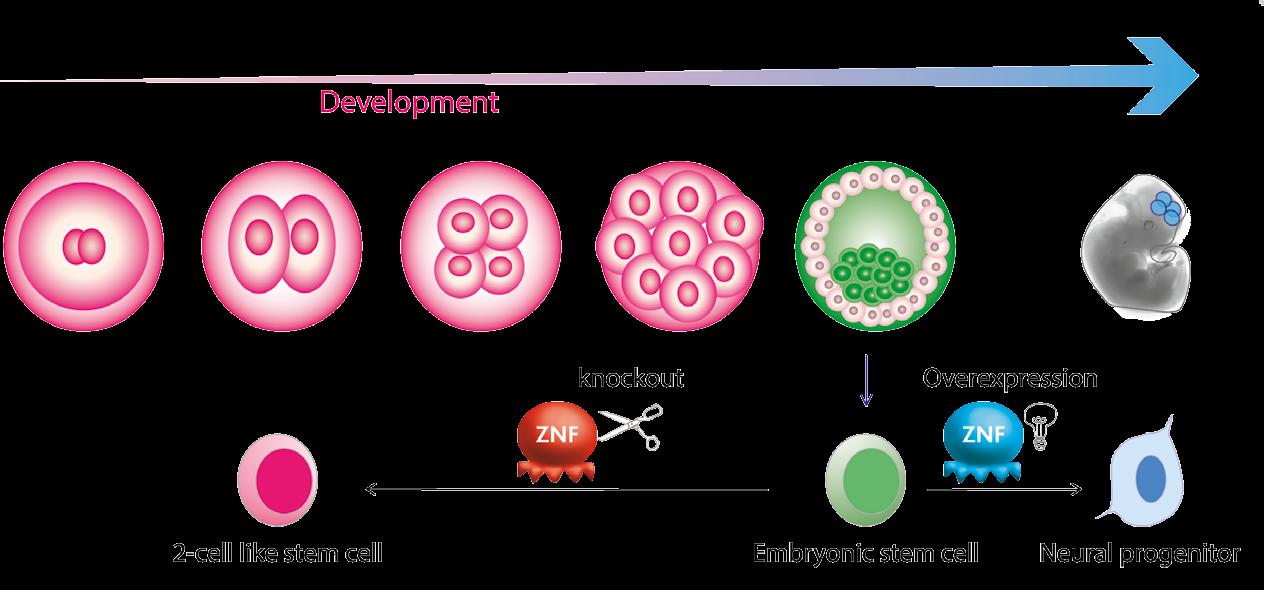
9 minute read
TransposonsReprogram
The making of more powerful stem cells
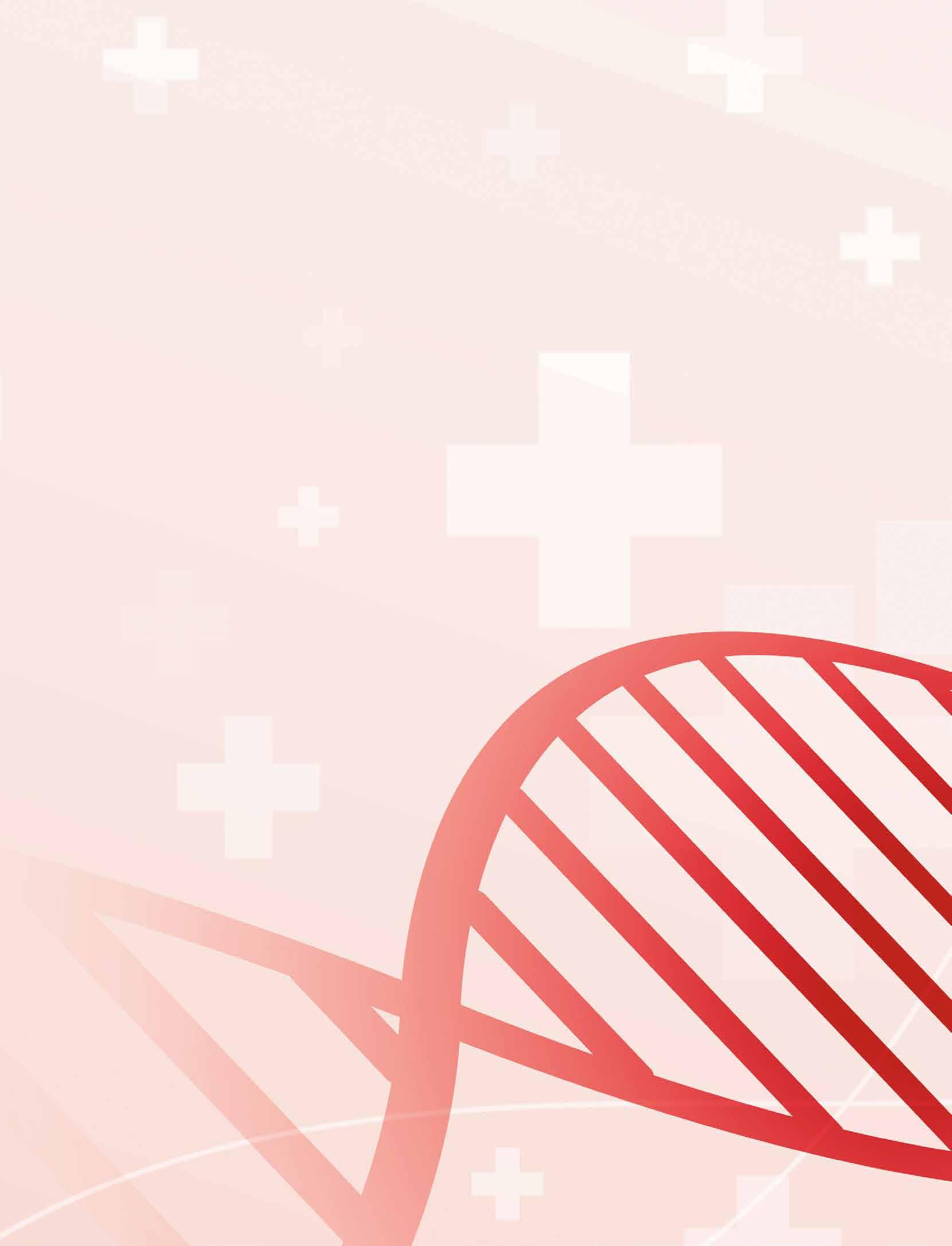
Transposable elements have been inserting new copies of themselves into our genome for millions of years, and today they form a large part of our DNA, yet relatively little is known about them. While most of these transposable elements are no longer mobile, some have the power to immortalize cells by remodelling the genome, as Dr Helen Rowe explains.
Around half of the human genome is made up of transposable elements, including both retrotransposons and DNA transposons, which can be thought of as ancient viruses because they once replicated and inserted new copies of themselves into the genomes of our ancestors. While these elements are abundant in the genome (around half of our DNA), relatively little is known about them. “We don’t really know what’s in there as they are poorly characterised, and we know even less about their function,” says Dr Helen Rowe, Senior Lecturer in Epigenetics at Queen Mary University of London (QMUL). Retrotransposons replicate through an RNA intermediate, an RNA molecule, which is an important difference with DNA transposons. “They can reverse transcribe their RNA into DNA, which means that they can readily expand their copy number by making new copies of themselves to insert at new locations throughout the genome,” explains Dr Rowe. Although these ancient viruses are no longer mobile, many have retained fragments called ‘enhancers’ that can switch on expression of our own genes.
