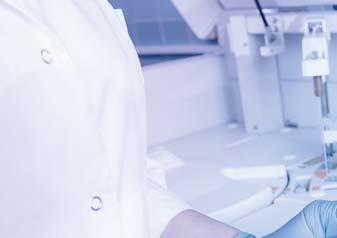
41 minute read
The Importance of Rapid Diagnostic Testing and Stewardship For Infectious Disease Management
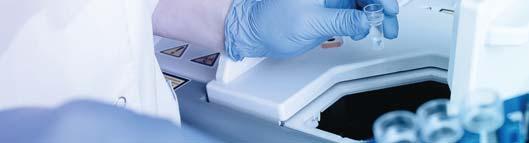
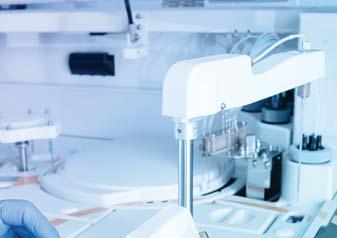
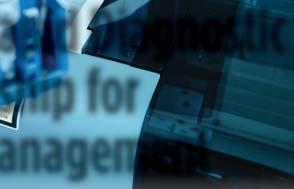
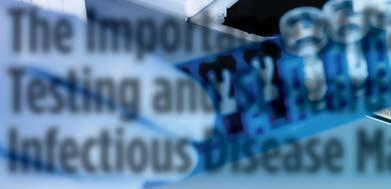
KAREN FONG, PHARMD, BCIDP
Rapid diagnostic tests (RDTs) have fundamentally transformed infectious disease management by providing rapid and robust microbiological diagnoses. In the modern-day expansion of molecular technology, direct-specimen rapid amplification and detection platforms and next-generation sequencing (NGS) are techniques that provide laboratory diagnoses at a speed, sensitivity, and breadth never before possible with conventional microbiology.1 Newer technologies have expanded rapidly to detect genotypic markers of resistance, but rapid phenotypic antimicrobial susceptibility testing has been available only recently. Thus, these commercial advances add data to the clinical presentation and assist empiric antimicrobial selection by enabling the prediction of susceptibility patterns based on local antibiograms, but are not yet a replacement for cultures. Moreover, there may be drawbacks associated with RDTs, including cost and overuse, highlighting the potential beneficial role of stewardship in making clinical decisions.1 The general advantages and disadvantages of RDTs, specific to bloodstream infections (BSIs), are outlined by Briggs et al in Table 1. 2
Due to the complexity of intervention with RDTs, RDT development and implementation must be coupled with education to facilitate informed decision making about implementation.2 Before implementation, an assessment of the clinical utility of these tests should be conducted, considering patient population and local epidemiology, along with antimicrobial stewardship programs (ASPs) to justify the acquisition of new equipment testing methodologies.3
Along with rapid turnaround time for pathogen identification and accurate interpretation of susceptibility results, great pragmatism is required to ensure results are actionable and promptly addressed to improve clinical outcomes and reduce unnecessary antimicrobial use.3 ASPs may facilitate the correct
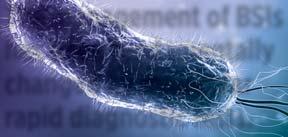
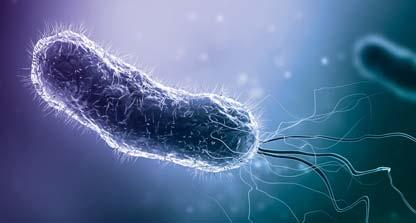
interpretation and rapid communication of results, directing clinicians to appropriate adjustment of antimicrobial therapy.4
RDTs combined with ASP intervention, particularly for BSIs and rapid testing for broad panels of respiratory viruses, have been deemed important interventions to optimize antimicrobial use and patient outcomes.5-7 Although RDTs may provide a promising level of diagnostic accuracy, combination with routine ASP efforts has been found to be imperative, to improve clinical outcomes for BSIs.6,8-10 We should be mindful that evaluations of the clinical efficacy of RDTs beyond BSI are still evolving as we discuss novel RDTs, including advances in blood culture testing, syndromic panels of direct-specimen molecular testing for respiratory infections, gastrointestinal (GI) infections, and meningitis/encephalitis, as well as prospects of outpatient point of care, and their performance with current diagnostic stewardship practices.11
Blood
The management of BSIs and blood culture contaminants (eg, coagulase-negative Staphylococcus) has been fundamentally changed by molecular RDT by providing actionable information much earlier in the course of treatment. Matrix-assisted laser desorption/ionization time of flight, or MALDI-TOF (eg, bioMérieux, BD Bruker), polymerase chain reaction (PCR)-based technologies (eg, BioFire FilmArray BCID, GenMark ePlex BCID), and nanoparticle probe technology (eg, Verigene BC-GP & BC-GN) have been associated with decreases in time to effective therapy, hospital length of stay (LOS), and mortality when paired with ASP interventions.6 Table 2 shows available blood RDTs with respect to detection method, sample type required, turnaround time, pathogen identification, resistance gene detection, and susceptibility testing. In a cost-effectiveness analysis, the benefits of molecular RDTs in BSIs have been observed.8 Interestingly, there is a strong synergism between stewardship and RDT being highlighted by these data—RDT has an 80% chance of cost-effectiveness with ASP but only 41.1% in its absence.
Recently, RDT platforms have been compared on potential desirability of antimicrobial therapy decisions using the Desirability of Outcome Ranking Management of Antimicrobial Therapy (DOOR-MAT) as a framework. When compared in an observational study at a single center, both Verigene BC and ePlex BCID had high positive predictive value (PPV) for on-panel targets, but ePlex BCID was able to identify more organisms than Verigene BC, resulting in higher mean DOOR-MAT scores (91.9 [SD ±23.1] vs 86.8 [SD ±28.5], P=0.01), respectively.12 Similarly, Verigene BC-GN was compared with BioFire BCID and BCID2. The mean DOOR-MAT score was higher for BioFire BCID2, 89.7 (SD ±24.7), than both BioFire BCID, 59.9 (SD ±33.7) (P<0.0001) and Verigene BC-GN, 83.8 (SD ±25.7) (P=0.07).13 Higher mean DOOR-MAT score for BioFire BCID2 can be largely attributed to the expanded detection of panel organisms and resistance determinants. These findings highlight the importance of considerations of local infection epidemiology with RDT implementation.
The introduction of the Accelerate Pheno system from Accelerate Diagnostics, an automated rapid phenotypic testing system, has developed further potential changes in the management of BSIs.
This system can yield organism identification, minimum inhibitory concentration (MIC), and susceptibility interpretation with a turnaround time of approximately 7 hours after positive blood culture. Compared with routine methods, overall sensitivity and specificity for the identification of organisms and essential agreement and categorical agreement for antimicrobial susceptibility were 95.6%, 99.5%, 95.1%, and 95.5%, respectively.14
Recently, several studies explored the Accelerate Pheno system coupled with ASP intervention. A randomized controlled trial of patients with gramnegative BSIs combined with prospective audit and feedback demonstrated significantly faster antibiotic changes (median decrease of about 25 hours for gramnegative antibiotics, P<0.0001) and antibiotic deescalation (median decrease of about 43 hours; P=0.01) with Accelerate Pheno, but no differences in clinical outcomes were seen compared with culture-based methods.15 Five quasi-experimental before-and-after observational studies that analyzed the integration of Accelerate Pheno with ASP intervention had variable results in patient outcomes. Although median time to optimal or targeted therapy and step-down antimicrobial therapy were significantly shorter with the use of Accelerate Pheno, LOS and duration of therapy (DOT) were not consistently shorter despite ASP intervention.16-20
Future studies on a larger scale with ASP intervention are needed to assess its impact on clinical outcomes.
