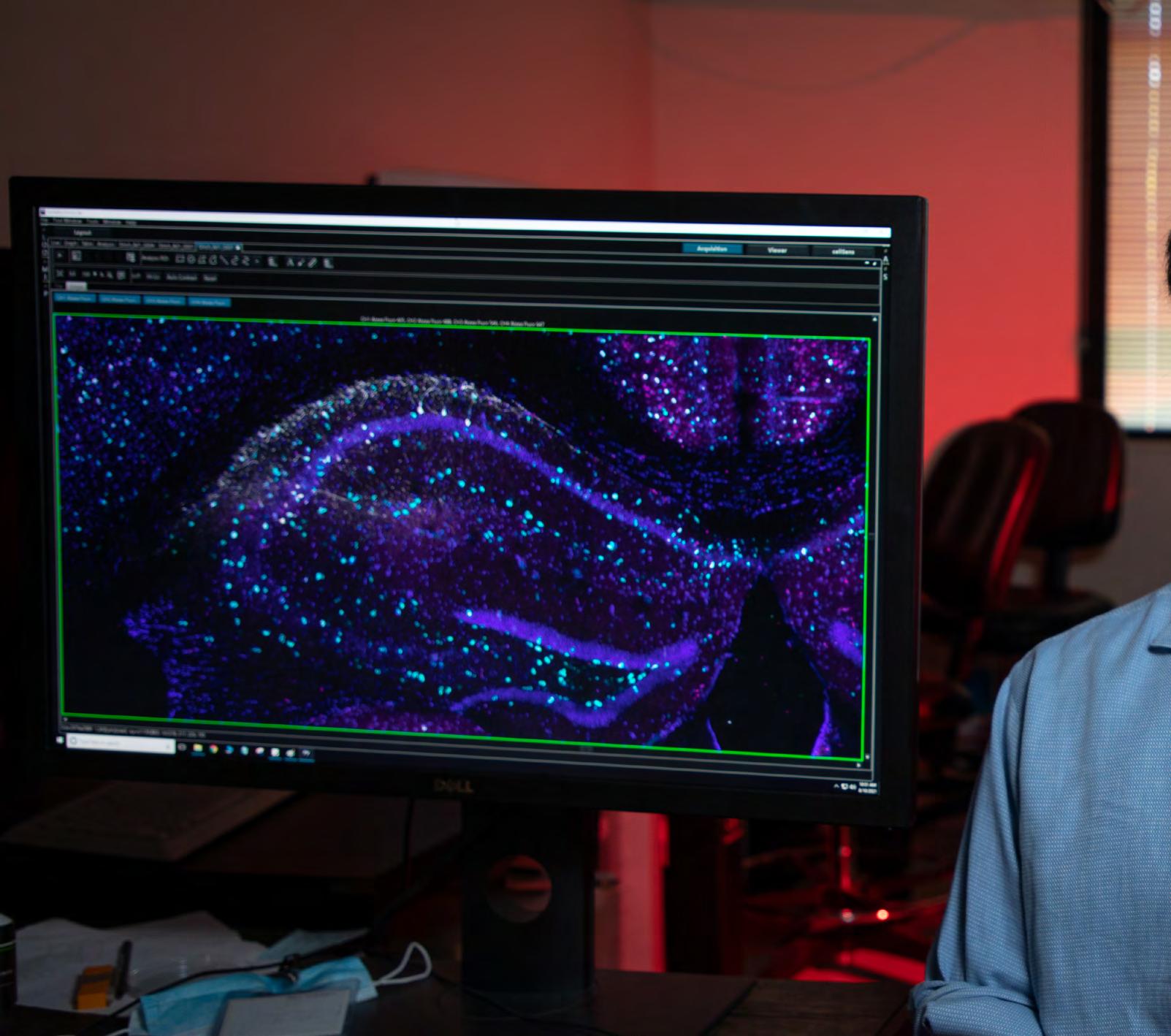
4 minute read
OW IS THE BRAIN PUT TOGETHER?
from CerebrumSpring2022
HOW DOES IT WORK—WHAT HAPPENS WHEN WE THINK, FEEL, ACT?
What goes wrong in psychiatric and neurological illness? A giant step toward answering these central questions of neurobiology emerged over the last 15 years with the development of optogenetics. This now-essential instrument in the neuroscientist’s toolkit represents a convergence of botany, microbiology, proteomics, genomics, and optics, and an accordingly wide array of contributions by an impressive cast of researchers.
