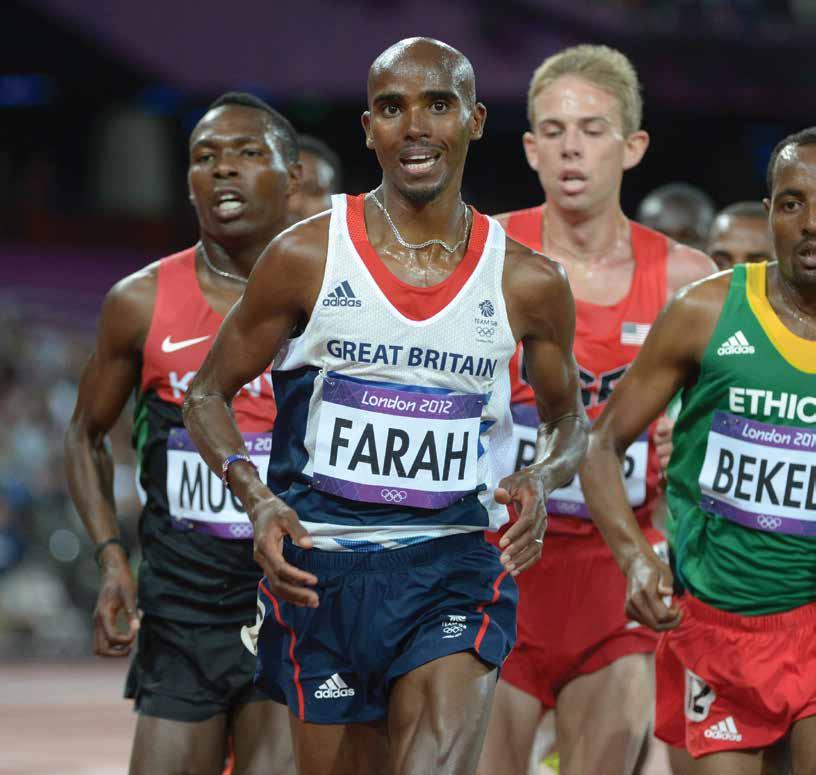
21 minute read
Biokinetic Energy
Identifying the Fourth Energy System for all Track & Field Events
PETER JOHN L THOMPSON
ENERGY SYSTEMS
The simplest contributor to any athletic movement or performance is how the athlete creates, manages, utilises and expresses energy. We can now consider the human body to be like a hybrid car, as it has two principal sources of energy available to create movement. This is true for all the events of Track & Field, but with obvious shifts in emphasis of the source, production and expression.
The two principal sources of energy are: • The Bioenergetic (Metabolic) Energy Systems • The Biokinetic Energy System
The Bioenergetic or Metabolic energy systems involve the muscles working like engines by burning fuel to produce movement. They are energy converters changing the chemical energy in the food we eat into the energy of movement. This chemical or metabolic energy of movement can be produced in different ways by the three distinct metabolic energy systems, ATP-CP, Lactate and Aerobic.
But the energy for movement is also produced by the body’s kinetic chain storing and returning energy, and coaches have long recognized the importance of this “elastic” or “reactive” contribution without previously being able to have a full understanding of the process and tissues involved. This energy source and process largely involves the joints, muscles, tendons and aponeuroses within the kinetic chain. Because the process involves the kinetic chain, it is called the Biokinetic Energy System and can be clearly thought of as the Fourth Energy System. This biokinetic energy source is fundamental, then, to all events and goes hand in hand with having an optimally functioning kinetic chain. The primary role of the biokinetic energy system is to add to muscular power production and to provide a metabolic energy-sparing input that improves economy and endurance expressed in all the events of track & field athletics.
THE BIOKINETIC ENERGY SYSTEM - THE FOURTH ENERGY SYSTEM
We have stated that the energy for human movement can be produced by the body’s kinetic chain storing and returning energy. This energy source and process largely involves the joints, muscles, tendons and aponeuroses, along with the kinetic chains that they comprise.
Most coaches recognise that biokinetic capabilities are important in the ‘power’ events of athletics: the sprints, hurdles, jumps, throws and combined events. But there is a common misconception that these capabilities and the role of biokinetic energy is unimportant or insignificant in endurance activities, like middle and long distance running and race walking.