The management of BSIs has been fundamentally changed by molecular rapid diagnostic tests.
Table 1. Advantages and Disadvantages of Rapid Blood Culture Assessment
Advantages Disadvantages
• Major pathogens identified ~24 h sooner than conventional methods • High-impact resistance markers or phenotypic antimicrobial susceptibility testing detected ~48 h sooner than conventional methods • Reduced overall cost of hospital stay • Improved patient outcomes in observational studies • Limited panel sizes • Reduced diagnostic quality identifying polymicrobial cultures • All systems require concurrent conventional identification and antimicrobial susceptibility testing • Platforms expensive to implement; no additional direct Medicare reimbursement • Sparse high-quality outcome studies (eg, randomized controlled trials) • Co-implementation of ASP may be required to appreciate full benefit
The Accelerate Pheno system lacks resistance gene testing. Bhalodi et al evaluated 40 Escherichia coli, Klebsiella pneumoniae, and Proteus mirabilis isolates to understand the correlation between ceftriaxone nonsusceptibility of Accelerate Pheno and extendedspectrum beta-lactamase (ESBL) production. Ceftriaxone categorical agreement of the Accelerate Pheno and broth microdilution was 97.5%.21 The ESBL confirmatory disk test was positive in 84.6% (22/26) of ESBL-producing isolates and negative in 92.9% (13/14) of non–ESBL- and/or AmpC-producing isolates, resulting in 4 false negatives and 1 false positive.21 ESBL production is well correlated with ceftriaxone nonsusceptibility, allowing expedited phenotypic detection for potential optimization of antimicrobial regimens and improved clinical outcomes.
In critically ill patients, the ability to optimize therapeutic dosing to maximize pharmacokinetic and pharmacodynamic (PK/PD) parameters in the setting of a known MIC has been associated with improved outcomes.22 Moreover, prospective evaluations of critically ill patients have reflected frequent underdosing for PK/PD targets.23
Despite variable susceptibility interpretation due to MIC testing, the introduction of rapid phenotypic testing may be of particular importance to ASPs as it relates to PK/PD optimization.24 The use of Accelerate Pheno with therapeutic drug monitoring in this patient population may have the potential to significantly improve future patient outcomes.
In the United States, candidemia is one of the most common hospital-acquired BSIs, associated with up to 47% attributable mortality, which is even higher among patients who develop septic shock. As much as a 50% reduction in mortality has been associated with prompt initiation of appropriate antifungal therapy and source control. However, this is often delayed due to blood culture insensitivity, prolonged turnaround time (median time to positivity of 2-3 days, ranging from 1 to ≥7 days) needed to yield growth, and possibility of negative growth with invasive abdominal candidiasis.25 Considering these limitations, overuse of empiric antifungal therapy for suspected invasive candidiasis has been propagated.26 The Fungitell Beta-Dglucan (BDG) detection assay (Associates of Cape Cod) and the T2Candida Panel (T2 Biosystems) are nonculture diagnostic tests with a much shorter turnaround time (3-5 hours), and have entered clinical practice as adjunctive RDTs to cultures.25,27,28
BDG is a component of the cell wall in Candida species, Aspergillus species, and Pneumocystis jiroveci. Due to cross-reactivity with other organisms, true-positive results have limited specificity for candidemia, and false positivity may be caused by physiologic changes, selected antimicrobials, hemodialysis, albumin or immunoglobulin therapy, or use of surgical material containing glucan.25 A few studies explored the use of BDG in suspected candidemia and showed deescalation of antifungal therapy through avoidance and reduction, but they were limited by small sample size and did not incorporate active ASP intervention.29,30 In 2 small, single-center cohort studies, BDG combined with ASP intervention showed mixed results in antifungal use and clinical outcomes of patients with suspected or proven invasive candidiasis. Overall antifungal use was not consistently decreased, and improvements in clinical failure rate and mortality were likely not related to BDG but rather more likely influenced by choice of antifungal, dosage adjustments, source removal, and further workup of invasive candidiasis, as recommended by the respective ASP interventions.31,32
Furthermore, the findings from a recent retrospective study challenge the test’s frequent use in the early discontinuation of empiric antifungal therapy. In a small cohort of adult patients with proven candidemia, 17.6% of patients had persistently negative BDG tests probably due to lower systemic fungal burden, signaling a concern for false-negative BDG results.33
Molecular Candida platforms, such as the T2Candida Panel by T2 Biosystems and Karius Test by Karius that detect Candida species DNA from whole blood, have emerged. While sensitivity and specificity seem to be much more promising compared with blood cultures—91% and 99%, respectively—the role of the T2Candida Panel in the early diagnosis and management of candidemia remains unclear.25,28 In 3 retrospective, single-center cohort studies, the T2Candida Panel was evaluated with the combination of active ASP intervention in adult patients with suspected
Selected RDTs for BSIs: Detection Method, Sample Type, Turnaround Time, Pathogen identification, Table 2. Resistance Markers, and Susceptibility
Susceptibility Testing
Turnaround Time Pathogens/Resistance Markers
Direct From Whole Blood
Detection Method
Blood
No
Enterococcus faecalis, Enterococcus faecium, Listeria monocytogenes, Staphylococcus, Staphylococcus aureus, Staphylococcus epidermidis, Staphylococcus lugdunensis, Streptococcus, Streptococcus agalactiae, Streptococcus pneumoniae, Streptococcus pyogenes, Acinetobacter baumannii, Bacteroides fragilis, Escherichia coli, Enterobacter cloacae complex, Haemophilus influenzae, Klebsiella aerogenes, Klebsiella oxytoca, Klebsiella pneumoniae, Neisseria meningitidis, Proteus, Pseudomonas aeruginosa, Salmonella, Serratia marcescens, Stenotrophomonas maltophilia, Candida albicans, Candida auris, Candida glabrata, Candida krusei, Candida parapsilosis, Candida tropicalis, Cryptococcus neoformans
Pathogens:
Resistance markers: mecA, mecC, vanA, vanA/B, CTX-M, IMP, KPC, mcr-1, NDM, OXA-48-like, OXA
mPCR No 1 h
FilmArray Blood Culture Identification (BioFire) No
Bacillus cereus group, Bacillus subtilis group, Corynebacterium, Cutibacterium acnes, Enterococcus, E. faecalis, E. faecium, Lactobacillus, Listeria, Listeria monocytogenes, Micrococcus, Staphylococcus, S. aureus, S. epidermidis, S. lugdunensis, Streptococcus, S. agalactiae, Streptococcus anginosus, S. pneumoniae, S. pyogenes, A. baumannii, B. fragilis, Citrobacter, Cronobacter sakazakii, E. coli, E. cloacae complex, Enterobacter (non-cloacae complex), Fusobacterium necrophorum, Fusobacterium nucleatum, H. influenzae, K. oxytoca, K. pneumoniae, Morganella morganii, N. meningitidis, Proteus, Proteus mirabilis, P. aeruginosa, Salmonella, Serratia, S. marcescens, Stenotrophomonas maltophilia, Candida albicans, Candida auris, Candida dubliniensis, Candida famata, Candida glabrata, Candidaguilliermondii, Candida kefyr, Candida krusei, Candida lusitaniae, Candida parapsilosis, Candida tropicalis, Cryptococcus gattii, C. neoformans, Fusarium, Rhodotorula
Pathogens:
Resistance markers: mecA, mecC, vanA, vanA/B, CTX-M, IMP, KPC, NDM, OXA, VIM
NAAT No 1.5 h
ePlex Blood Culture Identification (GenMark) No Yes No No