Advertisement
A leader of the pack is unarguably Karl Deisseroth, M.D., Ph.D., professor of bioengineering and of psychiatry and behavioral sciences at Stanford University, one of three scientists awarded the 2021 Lasker Prize in Basic Medical Research for his pivotal work. Deisseroth, a practicing psychiatrist as well as a researcher, hit another milestone last year with the publication by Random House of Projections: A Story of Human Emotions, his widely-praised book that brings bench and bedside together with a detailed, empathic account of his work with patients, in the context of the brain dynamics underlying their difficulties—according to optogenetics.
CARL SHERMAN: Let’s talk about optogenetics. Can you give me a capsule version of just what it is?
KARL DEISSEROTH: Optogenetics is a way of using light to study how a biological system works. But it’s the opposite of how we normally use light, which is to collect information, to look at something and see what happens. Instead, optogenetics uses light to control what happens.
The way we do this is make some cells responsive to light, so we can turn them on or off with it. Then we can see what kinds of activity, what kinds of cells, and what connections between cells actually matter for the mysterious and wonderful things that the brain accomplishes; for sensation, cognition, action.
More specifically, how do you transform these cells?
DEISSEROTH: We use genes from microbes, single-celled organisms, that for their own reasons have evolved to make light-activated ion channels, little molecules that sit in the cell membrane and move ions—charged particles— across it. They create a current in response to light.
In evolving these beautiful, light-sensitive proteins, these microorganisms have done an important job for us, because we can use genetic tricks with viruses to put their genes into neurons, and neurons use electricity to turn themselves on and off. Then we can guide light into the brain, and only the cells that have this gene will be turned on or off by it, depending on which microbial gene we put in.
It can get very fancy. We can use holographic techniques to guide dozens or hundreds of spots of light to individual cells and control them.
I understand that discoveries by a number of scientists went into the development of this technique, starting with the identification of these light-sensitive proteins 150 years ago. What was your lab’s contribution?
DEISSEROTH: I’m a neuroscientist, but I’m also a biochemist by training. All through my career I’ve taken a molecular approach to systems-level questions. What my lab did was figure out how these molecules work, bridging all the way to their implementation in neuroscience. A lot of real basic science that enabled the applications came later.
First, we discovered the structures of channelrhodopsins, these light-activated proteins, which allowed us to see how they work, and exactly which atoms and which movements are important. That let us redesign them, to change the colors of light that they respond to, and the kind of ions that they let through. Along the way we discovered major new types of channelrhodopsins.
And we built the tools to make optogenetics work—the viral vectors to put these genes into neurons in the brains of mammals, and the fiberoptic methods to guide light and target cells and connections in different parts of the brain.
Just how do you target and activate specific neurons? You’re introducing the viral vector into an area of the brain, yet it only affects certain cells in that area. How do you make this happen?
DEISSEROTH: The virus gets the gene into all the nearby cells, but the genes are not turned into functional protein (channelrhodopsins) in all of them. That step is achieved with bits of DNA called promoters and enhancers, which regulate gene function, and we design the virus so that the protein is only made by the specific, targeted type of cell. We achieve additional specificity by guiding light spots to a subset of the cells in which the protein is made. And then finally, we developed projection targeting, where you deliver the opsin—the light-sensitive protein—to one part of the brain and deliver the light to another.
The reason this works is that the opsin protein fills the whole neuron; it gets trafficked out into the long axons that extend from one part of the brain to another. If you put your fiber optic in that other brain region, the only thing that’s sensitive to light will be the axons that started from where you injected your virus. So you get specificity by connection type.
So you can use optogenetics to discover neural connections that hadn’t been mapped before and delineate them with more precision?
