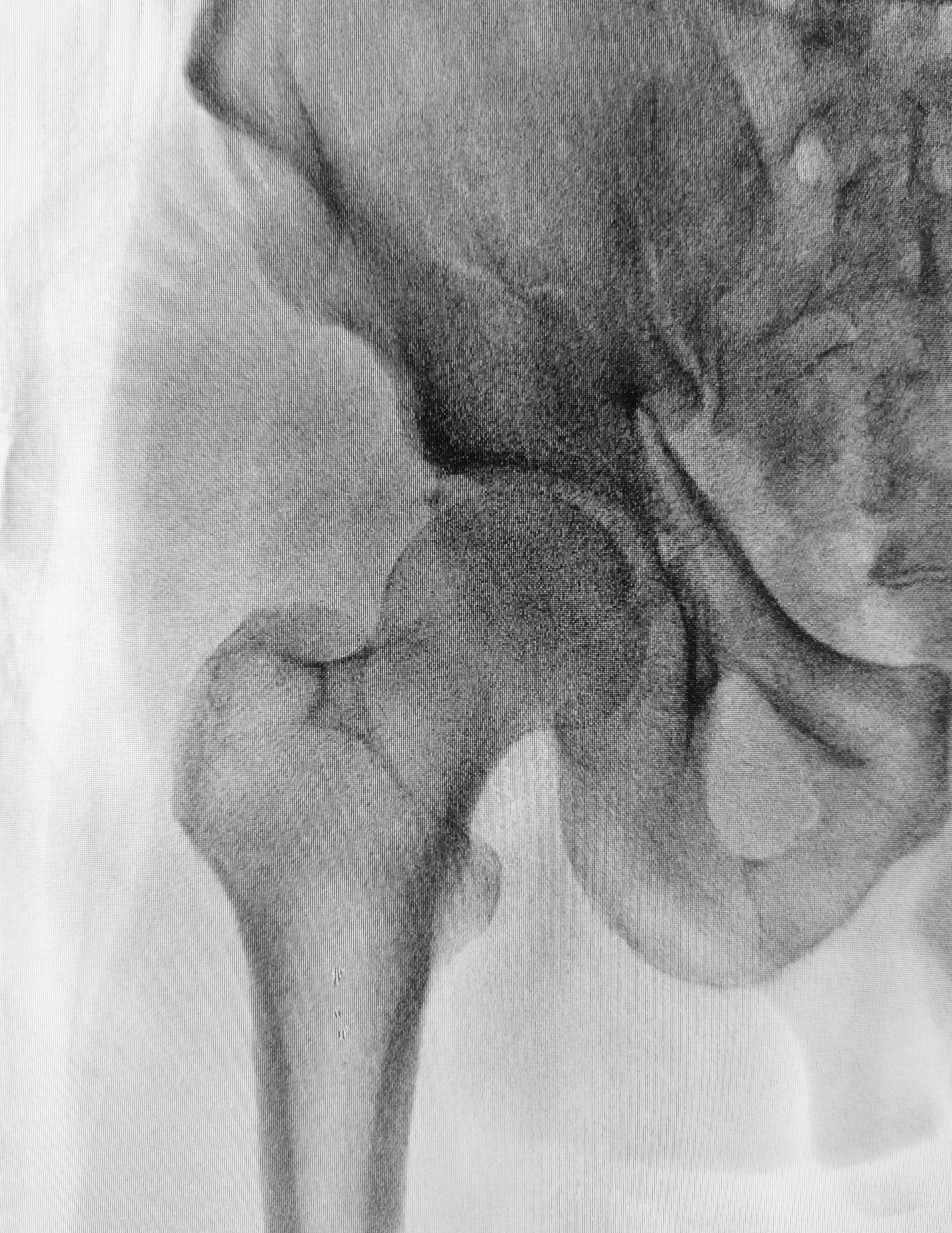
10 minute read
LAB-GROWN BONE: USING STEM CELLS TO SUCCESSFULLY REPLICATE THE NATIVE BONE HEALING ENVIRONMENT
By Rithika Adavikolanu ‘22
INTRODUCTION
Advertisement
An estimated 2.2 million bone graft procedures are performed annually worldwide due to bone diseases, osteoporosis-related fractures, trauma, bone malformations, and tumor resections.1 Autografts, where a patient’s own bone is harvested from another site, are considered the golden standard for bone defect treatment; however, the limited supply and potential complications at the donor site remain a significant problem. Allografts, bone from cadavers, are a widely used alternative, but these pose a serious risk of disease transmission.1 Bone tissue engineering seeks to develop better treatment alternatives for the large population with conditions that negatively impact bone healing.