An athlete’s ability to apply force rapidly and accelerate their body mass is the rule, rather than the exception, in all of the events of track & field athletics. While the endurance events certainly do require an emphasis on metabolic capacity over time, by definition, they also involve repeated brief, explosive ‘spikes’ in power output each time the foot contacts the ground. Biokinetic contributions must be considered fundamental for both force production and economical metabolic energy-sparing, and therefore, it would be incorrect to think of these endurance events as being solely sub-maximal activities. The primary role of the biokinetic energy system is then to add to muscular force and power production and to provide a metabolic energy-sparing input that improves economy and endurance expressed in all the events of athletics.
It does not matter whether we are examining, for example, the endurance of a 100m sprinter in the last 20m of a race or a marathoner at mile 20 in a marathon race. If the metabolic energy systems are depleted, the athlete will have no option but to slow down for this reason. The athlete, however, who is able to maintain the stability and function, the optimal stiffness, of their kinetic chain while metabolically fatiguing, is able to continue accessing the contribution of their biokinetic energy system to fuel and maintain their movement.
There is ample evidence that the control of stiffness and the attaining of optimal stiffness is a trainable response in the athlete, both neurologically and through adaptations of the related tissues, the muscles, tendons, aponeuroses and ligaments. It is, however, currently unclear how much of the tissue response in action is a conscious response and how much is unconscious. But this is not a limiting factor, nor a ‘need to know’ for application by coaches and athletes training to develop and improve control of the kinetic chain and so, optimize stiffness.
KINETIC CHAINS – CONTROLLING STABILITY AND MOVEMENT
We are aware that the body is supported by an internal skeleton that is capable of stability and movement when pulled on by muscles acting across joints and under instruction by signals from the nervous system. The combination and inter-relation of the nervous, muscular and skeletal systems, along with the connective tissues, is given the term the Kinetic Chain.
FIGURE 1: THE KINETIC CHAIN
The concept of the “Kinetic Chain” originated in 1875, when a mechanical engineer named Franz Reuleaux proposed that if a series of overlapping segments were connected via pin joints, these interlocking joints would create a system that would allow the movement of one joint to affect the movement of another joint within the kinetic chain. In 1955, Dr. Arthur Steindler adapted this theory to include an analysis of human movement. Steindler suggested that the extremities be viewed as a series of rigid, overlapping segments and defined the Kinetic Chain as a “combination of several successively arranged joints constituting a complex motor unit.”
The body’s Kinetic Chain runs from the top of the head to the tip of the toes and involves the major joints of the body from the neck, through the upper back, lower back, pelvis, hips, knees, ankles and toe joints. We can also look at parts of this kinetic chain separately, such as the Anterior, or Frontal, Kinetic Chain; the Posterior, or Rear, Kinetic Chain and the Oblique Kinetic Chains. Sometimes we focus on other parts of the chain, such as the Upper or Lower Kinetic Chains, but always remembering that we must take into account the context of the whole-body Kinetic Chain to provide optimal segmental control, functional stability and movement of any parts of the body.
The importance of the kinetic chain for coaches is understanding that for optimal functional efficiency and performance, all ‘links’ in the chain must be in the correct position and able to function effectively. If one link or component in the chain is out of alignment or not functioning optimally, the entire kinetic chain is compromised. It cannot function correctly, and ultimately, the movement and performance will be negatively impacted. Of all the links in the chain, the pelvis is a fundamental link. Its correct alignment in all planes is a key determinant of posture and the integrity of the whole chain. (See Figure 1)
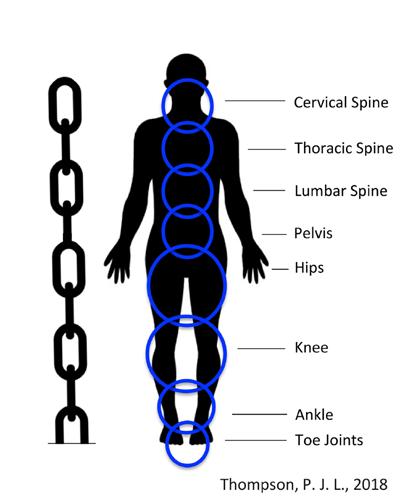
CURRENT UNDERSTANDING OF THE INVOLVED TISSUES
As well as providing for integrated segmental control, the Kinetic Chain is capable of storing and returning energy, known as Biokinetic Energy. It used to be thought that the muscles were the primary active systems in this energy storage and return, with the tendons and other connective tissues having a relatively passive role. In recent years, however, modern research equipment and techniques such as ultrasonography have been developed that can examine the structures and internal and external forces in the human kinetic chain during movement and exercise.
