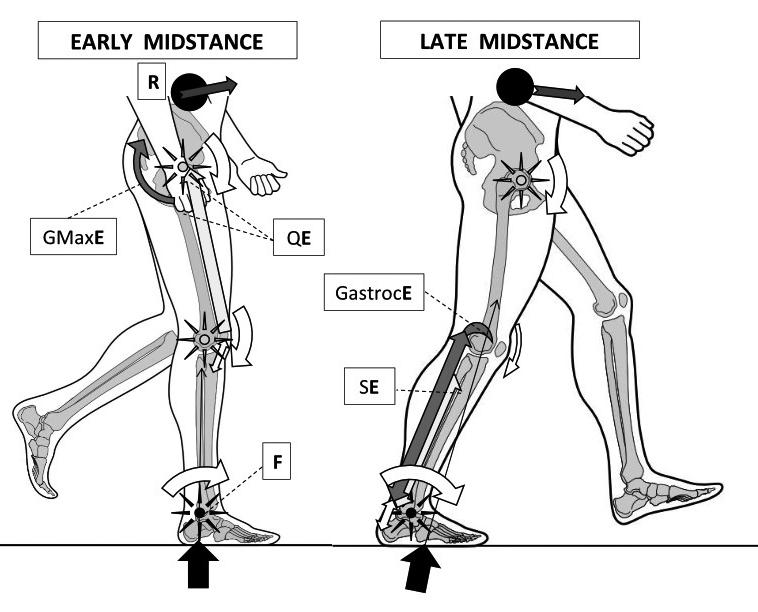
15 minute read
4 page CPD article
Essentials of Biomechanics
Principles of gait: The foot’s option’s at Acceleration
Advertisement
By Andy Horwood
Visiting Lecturer & Fellow Staffordshire University Product Designer & Research at Healthy Step Ltd
INTRODUCTION
Acceleration from the foot usually involves changing its material properties from a structure that dissipates impact/collision energy to one that provides energy storage and force transfer. Thus, the foot is altering its material properties by responding to and creating variable gait impulses. This is only a reinterpretation of the classic description of the foot as a mobile adaptor becoming a rigid platform, a concept taught for over century. Research has now indicated how the foot changes its material properties in variable ways during each step (Kokubo et al, 2012; Bjelopetrovich and Barrios, 2016; Farris et al, 2019; Takabayashi et al, 2020). The foot is never fully compliant or totally rigid. However, it should become variably semi-stiffened to permit the transfer of Achilles tendon power across it and into the ground under the forefoot, as the heel raises (Holowka et al, 2017). This acceleration power provides forefoot stability on the ground while body weight is transferred forward onto the heel of the contralateral foot, an action which occurs during the brief last moments of ipsilateral single-limb support. It is important to appreciate how mechanisms are at work across the foot, that is acting as a beam to transfer acceleration power from the rearfoot to the forefoot, for this tells us much about what can go wrong.
Accessing the Power of the Triceps Surae
Energetics is the study of energy. On a biological basis, locomotive energy must be used effectively for it results from and drives evolutionary change (Pontzer et al, 2014). Muscle contraction requires energy. Some types, such as concentric and isometric contractions, are more expensive than others: i.e., eccentric contraction. Animal gaits utilise both elastic energy dissipation and storing properties of connective tissue combined with muscle generated forces, to creating safe power and stability during locomotion. The use of the triceps surae complex during late midstance in human walking is one of the best energetic examples of such mechanics among terrestrial animals. Its worth adding that this is not the same process as used in human running, which explains why humans are excellent endurance walkers but slow runners.
