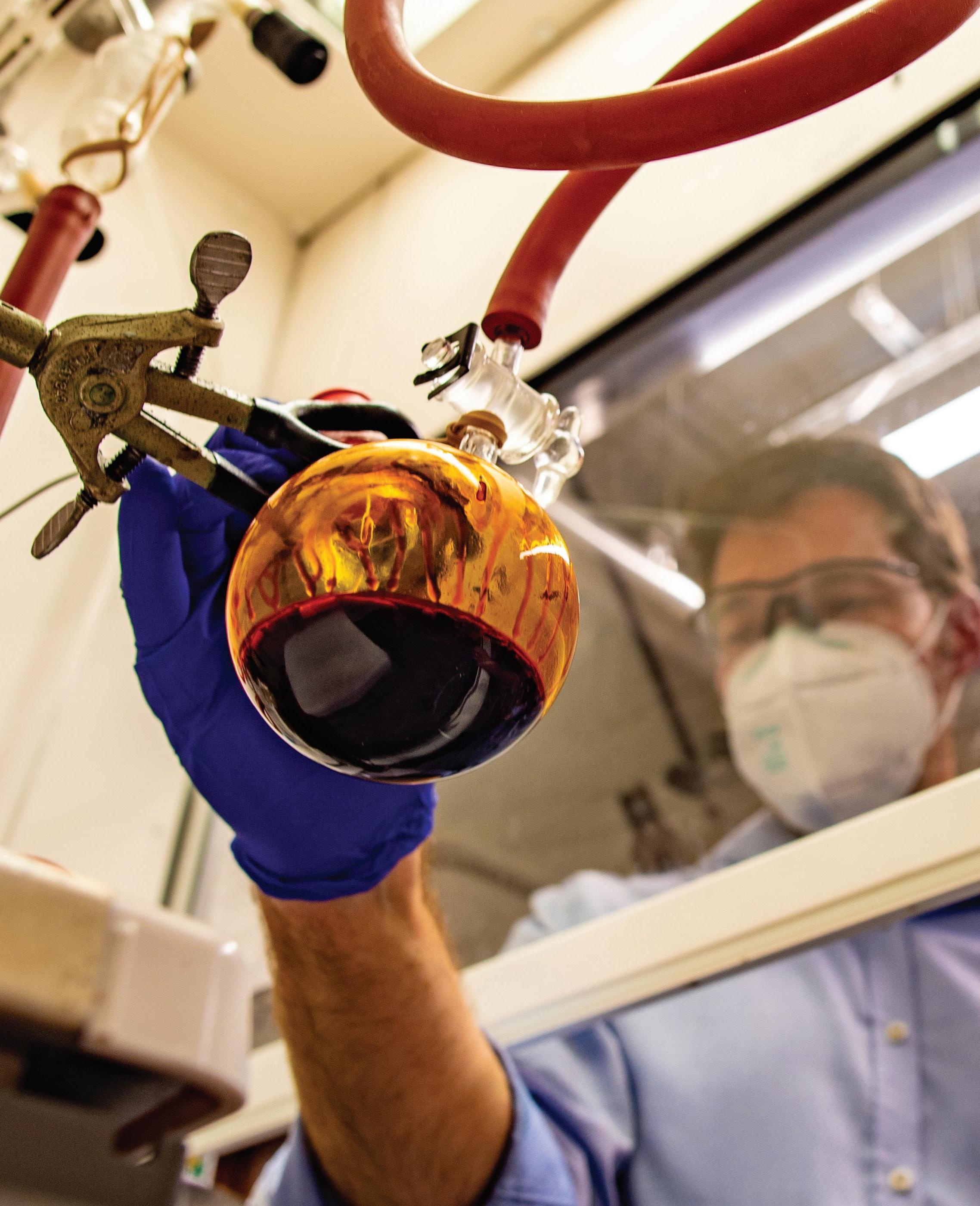
20 minute read
Fixing Phosphorus
We use phosphorus to grow food, make medicines, manufacture electronics. It’s also a finite resource that comes from mining. “How can we not waste it?” asks Professor Rory Waterman. In his lab at UVM’s Discovery Hall, Waterman and his students are exploring the fundamental chemistry of phosphorus— uncovering new, greener ways to use this element more efficiently. Here, he works with a red solution of diphenyl phosphide, an anion of phosphorus.
