The vastness of the universe is unimaginable. Its shape is unknown; it may possibly be spherical or shaped like a flattened coin. Some cosmologists theorize that it may be one of many in a finite or even infinite multiverse system. There are a great many mysteries yet to be learned about the universe in which we live.
A spherical universe might be envisioned as a balloon with, in this analogy, us living at its center. Like the walls of a balloon as it is inflated, the surface area of the universe is expanding, its “edges” moving further and further away from the center point. Albert Einstein called this spherical model a “finite yet unbounded universe.”
On the other hand, a flat shape could produce an infinite universe. NASA’s Nobel Prize-winning cosmologist John C. Mather, Chief Scientist for the James Webb Space Telescope (JWST), suggested that the universe may be flat “like an [endless] sheet of paper . . . you could continue infinitely far in any direction and the universe would be just the same, more or less.”
Within our ability to observe our universe—up to as far as 92 billion light-years away—there are perhaps 2 trillion galaxies, but we are left to wonder how much more universe is out there, beyond our viewing capabilities. We do know that the universe is 13.8 billion years old, give or take a few million years, and that it probably originated with what scientists call the “Big Bang,” when a super-dense object smaller than a subatomic particle began rapid expansion. Our universe grows exponentially every day, with galaxies retreating from one another at everaccelerating speeds. Why, is a question yet to be answered. Additionally, only about 4.9 percent of the universe is composed of visible matter. The remaining 95.1 percent is made up of invisible dark matter and dark energy, which may only be theoretically studied. Since scientists know so little about dark matter and dark energy, the best they can do is extrapolate based on observations indirectly detected through the actions of gravity and other energy on visible matter. For instance, scientists understand through observation that our universe is expanding. But if the universe is made up of only galaxies, stars, and planets, gravity should be sufficient to hold things in place. Is dark matter and energy responsible for the expansion? This is yet another question to be pondered.
opposite NGC 346, a 200,000 light-years distant star cluster, was captured by the JWST’s Near-Infrared Camera (NIRCam) on January 11, 2023. Images such as this reveal the fundamental building blocks of galaxies, stars, and even planets, helping us to understand the vastness of the universe, and to characterize its matter.
THEORIES ABOUT THE SHAPE OF THE UNIVERSE
The spherical universe is not infinite, but it has no end, in the same way that there is no point on the sphere that could be considered an “end.”
The fact that our universe exists with the properties we observe today tells us that, very early on, the universe probably was very close to flat. If there were no dark matter or dark energy, a flat universe would expand forever but at a continually decelerating rate, with expansion evenually slowing to near zero.
Negative curvature of a universe is caused by a dearth of mass (manifested as gravity) to slow the expansion of the universe. In such a case, the universe will expand forever.
The Big Bang may be characterized as a “singularity” which, though it was no bigger than an electron, contained the totality of the matter of the universe. Its “explosion” set in motion the expansion of the universe and the formation of the matter in the objects currently observed in the cosmos. The galaxies that are ubiquitous in the modern sky are relative newcomers to the universe, formed a billion
years ago. In total, cosmologists estimate there are 221,373 galaxies in the local universe, within 2 billion light-years from Earth. This timeline of the universe, from the Big Bang to the present, flows from left to right. The universe has passed through several “eras.” Initially, the “Plank Era” occured from the Big Bang to approximately 10-43 seconds later. This is the closest we can come to the absolute
beginning of the universe. Next came the “Gut Era” and the “Inflationary Era,” still only 10-36 and 10-34 seconds respectively after the Big Bang explosion. Thereafter particles began to form, merging and changing over time to become the elements that make up our current universe.
BIG BANG
Cosmic microwave background (see page 46)
THE MYSTERY OF DARK MATTER AND DARK ENERGY
Even before the development of the Big Bang theory, scientists investigating the structure of the universe were confounded that their observations did not match the then current theories about the cosmos. They had to revise their ideas, especially in terms of the size of the universe and the amount of matter that was in it.
In 1884, eminent British scientist Lord Kelvin (1824–1907) theorized that of all the bodies existing in the universe “perhaps a great majority of them may be dark bodies” because of the discrepancy between observations and theoretical constructs. Gravity, a constant in the universe, was insufficient to explain everything observed by scientists, leading to questions of what other forces might be at work. In some cases, hot gases existed between matter, observable in the X-ray or gamma ray spectrum, but because of the actions of matter in the universe this phenomena seemed insufficient to explain everything in existence. This led astronomers such as Swiss scientist Fritz Zwicky (1898–1974) to theorize about dark matter and dark energy, so named because we do not yet have the instruments necessary to study them directly. They can be measured indirectly, however, and based on cosmological observations scientists have developed a theoretical model of the composition of the universe, in which roughly 68 percent of the universe is dark energy, dark matter makes up about 27 percent, and the rest, only about 5 percent, is normal, observable matter.