Triceps surae power is fundamental in restraining forward motion of the centre of mass (CoM) of the body and yet is does not generate the power that drives the body’s CoM forward (Honeine et al, 2013). During walking gait, triceps surae muscles of gastrocnemius and soleus start to activate after body weight is fully loaded onto the foot, once the braking events have ended. They continue their activity to the heel lift boundary (Crenna and Frigo, 1991; Anderson and Pandy, 2001; Franz and Kram, 2012; Murley et al, 2014). Triceps surae activity increases briefly before the CoM of the head, arms, and trunk segments (often called HAT) start to move anterior to the ankle joint. The swing limb should also be approaching the point where it passes anterior to the single-support phase limb, around absolute midstance. Early midstance activity involves concentric contraction of triceps surae (high energetic cost) causing fibreshortening of the Achilles’ (Ishikawa et al, 2007), tightening the local connective tissues in the posterior calf and knee. This increases elasticity/stiffness within the lower limb while providing some ‘braking’ of anterior momentum.
Everything rapidly changes as the CoM of HAT and the swing limb passes anterior to the stance limb’s ankle joint. Eccentric contraction of triceps surae starts as part of the action of ‘braking’ the forward momentum of the HAT’s CoM as it falls anteriorly under the effects of gravity. This gravitational force is enhanced by gaining CoM height during early midstance. The Achilles’ fibres now start to lengthen, after having been pre-stress and stiffened at the end of early midstance (Ishikawa et al, 2007). Eccentric contraction has high force production at exceptional low energy costs. This low cost is due to the fact that elasticity within the connective tissues (particularly tendon) and within the muscle fibres (via a protein called titIn) can passively help resist the fibre lengthening, making eccentric contraction highly efficient (Hessel et al, 2017). The Achilles tendon can passively do most of the lengthening in late midstance, reducing the muscle fibre length changes (Ishikawa et al, 2007). The stretchenergy is stored for acceleration very efficiently due to the Achilles’ special energy storage structure (Thorpe et al, 2013a; Shearer et al, 2017). The body moves over the foot in a manner similar to a class three lever mechanism (Fig 1 overleaf).
Figure 1: Walking’s early midstance (left) involves reducing ankle plantar-flexion angles around its axis or fulcrum (F – black star) without dorsiflexor muscle activity. Instead, reduced ankle dorsiflexion is coupled to decreasing hip flexion angles via effort from gluteus maximus (GMaxE) activity and reducing knee flexion angles through quadriceps muscle effort (QE). Together with the hip abductors and adductors, these muscles help pull the CoM up to its high point over the ankle, reducing ankle plantarflexion angles towards a neutral 90º angle to the leg. Thus, it is the lever arms operating around the hip and knee fulcrums (hollow-centred stars) that control the ankle moments. However, at absolute midstance the CoM of HAT slips anterior to the ankle joint axis, thereby requiring the ankle plantarflexor muscles to restrain and control the CoM’s anterior progression during late midstance (right image). Triceps surae now eccentrically controls acceleration around and against increasing ankle joint dorsiflexion moments, brought about by the interplay of the GRF and body weight falling forward under gravity. This sets up a class three lever arm with the triceps surae proximal attachments becoming points of effort (GastrocE and SE) that slow the proximal resistance (R) of the HAT CoM. Power for heel lift is being stored within the elastic stretch of the Achilles by this action. Gastrocnemius’ attachment to the posterior aspects of the femoral condyles can also resist the knee extension moment generated by the fall of R.
Image from the upcoming text ‘Clinical Biomechanics in Human Locomotion: Gait and Pathomechanical Principles’. (Permission www.healthystep.co.uk).
Thus, as the anterior rotation of the tibia causes ankle dorsiflexion to allow the body to rotate forward, the Achilles is stretched and elastically loaded with potential energy. This energy reaches its peak as heel lift approaches. Once the ground reaction force (GRF) maintaining heel contact decreases to a force lower than the power within the Achilles, the heel springs off the ground. Thus, as the body’s CoM moves over the forefoot and beyond, the heel lifts under elastic recoil energy within the Achilles. The activity of gastrocnemius and soleus can now switch off, but the ankle will continue to plantarflex under the power still being released from the Achilles, while the knee will flex under residual gastrocnemius connective tissue recoil (Honeine et al, 2013). The ankle plantarflexion power is derived primarily from soleus, especially at slower walking speeds (Lenhart et al, 2014; Orselli et al, 2017). The midfoot should plantarflex under the power released by tibialis posterior and peroneus longus after heel lift (Kokubo et al, 2012; Murley et al, 2014; Maharaj et al, 2016; Holowka et al, 2017; ). An arguable class two lever system is now created (think wheelbarrow). See figure 2.