Genome remodelling
As the Principal Investigator of an EU-funded project based at QMUL, Dr Rowe aims to shed new light on the role of retrotransposons in genome remodelling, using embryonic stem cells from mice as a model system. In earlier research, Dr Rowe investigated an epigenetic regulation pathway of these transposable elements. “We know the components of that pathway are transcription factors, proteins that bind to DNA sequences embedded in these retrotransposons. This is a novel class of transcription factors that are still mostly uncharacterised,” she
outlines. Dr Rowe and her colleagues have been studying this novel class of proteins – called zinc finger proteins (ZFPs) – which could lead to new insights into the functions of some of these transposable elements. “A huge number of those ZFPs are encoded by the human and mouse genome,” she says.
A very small proportion of these ZFPs have been characterised, a topic at the heart of the project’s overall agenda. The Rowe lab are focusing on ZFPs that bind to retrotransposons that are present at hundreds of copies in the mouse genome. Researchers are using a technique called CRISPR/Cas9 to edit the genome. “We can basically edit the DNA responsible for making these ZFPs, thereby preventing their function by generating a gene ‘knockout’. This approach helps us to dissect the function of the retrotransposons that these ZFPs bind to and usually switch off,” explains Dr Rowe.
We’re looking at zinc finger proteins that alter the chromatin landscape, and they bind to specific DNA sequences embedded within retrotransposons. We can map their binding sites using chromatin profiling techniques such as ‘Chromatin Immunoprecipitation-sequencing’ and ‘CUT&RUN’ .
The focus of attention here is on two specific transcription factors, ZFP37 and ZFP819. “We’ve got one PhD student, Liane Fernandes leading the project on ZFP819, and a Postdoctoral Fellow, Poppy Gould leading the project on ZFP37,” continues Dr Rowe. A second Postdoctoral Fellow, Rocio Enriquez-Gasca, is working on both projects, pioneering novel computational pipelines, which are essential to the analysis of sequence data derived specifically from retrotransposons. “We can specifically target the genes that encode those proteins
Transposons control genes encoding master transcription factors.
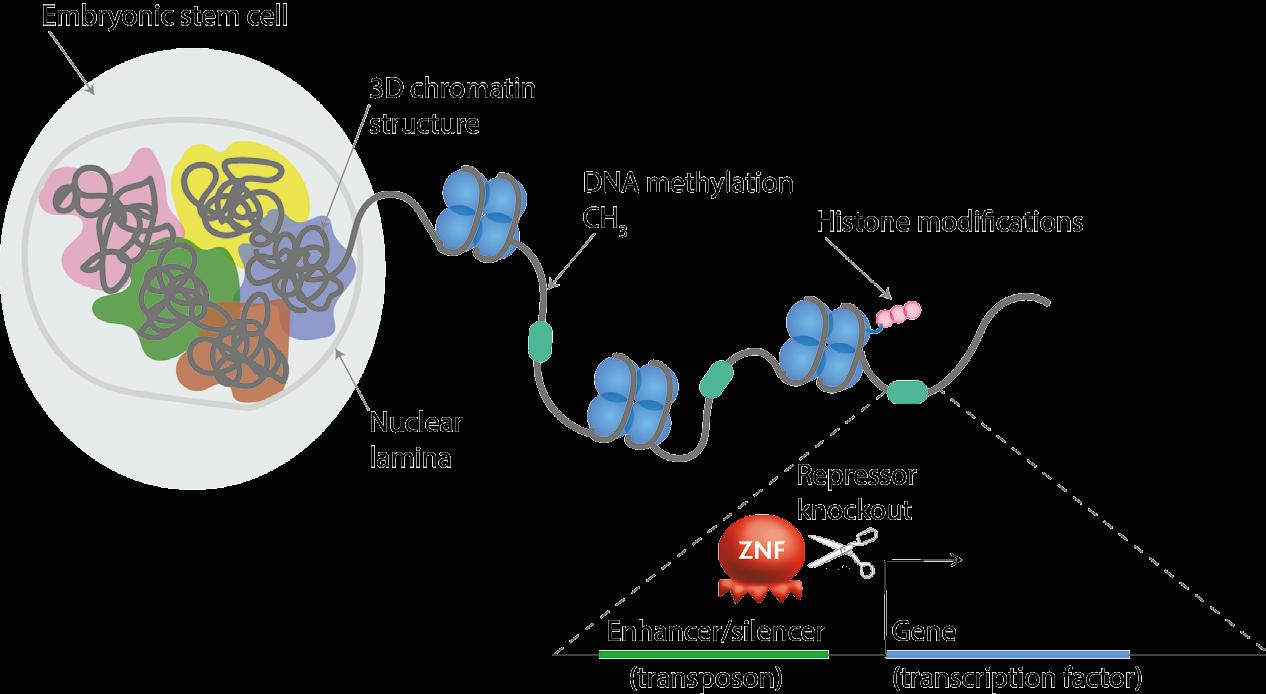
using CRISPR/Cas9 technology. We can direct sequence-specific RNAs to certain DNA sites and knockout the function of these proteins.”