E. faecalis, E. faecium, Listeria, Micrococcus, Staphylococcus, S. aureus, S. epidermidis, S. lugdunensis, Streptococcus, S. agalactiae, S. anginosus, S. pneumoniae, Streptococcus pyogenes, Acinetobacter, Citrobacter, Escherichia coli, Enterobacter, Klebsiella oxytoca, K. pneumoniae, Proteus, P. aeruginosa, S. marcescens
Pathogens:
Resistance markers: mecA, vanA, vanA/B, CTX-M, IMP, KPC, NDM, OXA, VIM
No 2.5 h
mPCR, gold nanoparticle technology
Verigene Blood Culture Test (Luminex)
E. faecalis, E. faecium, Staphylococcus, S. aureus, S. lugdunensis, Streptococcus, A. baumannii, Citrobacter, E. coli, Enterobacter, Klebsiella, Proteus, P. aeruginosa, S. marcescens, C. albicans, C. glabrata
Pathogens:
Identification: 2 h Susceptibility: 7 h
No
Fluorescence- labeled nucleic acid probe, morphokinetic cellular analysis
Accelerate Pheno (Accelerate Diagnostics)
E. faecium, S. aureus, E. coli, K. pneumoniae, P. aeruginosa Pathogens:
Yes 3-5 h
mPCR, magnetic resonance
T2Bacteria Panel (T2 Biosystems)
C. albicans, C. glabrata, C. krusei, C. parapsilosis, C. tropicalis Pathogens:
Yes 3-5 h
mPCR, magnetic resonance
T2Candida Panel (T2 Biosystems)
multiplex polymerase chain reaction; NAAT, nucleic acid amplifi cation test BSI, bloodstream infection; mPCR,
or proven candidemia. Time to appropriate therapy decreased in patients with proven candidemia while shorter micafungin DOT and cost savings were observed in patients without microbiological evidence of invasive candidiasis. However, antifungal discontinuation with negative tests was inconsistent, despite antimicrobial stewardship intervention such as prospective audit and feedback being performed on negative results.34-36
Bomkamp et al found that overall antifungal DOT improved after implementation of the T2Candida Panel, but the use of micafungin continued to decline after the panel was removed, likely due to the concomitant increased stewardship resources, including physician-directed prospective audit and feedback around implementation. Conversely, Steuber et al observed antifungal discontinuation with negative tests to be unexpectedly low even with pharmacist-driven prospective audit and feedback. In the regression model, LOS in the ICU was predictive of failure to discontinue antifungal therapy within 48 hours of negative results (odds ratio [OR], 0.96; 95% CI, 0.94-0.99; P=0.002).35 The stewardship potential of the T2Candida Panel may be heavily contingent on the effectiveness of the ASP intervention because clinicians are particularly apprehensive about deescalation in patients already at substantially high risk for fungal infections.
The performance of the T2Candida Panel on whole blood specimens for detection of intra-abdominal candidiasis (IAC) was assessed recently. IAC is another indication with high mortality rates if antifungal treatment is delayed, but limited by low sensitivity and slow turnaround of intra-abdominal cultures and deficiencies in the BDG.37,38 Blood cultures remain the gold standard for invasive candidiasis but are typically sterile in more than 80% of patients with IAC.37,38 In 48 highrisk patients for IAC, the sensitivity, specificity, positive predictive value, and negative predictive value (NPV) of the T2Candida Panel relative to blood cultures were 33%, 93%, 71%, and 74%, respectively.39 IAC was present, diagnosed by intra-abdominal culture, in 100% of cases with concordant positive T2Candida/BDG, but absent in 90% of concordant negative results.39 Further study is needed to determine whether the T2Candida Panel has a role in the diagnosis of IAC and other types of invasive candidiasis.
By detecting bacterial DNA with T2 magnetic resonance (T2MR) in whole blood, the T2Bacteria Panel by T2 Biosystems recently made its debut to improve early initiation of appropriate antibiotic therapy in BSIs. The T2Bacteria Panel’s sensitivity and specificity, paired with a single set of blood cultures, in diagnosing BSIs caused by Enterococcus faecium, Staphylococcus aureus, K. pneumoniae, Pseudomonas aeruginosa, and E. coli were 90% (95% CI, 76%-96%) and 90% (95% CI, 88%-91%), respectively.40 NPV was 99.7%. Limited to only 5 bacteria, sensitivity and specificity for any organism was 43% (95% CI, 32%-54%) and 89% (95% CI, 87%-91%).40 Time from initiation of testing to detection and identification of pathogens was shorter for the T2Bacteria Panel (mean, 7.70 [SD, 1.38] hours) than for blood cultures (71.7 [39.3] hours). A 10% false-positive rate was observed for its targeted organisms.40
A small prospective, noninterventional T2Bacteria Panel clinical study in the emergency department (ED) demonstrated that the T2Bacteria Panel, relative to blood culture, showed a 100% percent agreement and 98.4% negative agreement.41 Compared with blood culture, the T2Bacteria assay identified bacteria, on average, 56.6 hours faster with the potential to de-escalate therapy, reduce time to species-directed therapy, and reduce time to effective therapy.41 Although the T2Bacteria assay appeared to have detected 25% more positives than blood culture, which is thought to be associated with evidence of infection, true positivity remains unclear.41 In an interventional study, Drevinek et al evaluated 53 patients and observed high sensitivity and specificity—94% and 100%, respectively—with 36.4% (8/22) causes of BSI detected only by the T2Bacteria Panel.42 On average, the results were provided 55 hours faster than blood culture. Early targeted antibiotic therapy and/ or modifications of antimicrobial treatment occurred in 9 of 15 patients with positive results.42
A recent substudy of a larger prospective, multicenter clinical trial evaluated the significance of positive T2Bacteria cases when blood culture was negative to gain a better understanding of whether these results were false positives or potentially associated with an infection. In 233 participants, 20 patients were identified with 21 (9%) discordant results. Eleven (52.5%) cases had probable BSI, 4 (19%) had possible BSI, and 6 (28.5%) were presumptive false positives.43 Among the probable and possible BSIs, discrepancies appeared to be associated with closed space and localized infections (mostly, pyelonephritis and abscess) and recent use of active antimicrobial agents.43
A recent systematic review by Giannella et al, of 14 controlled studies (most were observational), comparing T2MR with blood culture for the detection of bacterial and fungal BSI found that patients testing positive received targeted antimicrobial therapy faster (about 42 hours; P<0.001) and patients testing negative were deescalated from empiric therapy faster (about 7 hours; P<0.02).44 LOS in the ICU (mean difference, –5.0 days; P=0.03) and hospital stay (mean difference, –4.8 days; P=0.03) were shorter with T2MR, but mortality rates were comparable (28.9% vs 29.9%; relative risk, 1.02; P=0.86).44 Further prospective, ideally interventional, studies are needed to validate the role of T2MR along with ASPs in patient care.45
The Karius Test offered a new potential tool in the
antimicrobial stewardship armamentarium of microbiologists. This novel metagenomic microbiological diagnostic test uses NGS of microbial cell-free DNA in plasma, which can identify 1,250 bacteria, fungi, parasites, and viruses.46,47 While clinical data are currently limited, this new technology has shown promise in diagnosing and identifying etiologies for pneumonia, bacteremia, infective endocarditis, and general sepsis despite pretreatment with antibiotics. Its role may be useful in immunocompromised hosts with febrile neutropenia to curb delay in targeted treatment and prolonged broad-spectrum antimicrobial use where a broader range of pathogens may be associated with illness. Given its noninvasive nature, NGS has been given the term “liquid biopsy” for the diagnosis of invasive mold infections (IMI).