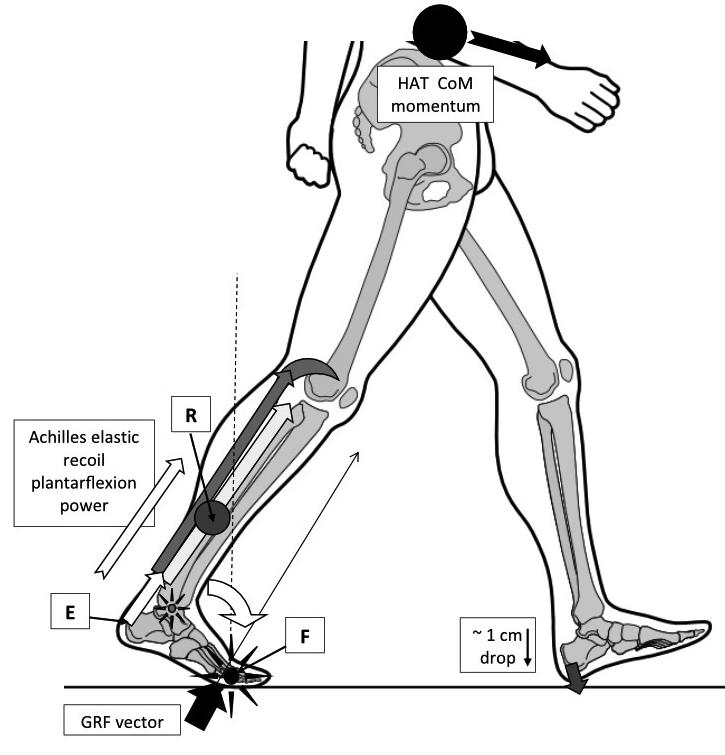
Figure 2: Heel lift power derives primarily from the triceps surae muscles, but it is released via energy stored within the Achilles from the midstance triceps surae eccentric muscle contraction. This provides the effort required to spring the heel off the ground. The heel lift effort raises all of the lower limb mass lying behind the metatarsophalangeal (MTP) joints acting as the fulcrum (F and vertical dashed line). This lower limb mass, consisting of the rearfoot and lower leg, provides the resistance that must be moved (R – grey circle).
However, plantarflexion-powered heel lift achieves so much more than just raising the heel. By forcing the foot to plantarflex at the ankle against the ground, the plantarflexion power generates a large GRF angled anteriorly that accelerates the body’s CoM forward and downward, assisting gravity to move body weight to the next step. This drops the swing limb around 1 cm to accelerate the rate of contact with the support surface, ending this swing phase.
Although this class two lever system does not primarily move the body’s CoM anteriorly (gravity and centrifugal forces from the swing limb do that), the effort it applies to gait during the terminal stance phase helps ‘power’ weight transfer onto the opposite limb, adding momentum to the HAT’s CoM that can persist into contralateral early stance phase.
Image from the upcoming text ‘Clinical Biomechanics in Human Locomotion: Gait and Pathomechanical Principles’. (Permission www.healthystep.co.uk)
How effective this power is transferred to acceleration of the body’s momentum in gait depends on what happens just before and at heel lift, for if the midfoot is too flexible acceleration power is lost.