The project is based in mouse embryonic stem cells, which are derived from the blastocysts of early embryos, and which can grow in a petri dish. These cells are ‘pluripotent’, meaning they can self-renew and differentiate into the different cell types of the body; now Dr Rowe and her colleagues are looking at the role of the ZFPs in genome remodelling. “We’re looking at whether – when we knockout genes encoding those ZFPs – these cells either differentiate or become reprogrammed to reflect an earlier stage of development, where they adopt a ‘totipotent’ rather than pluripotent state,” she outlines. This essentially means that the cells become more powerful stem cells because totipotent cells have the ability to produce all of the specialized cell types in an organism, including within the embryo and the placenta. “We’re looking at how embryonic stem cells can be reprogrammed, changing their potency, by reactivating the retrotransposons,” says Dr Rowe.
A variety of different techniques are being used in this research, with Dr Rowe and her colleagues looking at different stages of development, using their stem cell model. A lot of energy is centered on investigating the master regulators at each stage of development. “We look at the expression of these master regulators, as well as at the morphology of the cells. We use standard techniques such as Western blot, immunofluorescence and RNA sequencing, where you sequence all the RNA in a cell to identify the main determinants of change,” says Dr Rowe. Chromatin-based techniques are a fundamental tool in Dr Rowe’s research. “We’re looking at ZFPs that alter the chromatin landscape, and they bind to specific DNA sequences embedded within retrotransposons. We can map their binding sites using chromatin profiling techniques such as ‘Chromatin Immunoprecipitation-sequencing’ and ‘Cleavage Under Targets & Release Using Nuclease’ (CUT&RUN)” she continues.
Chromatin-based techniques enable scientists to capture a protein of interest, which here is the ZFPs, and then sequence all the DNA associated with it. This is how the retrotransposons targeted by the ZFPs of interest have been identified. “They don’t target genes, they actually target retrotransposons. We think that’s because they evolved originally to block the spread of these genome invaders, by targeting their regulatory sequences such as enhancers. But now, they regulate host genes located next-door to the retrotransposons,” outlines Dr Rowe. They do this by recruiting heterochromatin to the retrotransposon, which then spreads into host genes and regulates their function. “ZFP819 binds to a satellite repeat – which is a different class of transposable element – and recruits heterochromatin to it. This switches it off, and all the nearby genes,” explains Dr Rowe. “The main genes that are associated with that satellite repeat are the master regulators of totipotency.”
