one tool with the potential to change our world
Pictured above are Emmanuelle Charpentier and Jennifer Doudna, 2020 Nobel Prize winners in chemistry
Authors: Laurence D. Melton¹ and Andrew C. Allan²,³ 1 School of Chemical Sciences, University of Auckland, Auckland 2 Plant & Food Research, Mt Albert, Auckland 3 School of Biological Sciences, University of Auckland, Auckland
Introduction
Emmanuelle Charpentier and Jennifer Doudna, pictured above, were awarded the Nobel Prize in chemistry in 2020. This is the first time that two women have shared a Nobel Prize, by itself an important milestone. Their research on CRISPR-based gene editing heralds a new era in the biological sciences including food science. CRISPR has the potential to cure life-threatening hereditary diseases such as cystic fibrosis and muscular dystrophy, while also targeting some breast, ovarian, prostate and stomach cancers. Aside from its use in medicine, CRISPR can be applied to plants, animals, bacteria and fungi. If used in plant breeding, CRISPR-directed changes to the genome can generate new higher yielding wheat, rice and corn, to help feed the world’s burgeoning population. Gene editing can be used to produce disease resistant plants and animals, and facilitate production of plant proteins as meat substitutes. Nutrition is improved by targeted breeding of pigs with less fat and cooking oils with less saturated fat, or tomatoes with higher levels of Vitamin A. Heat and drought tolerant crops and animals will assist in dealing with global warming.
What is CRISPR?
CRISPR (pronounced “crisper”) stands for “clustered regularly interspaced short palindromic repeats”. At the core of CRISPR are pieces of RNA, which are commonly loaded into an enzyme (a DNAcutter) called Cas9. The role of the CRISPR system in nature is to protect bacteria from viruses. Bacteria that survive a viral infection keep part of the viral DNA, which is stored in the bacterial genome between CRISPR sequence repeats. If the same virus invades again, the bacteria have
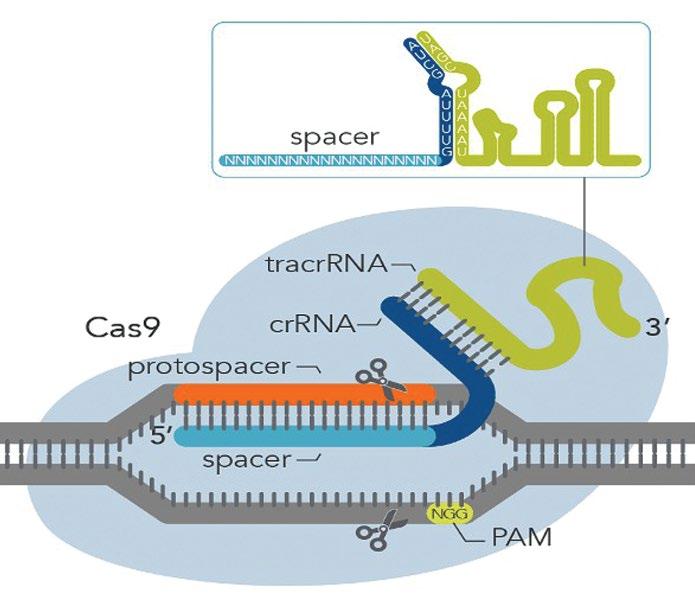
Fig. 2. Schematic representation of how CRISPR-Cas9 functions. The Cas9 endonuclease (pale blue silhouette) interacts with two small RNAs, CRISPR RNA (crRNA) (blue) which matches the DNA target sequence (orange). The part of the crRNA that is complementary to the target sequence is called the spacer. The second tracrRNA (green) base pairs with crRNA and acts as a to link the crRNA to Cas9. The other essential part of the system is PAM (protospacer adjacent motif) which is 2 – 6 nucleotides of DNA (in this example N = any nucleotide and G = guanine). Cas9 cuts the DNA strands precisely 3 nucleotides upstream from PAM.