The performance of NGS for specifically IMI in immunocompromised patients, mostly hematopoietic cell transplant recipients and leukemia/lymphoma patients, was evaluated in 3 studies. NGS was able to detect both biopsy-proven/probable Aspergillus and non-Aspergillus IMI with a sensitivity of 51%.48-50 The specificity and PPV were estimated to be 100% based on no findings of false positives in 19 controls.48 The NPV was estimated to be 81% to 99%.48 NGS combined with serum galactomannan yielded an improved sensitivity of 84% for patients with proven/probable IMI.48
Future larger studies are needed to validate the sensitivity and specificity of this approach. NGS was explored recently for periprosthetic joint infections where standard-of-care cultures fail to detect organisms in 10% to 20% of cases.51 In a prospective observational study, NGS was performed on peripheral blood of 53 adults with hip or knee periprosthetic joint infections along with standard-of-care intraoperative tissue and synovial fluid cultures. An organism was identified by cultures in 46 (87%) of patients while NGS identified the joint pathogen in 35 cases, including 4 of 7 culture-negative cases.51 As an adjunct to cultures, NGS increased detection from 87% to 94%.51 Additional organisms were detected in 14 cases not grown in cultures by NGS.51 This test may offer a novel resource for identification with promise as an adjunct to tissue cultures to increase cases with identified pathogens.
Respiratory
Respiratory Viral Panels
In the United States, pneumonia has been a major contributor to morbidity and mortality, with an estimated 63,000 annual deaths and 1.2 million annual hospitalizations.52,53 The American Thoracic Society (ATS) and Infectious Diseases Society of America (IDSA) recommendations for empiric antimicrobial therapy in community-acquired pneumonia (CAP) are based on selecting agents targeted against the major treatable respiratory bacterial pathogens.54 However, systematic surveillance studies indicate that patients hospitalized for suspected CAP are more than twice as likely to harbor respiratory viruses than bacteria, and influenza accounts for only a minority of respiratory viruses that could cause pneumonia.55-57 There is difficulty in distinguishing between bacterial and viral etiologies in lower respiratory tract infections (LRTIs) due to similar manifestations, commonly resulting in the overuse of antibiotics.58 In patients with isolated viral pneumonia, antibiotic therapy may be safely withheld if these infections can be easily differentiated from those with concomitant bacterial etiology.59
Procalcitonin (PCT) is a component of the innate proinflammatory response to bacterial challenge, discriminating between viral and bacterial infections.60 A Cochrane review demonstrated a 2.4-day reduction in antibiotic exposure (5.7 vs 8.1 days; 95% CI, –2.71 to –2.15 days; P<0.001), a lower risk for antibiotic-related adverse effects (16.3% vs 22.1%; adjusted OR, 0.68; 95% CI, 0.57-0.82; P<0.001), and significantly lower mortality (8.6% vs 10.0%; adjusted OR, 0.83; 95% CI, 0.70-0.99; P=0.037) with a PCT-guided antibiotic stewardship algorithm in adults with acute respiratory infections (ARIs) compared with usual care, respectively.61
Still, clinicians cannot rely solely on PCT to guide antibiotic treatment decisions according to findings from a multicenter, prospective surveillance study of adults hospitalized with CAP. A PCT threshold allowing perfect discrimination between viral and bacterial detection could not be identified.62 Results were further supported by a meta-analysis of CAP patients, demonstrating that the PCT sensitivity and specificity are too low and variable to provide reliable evidence to enable clinicians to confidently address whether the infection is bacterial and antibiotics need to be administered or whether it is viral and antibiotics may be withheld.63 The ATS/IDSA guidelines for the diagnosis and treatment of adults with CAP recommend empiric antibiotic therapy to be initiated in adults with clinically suspected and radiographically confirmed CAP regardless of initial serum PCT level.54 Furthermore, PCT use does not consistently reduce antibiotic days in patients with LRTI compared with usual care, likely a consequence of subpar adherence to the PCT antibiotic prescribing guideline and lack of real-time prospective audit and feedback.58
Respiratory viral PCR assays, such as FilmArray Respiratory Panel 1 & 2 by BioFire Diagnostics, XT-8 Respiratory Viral Panel and ePlex Respiratory Pathogen Panel 1 & 2 by GenMark Diagnostics, NxTAG Respiratory Pathogen Panel and Verigene Respiratory Pathogens Flex Test by Luminex, may be useful as an adjunctive RDT. As an important intervention to reduce the use of inappropriate antibiotics, antimicrobial stewardship guidelines advocate rapid testing for broad panels of respiratory viruses.7 In a multicenter, retrospective cohort analysis of adult patients
admitted with suspected pneumonia in 179 hospitals nationwide, only 24.5% (40,787/166,273) were tested for respiratory viruses, with most being tested for influenza.64 Viral assays were positive in 12.6% (5,133/40,787), typically for influenza and rhinovirus.64 Antibacterial courses were significantly shorter for virus-positive patients than negative patients overall (mean, 5.5 vs 6.4 days; P<0.001), but varied by bacterial testing: 8.1 versus 8.0 days (P=0.60) if bacterial tests were positive; 5.3 versus 6.1 days (P<0.001) if bacterial tests were negative; and 3.3 versus 5.2 days (P<0.001) if bacterial tests were not obtained (interaction, P<0.001).64
A minority of patients were tested for a fraction of the potential respiratory viruses. Patients with positive viral tests often received a prolonged duration of unnecessary antibacterial courses even with concurrent negative bacterial tests. Nonetheless, viral testing may have affected antibacterial use because patients with positive viral results were treated for 0.9 fewer days than those with negative viral results despite being generally older and sicker.
The IDSA’s Diagnostics Committee suggests that the combination of respiratory viral testing and PCT may be more likely to exclude bacterial coinfection with confidence in a meaningful period.11 In 2 multicenter, quasi-experimental studies, the respiratory viral panel (RVP) combined with PCT and either direct or indirect (automated best practice alert) ASP intervention had a higher proportion of antibiotic discontinuation or deescalation and significantly reduced antibiotic days of therapy (mean, 2.2 days [5.8 vs 8.0 days; P<0.001]).65,66 In the absence of ASP intervention, previous evidence showed low rates of antibiotic discontinuation in patients with negative PCT and positive RVP.67 In particular, findings from Moradi et al emphasize the importance of its real-world implementation strategy by leveraging indirect ASP intervention through an automated alert, which may be especially valuable in minimal resource settings.65,68 Reduction in antibiotic days of therapy observed with RVP and PCT combination with a varying level of ASP intervention appears to be similar if not greater and more consistent compared with solely PCT or RVP use with ASP intervention, but more robust head-to-head comparisons are needed to confirm such speculation.58,61,65,66,69 Lower Respiratory Tract Infection Panels
The BioFire FilmArray Pneumonia Panel and Curetis Unyvero Lower Respiratory Tract (LRT) Panel are multiplex syndromic molecular testing panels for LRTIs, and have recently been approved by the FDA.