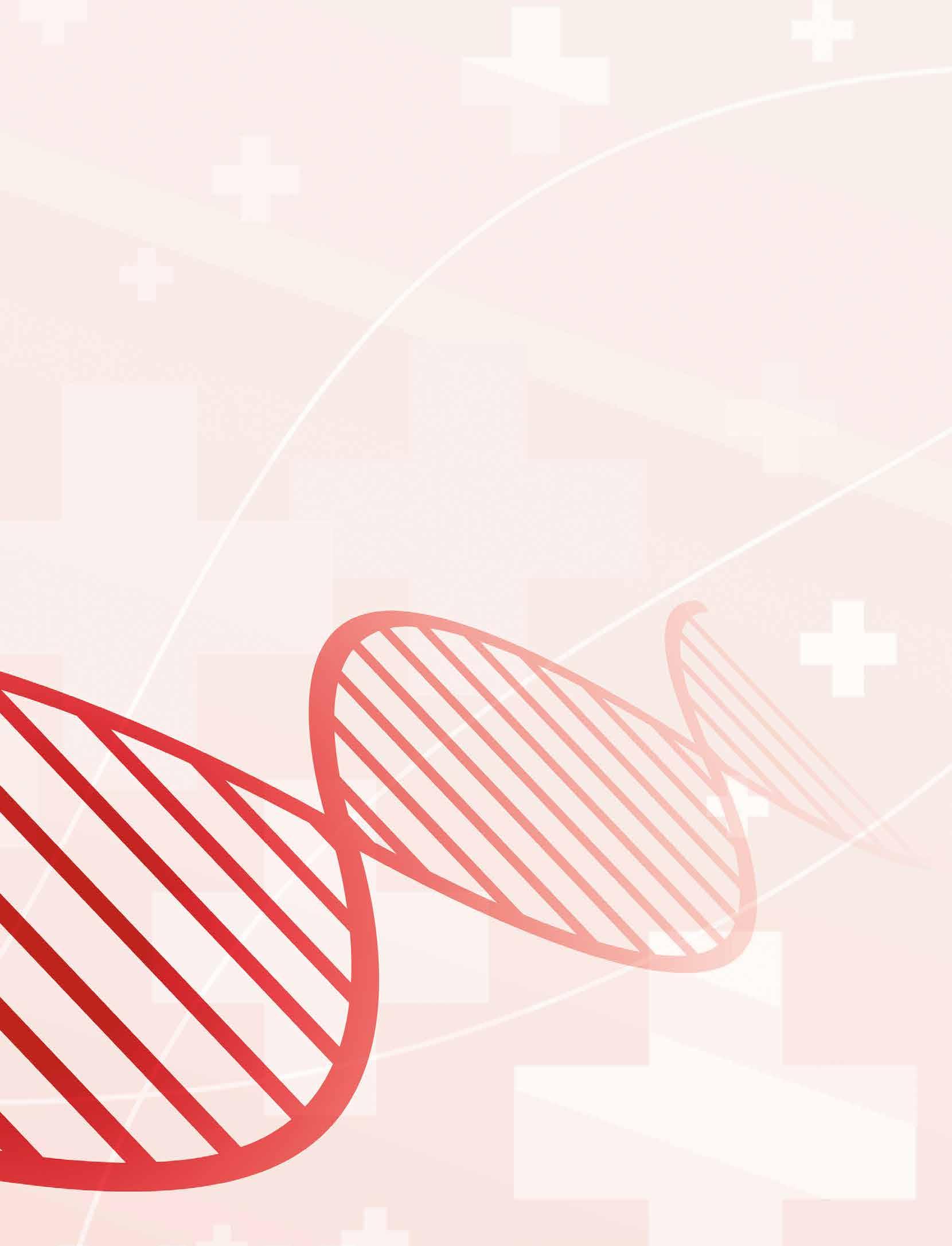
Project researchers (left to right): Rocio Enriquez-Gasca, Liane Fernandes, Helen Rowe, Poppy Gould, James Holt, Hale Tunbak.