The foot is semi-stiffened to act as a beam ready to become a crude class two lever for acceleration prior to heel lift (Horwood, 2022). For this to work, the midfoot must be relatively inflexible (stiffened) with the metatarsophalangeal (MTP) joints providing the primary location of any compliance and to thus act as a fulcrum point. Therefore, during late midstance the midfoot should only dorsiflex around 4º (Holowka et al, 2017). This midfoot compliance adaptability is necessary to prevent injury within the foot and ankle under the powerful ankle dorsiflexion moment that occurs during late midstance, prior to heel lift. Thus, during late midstance, some steps will absorb more power within the foot vault by using more midfoot motion, while the MTP joints should use their motion to provide energy dissipation during acceleration.
When on hard ground trying to walk softly, the foot might need to absorb more power, dissipating energy by providing greater compliance under reduced muscle activity. When walking very quickly, the foot might need to stay stiffer to use more power via released stored elastic energy by increasing foot stiffening-muscle activity. However, applying lots of power to a forefoot on very soft, unstable ground might cause the forefoot to sink too far into the ground for efficient walking. On very soft ground a more compliant foot and a slower gait speed is the best option. The somatosensory/sensorimotor system is the highly complex neuromusculoskeletal system that is used to get this process right. A subject for another time!
For power from the Achilles to be maximised, the fulcrum point needs to be kept at maximum distance from the Achilles effort point i.e., the attachment (enthesis) to the calcaneus. However, if that fulcrum point is too far away, then the forces within the Achilles required to achieve heel lift could result in the Achilles tendon or the triceps surae muscles being damage through excess power application (Takahashi et al, 2016). For every foot there will be a zone where power can be maximised safely, dependent on its length, foot strength, triceps surae strength, and the Achilles tendon quality/cross-sectional area. In this ‘Goldilocks zone’ should lie the freely moving (but tensioned) MTP joints that act as the fulcrums for heel lift. The resultant distance between the Achilles attachment and the fulcrum point used for heel lift is known as the ‘external moment arm of the Achilles’. Changing foot vault lengths and curvatures in gait help set this ‘Goldilocks zone’. Lowering the vault under sagging deflection caused by the ankle dorsiflexion moment of late midstance, increases the distance between the Achilles calcaneal attachment and the MTP joints. However, after heel lift the foot shortens and the vault rises to help stiffen it under reducing muscle activity (figure 3).
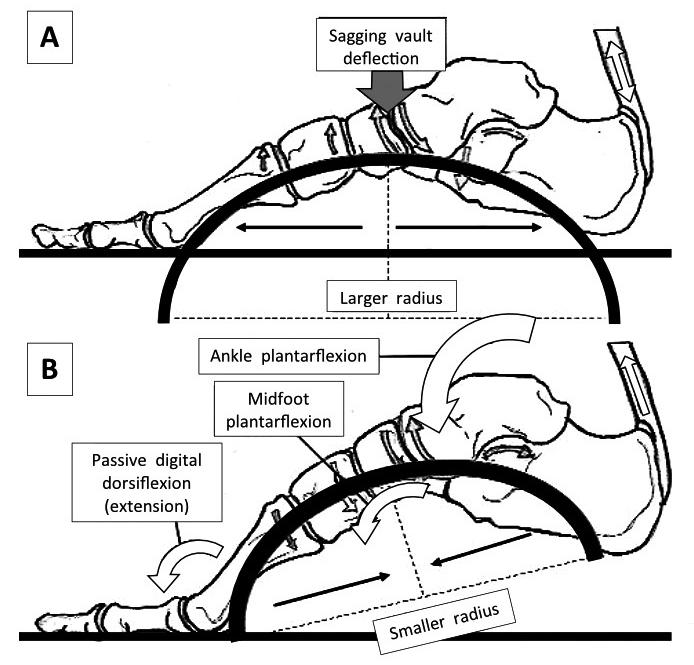
Figure 3: In midstance (A), the radius of curvature of the vault is increasing, meaning that stiffness via the mechanical laws of curvature is decreasing. The advantage of this is two-fold: energy can be dissipated through initial higher vault flexibility and that flexibility lengthens the connective tissues, stretch-stiffening them and storing elastic energy.