The goal of bone tissue engineering is to fabricate scaffolds with the healing potential of autografts. A common strategy employs three-dimensional scaffolds, typically seeded with cells and other biological molecules, that promote desirable cellular interactions with their host to create an osteogenic environment. This technique is used to promote the formation of new bone to replace the scaffold.2 Bone heals best when it is in an environment that closely resembles the native tissue. One way bone engineers replicate the native tissue environment is by coating scaffolds or implants with a cell-derived extracellular matrix (ECM), a complex mixture of proteins secreted by cells. The ECM resembles a bone that is developing or healing as opposed to an adult bone. For example, there are certain types of collagens (e.g., collagen VI and XII) found in developing bone that contribute to bone formation, but these are absent in adult bones.
Human mesenchymal stem cells (hMSCs) are one of the most common types of cells implanted on
these scaffolds. hMSCs have the ability to differentiate into multiple connective tissues (mainly bone, fat, and cartilage) and are found in high quantities within bone marrow, which is widely used for healing difficult bone defects. In addition, there is emerging evidence that the immuno-suppressive nature of hMSCs, along with their differentiation abilities, makes them highly attractive for regenerative techniques, as transplant rejections are a huge limiting factor for cell-based therapies.3
One way to coat scaffolds with ECM is to seed stem cells directly onto the scaffold, allow them to deposit a protein matrix, then remove the cells from the scaffold leaving behind just the matrix. However, this decellularization process can negatively affect the mechanical properties of the scaffold and the bioactivity of the ECM. This project investigates a method to covalently tether the ECM to scaffolds without compromising the integrity of either the ECM or the scaffold. We aim to achieve this through a process called click chemistry, which is a highly selective covalent reaction that occurs between two chemical groups (in our case, azide groups and alkyne groups) to “click” them together.
METHODS
We used human mesenchymal stem cells (hMSCs), which have promising regeneration potential and are traditionally isolated from the bone marrow. However, hMSCs have limited proliferation abilities and high variation in potency depending on what donor or tissue they are sourced from. To bypass this, we derived our hMSCs from induced pluripotent stem cells (iPSCs) to produce induced pluripotent human mesenchymal stem cells (ihMSCs), allowing them to produce a plethora of cells originating from only one donor or cell source. We then introduced the small molecule GW9662 (GW) to our stem cells, which encouraged our cells to differentiate into bone cells. Importantly, the addition of GW also induces the secretion of ECM.
We utilized a process called metabolic labeling to incorporate an azide group, which is not naturally occurring in cells, into the ECM. We introduced our cells to L-azidohomoalanine (AHA), an amino acid
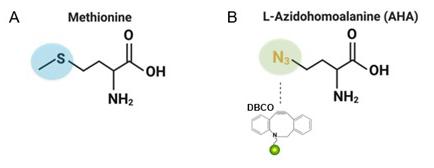
Figure 1. A) A normal methionine amino acid with a typical sulfur group B) L-Azidohomoalanine containing an azide group (opposed to the typical sulfur group) shown binding to an alkyne group tagged with a fluorescent dye.
containing an important azide group (Figure 1B). Cells recognized AHA as an alternative to a methionine amino acid (Figure 1A) and thus incorporated it into protein synthesis, meaning that every new protein produced is labeled with AHA. Azides react very specifically with alkynes such as dibenzocyclooctyne (DBCO), thus we can track the deposition of proteins by adding fluorescently labelled DBCO. We are using this same chemistry to bind our azide-containing ECM to a scaffold labeled with DBCO.
We prepared our scaffolds using a hydrogel polymer, gelatin methacrylate (GelMA), creating it in a two-step process to produce a hydrogel scaffold labeled with DBCO (Figure 2, top row). ECM was produced by introducing GW and AHA to our ihMSCs, which allowed the cells to differentiate into bone cells, encouraged the secretion of the ECM, and labeled our cell proteins with azide groups (Figure 2, middle row). The cells were allowed to grow for ten days to deposit an ECM made up of these newly synthesized, AHA-containing proteins. After the cells deposited the ECM, it was then extracted and the ihMSCs were removed. The decellularized matrix was consequently solubilized, leaving a protein stock to tether to the hydrogel scaffolds. Finally, we tethered the ECM to the DBCO-hydrogel scaffold by allowing the alkynes to react with the solubilized ECM containing AHA azides. The potency of the scaffold was tested by adding bone marrow derived hMSCs (BM-hMSCs) and measuring biomarkers for bone formation.
