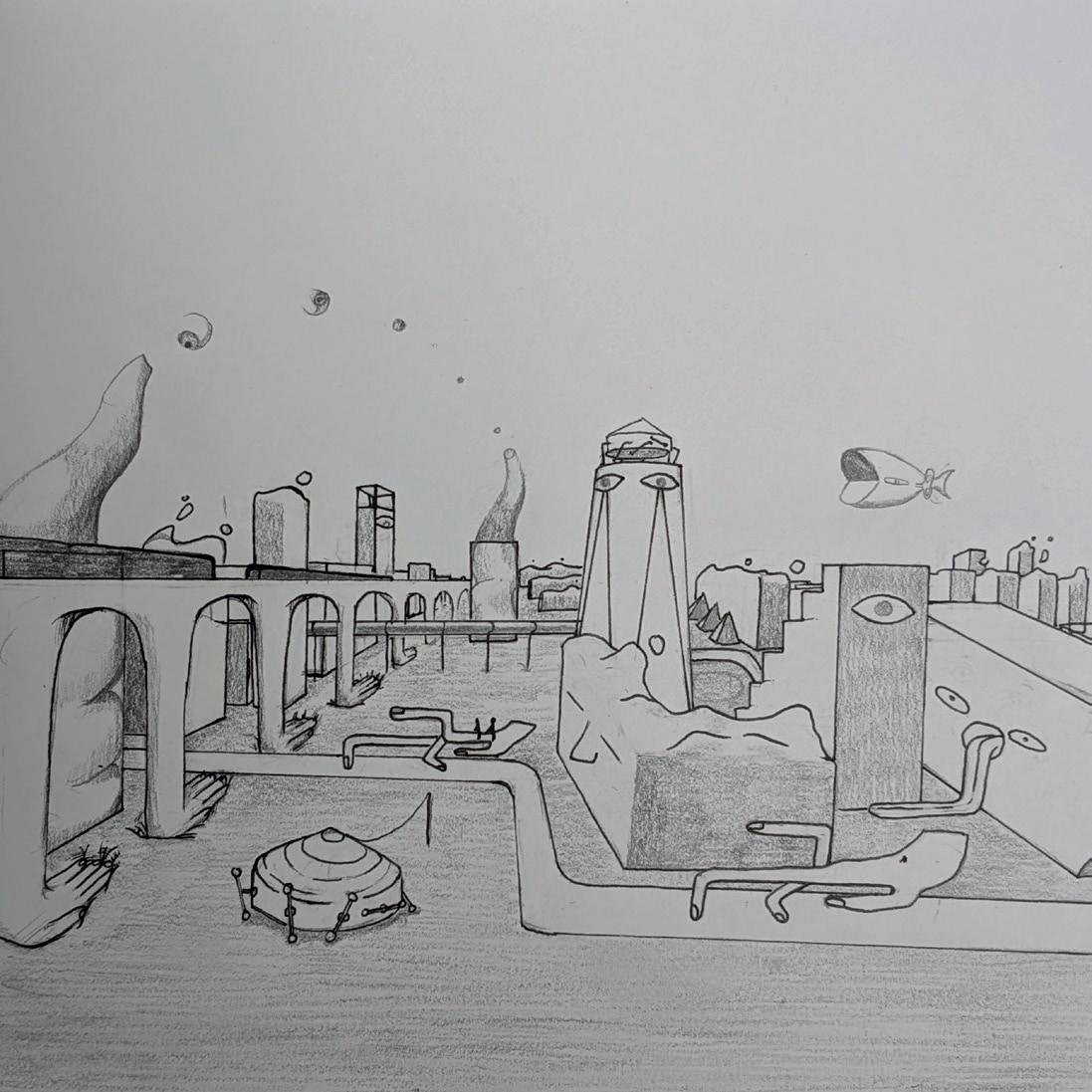
6 minute read
Quantum Internet An Entangled World
NOBEL PRIZE | Last October, the Nobel Prize was awarded to Alain Aspect, John Clauser and Anton Zeilinger, who independently conducted ground-breaking experiments on quantum entanglement with photons, which are what light is made up of. This is an important recognition as it was awarded for their work in quantum information which is gaining a lot of momentum.
We are so reliant on the internet that if the internet were to break down, it would have a devastating impact. Security and reliable transmission of messages are at the heart of the internet. The modern internet encodes messages into light signals, which are transferred from one location to another via fibre optic cables. These messages are often encrypted so that if the wrong person intercepts the message, the classical computers that we use today won’t be able to decrypt the message to figure out what it says.
A fundamental issue with classical cryptography is that it is often based on encryptions that are very difficult for a ‘classical computer’ to decrypt. Unfortunately, with the advent of quantum computers, the performance and efficiency of calculations will be so incredibly high that it will become very easy to crack such encryptions using quantum algorithms. This is where a quantum internet which also harnesses the principles of quantum mechanics becomes very important.
system or object - its position, spin or energy, just to list a few. For classical objects, these properties are well-defined given some initial conditions - for example, once we kick a football in the air, it will spin in air and there is a velocity at which it will land on the ground. We can use this information to completely describe the state of the ball when it lands. However, a quantum object can be ‘undecided’ on its properties, meaning that there will be a probability distribution over the values it can take for a physical quantity; the presence of these probability distributions is called a superposition.
Let’s consider a special case where a quantum object can take one of only two measurement outcomes with a particular probability. Such a quantum object is called a qubit. For example, the vertical component of an electron’s spin can only be either ‘up’ or ‘down’ each with a particular probability. But after measuring it, its vertical spin will be instantaneously “decided” and the measurement outcome will define its evolution from that point. So from the point at which the measurement is made, its value is determined and hence the range of values it can take “collapses under measurement”.