a complementary RNA sequence that guides a Cas9 nuclease to cut the viral DNA, stopping the infection. Scientists can make their own versions of CRISPR RNAs, which guide the Cas enzymes to cut specific DNA in target genes. In other words CRISPR-Cas acts as genetic scissors that can precisely edit or alter specific genes. In the CRISPR-Cas9 system (Fig.2) the short CRISPR RNA (crRNA) sequence matches the DNA target sequence. The part of the crRNA that is complementary to the target sequence is called the spacer. The tracrRNA base pairs with crRNA and acts as a scaffold to link the crRNA to Cas9 endonuclease (shown as a blue silhouette). The other essential part of the system is PAM (protospacer adjacent motif) which is a short (2-6 nucleotides) DNA region 3 nucleotides upstream from where Cas cuts the DNA strand. The Cas9 nuclease, isolated from Streptococcus pyogenes, recognises a PAM sequence of NGG (where N is any nucleotide base and G is Guanine). If the target region does not have NGG then Cas9 cannot act. However, there are many different Cas endonucleases (e.g. Cas12, Cas13) each isolated from different bacteria, and each one recognises a different PAM. For example, Cas9 from Staphylococcus aureus (SaCas9) recognises the PAM, NNGRRT (where R is a purine, T is thymine).
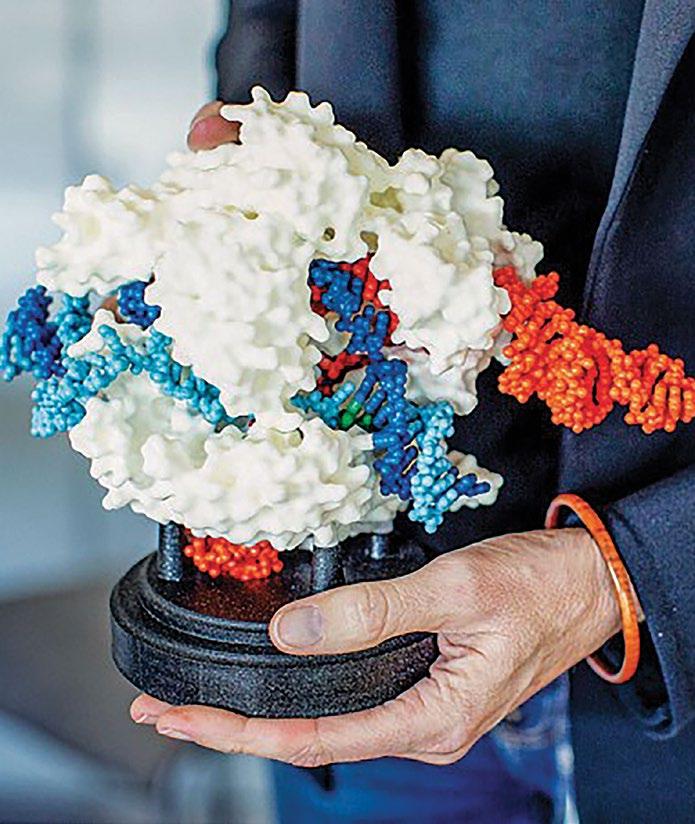
Fig.3 shows the space-filling model of the DNA double-helix (blue) being acted on by CRISPR (orange) and Cas nuclease (white). Fig. 3.(A) Model of CRISPR-Cas9 acting on DNA. Cas9 is coloured white, DNA strands are two shades of blue and CRISPR is orange. Source: Laura Morton Photography
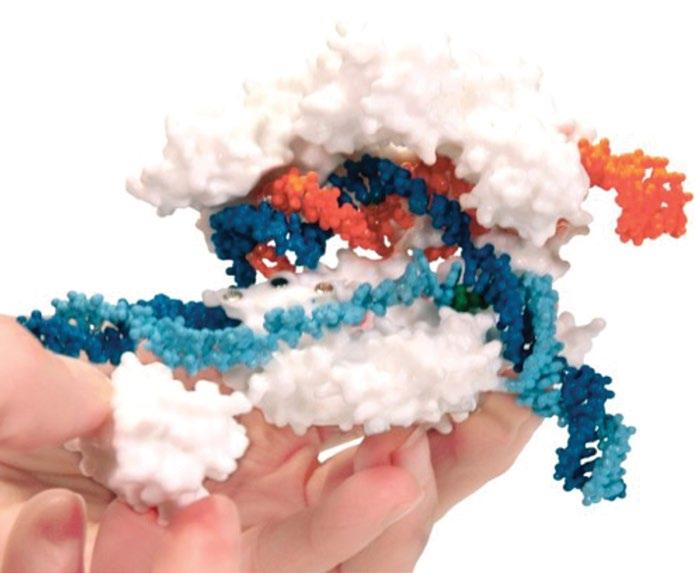
Fig3(B) Model opened to show the interaction of the Cas9 enzyme with DNA and CRISPR. Cas9 is coloured white, DNA strands are two shades of blue and CRISPR is orange
CRISPR, when used as a gene editing technique, can be targeted to more than one gene at the same time (by using more than one RNA guide sequence). It does not involve adding DNA from a different species into the host genome (eg. plant or animal), which could result in unintended or undesirable consequences. It can be applied to plants, animals, yeasts and bacteria. There are several other enzyme-based gene editing techniques, yet CRISPR remains faster and cheaper to use, and is now in use in thousands of laboratories world-wide.