Compared with conventional microbiology cultures, these panels provide increased sensitivity and detect presence of resistance markers with a turnaround time of 1 to 5 hours. The BioFire FilmArray Pneumonia Panel identifies 8 viruses, 8 resistance genes, 3 atypical bacteria using qualitative targets, and 15 bacterial targets with semiquantitative analysis, which facilitates the evaluation of infection versus colonization. The Curetis Unyvero LRT Panel detects 29 bacterial pathogens and 19 resistance genes. Both panels are compatible with multiple specimen types (sputum, endotracheal aspirates, bronchoalveolar lavage [BAL] fluid). Although clinical specificity
RDTs may be improved with semiquantitative analysis, neither molecular testing continue to panels nor culture distinguish airway colonizers from invasive pathogens. Molecular testing for bacterial advance the pathogens was not addressed by the current CAP or hospital- or vendiagnosis of tilator-associated pneumonia guidelines because their performance and infections. potential impact on clinical decision making have not been determined.54,70 A downstream effect of paradoxically increasing antimicrobial use should be considered a possibility. The clinical usefulness of these panels would be in situations where patients have new or worsening lung infiltrates, are moderately to severely ill, have received empiric antibiotics prior to obtaining cultures, and/or there is concern for multidrug-resistant bacteria or a polymicrobial infection.11 The BioFire FilmArray Pneumonia Panel demonstrates a PPA and an NPA of 98.1% and 96.2%, respectively, for the identification of bacterial targets in BAL specimens compared with culture.71 Similarly, a high overall agreement of 99.2% (95% CI, 98.4%-99.6%) for viral detection is observed between the BioFire FilmArray Pneumonia Panel and culture.72 In 396 endotracheal or BAL specimens, Rand et al found a sensitivity of 97.8% (95% CI, 94.3%-99.4%), specificity of 80.4% (95% CI, 74.5%-85.4%), PPV of 80% (95% CI, 75.5%-84%), and NPV of 97.8% (95% CI, 94.3%-99.1%) for the BioFire FilmArray Pneumonia Panel for bacterial pathogens compared with culture. The panel semiquantitative copy numbers were strongly correlated with the report of white blood cell (WBC) count on initial Gram stain and conventional bacterial semiquantitation.73 Bacterial targets discovered by the panel, not found in culture, had significantly higher levels of WBC reported on Gram stain, suggesting a host response and potential pathogenicity.73 Likewise, Kolenda et al observed 100% sensitivity and 88.4% to 100% specificity with the panel in 99 severe acute respiratory syndrome coronavirus 2 (SARS-CoV-2) patients compared with culture.74 Of concern, 60.5% of the bacterial targets reported using
the panel were not recovered by culture and 76.9% of discordant results corresponded to bacteria belonging to commensal oral flora and/or report with 105 copies/ mL or fewer bacterial nucleic acids.74
The BioFire FilmArray Pneumonia Panel may be useful to rule out bacterial coinfections and avoid inappropriate prescribing of antibiotics, but positive results should be interpreted with caution. Although the BioFire FilmArray Pneumonia Panel can detect resistance genes with concordance with culture results, CTX-M and carbapenemase genes could not be definitively linked to the microorganism(s) detected.75 Thus, panel results should be used with culture results to confirm susceptibility or resistance. A retrospective multicenter study observed antimicrobial deescalation in 63 of 159 (40%) and escalation in 35 (22%) of hospitalized pneumonia patients based on BioFire FilmArray Pneumonia Panel results, reinforcing its potential to reduce unnecessary antimicrobial exposure and increase the appropriateness of empiric antibiotic therapy.76
The Curetis Unyvero LRT Panel has reported comparable diagnostic accuracy. Klein et al found an overall positive and negative percent agreement with culture of 93.4% and 98.3%, respectively, but 21.7% of specimens had additional potential pathogens identified by the panel. The positive percent agreement for antibiotic resistance markers compared with antibiotic susceptibility testing ranged from 80% to 100%.77 Challenges in interpretation also have been noted for the Curetis Unyvero LRT Panel—not all genes could be attributed to an organism, highlighting the essential continuation of current culture methods with antimicrobial susceptibility testing.78 Pickens et al reported an overall sensitivity of 85.7% (95% CI, 82.3%-88.7) and specificity of 98.4% (95% CI, 98.2%-98.7%) with an NPV of 97.9% (95% CI, 97.6%-98.1%) relative to culture.79 Results predicted antibiotic deescalation from unnecessary coverage for methicillin-resistant S. aureus (MRSA) and P. aeruginosa in 65.9% (405/615) of patients.79 Challenges in interpretation of newer RDTs, especially with resistance genes, may be potentially mitigated by ASP intervention, requiring further exploration.
The MRSA nasal PCR, Cepheid GeneXpert SA Nasal, has become a robust ASP tool for deescalation from MRSA therapy, such as vancomycin and linezolid, in predominantly patients with suspected or confirmed pneumonia. Evidence for the MRSA nasal PCR to rule out MRSA pneumonia has repeatedly demonstrated an NPV of more than 95%.80 Thus, the current CAP guidelines recommend the routine use of the MRSA nasal PCR for the deescalation of MRSA coverage.54 The implementation of this tool combined with ASP intervention have been associated with a median decrease of 2.1 days of vancomycin use (P<0.01).81
A pharmacist-driven MRSA nasal PCR–based testing protocol with ASP effort had a 70% acceptance rate for vancomycin discontinuation within 24 hours of negative results, significantly reducing unnecessary vancomycin use with an estimated cost avoidance of $40 per vancomycin course.82 Vancomycin avoidance in suspected or confirmed pneumonia with MRSA nasal screening among ICU patients has been associated with an even higher cost avoidance of $108 per patient based on the cost of surveillance testing,
For GI infections, conventional testing with stool cultures has subpar sensitivity with turnaround times of 3 to 5 days.
vancomycin, and vancomycin therapeutic drug monitoring levels.83 During implementation, the ability of the MRSA nasal PCR to hold its NPV for 7 days after results and vancomycin exposure lacking effect on results of testing should be recognized.84,85 The clinical utility of the MRSA nasal PCR appears to extend to other indications, such as skin and soft tissue infections (SSTIs).86
A national Veterans Affairs system study has supported this notion in the largest cohort to date, which includes 561,325 clinical cultures.87 A high overall NPV was observed for all infection types (96.5%) and specific infections such as BSIs (96.5%), intra-abdominal infections (98.6%), respiratory infections (96.1%), SSTIs (93.1%), and urinary tract infections (99.2%).87 Conversely, despite gram-negative resistance surveillance using rectal swab testing (eg, Streck ARM-D resistance detections kits) being standard infection prevention and control practice, its clinical utility for antimicrobial decision making has yet to be determined.88,89
Gastrointestinal Panels
Syndromic panels, FilmArray Gastrointestinal Panel (BioFire), xTAG Gastrointestinal Pathogen Panel (Luminex), and Verigene Enteric Pathogens Test (Luminex), have been developed to identify causative pathogens of infectious diarrhea. Conventional testing with stool cultures has subpar sensitivity with a turnaround time of 3 to 5 days.3 These rapid panels are highly sensitive, encompass a broad range of community-acquired bacterial, viral, and parasitic pathogens in the United States, and typically have results in 1 to 5 hours. However, most infectious diarrhea is self-limited, treatment is not recommended, and most targets lack associated antimicrobial treatment.
Negative consequences of this molecular platform may be introduced through inclusion of several targets of questionable significance, including enteropathogenic E. coli (EPEC), Clostridioides difficile, and lowincidence targets, and be further exacerbated by its nonquantitative nature.90 Additionally, specimens are often positive for multiple targets, creating further difficulty in treatment selection.91 EPEC is one of the most frequently positive targets, followed by C. difficile, but may represent colonization and lead to unnecessary treatment due to its prevalence in adults in the United States.91,92 The inclusion of C. difficile toxin raises serious concern because C. difficile colonizes more than half of children younger than 12 months of age and 5% to 10% of asymptomatic adults.93 Since detection does not differentiate between infection and colonization, testing should only be performed in the appropriate clinical context, paving the way for diagnostic and ASP partnerships.1,90
Development of clinical criteria for testing, selective reporting of results, and maintenance of separate testing methods for C. difficile are potential ASP strategies to mitigate incidental detection and inappropriate treatment.94
Although data have shown a modest decrease in the number of antibiotic days, antibiotic prescriptions, imaging studies, hospital LOS, and hospitalization cost with panel testing, these studies were retrospective without ASP intervention.95,96 In a single-center, prospective observational study, Keske et al reported that the panel post-ASP intervention, educational meetings, and activities with providers decreased antibiotic use compared with pre-ASP intervention (42.9% vs 25.8%, respectively; P=0.023).92 In a single-center, quasi-experimental study, Marcelin et al implemented a hard stop in the electronic health record as diagnostic stewardship to prevent clinicians from ordering the panel more than once per admission or in patients with diarrhea hospitalized for more than 72 hours, resulting in significant reductions in inappropriate testing and cost savings. There was an estimated 30% reduction in total ordering rates (relative risk, 0.70; 95% CI, 0.63-0.78; P<0.001), with the rate of inappropriate tests also decreasing from 21.5% to 4.9% (P<0.0001).97 Total savings calculated, factoring only orders triggering the hard stop, were about $67,000.97 Robust studies with diagnostic stewardship are needed to fully capture the impact of these panels on hospital clinical and financial outcomes.