This equipment and these techniques have been refined, and since 2013, they can identify the relative contribution of the muscles, tendons and other connective tissues in vivo, in the living and moving individual. Not surprisingly, we are finding that the performance and properties of living tissues in situ, in the body, and undergoing specific movements are very different from what we have seen previously when examining tissues in vitro, in a cadaver. Dead tissue is, unsurprisingly, unresponsive and inelastic. The latest methods are yielding startling new insights and understandings of human movement and providing a more accurate picture of the dynamic interaction, nature and synergy of tissues in the kinetic chain to respond to the variety of movement and force production possibilities.
From the recent research findings, there has to be a reevaluation of the traditionally taught Stretch-Shortening Cycle, SSC, and its role in biokinetic energy production. The traditional view of the elastic properties of the lower kinetic chain, for example, was to imagine a dynamic muscle contraction going from eccentric to concentric, with the muscletendon system acting like a ‘rubber band’. While loading and stretching, energy would be stored primarily in the muscle (eccentric phase), with some stretching of the tendon ,and this energy would be regained at muscle shortening (concentric phase) for toe-off. This is now viewed as an oversimplistic and misleading description of the variability of responses of the body’s tissues to differing movement and force production demands. Additionally, we now appreciate more what an aponeurosis is and the part that aponeuroses play in biokinetic energy production, as will be explained in more detail later.
We now appreciate that the eccentric contraction phase of the muscle effectively does not operate as we thought and is not so important as the elastic properties of the tendons and aponeuroses. For economy of movement, the associated muscles need to be emphasised as being in isometric, or close to isometric, mode. This is essentially the same ‘rubber band’ analogy except now there is a recognition that the muscle response and contribution in running at all speeds is not as great as it was thought to be for creating force but is vital as a stabiliser and resistance. The ‘rubber band’ is now the tendon and aponeurosis working in synergy. The biokinetic contribution to performance will be optimal, and
TENDONS
tough and resilient and primarily serve to connect muscles to bones
a white opaque shiny rope-like structure
acts as a Series Elastic Element (SEE)
only permits elastic properties relating to strain created from changes in their rope-like length
uniaxial action
APONEUROSES
tough and resilient and may involve muscles or connects bone to bone
acts as both a Parallel Elastic Element (PEE) and as a Series Elastic Element (SEE)
permits elastic properties relating to strain in a multi-directional response
multi-axial action.
most energy will be regained if the stiffness of the kinetic chain is optimal. The level of isometric stabilisation or activation by the muscles and positioning of the joints helps determines the stiffness of the system. But what exactly do we mean by this ‘stiffness’?
STIFFNESS
Kinetic chains can absorb, store and return energy as the body moves. How well the energy is absorbed and returned is dependent on the ‘stiffness’ of the kinetic chain. Here we must not confuse kinetic chain ‘stiffness’ with the standard dictionary definition of stiffness, which is, “rigid, firm, difficult or impossible to bend.” To better understand kinetic chain ‘stiffness,’ let’s look at running as an example. At its simplest, running is based on propelling the body forward while keeping its centre of mass (CM) relatively level during the running cycle. During impact with the ground, the leg acts much like a biomechanical spring, with the Lower Kinetic Chain absorbing energy and releasing it later in the running cycle at toe-off. Following this model, the closer the ‘stiffness’ of the kinetic chain is to optimal, the better the elastic return and the less metabolic energy needed to run at a certain velocity, or you can run faster for the same metabolic contribution. Stiffness is crucial and positive in defining biokinetic performance capacities in all events.