RESULTS
Verification testing was performed to ensure the successful binding of DBCO alkynes to the hydrogel scaffold. Since alkynes have a high reaction rate to azide groups, we used a fluorescent dye linked to an azide group to visualize the presence of DBCO alkynes. We placed DBCO functionalized GelMA in TAMU molds with a plain GelMA outer circle. The
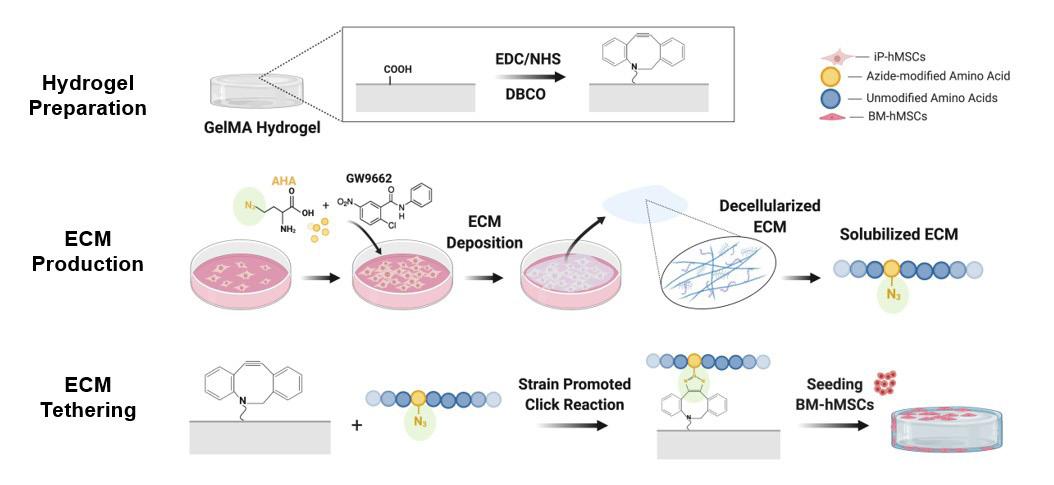
Figure 2. A visual depiction of the overall methods, with the first row illustrating the hydrogel preparation, second row illustrating the ECM production, and the third row illustrating the ECM tethering. Figure created using BioRender.
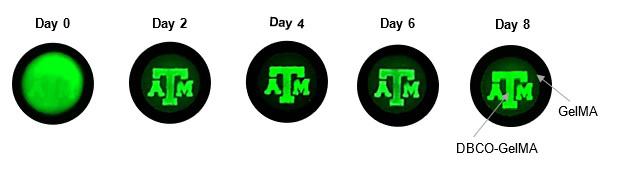
Figure 3. Plain GelMA and DBCO functionalized GelMA both placed inside a TAMU mold, treated with azide dye for two hours, and allowed to wash for up to eight days.
entire gel was dyed with an azide dye for two hours and allowed to wash for up to eight days to allow the unbound dye to completely wash away. After the conclusion of eight days, the DBCO functionalized GelMA was still fluorescent, indicating a stable reaction had occurred.
The ECM consists of high levels of collagen VI and XII, which play an important role in endowing osteogenic properties to the ECM. Therefore, when testing the properties of the ECM it is important to probe for the presence of collagen VI and collagen XII proteins to verify that the ECM is accurately replicating the healing bone environment. Furthermore, a major concern with click chemistry is that the composition and the function of the labeled proteins will be compromised. To ensure that feeding cells with AHA would not alter the composition of the ECM, we analyzed the ECM monolayers grown in an increasing range of AHA concentrations from 0 to 300 micromolar. After ECM deposition, we performed fluorescence microscopy to stain for the presence of AHA. As shown in Figure 4, we found a direct relationship between AHA concentration and resulting fluorescence, proving that AHA was effectively incorporated into the ECM. Furthermore, the expression of both collagens VI and XII was unaffected by increased AHA concentration, indicating that AHA does not alter the composition of these important collagens.
Additionally, to determine the effect of AHA on the expression of collagen XI and XII, a western blot was performed (Figure 5); which is a common laboratory technique used to separate and probe for specific proteins in a sample of several different proteins. The same experimental groups described above were used for the western blot in duplicates. Results showed that the addition of AHA had no effect on the presence of collagens VI and XII within the ECM. This further demonstrated that the osteogenic properties of the ECM remained intact even after the metabolism of AHA.