30 | UVM MAGAZINE “When phosphorus runs off the land, or gets thrown away, it ends up in the ocean,” Waterman says. “And then we can’t get it back.”
Advertisement
FIXING
PHOSPHORUS
Story & photographs by Joshua Brown
In the beginning, there was no phosphorus.
The young universe was quiet and dark, awash in nothing but gigantic clouds of gas. Only after gravity had crushed together clumps of this hydrogen and helium, 100 million years after the Big Bang, did the first stars ignite. Burning through the end of their fuel, stars fall in upon them themselves—some exploding with the mind-boggling force needed to ram particles together into larger nuclei. The death of stars gave birth to the elements essential for life: carbon, nitrogen, sulfur, oxygen—and, most rare of these, phosphorus with its fifteen protons.
Phosphorus swirled in the dust that formed our planet and it may have been in the primordial soup from which life arose. “No phosphorus, no biology,” says University of Vermont chemist Rory Waterman (at left). Like superglue in RNA and DNA, phosphorus with sugar forms a backbone, holding together these essential genetic molecules. A triple dose of the stuff is at the heart of all life, by way of adenosine triphosphate, or ATP, the chemical compound every organism uses to store energy. “It’s so heavily utilized in agriculture because plants need it to grow,” says Waterman. It’s in each of your cells; it forms your bones.
But phosphorus also can wreck your day at the beach or turn a blue lake into a “nasty spinach smoothie,” says UVM doctoral student Katelynn Warner. That’s because phosphorus—when scarce—limits the growth of plants and cyanobacteria, the blue-green algae that Warner studies. It’s this ancient, primitive, photosynthetic bacteria that fouls beaches with gunk, poisons dogs, and drives out the oxygen that fish require. Excess phosphorus is almost always the cause of this damage to freshwater—including to Lake Champlain.
Each year, some fifteen thousand miles of rivers and streams deposit more than two trillion gallons of water into Lake Champlain. This input has, for millennia, carried phosphorus and other nutrients. But in the recent era of people—with our farms, lawns, sewage, soap, pavement, earth-moving, stream-changing, tree-cutting, house-building, and mining of phosphate rocks for fertilizer—the rate of phosphorus running into Champlain, and waters around the world, has skyrocketed. By one estimate, this flow off the land has tripled since pre-industrial times.
All water, eventually, flows to the sea, where phosphorus and other fertilizers have created numerous dead zones along the coasts of North America, Europe, and Southeast Asia—thousands of miles of once-teeming waters now starved of oxygen. While vital for the super-productive farming used to feed our planet’s nearly eight billion people, phosphorus also is a worldwide water pollutant, devastating ecosystems, wildlife, and water quality. In short, phosphorus is both devil and angel.
Across the University of Vermont, dozens of professors and students are probing the complexities of phosphorus, discovering how to get it to stay where it’s wanted and out where it’s not. To learn more, I took a series of short trips from campus late this summer and into the fall—dropping in on scientists in the field (and wetland, garden, sewage plant, and, yes, bay of Lake Champlain). Here are five snapshots of UVM researchers—working to fix phosphorus.