THE SEARCH FOR DARK ENERGY
In 1990 NASA deployed a new space-based astronomical observatory that revolutionized knowledge of the cosmos. The impact of the Hubble Space Telescope (HST) has been remarkable—observations over a thirty-year period have measured the expansion of the universe and offered a compelling explanation of the role of dark energy in the process. In 1998 scientists using HST data found a discrepancy between the expansion rate as measured just after the Big Bang and what the universe is experiencing now. Nobel Laureate Adam Riess (b. 1969) led a team of researchers who discovered that the expansion of the universe was accelerating. The expansion rate, known as the Hubble constant, was calculated by Hubble a century ago at 67.5 plus or minus 0.5 kilometers per second per megaparsec. Riess’s team used more recent observations to determine the speed was actually 73 kilometers per second per megaparsec. In Riess’s estimation, the universe’s expansion is accelerating based on dark energy still yet to be calculated.
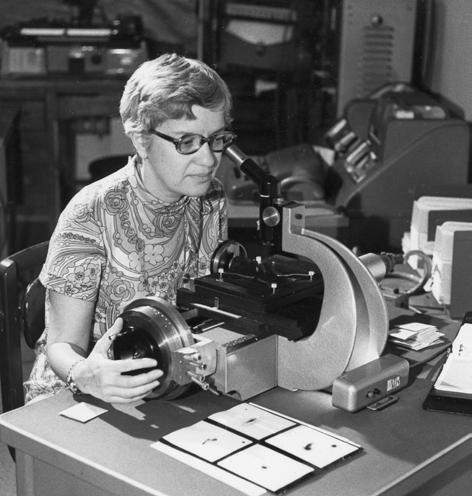
VERA RUBIN (1928–2016)
Vera Rubin, who had always wanted to be an astrophysicist, demonstrated that galaxies are composed mostly of dark matter. Her work with W. Kent Ford (b. 1931), beginning in the 1960s, led to the publication of pathbreaking observations on the rotation of spiral galaxies. Beginning with the nearby Andromeda galaxy, they investigated the flat rotational curves, finding that the outermost arms turned as quickly as those in the center. This indicated that spiral galaxies should fly apart if gravity was all that kept them in formation. Instead, a large amount