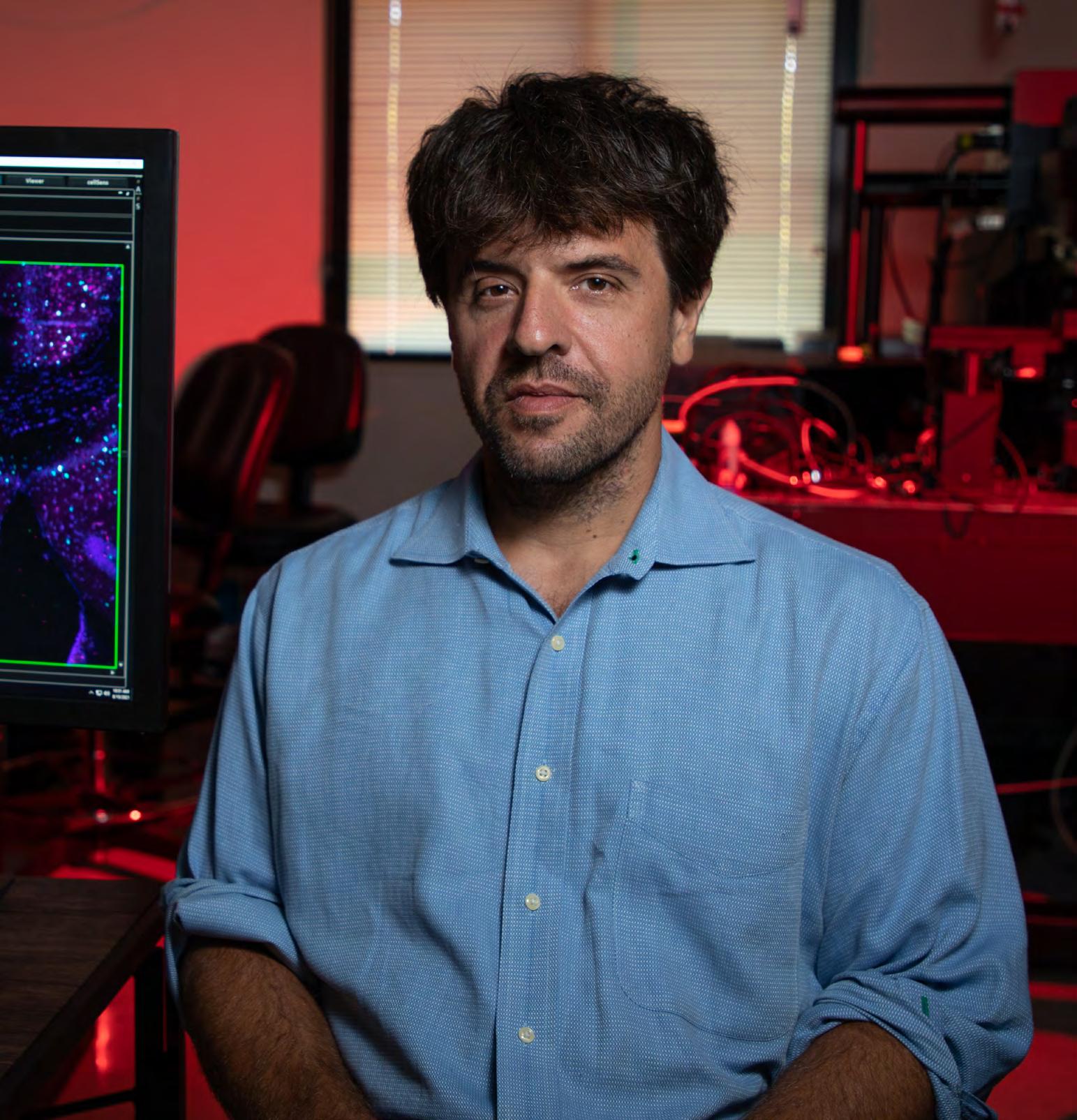
DEISSEROTH: Exactly. You could demonstrate functional connectivity very efficiently, and on different scales. On a local circuit scale, you can zip your light around to all the cells individually or in combination, while listening to or recording activity. You can see which of the cells you stimulate, which types of cells, which locations, which combinations give rise to the activity of interest.
On the other hand, if you test all the cells and combinations of cells with the broad, high-content recording technologies we’ve developed, you can be quite global and unbiased about the kind of information you collect.
Also, there have long been ways to slowly ramp up or ramp down the activity of individual neurons—chemical methods, for example. But with those methods you don’t have naturalistic timing information, which turns out to be critical. With optogenetics you can be very, very rapid and swift.
Why is timing so important?
DEISSEROTH: The brain is designed to work on millisecond time scales. The speed, the brevity of action potential transmission, is important for survival. We see this all over neuroscience. If you give a stimulus the frequency of 10 Hertz, you’ll get a totally different result than if you stimulate at 80 or 100 Hertz.
Could you give me examples how optogenetics has been applied in neuroscience? Some discoveries it’s facilitated, processes it’s clarified, etc.
DEISSEROTH: One thing is anxiety. We all know anxiety; we know it’s got these different parts to it. We know it feels bad. We know it affects our body: It makes us breathe more quickly and our heart beat faster. And we know it affects our choices: When we’re anxious, we don’t do the risky things that might otherwise happen. All these features, that are so different, come on together and go away together. So somehow our brain has built a way to create this state, to make it coherent and unitary, to have anxiety happen and then go away.