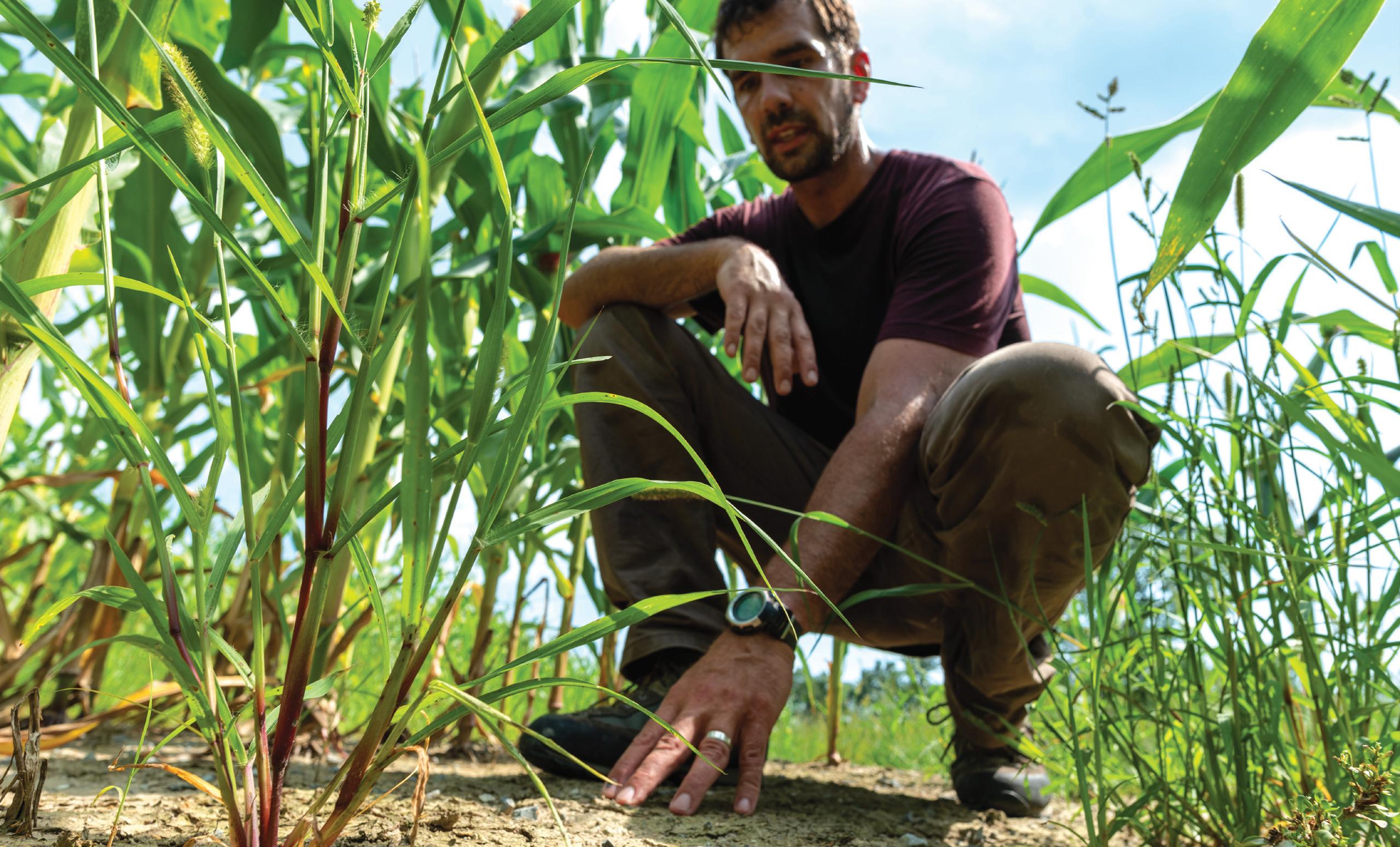
CORN FIELD
Panton, VT
In a corn field, just off Panton Road, outside Vergennes, Vt., it’s a bloody hot afternoon and Joshua Faulkner, a professor in UVM Extension, is down in a water-filled manhole in rubber boots. “It looks like this hose pulled out,” he says, and begins to feed a piece of clear tubing out of the hole while Ryan Ruggiero, a graduate student in plant and soil science, reconnects it through the sidewall of a fiberglass hut and into a water-sampling machine inside. Through a hedge on one side of the hole, we can see Dead Creek flowing, like too-milky coffee, toward Otter Creek and Lake Champlain beyond. On the other side, the tasseled corn forms a green wall.
They’re here to study phosphorus, how to keep it on this field and out of the water.
