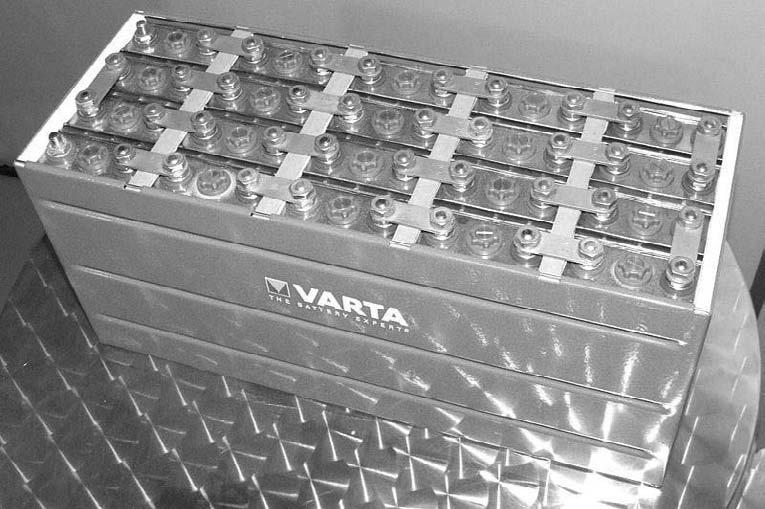
6 minute read
Future Batteries: The Big Picture
While lead and sulfuric acid would not be my initial choice for any construction project—one of the heaviest elements mated with one of the nastiest compounds— reliability, performance, and cost all weigh heavily in the lead-acid battery’s favor. In fact, lead-acid’s suitability for so many applications has greatly diminished even the need to search for alternatives.
But the recent save-the-environment, reduce-oil-dependency, and let’s-try-electricvehicles changed consciousness altered the pattern. Government, industry, and university laboratories all over the planet reflect this change. Pouring money on a problem never guarantees a solution, but it does guarantee that a lot more will be happening, and that some of what happens will be usable and good. Let’s look at future battery trends starting with the consortium that’s pushing the outside of the battery envelope—the USABC.
In 1991, Ford, General Motor, and Chrysler (joined by the Department of Energy and the Electric Power Research Institute) had a better idea—the United States Advanced Battery Consortium (USABC). In short, the Electric and Hybrid Vehicle Research, DevelopmentandDemonstrationActof1976recognizedtheneedforbatterydevelopment; the Department of Energy defined and funded it; and the USABC focused the efforts.
The near-term result was that a plethora of projects was honed down to just three high-energy battery research areas that could deliver significant vehicle range and power advantages: lithium polymer, lithium metal sulfide, and nickel metal hydride. Some of these batteries are considered the best of the best battery technologies on the market.
Future Batteries: The Big Picture
Table 8-5, adapted from an SAE paper, shows the entire story at a glance. Notice that 11 different battery technologies are on the list, and they are not equal. In very general terms, higher energy density and power density are desirable and look easy to do—on paper. Getting both at the same time, along with high cycle-life and low cost in a battery that operates efficiently over a range of temperatures, can be manufactured and supported by infrastructure, and causes no harm to people or the environment, has proven to be a bit more elusive. Notice that none of the batteries developed thus far— even the tried and proved lead-acid battery—even approaches its theoretical specific energy value. We still have a long way to go.
Lead-Acid
The big money involved in the lead acid battery business made some major improvements to the lead-acid batteries of the early 2000s superior to their 1990s counterparts. Along the way to higher specific energy and specific power, lead-acid batteries evolved to add sealed as a regular option. While it is higher in cost, it is less efficient (versus convenience of not watering). The flow-through (the conventional type you’re accustomed to) has improved by greater plate thickness, improved separators, and higher specific gravity electrolyte solution). The other options available are tubular (electrode improvement) and gelled (electrolyte improvement, which I have used in some of the electric vehicles I have worked with).