Finally, to determine if the GW and AHA added to the cells affected the protein yield in the ECM, we
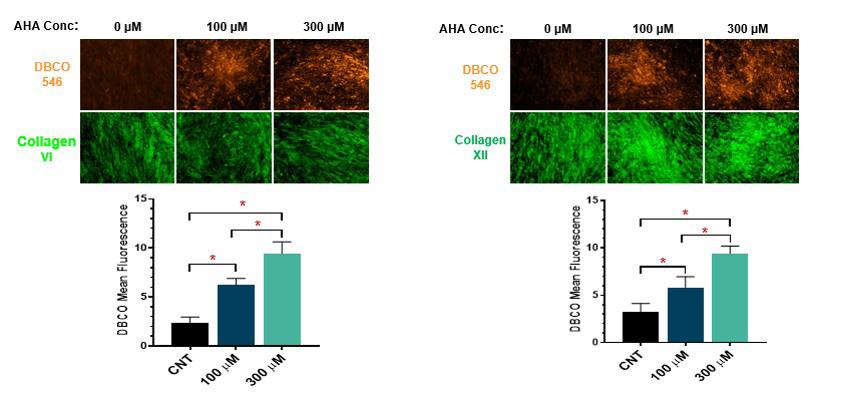
Figure 4. Fluorescence microscopy done on ECM monolayer in increasing AHA concentrations from 0 to 300 micromolar for both collagens VI and XII proteins.
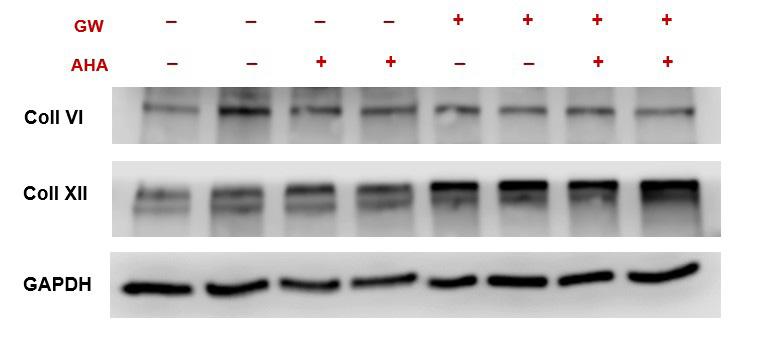
Figure 5. Western blot protein quantification bands for proteins of interest: Collagen VI, Collagen XII and GAPDH (housekeeping protein, loading control).
Figure 6. Quantification of protein concentration to observe the effect of GW and AHA on protein expression in the ECM. Four experimental groups were used: DMSO (control), DMSO and AHA, GW, and GW and AHA.
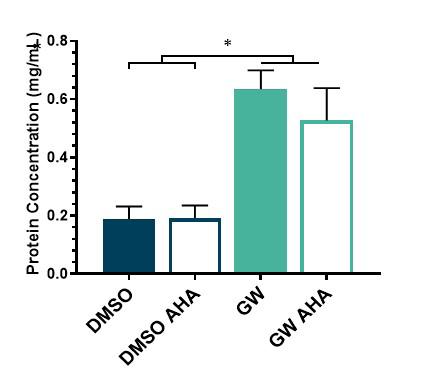
performed a bicinchoninic acid assay which quantifies the amount of protein present in each sample. We evaluated four groups: DMSO (control), DMSO and AHA, GW, GW and AHA for total protein expression present in each sample. Results showed that GW treated samples produced three-fold more ECM proteins than DMSO controls. This is a desirable outcome as GW was added to promote the secretion of the ECM and is therefore expected to yield a greater output of protein. Results also showed that the incorporation of AHA did not affect the ECM protein deposition, which is also desirable as the addition of a new metabolic group should not alter the inherent properties of the ECM proteins.
CONCLUSION
Unlike current bone treatments, such as autografts and allografts, our method aims to replicate the natural process of healing bone rather than simply replacing damaged tissue with fully formed bone. Furthermore, by using actual ihMSC-derived ECM, we are able to preserve the richness of the natural bone healing environment and enhance the osteogenic properties of biomaterials. The goal of this research is to covalently tether the osteogenic ECM to a hydrogel scaffold without negatively affecting its osteoinductive properties. In this work, we have begun to apply metabolic labeling and click chemistry techniques to develop a novel biomaterial ECM-scaffold. A simple interpretation of these results leads to the conclusion that GW treatment provides an avenue to stimulate ihMSCs to deposit higher levels of ECM than DMSO controls. More importantly, our AHA (methionine substitute) can effectively be incorporated into the osteogenic ECM and we confirmed that AHA does not affect the number of proteins our ihMSCs produce or its composition (collagen VI and collagen XII). Our results show that ihMSCs seeded onto the decellularized, AHA-containing ECM experience the same osteoinductive effects observed from a standard osteogenic ECM. Our work thus far provides the foundation for an ECM labeled biomaterial decorated with DBCO, which we hope to achieve in the near future.