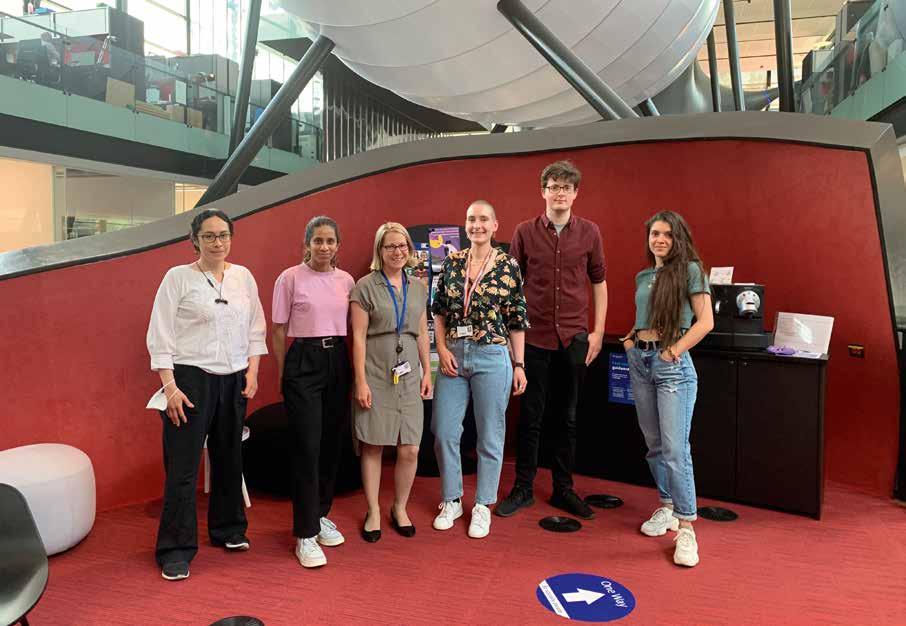
How retrotransposons remodel the genome during early development and reprogramming Project Objectives
This work in TransposonsReprogram will exploit mouse development to unravel the mechanism of how RTNs remodel the genome. It will help us to understand how ZFPs can be engaged to reprogram cells and in stem-cell therapies, and will explain more broadly how RTNs, which dominate our genomes, control cell fate.
Project Funding
TransposonsReprogram is funded by the European Union’s Horizon 2020 research and innovation programme under Grant agreement ID: 678350.
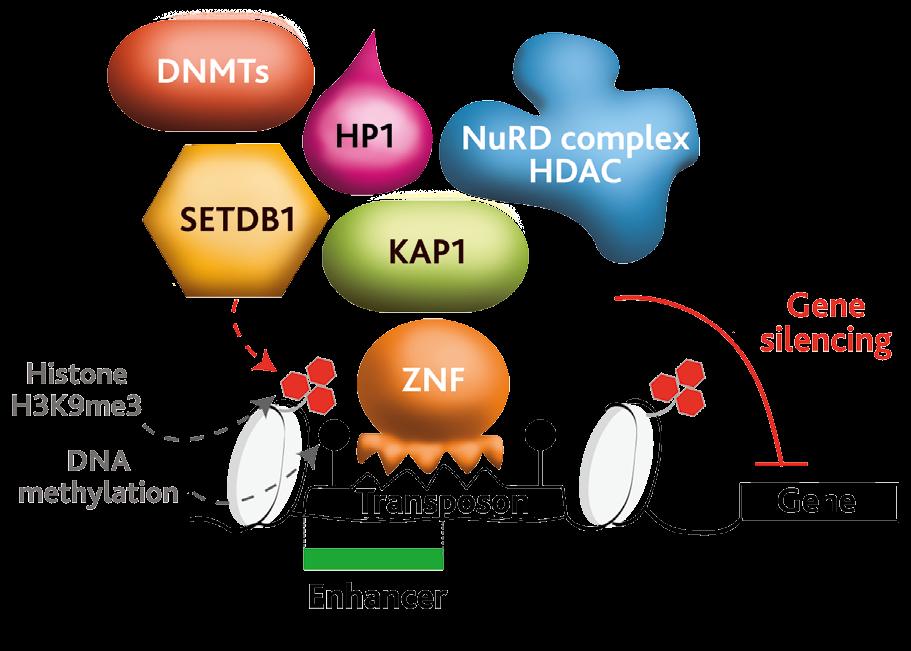
Scientific Collaborators