Human Diseases
CRISPR has the potential to cure many hereditary diseases that involve a single gene defect. These include sickle cell disease, cystic fibrosis, Duchenne muscular dystrophy, phenylketonuria, Tay-Sachs disease, multiple myeloma, hereditary diffuse gastric cancer, Huntington chorea, familial hypercholesterolemia, haemochromatosis and haemophilia. These are seriously life-threatening diseases, some of which, such as Huntington chorea, Duchenne muscular dystrophy and Tay-Sachs, have no effective treatment. In other cases the cures currently available can be drastic: for cystic fibrosis by lung transplant, for diffuse gastric cancer removal of the entire stomach, haemophilia by liver transplant and haemochromatosis by old-fashioned bloodletting. Sickle cell anaemia affects millions of people in Africa and as many as 100,000 in the USA. The life expectancy in Africa is 5 to 8 years and in the USA 40 years. For CRISPR-therapy, blood taken from the patient could provide pluripotent stem cells to be gene edited using CRISPR to knock out the defective gene, and the blood returned to the patient¹. Matthew Porteous reported² that while a 20% alteration was sufficient to obtain a cure in mice, they were able to reach 70%. What is not known at this time is how long the cure lasts, but it has lasted two years in mice². Clinical trials with CRISPR-treated patients led to an announcement in January 2021 that one patient with sickle cell disease and one patient with beta-thalassemia, an abnormal form of haemoglobin causing the destruction of red blood cells resulting in anaemia, had been cured³. At least five more clinical trials are being conducted in China. Replacing blood is relatively easy. Other organs such as liver, pancreas, kidneys and brain present a much greater challenge. One approach is to use in vivo gene editing (e.g. by injection of CRISPR/Cas9 plasmids into the liver4). Less invasively, CRISPR-treated stem cells can be packaged in fatty nanoparticles that pass through the target-cell membranes, and are carried by the blood system to the liver5. This procedure is under industrial devlopment6. Hereditary blindness has been treated by injecting, into the eye, a virus that produces the CRISPR system, bypassing the need to use the blood stream. Initial work with mice has led to a human trial6. A recent review7 covers additional delivery systems for different organs. Transplant organs from pigs
Due to the shortage of human organs for transplants, pigs are potential alternatives. The problem of introducing foreign antigens can be solved by using CRISPR to knock out key genes8. In New Zealand, AgResearch has a science team that aims to produce transplant organs using pigs.
Feeding this world and climate change
Some of the diseases that may be cured by CRISPR gene editing techniques involve a relatively small number of people. For example, only one baby in 15,000 is expected to have phenylketonuria, and there are about 5 cases of Huntington’s chorea per 100,000. In contrast, food supply is essential for everyone. The world population is expected to reach 7.8 billion in 2021 and increase by 24% to 9.7 billion in 2050. There are already food shortages in parts of the world. The Guardian Weekly (1st March 2021) reported that famine in Yemen is likely to be the worst the world has seen in decades. UNICEF estimates that with the added pressure of Covid-19, 54 million children worldwide are currently suffering from life-threatening malnutrition and this is likely to be exacerbated by global warming. Plant breeding has been integral to the world’s success in coping with increased population. New tools, such as CRISPR, have the potential to help deal with the dual challenge of more people and global warming. CRISPR can be applied to all aspects of food production9. See Table 1, below.
Technique Application
Knockin Produces leaner pigs
Knockout Increases rice yields
Endogenous use of Cas9 Increases phage protection in yoghurt
Knockin Increases drought resistance in corn
Knockout Reduces browning in mushrooms
nCas9 Increases tuberculosis resistance in cattle
Table 1. Applications of CRISPR-Cas9 technology to agriculture and food production.9
It has been used to increase yield and other desired traits in crops such as wheat10 , 11, corn¹² and rice¹³. With a more consumer-focus CRISPR edits can produce pork with less fat14, while gluten-reduced wheat has been achieved resulting in bread for people with coeliac disease15 . Tomatoes bought in supermarkets can be tasteless because they are harvested too early, while home grown tomatoes taste great. Using CRISPR the softening enzyme pectate lyase was turned off16 so the tomatoes can be left on the vines longer to potentially develop more flavour. Possibly the first gene-edited food was high oleic acid soybean oil with longer frying life, which came on the market in the USA in March 2019. However, it was gene edited by a method called TALEN, considered slower and more expensive compared to CRISPR. Potatoes stored at low temperature turn an unattractive brown when fried at high temperatures to prepare chips (French fries). TALEN was used to prevent the breakdown of starch to glucose, which results in the browning reaction, and to reduce acrylamide formation17. These potatoes have been on sale in the USA since 2019.