Central Nervous System Panel
Infections of the central nervous system (CNS) are associated with significant morbidity and mortality, requiring timely medical management including rapid identification of infectious etiology and administration of antimicrobial therapy. Bacterial meningitis and viral encephalitis rely heavily on cerebrospinal fluid (CSF) examination performed after lumbar puncture. In bacterial meningitis, CSF cultures are positive in 70% to 85% of patients who have not received prior antimicrobial therapy, but cultures may take up to 48 hours for organism identification.98 For viral encephalitis, more than 10% of patients can have normal CSF findings and isolation of viral causes by CSF cultures provides limited value.99
Current meningitis and encephalitis treatment guidelines support the adjunctive use of multiplex molecular diagnostics to establish the specific etiology of infection.98,99 The FilmArray Meningitis/Encephalitis Panel (BioFire) tests for 14 common communityacquired bacterial (E. coli K1, Haemophilus influenzae, Listeria monocytogenes, Neisseria meningitidis, Streptococcus agalactiae, Streptococcus pneumoniae), viral (cytomegalovirus, enterovirus, herpes simplex virus 1 [HSV-1], herpes simplex virus 2 [HSV-2], human herpesvirus 6, human parechovirus, varicella zoster virus), and fungal pathogens (Cryptococcus neoformans and Cryptococcus gattii) in about 1 hour. The panel may be most useful for excluding the diagnosis of bacterial
meningitis with its 100% sensitivity and NPV.98,100
Despite the speed and ease of use of this panel, false positives, particularly for S. pneumoniae101; variable sensitivity for HSV102; and inferior sensitivity for Cryptococcus compared with antigen testing, have been seen.103,104 Patient outcomes, LOS and antimicrobial duration surrounding implementation of the panel have inconsistent results.105,106 In a review by Goodlet et al, 10 controlled studies evaluated antimicrobial use following implementation of the panel compared with usual care. Only half of the studies identified significant reductions in antibiotic duration, with 8 of 10 reporting modest reductions in acyclovir use.107 Further analytical and clinical validation, possibly with an ASP approach, may be beneficial since the panel falls short as a stand-alone test, requiring further additional tests for confirmation.108
Outpatient Antimicrobial Prescribing And Diagnostic Potential
Respiratory
Although the target for ASPs has been primarily the inpatient setting, the importance of ASPs in the outpatient setting has been increasingly recognized and has begun to gain widespread attention, leading to the release of the Core Elements of Outpatient Antibiotic Stewardship.109 Based on population database evaluations in the United States, at least one-third of antimicrobial prescribing is considered inappropriate, attributed to mainly respiratory infections.110 Interventions such as C-reactive protein testing, shared decision making, and PCT-guided management have shown potential to influence primary care prescribing behavior for ARIs in a systematic review. The utility of outpatient RDTs is evolving, but conclusions cannot be drawn from studies of low or very low quality.111
Molecular point-of-care testing—ID NOW and BinaxNOW (Abbott), Cobas Liat PCR (Roche Diagnostics), Xpert Xpress (Cepheid), BD Veritor Plus (BD), and FilmArray Respiratory Panel EZ (BioFire)—is widely available in the outpatient setting, but its use is limited by regulations for testing (Clinical Laboratory Improvement Amendments [CLIA] waivers) and logistical circumstances of achieving practical turnaround time during primary care visits. ID NOW influenza A & B 2 (ID NOW 2) was found to have a significantly higher sensitivity (97.8%; 95% CI, 92.2%-99.7% vs 78.9%; 95% CI, 69.0%-86.8%) compared with the rapid antigen detection test (RADT), respectively. The low analytical sensitivity of the RADT was attributed to the low viral loads in patients during the early period of 12 hours from disease onset. ID NOW 2 had high specificity as well, at better than 98%.112 In a study comparing the performance of ID NOW 2, Cobas influenza A/B nucleic acid test, and Xpert Xpress Flu for Flu A and Flu B, the overall sensitivities were 93.2%, 100%, and 100% and 97.2%, 94.4%, and 91.7%, respectively. The specificity for influenza A and B by all methods was higher than 97%.113 These tests outperformed the BD Veritor Flu A/B antigen test, with sensitivities of 79.5% for flu A and 66.7% for flu B. In contrast, BD Veritor Flu A/B antigen test compared with BinaxNOW influenza test had higher sensitivity (89.6%; 95% CI, 82.2%-94.3% vs 72.4%; 95% CI, 63.2%-80.0% and 98.8%; 95% CI, 92.6%99.9% vs 100%; 95% CI, 94.5%-100.0%) in the detection of influenza A and B, respectively.114
The impact of Xpert Xpress Flu on clinical decisions in the ED/urgent care clinic settings was evaluated in 339 pediatric encounters. After results were available, original plans were changed by clinicians in 44.5% of positive cases compared with 92.6% of negative cases (P<0.00001).115 Change in plans for antiviral use was observed in 26% of positive cases compared with 77% of negative cases (P<0.00001).115 A total of 135 antiviral prescriptions were avoided in patients with negative results.115 The FilmArray Respiratory Panel EZ (RPEZ) has been demonstrated to significantly improve the appropriate use of antibiotics and was associated with a decrease in clinic appointment duration in the outpatient pediatric clinic setting.116 Although promising, these results are likely limited by the test’s turnaround time of approximately 1 hour compared with the other tests. Additionally, there is uncertainty whether the implementation of RPEZ would reduce the use of downstream health care resources, including radiological and laboratory tests, telephone calls, and followup appointments.117
The significance of rapid turnaround time cannot be overemphasized in outpatient settings because this determines whether results are clinically actionable. Post hoc analysis of randomized controlled trial data on the use of RVP for patients presenting to EDs with respiratory symptoms has associated faster turnaround times with improved patient management compared with longer turnaround times.118 The logistics of primary care require technologies to accommodate the time constraints of a brief office visit for respiratory tract infections, which are on average only 15 minutes.119 Implementation of these new technologies will require consideration of appropriate patient populations and process measures, such as antibiotic avoidance, and use of antivirals, radiological and laboratory tests, and other health care resources.120 Optimal use of results may be derived through clinical decision support, because there likely is minimal prospective audit and feedback by ASPs in the outpatient setting.68
Conclusion
A multitude of technological advances for the management of BSIs, invasive candidiasis, respiratory infections, infectious diarrhea, CNS infections, and outpatient respiratory infections have continued to advance the field of infectious diseases. RDTs have been recognized increasingly for their significant
impact on clinical outcomes, antimicrobial use, and cost savings. Many of these RDTs show promise in their potential to improve patient care, but implementation of efficient evidence-based ASP interventions and diagnostic stewardship with pragmatism in mind are expected to be necessary to augment appropriate use and interpretation for translation outcomes. As clinicians, we should ensure the value of RDTs is not only increasing the accuracy and speed of diagnosis, but also changing clinical management, improving patient outcomes, and yielding overall cost-effectiveness, thus highlighting the importance of continued efficacy evaluations of these technologies.