To continue with running as an example, think about what would happen if you were to run gently across a concrete car park adjacent to a sandy beach and continue straight onto the sand. What would happen? Most probably, when you hit the sand your legs would remain extended to a much greater degree at each joint than they were while running over the parking lot. In other words, your legs would become stiffer on the sand. The stiffness of the leg is a function of the Lower Kinetic Chain involving the pelvis, hip, knee, ankle, foot and first metatarsophalangeal, “big toe”, (MTPJ) joints, coupled with the muscles, tendons and aponeuroses. But, if you were to sprint across the concrete onto the sand, you might well stumble and fall, as your legs might not have time to adjust to the new soft and giving surface and would not be sufficiently stiff to support the CM. Usually the body is able to adapt to terrain, and there will be a relative increase of leg stiffness on softer surfaces and a relative decrease on harder surfaces. (See Figure 2)
Incorrect stiffness on any surface produces negative results in either direction. If the Lower Kinetic Chain is too stiff, then ground impact and reaction forces are increased, and the kinetic, elastic, energy is dissipated, lost, in the impact. If the stiffness is not sufficient, then the energy is dissipated, lost, into the squidgy ‘biomechanical spring,’ and another consequence is that the muscles will have to activate more and therefore use more metabolic energy. Ideal or ‘optimal stiffness’ for any individual also prevents injury. If an athlete has too much stiffness they will be liable for bony injuries, while too little stiffness is associated with soft tissue injuries. This seems to make intuitive sense, that if the force at foot-strike, whether rear-, mid- or forefoot is too high from too much stiffness, the soft tissues cannot absorb the strain and so this force is taken up in the bony structures. Conversely, if the stiffness is too low, the soft tissues must be employed to control the foot strike, leading to transfer of strain to the soft tissue.
Stiffness is important to all the events of athletics. For the power event, athletes in the sprints, hurdles and jumps, stiffness is relevant largely in the same way that it is for a middle and long distance runner or race walker. In general, when running speed increases, there is a concomitant increase in stiffness. For throwers and combined event athletes, the oblique kinetic chains come into play to a much greater extent and, if the stiffness is optimal, can enhance the power created in their rotational and torsional movements.
To summarise stiffness is to view it as, “a skilled, responsive, adaptable, qualitative bio-mechanism involving the joints and tissues of the kinetic chains that determines the ability to optimise movement and biokinetic energy production and expression.” (Thompson, P.J.L., 2016).
TENDONS AND APONEUROSES – VITAL ELASTIC TISSUE
There is a need to review the elastic tissues involved with the Kinetic Chain and Biokinetic Energy production since research articles frequently use the terms, ‘aponeurosis’, and the plural ‘aponeuroses’, that have not previously been incorporated into coach education materials. All coaches are now familiar with bones, muscles, tendons, ligaments and what they understand by ‘connective tissue’, but what are the aponeuroses, what are their properties and why might a coach need to know about them?
An aponeurosis is a ribbon or sheet of collagenous connective tissue, either as a separate structure or providing a wide area of attachment for one or both ends of flat muscles. Probably the best-known separate structure is the plantar aponeurosis on the under surface of the foot, commonly referred to as the plantar fascia. Most coaches are familiar with the plantar aponeurosis, but this awareness is predominantly triggered from their athletes sustaining an injury to this structure, usually plantar fasciitis. But, with such awareness, how many coaches are actually knowledgeable
FIGURE 2: COMPARING THE SPRING-MASS MODEL AND THE FUNCTIONING LOWER KINETIC CHAIN
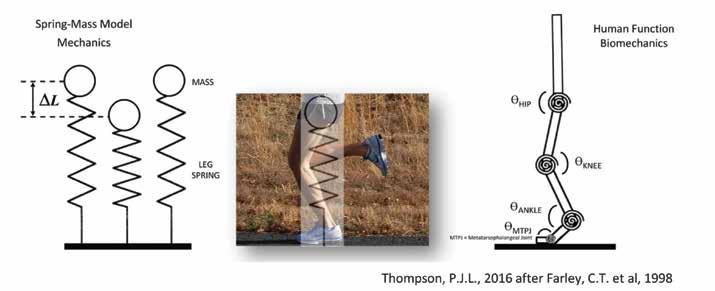
of all the functions of the plantar aponeurosis? In one summary article, a researcher identified ten key biomechanical functions of the plantar aponeurosis, reinforcing the vital contribution of this structure to the biokinetic function of the foot. Aponeuroses are found throughout the body and are now known to be a vital elastic component of the kinetic chain, contributing to stability, movement and biokinetic energy production.