Aiding food and agriculture
CRISPR has the potential to aid food and agriculture in even more ways (Table 2.) including breeding plants that are disease resistant, or herbicide resistant18 , 19. Because CRISPR systems are a natural defence against viruses they are ideal for stopping viral infections20 . Moreover, CRISPR can be used to facilitate the production of bioactive compounds18 such as lysine, beta-carotene, lycopene as well as D-pantothenic acid²¹. Most importantly CRISPR can be used to adapt plants and animals to increasing heat and drought caused by global warming. The USDA ARS is developing drought- and salt- tolerant soybeans and DuPont is working on corn to produce very high amylopectin starch²². Moreover, alleles (gene variants) that are observed in a domestic plant (such as tomato), can be re-created using CRISPR, to improve the yield of a drought resistant wild variety.²³ In New Zealand, AgResearch has committed to a program of producing heat tolerant cows with increased milk production and lower greenhouse gas emissions (N.Z. Herald, 1st Oct. 2019). Plant & Food Research is using CRISPR to edit flowering24 , 25 and ripening related genes26 with the aim of the research being to reduce the time needed to breed perennial plants, as well as increasing the storage life of fruit.
Food Safety
Applications with bacteria and viruses
CRISPR-Cas13a can be used to detect bacteria causing food poisoning (e.g. Staphylococcus aureus, Escherichia coli, Salmonella). Moreover, CRISPR technology can identity different strains of these bacteria as well as those of lactobacilli and streptococci. The strains may have almost identical genomes, except for the small differences detected by CRISPR. Fingerprinting means superior strains can be chosen for food fermentations and production of probiotics. CRISPR naturally acts on and destroys bacteriophages, consequently improving yoghurt manufacture, for example. Killing targeted microbes is achievable using CRISPR. Hau Liu et al.27 combined CRISPR-Cas12a with PCR amplification to detect food poisoning bacteria, meat adulteration and genetically modified crops. The detection procedure took just 45 minutes. Similarly, CRISPR-Cas13a with PCR amplification has been used to detect Staphylococcus aureus in milk, juice and beer.28 Table 2. Further applications of CRISPR- Cas gene-editing in food and agriculture.18 - See article in Magazine for Table 2
In a further development, CRISPR-Cas13a editing with a three-stage amplification but without bacterial isolation, detected Salmonella enterica Enteritidis close to 1 CFU in milk.29
New Zealand regulations compared to other countries
In New Zealand, CRISPR gene editing to improve foods is treated under the same regulations as GMOs. EU regulations currently have a similar level of restriction. In contrast other countries are far less regulated. Australia has indicated that an organism with changes to its DNA equivalent to spontaneous mutations that arise naturally, need not be regulated. Sweden has announced that CRISPR isn’t a GMO technology. Canada and the USA work on a case-by-case basis. The USA has already approved more than ten gene edited crops as having non-regulated status. Gene editing to cure human diseases in New Zealand is regulated by the Hazardous Substances and New Organisms Act (HSNO Act) and the Medicines Act, meaning CRISPR is treated like a GMO. Approval of gene drives in New Zealand is complicated: they are regulated under the HSNO Act and the Agricultural Compound and Veterinary Medicines Act and four other Acts have to be considered.
Concluding Remarks
There are many exciting and ground-breaking implications of the use of CRISPR both in human health, and in the supply of food. Some aspects to consider that will aid feeding of an increasing population, under the increasing pressure of climate change, include: • Plants and animals resistant to diseases, leading to less food waste. • Longer life of fresh vegetables and fruit (and cheaper processing), leading to less food waste • Reduction of pesticides • Tastier plant-based foods (flavour, colour, texture) • Plant and animal adaption to climate change • Greater CO2 fixation by plants • Improvements in monitoring food safety The main concerns of the wider use of CRISPR-based editing should include careful testing for unintended effects and scrutiny of who benefits and who is asked to take any risks associated with any innovation. However, restrictive legislation aimed at stopping innovation risks further harm to our planet and its inhabitants.