References
1. Graf EH, Pancholi P. Curr Infect Dis Rep. 2020;22(2):5. 2. Briggs N, Campbell S, Gupta S. Diagn Microbiol Infect Dis. 2021;99(1):115219. 3. Palavecino EL, Williamson JC, Ohl CA. Infect Dis Clin North Am. 2020;34(1):51-65. 4. Messacar K, Parker SK, Todd JK, et al. J Clin Microbiol. 2017;55(3):715-723. 5. Wenzler E, Timbrook TT, Wong JR, et al. Am J Health Syst
Pharm. 2018;75(16):1191-1202. 6. Timbrook TT, Morton JB, McConeghy KW, et al. Clin Infect Dis. 2017;64(1):15-23. 7. Barlam TF, Cosgrove SE, Abbo LM, et al. Clin Infect Dis. 2016;62(10):e51-e77. 8. Pliakos EE, Andreatos N, Shehadeh F, et al. Clin Microbiol Rev. 2018;31(3):e00095-17. 9. Bookstaver PB, Nimmich EB, Smith TJ 3rd, et al. Antimicrob
Agents Chemother. 2017;61(9):e00189-17. 10. Avdic E, Wang R, Li DX, et al. J Antimicrob Chemother. 2017;72(11):3191-3198. 11. Hanson KE, Azar MM, Banerjee R, et al. Clin Infect Dis. 2020;71(10):2744-2751. 12. Claeys KC, Hopkins TL, Schlaffer K, et al. Antimicrob Agents
Chemother. 2021;65(9):e0044121. 13. Claeys KC, Schlaffer K, Smith R, et al. Clin Infect Dis. 2021;ciab262. 14. Charnot-Katsikas A, Tesic V, Love N, et al. J Clin Microbiol. 2017;56(1):e01166-17. 15. Banerjee R, Komarow L, Virk A, et al. Clin Infect Dis. 2021;73(1):e39-e46. 16. Dare RK, Lusardi K, Pearson C, et al. Clin Infect Dis. 2020;56(1):e01166-e01117. 17. Ehren K, Meissner A, Jazmati N, et al. Clin Infect Dis. 2020;70(7):1285-1293. 18. Sheth S, Miller M, Prouse AB, et al. Antimicrob Agents
Chemother. 2020;64(9):e00578. 19. MacVane SH, Bhalodi AA, Dare RK, et al. J Antimicrob
Chemother. 2021;76(9):2453-2463. 20. Robinson ED, Stilwell A, Attai AE, et al. Clin Infect Dis. 2021;73(5):783-792. 21. Bhalodi AA, Magnano P 3rd, Humphries RM. Diagn Microbiol
Infect Dis. 2020;98(4):115171. 22. Lodise TP Jr, Lomaestro B, Drusano GL. Clin Infect Dis. 2007;44(3):357-363. 23. Roberts JA, Paul SK, Akova M, et al. Clin Infect Dis. 2014;58(8):1072-1083. 24. Mouton JW, Muller AE, Canton R, et al. J Antimicrob Chemother. 2018;73(3):564-568. 25. Pappas PG, Kauffman CA, Andes DR, et al. Clin Infect Dis. 2016;62(4):e1-e50. 26. Clancy CJ, Nguyen MH. Curr Opin Infect Dis. 2019;32(6):546-552. 27. Gill CM, Kenney RM, Hencken L, et al. Diagn Microbiol Infect Dis. 2019;95(2):162-165. 28. Mylonakis E, Clancy CJ, Ostrosky-Zeichner L, et al. Clin Infect
Dis. 2015;60(6):892-899. 29. Nucci M, Nouer SA, Esteves P, et al. J Antimicrob Chemother. 2016;71(9):2628-2633. 30. Posteraro B, Tumbarello M, De Pascale G, et al. J Antimicrob
Chemother. 2016;71(8):2262-2269. 31. Rautemaa-Richardson R, Rautemaa V, Al-Wathiqi F, et al.
J Antimicrob Chemother. 2018;73(12):3488-3495. 32. Ito-Takeichi S, Niwa T, Fujibayashi A, et al. J Clin Pharm Ther. 2019;44(3):454-462. 33. Agnelli C, Bouza E, Del Carmen Martinez-Jimenez M, et al.
Clin Infect Dis. 2020;70(9):1925-1932. 34. Patch ME, Weisz E, Cubillos A, et al. J Antimicrob Chemother. 2018;73(suppl_4):iv27-iv30. 35. Steuber TD, Tucker-Heard G, Edwards J, et al. Diagn Microbiol
Infect Dis. 2020;97(4):115086. 36. Bomkamp JP, Sulaiman R, Hartwell JL, et al. J Clin Microbiol. 2020;58(3):e01408-e01419. 37. Bassetti M, Righi E, Ansaldi F, et al. Intensive Care Med. 2015;41(9):1601-1610. 38. Vergidis P, Clancy CJ, Shields RK, et al. PLoS One. 2016;11(4):e0153247. 39. Lamoth F, Clancy CJ, Tissot F, et al. Open Forum Infect Dis. 2020;7(3):ofaa075. 40. Nguyen MH, Clancy CJ, Pasculle AW, et al. Ann Intern Med. 2019;170(12):845-852. 41. Voigt C, Silbert S, Widen RH, et al. J Emerg Med. 2020;58(5):785-796. 42. Drevinek P, Hurych J, Antuskova M, et al. Microbiologyopen. 2021;10(3):e1210. 43. Kalligeros M, Zacharioudakis IM, Tansarli GS, et al. BMC Infect
Dis. 2020;20(1):326. 44. Giannella M, Pankey GA, Pascale R, et al. Expert Rev Med
Devices. 2021;18(5):473-482. 45. Weinrib DA, Capraro GA. Ann Intern Med. 2019;170(12):888-889. 46. Goggin KP, Gonzalez-Pena V, Inaba Y, et al. JAMA Oncol. 2020;6(4):552-556. 47. Hogan CA, Yang S, Garner OB, et al. Clin Infect Dis. 2021;72(2):239-245. 48. Hill JA, Dalai SC, Hong DK, et al. Clin Infect Dis. 2020;ciaa1639. 49. Hong DK, Blauwkamp TA, Kertesz M, et al. Diagn Microbiol
Infect Dis. 2018;92(3):210-213. 50. Armstrong AE, Rossoff J, Hollemon D, et al. Pediatr Blood
Cancer. 2019;66(7):e27734. 51. Cheverria AP, Cohn IS, Danko DC, et al. J Bone Joint Surg Am. 2021 Jul 22. doi: 10.2106/JBJS.20.02229 52. Kung HC, Hoyert DL, Xu J, et al. Natl Vital Stat Rep. 2008;56(10):1-120. 53. Agency for Healthcare Research and Quality. Accessed September 15, 2021. http://www.ahrq.gov/research/sep08/0908RA40. htm 54. Metlay JP, Waterer GW, Long AC, et al. Am J Respir Crit Care
Med. 2019;200(7):e45-e67. 55. Burk M, El-Kersh K, Saad M, et al. Eur Respir Rev. 2016;25(140):178-188. 56. Piralla A, Mariani B, Rovida F, et al. J Clin Virol. 2017;92:48-51. 57. Jain S, Self WH, Wunderink RG, et al. N Engl J Med. 2015;373(24):2382.
