
9 minute read
How Sensory Gating May Aid in Better Perception of Speech in Noise
Typically developing children with typical hearing have more difficulty understanding speech in background noise than typical-hearing adults. This difference may be due to development of the central auditory pathways, as peripheral audition, which is measured with usual hearing evaluations, does not differ between children and adults, and the timelines between central auditory development and speech perception-in-noise (SPiN) ability are similar. For instance, children around the ages of 10 to 12 years begin to have adult-like SPiN skills, an age at which central auditory systems also begin to become adult-like.
One central auditory function that is also developing during this period is sensory gating. Sensory gating acts as an automatic “filter” that may suppress noise before reaching levels of attention. Indeed, my team and I have previously found that typical-hearing adults who struggle in background noise also tend to have worse gating function. Therefore, the development of gating may contribute to maturational trends in SPiN.
Advertisement
With this in mind, we measured sensory gating function in adults ages 22 to 24 years and children ages 5 to 8 years using cortical auditory evoked potentials (CAEPs) recorded via high-density electroencephalography. From this data, we were able to model the cortical gating response in both the children and adults, showing distinct developmental central auditory networks. Our results appeared in the Journal of Speech, Language, and Hearing Research in January 2023.
We also measured SPiN ability using a clinical measure, the BKB-SiN, which provides the signal-to-noise ratio necessary to correctly perceive 50 percent of the words in given sentences. While both SPiN and gating function were significantly decreased in children, there was no significant relationship between gating function and SPiN in the children.
When these results are considered with our previous, similar study conducted in typical-hearing adults with SPiN ability ranging from typical to moderate deficits, it may be the case that sensory gating only underlies clinical SPiN deficits. For instance, the child group in our 2022 study, while having decreased SPiN ability, still performed within age-appropriate limits. Similarly, in the adult group in our 2020 study, there was no correlation between gating function and SPiN ability when performance was within typical limits.
In this image in the Journal of Speech, Language, and Hearing Research, a portion of the study’s cortical auditory evoked potential (CAEP) gating current density reconstructions are shown; yellow indicates the most likely area of activation.
In other words, worse gating appears to be a significant factor only when SPiN performance is outside of typical limits. In future studies, we aim to examine sensory gating function in typical-hearing children with clinically low SPiN outcomes. Taken together with our other research, these studies can help test the hypothesis that tinnitus perception may arise from faulty “gating mechanisms” in the brain. This study is important because faulty gating mechanisms, also observed in tinnitus and hearing loss, appear to play a role in auditory disorders.
—Julia Campbell, Ph.D., Au.D.
A 2016 ERG scientist generously funded by the Les Paul Foundation, Julia Campbell, Ph.D., Au.D., CCC-A, FAAA, is an assistant professor of communication sciences and disorders in the Central Sensory Processes Laboratory at the University of Texas at Austin.
Hearing Involves Highly Distorted Processing of Sound by Sensory Hair Cells
Audiophiles typically seek out sound systems that most accurately reproduce acoustic recordings and add minimal distortion. Interestingly, our sense of hearing does not work in quite such a transparent way. In fact, a considerable amount of distortion is introduced by the sensory hair cells within the inner ear.
These cells—the inner and outer hair cells (see figure opposite page)—are responsible for converting soundevoked vibrations of the surrounding structures into electrical signals that are transmitted to the brain. Distortion results from the fact that this conversion process is highly nonlinear (i.e., the relationship between vibration and the resulting electrical signal follows a sigmoidal curve and not a straight line).
Studying the distortions generated within the inner ear tells us how these signals may influence our perception of sound and also provides insight into the processes that are involved in basic sensory hair cell function. This function is very difficult to study in live ears, as the auditory sensory organ—the organ of Corti—is located deep within the temporal bone.
To better understand the distortion that sensory hair cells generate, I used an imaging technique called optical coherence tomography (OCT) to see into the mouse inner ear and measure vibrations of the hair cells in response to sound. While OCT is commonly used to examine the health of the human retina, it is also powerful enough to see through the bone encasing the inner ear in mice, allowing me to visualize how the hair cells vibrate. Since the outer hair cells, in particular, use their electrical signals to drive the generation of mechanical forces, any distortions in the electrical signals are converted into vibrations of the cells and surrounding structures, where they can be detected using OCT.