It also increases the distance between the Achilles and the MTP joints as the foot stiffens. After heel lift (B), ankle and midfoot plantarflexion couples to digital extension, actions that together raise the foot vault, increasing stiffness. A stiffer foot helps transfer stored plantarflexion muscle power into the forefoot creating a stabilising GRF.
However, it is the increased curvature across the smaller span distances within the transverse plane that stiffen the foot from medial to lateral, that are most likely necessary for permitting longitudinal changes in vault curvature and stiffness.
Image from the upcoming text ‘Clinical Biomechanics in Human Locomotion: Gait and Pathomechanical Principles. (Permission www.healthystep.co.uk).
Shortening and stiffening the foot after heel lift helps maintain ankle plantarflexion under decreasing power. However, if the foot is too lengthened and flexible at heel lift, the ‘Goldilocks zone’ is lost. This is also true if the foot is overly shortened and stiffened. This is why the continued stating that ‘foot supination occurs prior to heel lift to stiffen it’, is not only scientifically proven wrong, but is mechanically illogical. Those who repeat this falsehood are perpetuating an early misunderstanding of foot function. For every step, there are a number of factors that will dictate where best to position the MTP joints that are going to act as the acceleration fulcrum. This requires adaptability via variable foot pronation and gait angles prior to heel lift.
One common problem is insufficient stiffness generated across the midfoot during late midstance. If the foot fails to stiffen adequately, heel lift may occur around a fulcrum within the midfoot, with mobility derived particularly at the talonavicular and 4th and 5th tarsometatarsal joints (Lundgren et al, 2008; Wolf et al, 2008). Heel lift using midfoot joints as fulcrums of rotation cause midfoot dorsiflexion at heel lift and is known as midfoot break (DeSilva and MacLatchey, 2008; DeSilva et al, 2015).
Summary
Acceleration demands lots of energy during walking gait (Griffin et al, 2003), indicating that human muscles are busiest around the heel lift boundary. That means heel lift is a very vulnerable event in gait. Because there is no ‘active push-off’ during acceleration, the lower limb is required to facilitate an ‘easy’ mechanical heel lift from passive power. This requires the foot to be pre-stiffened (variable in levels of semi-stiffness) during late midstance to create a beam for a class two lever system that uses elastic recoil from the Achilles. This elastic recoil powers heel lift, adding momentum into the next step but does not provide the primary forward momentum (Ruina et al., 2005; Honeine et al, 2013).
In the next issue we will look at the consequences of midfoot break on the power of acceleration.
REFERENCES:
Anderson FC, Pandy MG. (2001). Dynamic optimization of human walking. Journal of Biomechanical Engineering. 123(5): 381-390.
Bjelopetrovich A, Barrios JA. (2016). Effects of incremental ambulatory-range loading on arch height index parameters. Journal of Biomechanics. 49(14): 3555-3558.
Crenna P, Frigo C. (1991). A motor programme for the initiation of forwardoriented movements in humans. Journal of Physiology. 437(1): 635-653.
DeSilva JM, MacLatchy LM. (2008). Revisiting the midtarsal break. [In: Abstracts of AAPA poster and podium presentations.] American Journal of Physical Anthropology. 135(S46): 89.
DeSilva JM, Bonne-Annee R, Swanson Z, Gill CM, Sobel M, Uy J, et al. (2015). Midtarsal break variation in modern humans: Functional causes, skeletal correlates, and paleontological implications. American Journal of Physical Anthropology. 156(4): 543-552.
Farris DJ, Kelly LA, Cresswell AG, Lichtwark GA. (2019). The functional importance of human foot muscles for bipedal locomotion. Proceedings of the National Academy of Sciences of the United States of America. 116(5): 16451650.
Franz JR, Kram R. (2012). The effects of grade and speed on leg muscle activations during walking. Gait & Posture. 35(1): 143-147.
Hessel AL, Lindstedt SL, Nishikawa KC. (2017). Physiological mechanisms of eccentric contraction and its applications: A role for the giant titin protein. Frontiers in Physiology. 8: 70. doi: 10.3389/fphys.2017.00070.