“A lot of this phosphorus comes into Vermont in the form of feed from the Midwest,” says Faulkner, pointing down in a row of corn to reveal large cracks spidering out through the soil. “We feed that grain to our cattle, and that gets in their manure.” Nutrients in a cow’s waste lets the corn here grow above our heads. “But if you apply manure to meet the nitrogen needs of the corn, you’re inadvertently applying more phosphorus than the crop needs,” Faulkner says. “So a lot of the phosphorus we bring in is not used by our crops, which means it doesn’t leave the state in milk and dairy products. It stays on the farms or in the waterways.”
Under this field, a network of drainage tiles keeps the soil from getting too waterlogged to work or from washing away during heavy rains now more common with a warming climate. Faulkner and Ruggiero’s study here aims to find out which conservation techniques—like reduced tillage and cover crops between rows—will do the best job of keeping the phosphorus on the land. Drainage may help too, but Faulkner—who runs the Farming and Climate Change Program in UVM’s Center for Sustainable Agriculture—is concerned the cracks in the soil could represent leakage points, where rainwater rushes down, carrying phosphorus underground and out to Dead Creek. Studies from the Midwest indicate that the effects of drainage are different than what Faulkner sees happening in the Champlain Basin. “We need local data, Vermont science to make Vermont policy,” he says.
Faulkner’s studies extend from this field to comparisons of whole watersheds. “There’s a lot of energy behind improving soil health on farms, but the assumption that improved soil health leads to improved water quality is not well established,” he says. “If we put all of these conservation practices to work on farms across a watershed, how much of an impact will we have at reducing phosphorus at the mouth of the Dead Creek or Little Otter Creek? We’re going to find out.”
Joshua Faulkner walks through the shimmering corn toward his truck. “I grew up on a farm. I love the natural world. I want to see those two co-exist and agriculture not adversely impact the other,” he says. “We all eat three meals a day. Where’s that food coming from? I want to still be able to produce food for a food system here in Vermont.”
WETLAND
Charlotte, VT
It’s early on a September morning, one of those days where the sun paints everything summer-gold, but the trees are dressing for fall. Eric Roy walks slowly over a hayfield near Spear Street in Charlotte, then down through a wooded hillside which suddenly opens onto a glittering wetland, a network of blue water under tall reeds and purple flowers. Two ducks touch down at the same moment that a heron lifts off. Roy is tired but smiles into the sunshine. He ran a long way yesterday, a 35-mile adventure from Mount Mansfield to Camel’s Hump on Vermont’s Long Trail. “There’s something magical about wetlands,” he says, describing a beaver-made swamp, near Taylor Lodge, that he stopped at on his run. “I don’t think tons of people organize their weekend around visiting obscure wetlands, but they’re really nice—and important.”
“This one is beautiful too,” he says. “There’s a beaver dam down there,” he says, pointing to where the meandering water disappears into the forest. Roy, a professor in the Rubenstein School of Environment and Natural Resources with a secondary appointment in the Department of Civil and Environmental Engineering, has five research plots here. He and his students in UVM’s Nutrient Cycling and Ecological Design Lab know that phosphorus washes into this wetland from upstream. They’re collecting data to estimate how much of it gets soaked up by soils and plants. It’s not news to scientists that wetlands improve water quality. But what is remarkable: this wild wetland, just a few years ago, was a hayfield. “Yeah, there was an agricultural ditch here—water would shoot right through and into Lewis Creek,” he says. A few minutes ago, we chatted with Sheila Burley, a farmer, whose family sold 150 acres of this land to The Nature Conservancy. “Dad used to clear that meadow, brushhogging, keeping everything down, getting stuck on his tractor,” she said. “Then the Conservancy come in and plug the river and plant trees all over, bring it back to its natural state. It’s ironic how things go around,” she said, laughing. “Still, it’s nice to not have fifty-five houses over back, taking another piece of Vermont.”