• George Kassiotis, The Francis Crick Institute, UK • Didier Trono, EPFL, Switzerland
Contact Details
Dr Helen M Rowe Group Leader Centre for Immunobiology Blizard Institute Queen Mary University of London 4 Newark St, London E1 2AT, UK E: h.rowe@qmul.ac.uk W: https://www.qmul.ac.uk/blizard/allstaff/profiles/helen-rowe.html
Dr Helen M Rowe
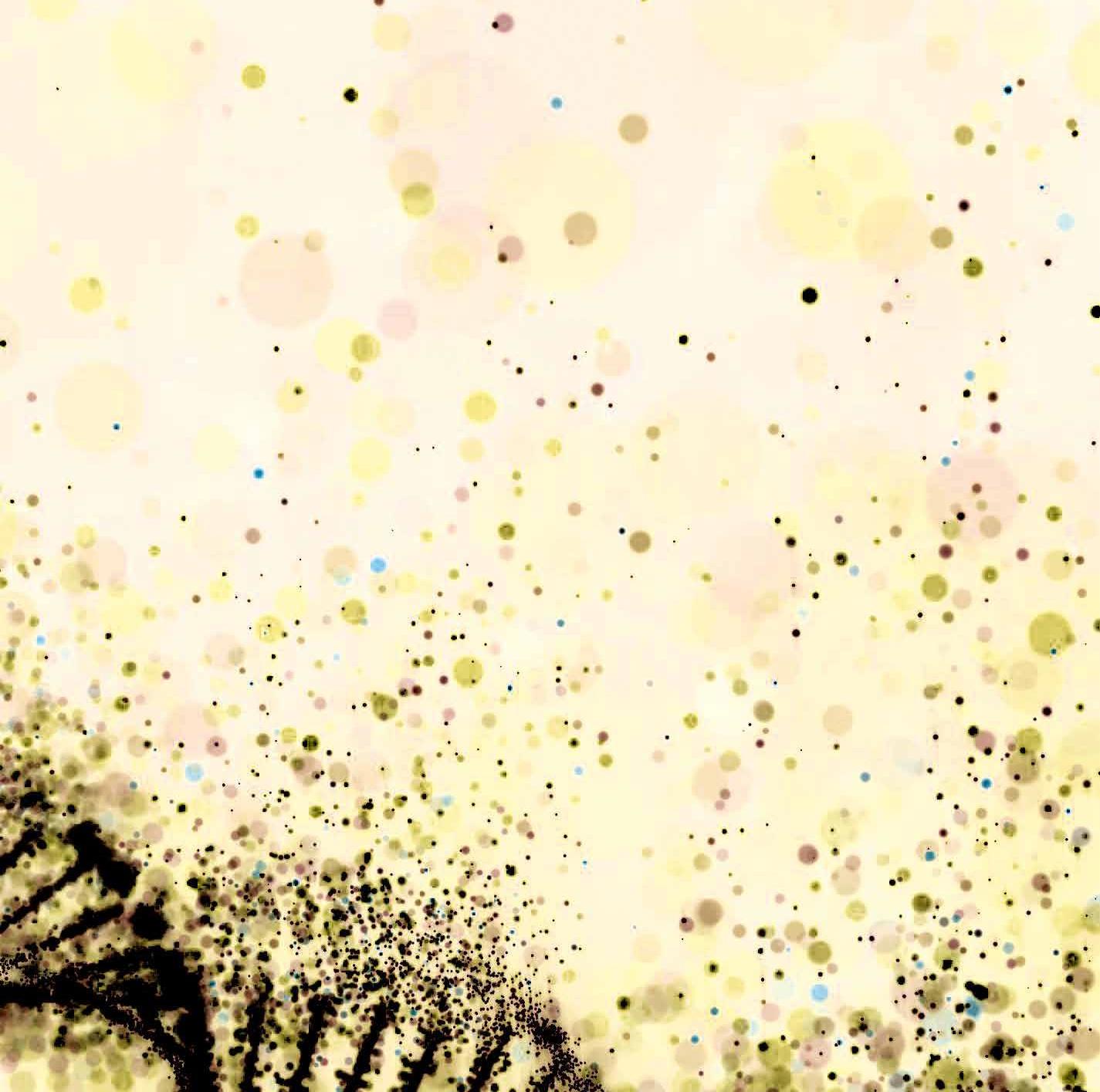
Dr Helen Rowe is Senior Lecturer in Epigenetics at the Centre for Immunobiology, part of Queen Mary University of London. Her lab is working on how epigenetic complexes regulate transposons and cellular genes in development, and how these pathways can go wrong in cancer and autoinflammatory diseases.