By presenting two tones to the mouse ear, one at a frequency f1 and the other at a frequency f2, I was able to study vibratory distortions at mathematically related frequencies like f2-f1 and 2f1-f2. These distortion frequencies are of interest as they theoretically depend on different properties of the underlying nonlinear function. While the 2f1-f2 distortion is often assumed to be largest and has been most widely studied, I actually found that f2-f1 was much larger for a wide variety of stimulus frequencies and amplitudes.
Remarkably, the distortions were sometimes as large as the responses at the frequencies of the stimulus tones themselves. The resolution and sensitivity of the OCTbased approach allowed me to characterize how the distortions were shaped as they were transmitted from the outer hair cells to the surrounding basilar and tectorial membranes, and how they traveled to different inner ear locations, just like the vibrations that were produced by sounds directly presented to the ear.
The findings, published in the Journal of the Acoustical Society of America (JASA) Express Letters in November 2022, clarify how distortions are produced by the outer hair cells and how these additional signals may influence peripheral sound encoding.
Analysis of the distortions also allowed estimation of the nonlinear function that determines how the cells respond to sound, which so far has primarily been studied in vitro. Since the forces generated by outer hair cells serve to amplify the stimulation of the inner hair cells, which are the main communicators of auditory information to the brain, the findings also reveal aspects of the physical processes that are required for sensitive hearing.
—James Dewey, Ph.D.
This schematic cross-section from JASA Express Letters shows the organ of Corti in the inner ear, which contains the sensory inner and outer hair cells. Vibrations of the hair-like structures at the tops of the cells are converted to distorted electrical signals. Outer hair cells generate force in response to this electrical signal, causing distorted vibrations of the surrounding structures.
Support our research: hhf.org/donate.
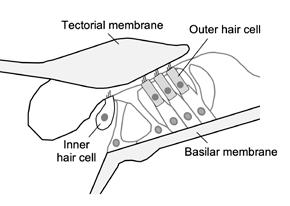
A Unique, Fast Inner Ear Synapse Keeps Us From Falling
In a discovery more than 15 years in the making, a small group of neuroscientists, physicists, and engineers from several institutions has unlocked the mechanism of the synapses, paving the way for research that could improve treatments for vertigo and balance disorders that affect as many as 1 in 3 Americans over age 40.
The study published in the Proceedings of the National Academy of Sciences in January 2023 describes the workings of “vestibular hair cell-calyx synapses,” which are found in organs of the innermost ear that sense head position and movements in different directions.
“Nobody fully understood how this synapse can be so fast, but we have shed light on the mystery,” says Robert Raphael, Ph.D., a Rice University bioengineer who coauthored the study with the University of Chicago’s Ruth Anne Eatock, Ph.D., the University of Illinois Chicago’s Anna Lysakowski, Ph.D., current Rice graduate student Aravind Chenrayan Govindaraju, and former Rice graduate student Imran Quraishi, M.D., Ph.D., now an assistant professor at Yale University.
Synapses are biological junctions where neurons can relay information to one another and other parts of the body. The human body contains hundreds of trillions of synapses, and almost all of them share information via quantal transmission, a form of chemical signaling via neurotransmitters that requires at least 0.5 milliseconds to send information across a synapse.
Prior experiments had shown a faster, “nonquantal” form of transmission occurs in vestibular hair cell-calyx synapses, the points where motion-sensing vestibular hair cells meet afferent neurons that connect directly to the brain. The new research explains how these synapses operate so quickly.
In each, a signal-receiving neuron surrounds the end of its partner hair cell with a large cuplike structure called a calyx. The calyx and hair cell remain separated by a tiny gap, or cleft, measuring just a few billionths of a meter.
“The vestibular calyx is a wonder of nature,” Lysakowski says. “Its large, cup-shaped structure is the only one of its kind in the entire nervous system. Structure and function are intimately related, and nature obviously devoted a great deal of energy to produce this structure. We’ve been trying to figure out its special purpose for a long time.”
From the ion channels expressed in hair cells and their associated calyces, the authors created the first computational model capable of quantitatively describing the nonquantal transmission of signals across this nanoscale gap. Simulating nonquantal transmission allowed the team to investigate what happens throughout the synaptic cleft, which is more extensive in vestibular synapses than other synapses.