Holowka NB, O’Neill MC, Thompson NE, Demes B. (2017). Chimpanzee and human midfoot motion during bipedal walking and the evolution of the longitudinal arch of the foot. Journal of Human Evolution. 104: 23-31.
Griffin TM, Roberts TJ, Kram R. (2003). Metabolic cost of generating muscular force in human walking: insight from load-carrying and speed experiments. Journal of Applied Physiology. 95(1): 172-183.
Honeine J-L, Schieppati M, Gagey O, Do M-C. (2013). The functional role of the triceps surae muscle during human locomotion. PLoS ONE. 8(1): e52943. doi: 10.1371/journal.pone.0052943.
Horwood A (2022). Essentials of biomechanics. Principles of gait: Using the acceleration lever system effectively. Podiatry Review. 79(4): 23-26.
Ishikawa M, Pakaslahti J, Komi PV. (2007). Medial gastrocnemius muscle behavior during human running and walking. Gait & Posture. 25(3): 380-384.
Kokubo T, Hashimoto T, Nagura T, Nakamura T, Suda Y, Matsumoto H, et al. (2012). Effect of the posterior tibial and peroneal longus on the mechanical properties of the foot arch. Foot & Ankle International. 33(4): 320-325.
Lenhart RL, Francis CA, Lenz AL, Thelen DG. (2014). Empirical evaluation of gastrocnemius and soleus function during walking. Journal of Biomechanics. 47(12): 2969-2974.
Lundgren P, Nester C, Liu A, Arndt A, Jones R, Stacoff A, et al. (2008). Invasive in vivo measurement of rear-, mid- and forefoot motion during walking. Gait & Posture. 28(1): 93-100.
Maharaj JN, Cresswell AG, Lichtwark GA. (2016). The mechanical function of the tibialis posterior muscle and its tendon during locomotion. Journal of Biomechanics. 49(14): 3238-3243.
Murley GS, Menz HB, Landorf KB. (2014). Electromyographic patterns of tibialis posterior and related muscles when walking at different speeds. Gait & Posture. 39(4): 1080-1085.
Orselli MIV, Franz JR, Thelen DG. (2017). The effects of Achilles tendon compliance on triceps surae mechanics and energetics in walking. Journal of Biomechanics. 60: 227-231.
Pontzer H, Raichlen DA, Rodman PS. (2014). Bipedal and quadrupedal locomotion in chimpanzees. Journal of Human Evolution. 66: 64-82.
Ruina A, Bertram JEA, Srinivasan M. (2005). A collisional model of the energetic cost of support work qualitatively explains leg sequencing in walking and galloping, pseudo-elastic leg behavior in running and the walkto-run transition. Journal of Theoretical Biology. 237(2): 170-192.
Shearer T, Thorpe CT, Screen HRC. (2017). The relative compliance of energy-storing tendons may be due to the helical fibril arrangement of their fascicles. Journal of the Royal Society: Interface. 14(133): 20170261. doi: 10.1098/rsif.2017.0261.
Takabayashi T, Edama M, Inai T, Nakamura E, Kubo M. (2020). Effect of gender and load conditions on foot arch height index and flexibility in Japanese youths. Journal of Foot & Ankle Surgery. 59(6): 1144-1147.
Takahashi KZ, Gross MT, van Werkhoven H, Piazza SJ, Sawicki GS. (2016). Adding stiffness to the foot modulates soleus force-velocity behaviour during human walking. Scientific Reports. 6: 29870. doi: 10.1038/srep29870.
Thorpe CT, Klemt C, Riley GP, Birch HL, Clegg PD, Screen HRC. (2013a). Helical sub-structures in energy-storing tendons provide a possible mechanism for efficient energy storage and return. Acta Biomaterialia. 9(8): 7948-7956.
Wolf P, Stacoff A, Liu A, Nester C, Arndt A, Lundberg A, et al. (2008). Functional units of the human foot. Gait & Posture. 28(3): 434-441.