of unseen mass—dark matter—held them together in a demonstration of the missing mass problem Lord Kelvin and others had theorized about. Galaxies contained five to ten times as much dark matter as ordinary matter. This discovery has been confirmed and reconfirmed many times since.
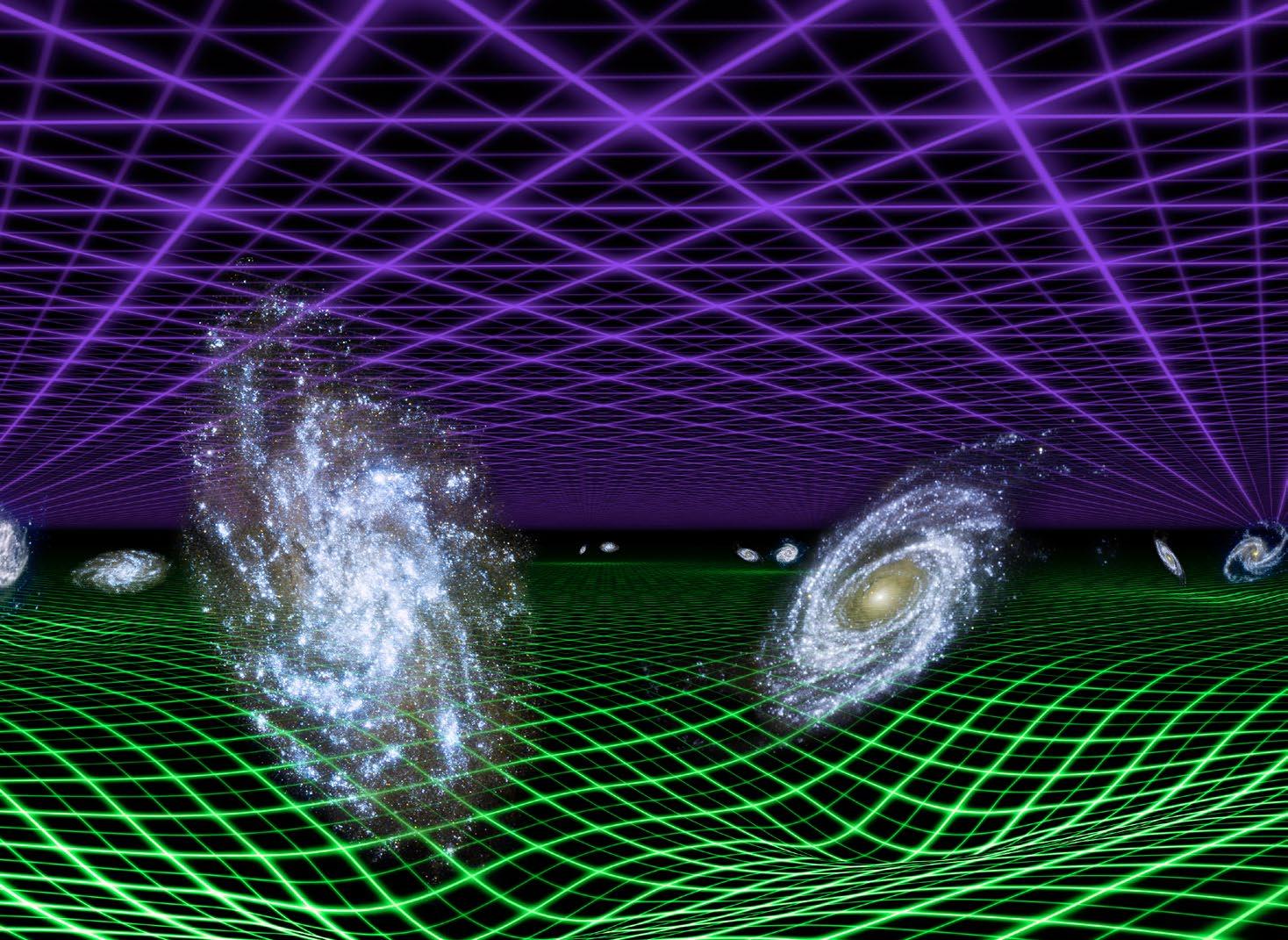
In this artist’s conception of the universe, the purple grid above depicts dark energy and the green grid below is gravity. While gravity is a constant presence in the universe, its effects are localized because its power over matter is directly related to the distance objects are from one another. Dark energy, on the other hand, is also constant but operates to push everything apart. At present, many scientists think that dark energy is the more powerful of the two forces.
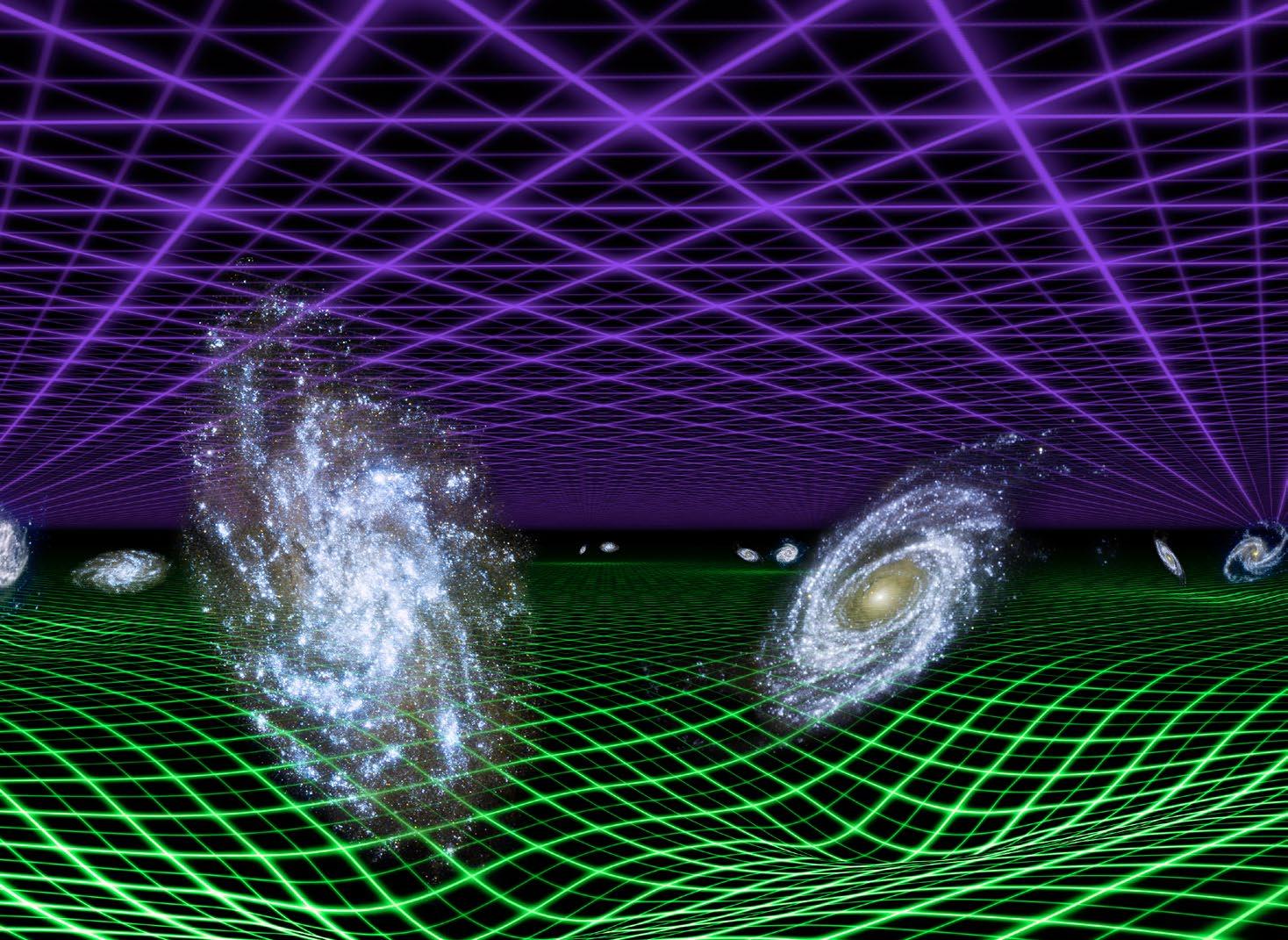
The missing matter of the universe has long perplexed astrophysicists, and revisions have been made based on the quality of the measurements enabled by scientific instruments. The ESA’s Planck spacecraft allowed scientists in 2013 to compute the percentages shown in the right-hand pie chart. It represented the most precise readings yet available, with normal matter making up stars and galaxies contributing just 4.9 percent of the universe’s total mass and energy. Invisible but nonetheless detectible through gravitational influence, dark matter accounts for 26.8 percent of the universe, more than previously thought. Dark energy is the most difficult entity to study, yet it is most assuredly accelerating the expansion of the universe. These measurements represent a slimmed down 68.3 percent of the whole of the universe, somewhat less than previously thought, as shown in the left-hand pie chart.