“The mechanism turns out to be quite subtle, with dynamic interactions giving rise to fast and slow forms of nonquantal transmission,” Raphael says. “To understand all this, we made a biophysical model of the synapse based on its detailed anatomy and physiology.”
The model simulates the voltage response of the calyx to mechanical and electrical stimuli, tracking the flow of potassium ions through low-voltage-activated ion channels from presynaptic hair cells to the postsynaptic calyx.
Raphael says the model accurately predicted changes in potassium in the synaptic cleft, providing key new insights about changes in electrical potential that are responsible for the fast component of nonquantal transmission; explained how nonquantal transmission alone could trigger action potentials in the postsynaptic neuron; and showed how both fast and slow transmission depend on the close and extensive cup formed by the calyx on the hair cell.
“The key capability was the ability to predict the potassium level and electrical potential at every location within the cleft,” Eatock says. “This allowed the team to illustrate that the size and speed of nonquantal transmission depend on the novel structure of the calyx. The study demonstrates the power of engineering approaches to elucidate fundamental biological mechanisms, one of the important but sometimes overlooked goals of bioengineering research.”
Quraishi began constructing the model and collaborating with Eatock in the mid-2000s when he was a graduate student in Raphael’s research group and she was on the faculty of Baylor College of Medicine, just a few blocks from Rice in Houston’s Texas Medical Center.
Quraishi’s first version of the model captured important features of the synapse, but he says gaps in “our knowledge of the specific potassium channels and other components that make up the model was too limited to claim it was entirely accurate.”
This illustration and microscopic images from Rice University show the relationship between motion-sensing vestibular hair cells (blue) of the innermost ear and the cup-shaped “calyx” (green) structures of adjoining nerves that connect directly to the brain. The rapid flow of information through the synapses helps stabilize balance and vision in humans and many other animals.
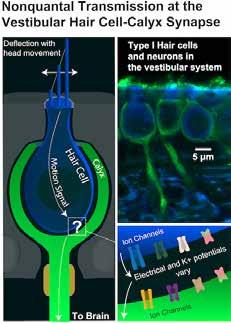
Since then, Eatock, Lysakowski, and others discovered ion channels in the calyx that transformed scientists’ understanding of how ionic currents flow across hair cell and calyx membranes.
“The unfinished work had weighed on me,” Quraishi says, and he was both relieved and excited when Govindaraju, a doctoral student in applied physics, joined Raphael’s lab and resumed work on the model in 2018.
“By the time I started on the project, more data supported nonquantal transmission,” Govindaraju says. “But the mechanism, especially that of fast transmission, was unclear. Building the model has given us a better understanding of the interplay and purpose of different ion channels, the calyx structure, and dynamic changes in potassium and electric potential in the synaptic cleft.”
“One of my very first grants was to develop a model of ion transport in the inner ear,” Raphael says. “It is always satisfying to achieve a unified mathematical model of a complex physiological process. For the past 30 years—since the original observation of nonquantal transmission—scientists have wondered, ‘Why is this synapse so fast?’ and, ‘Is the transmission speed related to the unique calyx structure?’ We have provided answers to both questions.”
Raphael says the link between the structure and function of the calyx “is an example of how evolution drives morphological specialization. A compelling argument can be made that once animals emerged from the sea and began to move on land, swing in trees, and fly, there were increased demands on the vestibular system to rapidly inform the brain about the position of the head in space. And at this point the calyx appeared.”
Raphael says the model opens the door for a deeper exploration of information processing in vestibular synapses, including research into the unique interactions between quantal and nonquantal transmission.
He adds that the model could also be a powerful tool for researchers who study electrical transmission in other parts of the nervous system, and he hopes it will aid those who design vestibular implants, neuroprosthetic devices that can restore function to those who have lost their balance. —Jade Boyd
This originally appeared on the Rice University Office of Public Affairs website. A 2022 ERG scientist, Robert Raphael, Ph.D., is an associate professor of bioengineering in Rice’s George R. Brown School of Engineering. He is also a 2007 ERG scientist. A former member of HHF’s board of directors and a 1987–88 and 1994 ERG scientist, Ruth Anne Eatock, Ph.D., is a professor of neurobiology at the University of Chicago.
For references, see hhf.org/spring2023-references.
This sponsored page shows current trends in technology.