Aponeuroses are tough and resilient, similar to tendons in both function and composition, only tendons primarily serve to connect muscles to bones. The aponeurosis is a white, transparent sheath, a flat structure like a sheet, whereas a tendon is a white, shiny, rope-like structure. Both aponeuroses and tendons are capable of resisting considerable strain and have elastic properties that may return, amplify or attenuate forces. The difference between the two lies in their structure. Tendons are only elastic in one direction, relating to strain created from changes in their rope-like length. Aponeuroses, however, can react to strain in a multi-axial manner and provide a multi-directional response to strain, endowing aponeuroses with the capacity to function dynamically across a range of stiffnesses and movements. For many muscles, a significant portion of the tendon is an aponeurosis. The calf, or gastrocnemius aponeurosis, for example, is a sheet-like aponeurosis that is continuous and merges with the achilles tendon and extends over the gastrocnemius muscle bellies. We can now appreciate that the primary elastic connective tissues are the tendons and aponeuroses and that they frequently work in synergy in the kinetic chain to yield biokinetic energy. Understanding the kinetic chain and how the body and all of its segments work together is essential for developing effective exercise and training programs to optimise Biokinetic Energy production and expression.
DEVELOPING THE BIOKINETIC ENERGY SYSTEM - CONTROL OF OPTIMAL STIFFNESS
Control of the optimal stiffness of the Kinetic Chain and component kinetic chains can be achieved by an athlete’s adaptive responses to the blending of the following types of training: • Postural exercises for the controlled stability and movement of the whole kinetic chain • Focus and emphasis on the postural control of the pelvis in all three planes • Ensuring sufficient functional mobility throughout the kinetic chain with an emphasis on sufficient hip extension, ankle dorsiflexion and flexion of the first metatarsophalangeal, “big toe,” joints • Exploring the fundamental movements of kinetic chains e.g. bracing, hinging, squatting and lunging • Varying free weight loadings and exercises using implements, kettlebells, medicine balls and Olympic lifts - inter-session and intra-session • Varying the pattern and speed of movements, including running velocity - intersession and intra-session • Running on surfaces of varying compliance, on the continuum from effectively non-compliant, solid surfaces like concrete to hyper-compliant, particulate surfaces such as sand - inter-session and intrasession • Training in shoes with midsoles of varying cushion and including barefoot running for the very small minority of athletes whose lower kinetic chain flexibility and function permits - inter-session and intrasession • Running on inclines, declines and undulations of varying steepness and length - inter-session and intra-session • Not over-striding, or over-reaching in any rotational movement, either naturally or deliberately.
As with most training, training the Biokinetic Energy system emphasises the quality of movement over quantity of movement, the quality of intensity over quantity of intensity and continual variety so that optimal adaptive stiffness responses and capabilities are developed. At the appropriate stage of athlete development, once tissues are conditioned and strong enough, and once skilled control of the Kinetic Chain and Stiffness is achieved, only then should there be challenges in training to maintain form and Biokinetic Energy production under competitionsimilar, fatigued conditions.
In summary, the Biokinetic Energy source is fundamental to all events and goes hand in hand with having an optimally functioning Kinetic Chain. Together they provide optimal movement and energy control, the Fourth Energy System, augmenting, enhancing and working in synergy with the contribution of the three Bioenergetic Energy Systems.
REFERENCES
ALBRACHT, K. & ARAMPATZIS, A. (2013) Exercise-induced changes in triceps surae tendon stiffness and muscle strength affect running economy in humans. Eur J Appl Physiol 113(6): 1605-1615.
BARNES, K.R.; HOPKINS, W.G.; MCGUIGAN, M.R. & KILDING, A.E. (2015) Warm-up with a weighted vest improves running performance via leg stiffness and running economy. J Sci Med Sport 18(1): 103-108.
BARNES, K.R.; MCGUIGAN, M.R. & KILDING, A.E. (2014) Lower-body determinants of running economy in male and female distance runners. J Strength Cond Res 28(5): 1289-1297.