References
1. Park, S. H.; Lee, C.M.; Dever, D. P.; ……Porteous, M.; Sheehan, V.A. Bao. G. Blood 2018, 132, 2192.
2.Porteous, M. Genome Editing of Human Stem Cells. School of Biological Sciences Seminar, University of Auckland, 30 August 2019.
3. Frangoul, H.; Altshuler, D.; Cappellini, M.D.;……Corbacioglu, S. New Eng. J. Med. 2021, 384 (3), 252 – 260.
4. Yin, H.; Xue, W.; Chen, S.;……Anderson, D.G. Nat. Biotechnol. 2014, 32 (6), 551 – 553
5. Yin, H.; Song, C.Q.; Dorkin, J.R.;……Anderson, D.G. Nat. Biotechnol. 2016, 34 (3),328 – 333.
6.Ledford, H. Nature, 2020, 577. 156
7.Haasteren, J.; Li, J.; Scheideler, O.J.; Murthy, N.; Schaffer, D.V. Nat. Biotechnol. 2020, 38, 845 – 855.
8.Tanihara, F.; Hirata, M.; Nguyen, N.T.;……Otoi, T. BMC Biotechnol. 2020, 20, 40 – 51.
9.Brandt, K.; Barrangou, R. Annu. Rev. Food Sci. Technol. 2019, 10, 133 – 150.
10. Okada, A.; Arndell, T.; Borisjuk, N; ……Whitford, R. Plant Biotechnol. J. 2019, 17, 1905–1913.
11.Kumar, R.; Kaur, A.; Pandey, A.; Mamrutha, H.M.; Singh, G.P. Mol. Biol. Rep. 2019, 46, 3557 – 3569.
12. Tian, J.; Wang, C.; Xia, J.;……Tian, F. Science 2019, 365, 658 – 664.
13. Usman, B.; Nawaz, G.; Zao, N.;……Li, R. Int. J. Mol. Sci. 2021, 22, 249 – 267.
14. Zheng, Q.; Lin, J.; Huang, J.;……Zhao, J. Proc. Natl. Acad. Sci. U.S.A. 2017, 114(45), E9474 – E9482.
15. Jouanin, A.; Gilissen, L.J.; Schaart, J.G……Smulders, M.J. Front. Nutr. 2020, 7, 51.
16. Wang, D.; Samsulrizal, N.H.; Yan, C.;…...Seymour, G.B. Plant Physiol. 2019, 179, 544 – 557.
17.Waltz, E. Nat. Biotechnol. 2019, 37, 573 – 575
18. Es, I.; Gavahian, M.; Marti-Quijal, F.J.; ……Barba, F.J. Biotechnol. Adv. 2019, 37, 410 – 421.
19.Schenke, D.; Cai, D. iScience 2020, 23, 101478.
20. Kalinina, N.O.; Khromov, A.; Love, A.J.; Taliansky, M.E. Phytopathology, 2020, 110, 18 – 28.
21.Zhang, B.; Zhang, X.-M.; Wang, W.; Liu, Z.-Q.; Zheng, Y.-G. Food Chem. 2019, 294, 267 – 275.
22.Waltz, E. Nat. Biotechnol. 2018, 36, 6 – 7.
23. Khan, M.Z.; Zaidi, S.S.; Amin, I.; Mansoor, S. Trends Plant Sci. 2019,
24 (4), 293 – 296. 24. Akagi, T.; Pilkington, S.M.; Varkonyl-Gasic, E.; ……Tao, R. Nature Plants 2019, 5, 801 – 809.
25. Varkonyl-Gasic, E.; Wang, T.; Voogd, C.;……Allan, A.C. Plant Biotech. J. 2019, 17, 869 – 880.
26. Wang, R.; Nardozza, S.; Nieuwenhuizen,N.J.;……Schaffer, R.J. N.Z.J. Crop. Hort. Sci. 2021, 49, 277 – 293.
27. Liu, H.; Wang, J.; Zeng, H.; ……Tang X. Food Chem. 2021, 334, 127608.
28. Zhou, J,; Yin, L.; Dong, Y.;……Ma, L. Anal. Chim. Acta 2020, 1127, 225 – 233.
29. Shen, J.; Zhou, X.; Shan, Y.; ……Xing, D. Nature Commun. 2021, 11, 267.