ACKNOWLEDGEMENTS
I would like to thank Dr. Roland Kaunas for encouraging me to pursue various research opportunities and allowing me to take part in his research lab for the past four years. I would also like to thank my graduate mentors, Eli Mondragon and Berkley White, for their continued guidance and support throughout the course of this research project.
REFERENCES
1. A. R. Amini, C. T. Laurencin, and S. P. Nukavarapu, “Bone tissue engineering: recent advances and challenges,” Critical Reviews
in Biomedical Engineering 40, no. 5 (2012): 363–408. 10.1615/critrevbiomedeng.v40.i5.10.
2. V. Campana et al. “Bone substitutes in orthopedic surgery: from basic science to clinical practice,” J Mater Sci Mater Med 25, no. 10 (2014): 2445–61. https://doi.org/10.1007/ s10856-014-5240-2.
3. Amini, Laurencin, and Nukavarapu, “Bone tissue Engineering,” 363–408.
4. R. A. Hortensius and B. A. C. Harley, “Naturally derived biomaterials for addressing inflammation in tissue regeneration,”
Experimental Biology and Medicine 241, no. 10 (May 2016): 1015–24. https://doi. org/10.1177%2F1535370216648022.
5. C. M. McLeod and R. L. Mauck, “High fidelity visualization of cell-to-cell variation and temporal dynamics in nascent extracellular matrix formation,” Scientific Reports 6, (2016): 38852. 10.1038/srep38852
6. S. Viswanathan et al., “Soliciting strategies for developing cell-based reference materials to advance MSC research and clinical translation,” Stem Cells and Development 23, no. 11 (2014): 1157–67. 10.1146/annurev-cellbio-100913-013132.
7. B. H. Clough et al., “Bone regeneration with osteogenically enhanced mesenchymal stem cells and their extracellular matrix proteins,”
Bone and Mineral Research 30, no. 1 (2015): 83–94. https://doi.org/10.1002/jbmr.2320.
8. E. P. McNeill et al., “Characterization of a pluripotent stem cell-derived matrix with powerful osteoregenerative capabilities,” Nature
Communications 11, (2020): 3025. https://doi. org/10.1038/s41467-020-16646-2.
9. S. Zeitouni, “Human Mesenchymal Stem
Cell–Derived Matrices for Enhanced Osteoregeneration,” Science Translational Medicine 4, no. 132 (May 2012): 132ra55. 10.1123/scitranslmed.3003396.
RITHIKA ADAVIKOLANU '22
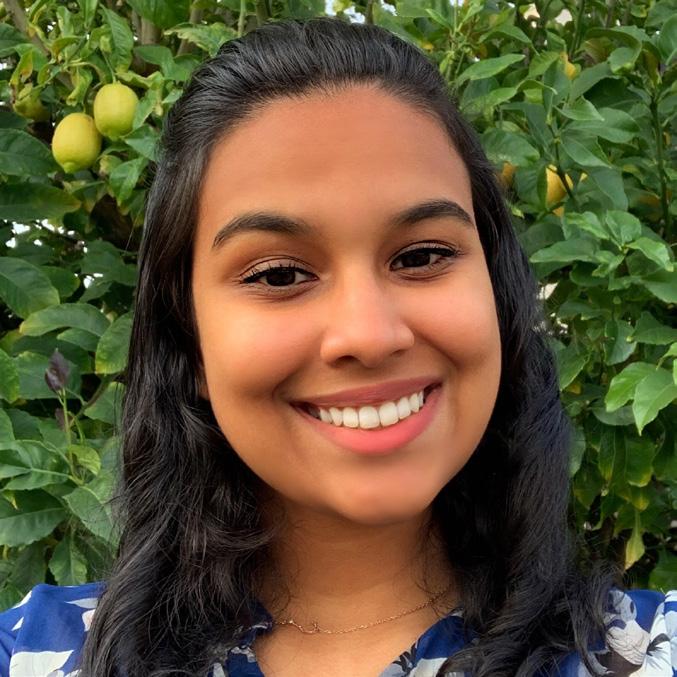
Rithika Adavikolanu '22 is a biomedical engineering major from San Francisco, California. She has been involved in undergraduate research from 20182022, where she has studied various topics within the field of regenerative medicine. After graduation, Rithika will be researching host-virus interactions at UCSF before pursuing her Ph.D. in genetic engineering.