BLICKHAN, R. (1989) The spring-mass model for running and hopping. J. Biomech. 22: 1217-1227.
BUTLER, R.J.; CROWELL III, H.P. & MCCLAY DAVIS, I. (2003) Lower extremity stiffness: implications for performance and injury. Clinical Biomechanics 18: 511–517.
DALLEAU, G.; BELLI, A.; BOURDIN, M. & LACOUR, J.R. (1998) The spring-mass model and the energy cost of treadmill running. Eur J Appl Physiol 77(3): 257-263.
DICHARRY, J. (2012) Anatomy for Runners: Unlocking Your Athletic Potential for Health, Speed and Injury Prevention. Skyhorse Publishing, New York, USA.
FARLEY, C.T.; HOUDIJK, H.H.; VAN STRIEN, C. & LOUIE, M. (1998) Mechanism of leg stiffness adjustment for hopping on surfaces of different stiffnesses. J Appl Physiol 85(3): 1044-55.
FERNÁNDEZ, P.J.; HOLOWKA, N.B.; DEMES, B. & JUNGERS, W. L. (2016) Form and function of the human and chimpanzee forefoot: implications for early hominin bipedalism. Sci Rep. 6: 30532.
FLETCHER, J.R. & MACINTOSH, B.R. (2015) Achilles tendon strain energy in distance running: consider the muscle energy cost. J Appl Physiol 118: 193-199.
FLETCHER J.R.; PFISTER, T.R. & MACINTOSH, B.R. (2013) Energy cost of running and Achilles tendon stiffness in man and woman trained runners. Physiological Reports: 1(7): e00178.
HEISE, G.D. (2016) The work and activation of lower extremity muscles in explaining interindividual variability in running economy. 34rd International Conference on Biomechanics in Sports Tsukuba, Japan, July 18-22.
HILL, A.V. (1938) The heat of shortening and the dynamic constants of muscle. Proc. R. Soc. Lond. B. London: Royal Society 126(843): 136-195.
HUDGINS, B.; SCHARFENBERG, J.; TRIPLETT, N.T. & MCBRIDE, J.M. (2013) Relationship between jumping ability and running performance in events of varying distance. J Strength Cond Res 27(3): 563–567.
KARAMANIDIS, K.; ARAMPATZIS, A. & BRÜGGEMANN, G-P. (2006) Adaptational phenomena and mechanical responses during running: effect of surface, aging and task experience. Eur J Appl Physiol 98(3): 284-298.
KELLY, L.A.; LICHTWARK, G. & CRESSWELL, A.G. (2015) Active regulation of longitudinal arch compression and recoil during walking and running. Jour Royal Soc Interface 12(102): 20141076.
KIRBY, K.A. (2016) Understanding ten key biomechanical functions of the plantar fascia. Podiatry Today 29(7)
KORFF, T.; HORNE, S.L.; CULLEN, S.J. & BLAZEVICH, A.J. (2009) Development of lower limb stiffness and its contribution to maximum vertical jumping power during adolescence. The Journal of Experimental Biology 212: 3737-3742.
LAI, A.; SCHACHE, A.G.; LIN, Y-C. & PANDY, M.G. (2014) Tendon elastic strain energy in the human ankle plantar-flexors and its role with increased running speed. The Journal of Experimental Biology 217: 3159-3168.