58. Huang DT, Yealy DM, Angus DC, the Pro ACTI. N Engl J Med. 2018;379(20):1973. 59. Ruuskanen O, Lahti E, Jennings LC, et al. Lancet. 2011;377(9773):1264-1275. 60. Gilbert DN. Clin Infect Dis. 2011;52 suppl 4:S346-350. 61. Schuetz P, Muller B, Christ-Crain M, et al. Cochrane Database Syst
Rev. 2012(9):CD007498. 62. Self WH, Balk RA, Grijalva CG, et al. Clin Infect Dis. 2017;65(2):183-190. 63. Kamat IS, Ramachandran V, Eswaran H, et al. Clin Infect Dis. 2020;70(3):538-542. 64. Klompas M, Imrey PB, Yu PC, et al. Infect Control Hosp
Epidemiol. 2021;42(7):817-825. 65. Moradi T, Bennett N, Shemanski S, et al. Clin Infect Dis. 2020;71(7):1684-1689. 66. Lee CC, Chang JC, Mao XW, et al. J Am Med Dir Assoc. 2020;21(1):62-67. 67. Timbrook T, Maxam M, Bosso J. Infect Dis Ther. 2015;4(3):297-306. 68. Timbrook TT. Clin Infect Dis. 2020;71(7):1690-1692. 69. Srinivas P, Rivard KR, Pallotta AM, et al. Pharmacotherapy. 2019;39(6):709-717. 70. Kalil AC, Metersky ML, Klompas M, et al. Clin Infect Dis. 2016;63(5):e61-e111. 71. Buchan BW, Windham S, Balada-Llasat JM, et al. J Clin Microbiol. 2020;58(7):e00135-20. 72. Webber DM, Wallace MA, Burnham CA, et al. J Clin Microbiol. 2020;58(7) e00343-20. 73. Rand KH, Beal SG, Cherabuddi K, et al. Open Forum Infect Dis. 2021;8(1):ofaa560. 74. Kolenda C, Ranc AG, Boisset S, et al. Open Forum Infect Dis. 2020;7(11):ofaa484. 75. Yoo IY, Huh K, Shim HJ, et al. Int J Infect Dis. 2020;95:326-331. 76. Monard C, Pehlivan J, Auger G, et al. Crit Care. 2020;24(1):434. 77. Klein M, Bacher J, Barth S, et al. J Clin Microbiol. 2021;59(3):e02497-20. 78. Collins ME, Popowitch EB, Miller MB. J Clin Microbiol. 2020;58(5):e02013-19. 79. Pickens C, Wunderink RG, Qi C, et al. Diagn Microbiol Infect Dis. 2020;98(4):115179. 80. Parente DM, Cunha CB, Mylonakis E, et al. Clin Infect Dis. 2018;67(1):1-7. 81. Willis C, Allen B, Tucker C, et al. Am J Health Syst Pharm. 2017;74(21):1765-1773. 82. Meng L, Pourali S, Hitchcock MM, et al. Open Forum Infect Dis. 2021;8(4):ofab099. 83. Smith MN, Erdman MJ, Ferreira JA, et al. J Crit Care. 2017;38:168-171. 84. Smith MN, Brotherton AL, Lusardi K, et al. Ann Pharmacother. 2019;53(6):627-638. 85. Carr AL, Daley MJ, Givens Merkel K, et al. Pharmacotherapy. 2018;38(12):1216-1228. 86. Butler-Laporte G, De L’Etoile-Morel S, Cheng MP, et al. J Infect. 2018;77(6):489-495. 87. Mergenhagen KA, Starr KE, Wattengel BA, et al. Clin Infect Dis. 2020;71(5):1142-1148. 88. Lindblom A, Karami N, Magnusson T, et al. Eur J Clin Microbiol
Infect Dis. 2018;37(8):1491-1497. 89. Rottier WC, Bamberg YR, Dorigo-Zetsma JW, et al. Clin Infect
Dis. 2015;60(11):1622-1630. 90. Dien Bard J, McElvania E. Clin Lab Med. 2020;40(4):393-420. 91. Buss SN, Leber A, Chapin K, et al. J Clin Microbiol. 2015;53(3):915-925. 92. Keske S, Zabun B, Aksoy K, et al. J Clin Microbiol. 2018;56(5):e00148-18. 93. Loo VG, Bourgault AM, Poirier L, et al. N Engl J Med. 2011;365(18):1693-1703. 94. Baghdadi JD, Coffey KC, Leekha S, et al. Curr Infect Dis Rep. 2020;22(15). doi:10.1007/s11908-020-00725-y 95. Beal SG, Tremblay EE, Toffel S, et al. J Clin Microbiol. 2017;56(1):e01457-17. 96. Axelrad JE, Freedberg DE, Whittier S, et al. J Clin Microbiol. 2019;57(3):e01775-18. 97. Marcelin JR, Brewer C, Beachy M, et al. Infect Control Hosp
Epidemiol. 2019;40(6):668-673. 98. Tunkel AR, Hartman BJ, Kaplan SL, et al. Clin Infect Dis. 2004;39(9):1267-1284. 99. Tunkel AR, Glaser CA, Bloch KC, et al. Clin Infect Dis. 2008;47(3):303-327. 100. Saravolatz LD, Manzor O, VanderVelde N, et al. Clin Infect Dis. 2003;36(1):40-45. 101. Leber AL, Everhart K, Balada-Llasat JM, et al. J Clin Microbiol. 2016;54(9):2251-2261. 102. Liesman RM, Strasburg AP, Heitman AK, et al. J Clin Microbiol. 2018;56(4):e01927-17. 103. O’Halloran JA, Franklin A, Lainhart W, et al. Open Forum Infect
Dis. 2017;4(4):ofx242. 104. Lewis PO, Lanier CG, Patel PD, et al. Med Mycol. 2020;58(3):408-410. 105. DiDiodato G, Bradbury N. Open Forum Infect Dis. 2019;6(4):ofz119. 106. Dack K, Pankow S, Ablah E, et al. Kans J Med. 2019;12(1):1-3. 107. Goodlet KJ, Tan E, Knutson L, et al. Diagn Microbiol Infect Dis. 2021;100(4):115394. 108. Vetter P, Schibler M, Herrmann JL, et al. Clin Microbiol Infect. 2020;26(6):706-712. 109. Sanchez GV, Fleming-Dutra KE, Roberts RM, et al. MMWR
Recomm Rep. 2016;65(6):1-12. 110. Fleming-Dutra KE, Hersh AL, Shapiro DJ, et al. JAMA. 2016;315(17):1864-1873. 111. Tonkin-Crine SK, Tan PS, van Hecke O, et al. Cochrane Database
Syst Rev. 2017;9:CD012252. 112. Mitamura K, Yamazaki M, Ichikawa M, et al. J Infect Chemother. 2021;27(3):450-454. 113. Kanwar N, Michael J, Doran K, et al. J Clin Microbiol. 2020;58(3):e01611-19. 114. Hassan F, Nguyen A, Formanek A, et al. J Clin Microbiol. 2014;52(3):906-910. 115. El Feghaly RE, Nolen JD, Lee BR, et al. J Pediatr. 2021;228:271277 e271. 116. Beal SG, Posa M, Gaffar M, et al. Pediatr Infect Dis J. 2020;39(3):188-191. 117. Fenton J, Posa M, Kelly M, et al. Pediatr Infect Dis J. 2020;39(9):e282-e283. 118. Brendish NJ, Malachira AK, Beard KR, et al. Eur Respir J. 2018;52(2):1800555. 119. Linder JA, Singer DE, Stafford RS. Clin Ther. 2003;25(9):2419-2430. 120. Kozel TR, Burnham-Marusich AR. J Clin Microbiol. 2017;55(8):2313-2320.
Dr. Fong reported no relevant financial disclosures.
About the Author
Karen Fong, PharmD, BCIDP, is a clinical pharmacist, Infectious Diseases and Antimicrobial Stewardship, in the Department of Pharmacy, University of Utah Health, Salt Lake City, Utah.