Nickel-Cadmium
Close on the heels of lead-acid today, this battery type promises to be even better in the future. Its advantages over lead-acid today (less pronounced decrease in capacity under
Battery Type Lead Acid Nickel Cadmium Nickel Metal Hydride Nickel Zinc Nickel Iron Nominal Cell Operating Theoretical 3 Hr Rate Energy Specific Power Power Density
Voltage Temp Range Life Spec Energy Spec Energy Density 30 sec pulse 30 sec pulse (volts) (degrees C) Cycles (wh/lb) (wh/lb) (kwh/cu ft) (watts/lb) (kw/cu ft) 2.1 35 – 70 600 79.5 15.9 2.55 72.7 8.50
1.25 30 – 50 2000 99.1 25.0 3.40 86.4 9.35
1.4 20 – 60 600 84.1 1.6 40 – 65 250 155.0 29.5 27.3 4.96 2.83 68.2 59.1 11.33 2.83
1.25 40 – 80 800 121.4 22.7 3.40 52.3 6.51
Sodium Sulfur
2.08 300 – 400 350 345.5 Sodium Nickel Chloride 2.59 250 – 350 1000 360.0 38.6 59.1 3.26 4.81 54.5 76.3 5.10 6.37
Zinc Bromine 1.8 0 – 45 500 194.5 31.8 1.98 38.6 3.26
Zinc Air
1.62 25 – 65 70 595.5 Lithium Iron Disulfide 1.66 400 – 450 500 295.5 59.1 75.0 1.84 6.80 22.7 170.5 1.84 1.56
Lithium Polymer 3.5 0 – 100 300 248.2 72.7 7.36 90.9 5.95
Table 8-5 Comparison of Future Electric Vehicle Battery Trade-Offs
high-discharge currents, higher cycle-life, slower self-discharge rate, better long-term storability, and improved low-temperature performance) will continue to provide markets to fund development aimed at improving its disadvantages over lead-acid: higher cost and environment-related cadmium issues.
Nickel Metal Hydride
The environmentally benign alter ego to the nickel-cadmium battery is also flat-out superior to it in specific energy and specific power comparisons, and should become the preferred alkaline battery of the future (Figure 8-8). The USABC certainly thought so and is invest its research and development dollars toward this technology.
In addition, when talking about nickel metal hydride batteries, Ron Freund’s study in the next section tells everyone how mid-range battery packs stand the test of time. Personally, the RAV4 EV was the most reliable EV to get me from White Plains, New York to lower Manhattan and back to White Plains, New York. That should tell it all, but let’s hear about Ron’s report of his experience with the RAV4.
Lithium Polymer
This battery’s specific energy and specific power numbers evoke nothing but envy from its competitors and mouth-watering anticipation from its advocates. The whole lithium group has shown great promise in the labs, and smaller lithium polymer batteries have greatly impressed users in the computer industry. But lithium still has to deliver on its promise when packaged in the giant, economy, suitable-for-powering-EVs size (see Figure 8-9). Stay tuned for future developments here—the USABC certainly will.
Figure 8-8 Nickel metal hydride battery
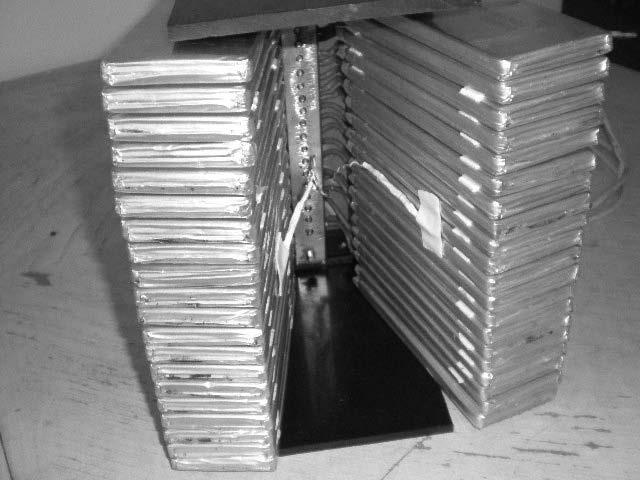
Figure 8-9 Lithium batteries ready to go into a P orsche for great speed and great range (Courtesy of EVPorsche.net).
Sodium-Sulfur
This technology has strong proponents in England, Germany, Japan, Canada, and the United States. This is good because its opponents have a continuous field day merely by labeling it and using scare tactics. Yes, sodium is combustible in air. Yes, sulfur is also on the head of matches. Yes, maintaining a “thermos bottle” at 350 degrees C to contain its molten sodium and sulfur electrodes and beta alumina electrolyte is inefficient. Yes, it can explode and/or do nasty things if punctured in an accident. So can internal combustion engine vehicles. On the other hand, it continues to be one of the most promising advanced battery systems for EV propulsion; its specific energy and specific power numbers are greatly superior to those for lead-acid batteries; and pilot plant battery production has already started. While many problems remain to be solved, perhaps low-cost production the largest among them, sodium sulfur is a hot technology in more ways than one.
Sodium Metal Chloride
If sodium sulfur is great, then all its advantages at a lower operating temperature with still better specific energy and specific power numbers has to be greater yet. And sodium metal chloride battery technology is. Throw in cells that can be assembled in the discharged state, have higher open-circuit voltage and better freeze/thaw and failuremode characteristics than sodium sulfur’s, and you have a real winner.
Lithium Iron Disulfide
The promise of lithium-iron disulfide batteries on the high temperature side is equally mouth-watering. This battery’s specific energy numbers are the best of all, and its specific power numbers simply leave all others in the dust.