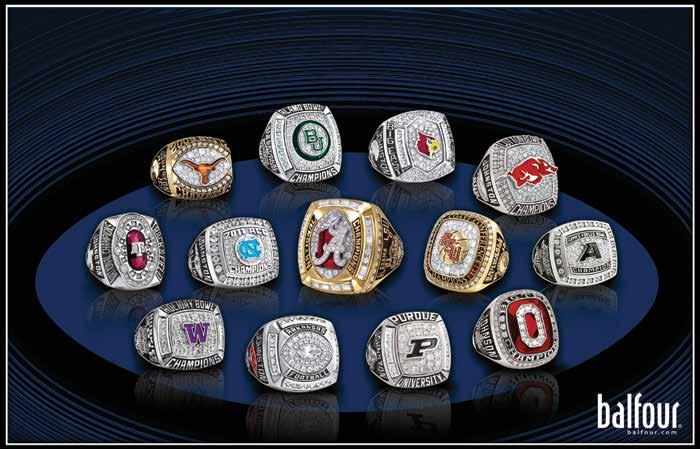
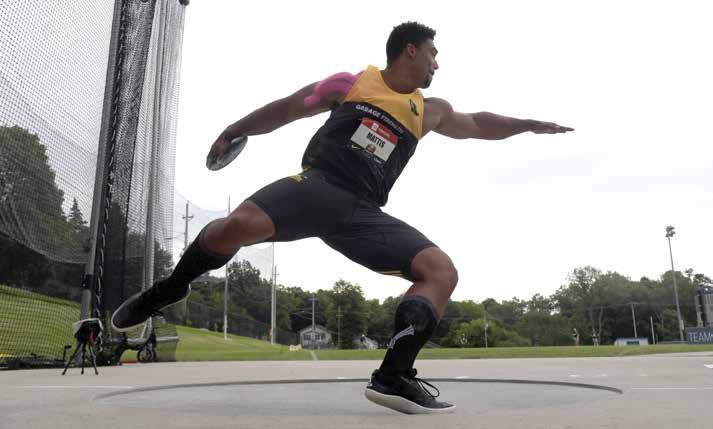
MOORE, I.S.; JONES, A.M., & DIXON, S.J. (2014) Relationship between metabolic cost and muscular coactivation across running speeds. J Sci Med Sport 17(6): 671-676.
MORITZ, C.T. & FARLEY, C.T. (2005) Human hopping on very soft elastic surfaces: implications for muscle pre-stretch and elastic energy storage in locomotion. The Journal of Experimental Biology 208: 939-949.
RAICHLEN, D.A., ARMSTRONG, H. & LIEBERMAN, D.E. (2011) Calcaneus length determines running economy: Implications for endurance running performance in modern humans and Neandertals. Journal of Human Evolution 60(3) 299-308.
ROBERTS, T.J. & AZIZI, E. (2011) Flexible mechanisms: the diverse roles of biological springs in vertebrate movement. The Journal of Experimental Biology 214: 353361.
PAAVOLAINEN, L.; HÄKKINEN, K.; HÄMÄLÄINEN, I.; NUMMELA, A. & RUSKO H. (1999) Explosive- strength training improves 5-km running time by improving running economy and muscle power. J. Appl. Physiol. 86(5): 1527-1533.
PERL, D.P.; DAOUD, A.I. & LIEBERMAN, D.E. (2012) Effects of Footwear and Strike Type on Running Economy. Med Sci Sports Exerc 44(7): 1335-1343.
SANO, K.; ISHIKAWA, M.; NOBUE, A.; LOCATELLI, E. et al. (2013) Muscle-tendon interaction and EMG profiles of world class endurance runners during hopping. Eur J Appl Physiol 113(6): 1395-1403.
SANO, K., NICOL, C., AKIYAMA, M., LOCATELLI, E. et al. (2015) Can measures of muscle-tendon interaction improve our understanding of the superiority of Kenyan endurance runners? Eur J Appl Physiol 115(4): 849-859.
SCHADE, F. (2010) Biomechanic services: a question of co-operation. IAAF New Studies in Athletics 25(2): 27-35.
STEARNE, S.M.; MCDONALD, K.A.; ALDERSON, J.A.; NORTH, I.; OXNARD, C.E. & RUBENSON, J. (2016) The foot’s arch and the energetics of human locomotion. Scientific Reports. 6: 1-10.
TAM, N.; SANTOS-CONCEJERO, J.; COETZEE, D.R.; NOAKES, T.D. & TUCKER, R. (2017) Muscle co-activation and its influence on running performance and risk of injury in elite Kenyan runners. Jour of Sports Science 35(2): 175-181.
THOMPSON, P.J.L. (2018) Kinetic Chains - Controlling stability and movement. The Body in Sport, UK Athletics Publications, England: 13.
THOMPSON, P.J.L. (2016) Current perspectives of Biokinetics in middle and long-distance running - an examination of the ‘Elastic Response’. IAAF New Studies in Athletics 31(1/2): 25-40.