PREVIOUS ASSUMPTIONS
ESA’S PLANCK DATA (2013)
Scientists have theorized three potential fates for the universe. Unstable dark energy could cause a “Big Rip,” pulling things apart as the universe expands, destroying galaxies, stars, planets, and atoms. A “Big Crunch” might come as the expansion of the universe eventually slows, stops, and begins to contract. It would end in an implosion and compression. The third option most scientists discuss, the “Big Freeze,” is one in which the universe endlessly expands, eventually cooling and becoming nothingness.
BIG CRUNCH BIG RIP
Present
Big Bang
BIG FREEZE
above A white oval, different from the one observed in a similar position at the time of the Voyager 1 encounter, is situated south of the Great Red Spot on Jupiter. Voyager 2 took this picture of the region extending from the equator to the southern polar latitudes in the neighborhood of the Great Red Spot on July 3, 1981, from a distance of 3.72 million miles (5.99 million kilometers).
VOYAGERS 1 AND 2: JUPITER
Voyager 1’s closest approach to Jupiter occurred on March 5, 1979, when it discovered a thin ring around the Jovian planet, and two new moons, Thebe and Metis. The rings suggested that Jupiter had more in common with Saturn than previously thought. Voyager 2 followed and flew within 350,000 miles (560,000 kilometers) of Jupiter’s cloud tops on July 9, 1979. Over the next four months, it returned 17,000 photographs and a treasure trove of scientific data about Jupiter and many of its moons, including the presence of volcanoes on Io (Jupiter’s rocky moon).