Researchers have found that ZFP819 and this satellite repeat are able to influence cell fate, because ZFP819 expression coincides with exit from totipotency. ZFP819 knockout leads to reactivation of the satellite repeats and the master regulators of totipotency. However, Dr Rowe says they have less information about ZFP37. “It’s expressed a bit later in development, so it comes in later and then it binds to a different retrotransposon, and that regulates a different developmental transition,” she says. There are over 300 of these ZFPs in the mouse and human genome, and the project provides solid foundations for further research into this protein family. “In the project, we’ve been looking to define new concepts and see how they could apply to other members of this ZFP family,” explains Dr Rowe. “We’ve been able to demonstrate that these retrotransposons and ZFPs do re-model the genome, and we believe this will apply to many other transposable elements within the genome.”
The next step beyond this is to understand how transposable elements exert their genome re-modelling effects. While the Rowe lab have made significant progress in terms of proving their hypothesis that retrotransposons direct cell fate transitions, Dr Rowe now aims to gain further mechanistic data. “We want to understand how these elements work,” she stresses. Initial findings suggest that these transposable elements are involved in regulating chromatin structure, now researchers are probing deeper. “We’re looking at things like DNA methylation, 3D chromatin structure, histone methylation and the accessibility of chromatin,” continues Dr Rowe. “Those things combined will help us to understand if these repetitive elements are simply regulatory elements, or if they are also structural elements controlling 3D chromatin organisation.”
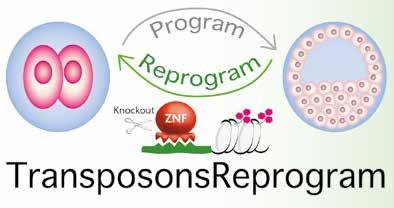
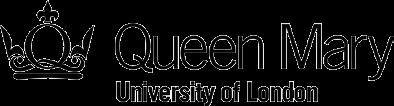
Stem cell therapies
This research also holds important implications for stem cell therapies. It is very difficult to manipulate cells and direct them into different differentiation pathways, and Dr Rowe says the project’s research could help provide a more detailed picture. “You usually start with human induced pluripotent stem (IPS) cells, which are similar to embryonic stem cells. Our findings would relate to that model, where you then would potentially be able to make patientspecific human IPS cells more efficiently,” she outlines. Those cells can then be directed down different differentiation pathways, depending on the patient’s needs; safety is a primary consideration here, and it’s important to consider the impact of a therapy that affects transposable elements. “The impact of cell fate changes on transposable elements is largely unknown. We’re trying to understand how these ancient viruses fit in,” says Dr Rowe.
The project’s research could help make stem cell therapies more efficient and ensure they are safe, and that they do not lead to the reactivation of transposable elements that might have unwanted side effects. Researchers have used mice as a model system so far, and in future Dr Rowe hopes to conduct similar experiments in a human system. “We would use human IPS cell cultures, which we can grow in petri dishes in the lab. Then we would look at their cell fate,” she outlines, after gene knockouts of human ZFPs. In future, Dr Rowe plans to pursue further research into how transposable elements mediate structural changes to chromatin, building on findings so far. “We’ve found that these transposable elements regulate developmental fate. They recruit heterochromatin, they act as enhancers, they mediate structural changes to chromosome compartments,” she says. “We are excited to find out more about what transposable elements can do and how.”