THOMPSON, P.J.L. (2009) Introduction to Coaching - the Official IAAF Guide to Coaching Athletics. IAAF Publications, Monaco.
TUCKER, R. (2013) The Kenyan advantage: Is it calf elasticity? The Science of Sport Blog
WAGER, J.C. (2015) Assessment of elastic energy in the plantar aponeurosis and its contributions to human running. Diss. The Pennsylvania State University.
PETER JOHN L THOMPSON IS OF BRITISH BIRTH AND HAS COACHED FOR 52 YEARS, WORKING WITH ATHLETES OF ALL AGES FROM MIDDLE SCHOOL TO PROFESSIONAL OLYMPIANS AND WORLD RECORD HOLDERS. HE HAS BEEN INVOLVED IN CREATING AND DELIVERING COACH EDUCATION FOR 46 YEARS AND WAS ON THE 1982 FOUNDING COMMITTEE FOR THE TAC/USA COACH EDUCATION PROGRAM. HE HAS COACHED ALL THE DISCIPLINES OF TRACK & FIELD AND WAS THE FIRST COACH OF DALEY THOMPSON, 1980 AND 1984 OLYMPIC GOLD MEDALIST. HE HAS COACHED IN EUGENE, OREGON FROM 1976-1990 AND FROM 2011 TO TODAY. PETER HAS ALWAYS BEEN INNOVATIVE IN HIS COACHING AND SINCE 1978 HAS CONCENTRATED ON THE MIDDLE AND LONG-DISTANCE EVENTS, FORMALLY DEFINING THE NEW INTERVAL TRAINING METHOD IN 1995. IN DECEMBER 2016 HE FORMALLY IDENTIFIED, DEFINED AND DEVELOPED THE TERMS, ‘BIOKINETICS’, ‘THE KINETIC CHAIN’, ‘STIFFNESS AND ‘THE FOURTH ENERGY SYSTEM’.
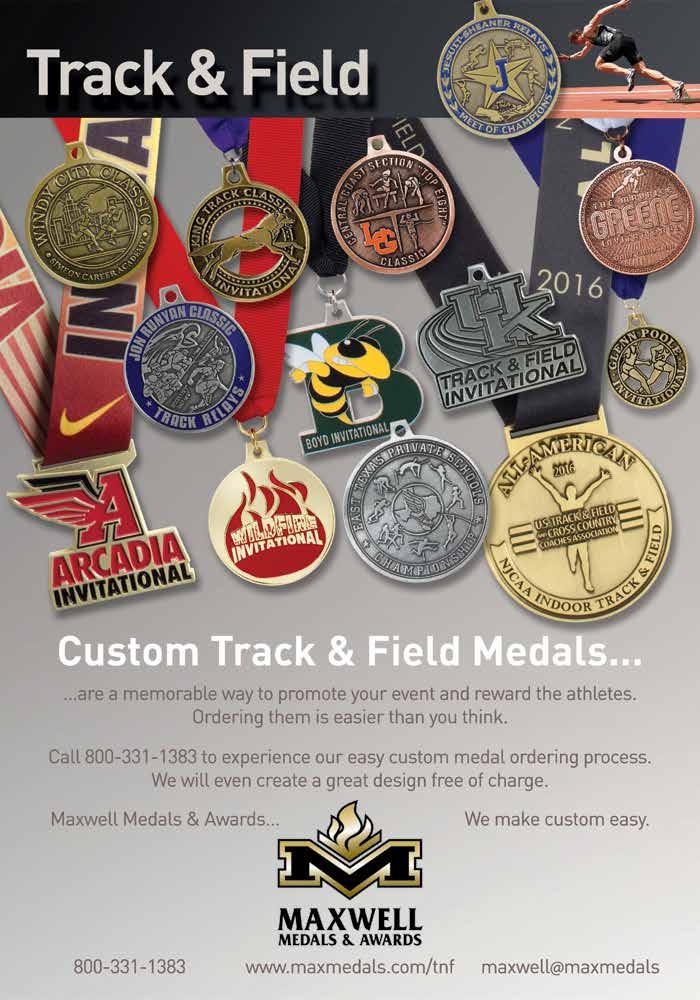