GALILEO TO JUPITER
Following the Voyager missions, sustained exploration of Jupiter commenced on October 18, 1989, when NASA deployed the Galileo spacecraft from Space Shuttle Atlantis (STS-34) and set it on a gravity-assisted journey to Jupiter, arriving in December 1995. The first spacecraft to orbit the giant planet, Galileo conducted a multiyear encounter, sending scientific data about the density and chemical makeup of the giant planet’s cloud cover back to Earth.
Galileo captured imagery of Comet Shoemaker-Levy 9’s collision with Jupiter in July 1994, discovered a turbulent Jovian atmosphere—complete with lightning and thunderstorms a thousand times the size of those on Earth—and conducted close-
right Known for its Great Red Spot, Jupiter’s atmosphere also captures charged particles like the auroras on Earth. Using the Hubble Space Telecope (HST)’s ultraviolet imaging capabilities, this 2016 image captures the glow of high-energy particles entering Jupiter’s atmosphere near its magnetic poles and then colliding with atoms of gas.
above On June 11, 1974, Pioneer 10 approached the gas giant Jupiter, giving humanity its first close-up look at the largest planet of the solar system.
JUPITER FAST FACTS
mean distance from sun 484 million miles (778 million kilometers) or 5.2 astronomical units diameter 43,440.7 miles (69,911 kilometers)
density 1,270 grams/cubic centimeter
surface gravity 24.79 meters/ second squared (the speed at which objects dropped toward Jupiter will accelerate toward the planet; an object on Jupiter would weigh 240 percent that experienced on Earth)
rotation period (length of day)
9.8 hours
revolution period (length of year) 11.9 Earth years
mean surface temperature -234 degrees Fahrenheit (-145 degrees Centigrade)
natural satellites 80 discoverer Galileo Galilei, 1610
WHY IS MARS SO HARD?
As the three maps on the following pages illustrate, of the fiftyone missions to Mars over the course of the Space Age, only slightly more than half—twenty-six in total—can be considered fully successful. While this statistic has improved with time— missions to Mars have fared better than this 50 percent success rate since the 1990s—even in the years since 1992, eight of twenty-six missions have failed or partially failed.
So what makes Mars exploration so difficult? It is a complex question, but the distance from Earth and the state of human technology have much to do with this issue. Mars remains hard to reach, and difficult to navigate and investigate on arrival.
The history of missions to Mars offers a reality check for anyone who believes that we might easily send humans to the planet in the near term. Thus far, only nation state actors have undertaken missions to Mars. That may change in the future if sufficient profit motive for undertaking these missions is found. For most of the Space Age, Mars missions were undertaken only by the United States and the Soviet Union. That has changed in the last few decades. Six of the twenty-six missions since the end of the Cold War are by entities other than the USA or Russia. A broadening of actors will continue into the future.
The various types of robotic missions thus far undertaken to Mars range from flybys to orbiters to landers to rovers to, most recently, atmospheric flyers (see page 238). We will see an increasing number of landers, rovers, and flyers in the future.
SAMPLES FROM MARS
Returning samples to Earth has been a longstanding objective of Mars exploration. The logic of such a mission is obvious—it would allow scientists on Earth to study Mars samples using the resources of the best laboratories on the planet.
NASA has considered several sample return options over a long period of time, but as yet none have achieved formal development status.
New technologies to land on Mars, roam and recover samples, and then to return to Earth will be required. Nine successful landers have reached Mars, though no sample return flights have taken place. This may change by 2035—NASA, the ESA, and the national space agencies of China, Russia, and Japan have developed proposals to do so.
In this ambitious proposal for a sample return mission, a landing would take place near Jezero crater, where Perseverance (far left) is located.
NASA would then land a Sample Retrieval Lander (far right) carrying a Mars Ascent Vehicle, shown here launching a sample return capsule into Mars orbit, where it would rendezvous with an Earth return vehicle.
left Launched on May 5, 2018, the Mars InSight Lander is one of the most sophisticated probes to reach the Red Planet. Its mission was to measure interior, formation, and seismic activity such as meteor impacts.
InSight deployed an active seismic monitoring station (the circular object which may be seen connected by cable to the lander), the first such instrument sent beyond Earth. It monitored several “marsquakes” on the planet. This is one of the last images taken by NASA’s InSight Mars Lander, just a few days before the mission ended on December 11, 2022.