Eric Roy reaches down near the water and snaps off a piece of grass. “These plants grow each year; they’re taking phosphorus up into their biomass and then they deposit it,” he says. And the decaying plant matter holds phosphorus in an organic form less likely to escape back into the water when it floods. Across Vermont, numerous wetlands have been restored on former farmland. In many of these, Roy and his colleagues observe that the soil becomes more organic over time—including in this recovering wetland in Charlotte, where work to increase flooding has led to a buildup of organic wetland soils. And, as an ecological engineer, Roy appreciates the real water experts restoring this landscape. “The beavers came in and finished the job,” he says.
Except the job takes time. In 2016, the US Environmental Protection Agency, in collaboration with the State of Vermont, released a new ruling, with stricter limits, about how much phosphorus could flow into Lake Champlain under the Clean Water Act. For decades, the shallow bays of the lake have been receiving far more phosphorus than experts have deemed healthy—and more than allowed under the law. Numerous projects, regulations, and incentives seek to slow this flow. “The state is interested in what we’re learning out here,” Roy says, because officials would like to create a crediting system where landowners could be rewarded for restoring wetlands—based on the mass of phosphorus taken up by each acre. “Wetlands can be great at capturing phosphorus, but we have to be in this for the long run,” Roy explains. “Say you have a former corn field that’s been heavily fertilized for years. If you flood that soil, reconnect it to the river, there’s legacy phosphorus there that could escape.” In other words, some places may get worse before they get better. Eric Roy’s work in wetlands across the state is building a clearer picture of where investments might best be made. With the state’s Functioning Floodplains project, his team is creating maps of risks and opportunities for river corridors in Vermont. “We want to help guide restoration so that we maximize phosphorus capture in restored wetlands and minimize the release of legacy phosphorus,” Roy says. “Lots of phosphorus is out there from decades of land management. It’s going to take decades to fix.”
finished the job.”
- Eric Roy
Professor of environmental sciences and Gund Institute Fellow
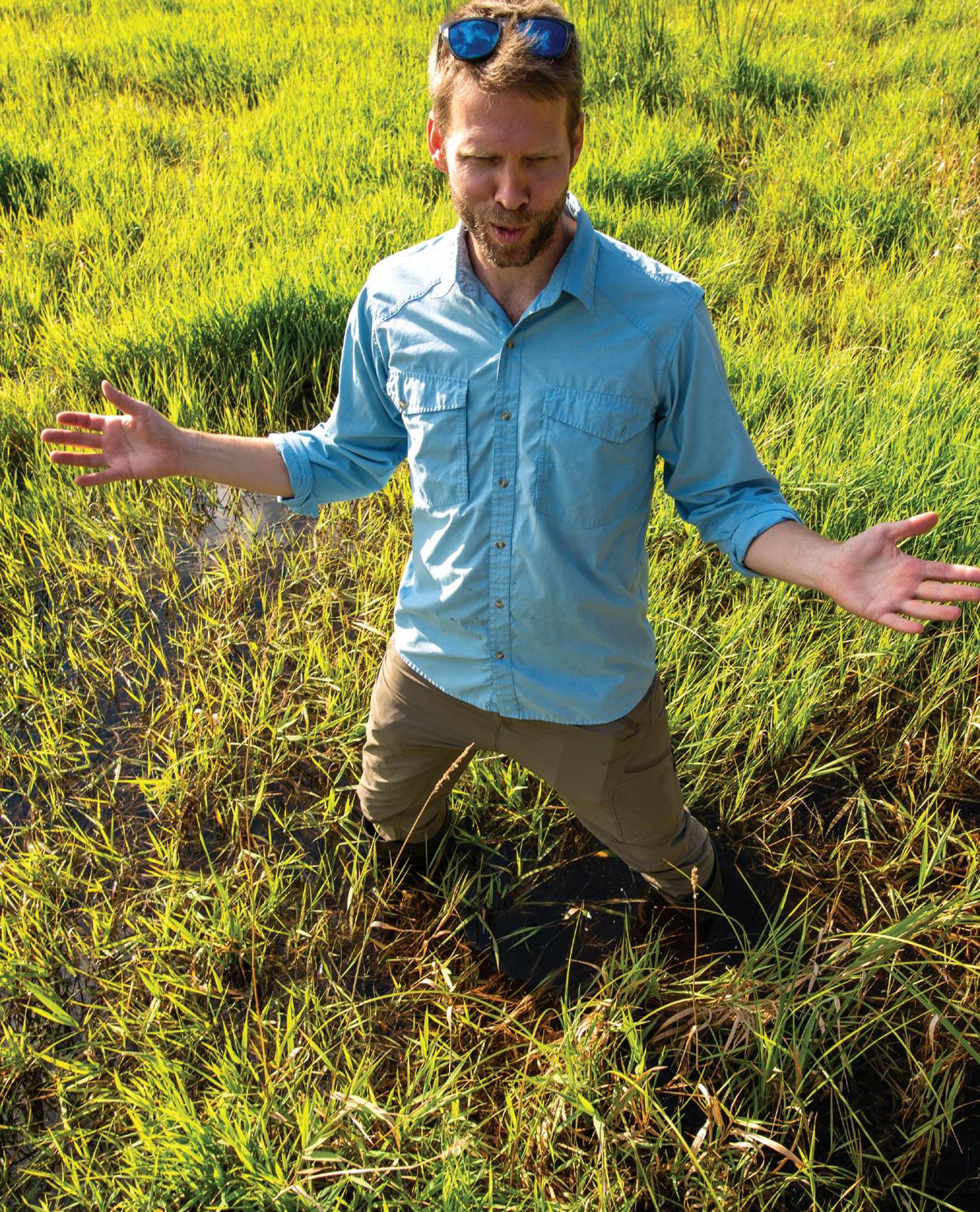
Essex Junction, VT