Crash Course On Quantum Mechanics
| Superposition, collapse under measurement and entanglement are three key principles of quantum mechanics that we can utilise. Entanglement will be the primary focus of this article, but to understand entanglement, we need to understand the other two principles first.
There are many physical quantities we can measure about a
Now that we’ve grasped superposition and collapse under measurement, it becomes slightly easier to understand what entanglement is. Entanglement is a way that distant photons can interact with each other, to make quantum communication possible between them. Quantum objects are said to be ‘entangled’ to each other if a different measurement outcome of a quantity of any one of these objects will result in a different overall state of the others, i.e. there is a correlation between the values that each object takes. Importantly, this interaction is instantaneous. Zeilinger and colleagues reported in 2015 that they were able to create entanglement between photons that were separated by 143 km between two Canary islands. This work was a major step in demonstrating that quantum entanglement could be used for long-distance quantum communications. Since then, China has released its famous Micius satellite, the world’s first quantum communications satellite, that could be used to create entanglement between ground stations that were over 1,120 km apart.
WHAT QUANTUM COMMUNICATION ISN'T |
We have seen that the overall state of entangled objects miles away can seemingly change instantaneously when we perform a measurement on a local particle. Does this mean we can have faster-than-light communication? This turns out not to be the case; let’s see why.
Let us introduce Alice (A) and Bob (B), who are in distant locations and share an entangled state of two photons that can take two possible polarisations ‘0’ or ‘1’ with equal probability (giving their superpositions). They are entangled so that if A’s photon is measured to have some polarisation, then B’s photon must also have that same polarisation, and vice versa. For example, if A measures her photon to be 0 then when B measures his photon he must also find that it’s 0.
Interestingly, there is a ‘no-signalling principle’ which states that Alice cannot convey any information to Bob by only performing local operations, so Alice and Bob must use some other non-local form of communication to tell each other what’s going on. Therefore, Bob cannot observe any change due to Alice’s actions faster than the speed of light since Bob can't be sure about what she did or what she observed. This certainly protects quantum mechanics from the violation, but this then raises the question about what quantum communication is even good for, if it can’t work independently of other forms of communicating. In the next section, you’ll see how in fact the quantum internet will pick up where our current internet has left off. To do these things, it becomes especially important to fully utilise measurement collapse.
ENTANGLEMENT SWAPPING AND ERROR CORRECTION |
The classical internet uses fibre optic cables to transfer information between locations. However, over long distances, the light signal will decay as it passes through the cable. As a result, we use intermediate ‘repeaters’ to amplify the signal and allow for long-distance transmission. Fibre optics can similarly be used for the quantum internet, but in the quantum setting, there is a ‘no-cloning theorem’ which prevents us from this amplification as it means that the signal cannot be copied exactly. Instead, ‘quantum repeaters’ use a process called “entanglement swapping” which we will look at in more detail.
Suppose now, Alice and Bob independently have two entangled photons each and they want a shared entangled photon state between them to be able to communicate, but they are too far apart (so A cannot just send one of her photons to B as it’ll deteriorate). We can introduce a third party, the Repeater R, which is directly halfway between A and B. Now A and B can each send one of their photons to R, and now they will have a much greater chance of reaching R as it’s half the distance as before. R will conduct some local operations, including an intermediary measurement, and this will cause the leftover photons of A and B to be entangled! R is actually a quantum repeater, and we can continue to slice the transmission distance of the photon in this way, by introducing more quantum repeaters until the distance is reasonable. This process of ‘entanglement swapping’ is also how Zeilinger et al were able to create entanglement at such an immense distance.
While using quantum repeaters means we may not have to worry about our photons reaching their destinations, there will be some inevitable accumulation of noise. Thus, we’ll have to conduct some error correction to remove this noise, which involves adding some redundancy to our encoded data. Making error correction scalable over long distances is an area of ongoing research, as this currently requires a lot of computing power and for us to produce a large entangled state of photons.
QUANTUM KEY CRYPTOGRAPHY | Quantum key cryptography improves upon classical cryptography by combining classical communication with quantum mechanics to produce ‘keys’ that encrypt and decrypt messages, which cannot be figured out by eavesdroppers equipped with quantum computers. An eavesdropper Eve (E) trying to decipher the communication between Alice and Bob will have to take some measurement to get information from their messages. But with measurement comes a collapse that Alice and Bob may be able to notice, and this is the basis for all quantum cryptosystems. For example, quantum key distribution protocols detect eavesdropping and as long as it’s below a certain level, so A and B can correct for this and generate a secret key that can be used for provably secure communication. Quantum key cryptography generally uses a lot of resources; it requires a lot of qubits to be sent to create a key, and each key can be used only once.
CLOSING REMARKS | We’ve seen that quantum communication and classical communication have clear differences. While quantum mechanics has imposed some theoretical limitations to quantum communication, by combining it with classical communication, we can improve the security of our communication.Yet, there are still some practical limits, since we still cannot create enough qubits to be able to use error correction for communication over long distances, and for quantum information processing to work we’ll need quantum memory storage devices. However, these issues will eventually be solved and once they are, quantum communication will fundamentally change how our devices interact and the quantum internet will be born.
Shreyas Iyar is a Master's student studying Mathematics at St Catharine's College. Shreyas continues to explore the field of quantum computing as a part of his degree, and believes it has the potential to revolutionise the world. Illustration by Josh Langfield.