While phosphorus began in the explosion of stars, our understanding of it began in a bucket of urine. In 1669, the story goes, a German alchemist named Hennig Brand boiled down samples that had been kindly volunteered by his neighbors at the tavern. He was hoping this would help him create gold. It did not. Instead, he was left with a strange waxy paste that glowed in the dark. He had discovered the 13th element, phosphorus.
Three-hundred-fifty-two years later, environmental engineering graduate student Kamruzzaman Khan (at right) climbs a stepladder inside a large shed at the Village of Essex Junction’s Wastewater Treatment Facility, and reaches his hand down into a 150-gallon plastic reactor tank. Inside are powdery white crystals. Extracted from human waste that passes through this plant, this powder is pure struvite, a mineral made from phosphorus.
“We’re able to recover lots of phosphorus from a side-stream in the wastewater,” says Raju Badireddy, a professor of civil and environmental engineering, climbing up the ladder after Khan. “It’s clean, fertilizer-grade phosphorus,” he says, and a local farmer is eager to use it on his fields.
In recent years, towns and cities in Vermont have made major investments in improving their wastewater treatment plants. And the newest edition of the “State of the Lake” report from the Lake Champlain Basin Program, issued in June of 2021, shows that these facilities do a good job. Of the more than two million pounds of phosphorus that flow into the lake each year, the report shows, about 6 percent of it comes from wastewater facilities, far less than farms (38%), forestland (20%), streambank erosion (18%) or developed land (16%).
Still, a lot of phosphorus comes into the state’s 92 wastewater plants. “Everyone poops,” says James Jutras, the Essex plant’s manager. The two million gallons of wastewater that move through this plant in Essex each day are treated with microbes and some chemicals, and then discharged—with less than 0.00000267 ounces of phosphorus per gallon—back into the Winooski River. The microbial sludge that remains, however, is high in phosphorus. “When we process the sludge, we release a lot of those nutrients that we just captured,” Jutras says. “So we have to re-capture that phosphorus in a side-stream and treat it over and over again. It’s expensive.”
To extract this high-strength phosphorus, Badireddy and Khan, in partnership with Essex Junction, have invented a low-cost technology—using start-up funds from the Governor of Vermont’s Phosphorus Innovation Challenge. They call it Pe-Phlo (pronounced “pee-flow”) and they’re working to scale it from their lab bench tests, up to these demonstration-scale reactor tanks, to a permanent installation here. “That’s what that big tank is for,” Badireddy says, pointing to a 1500-gallon
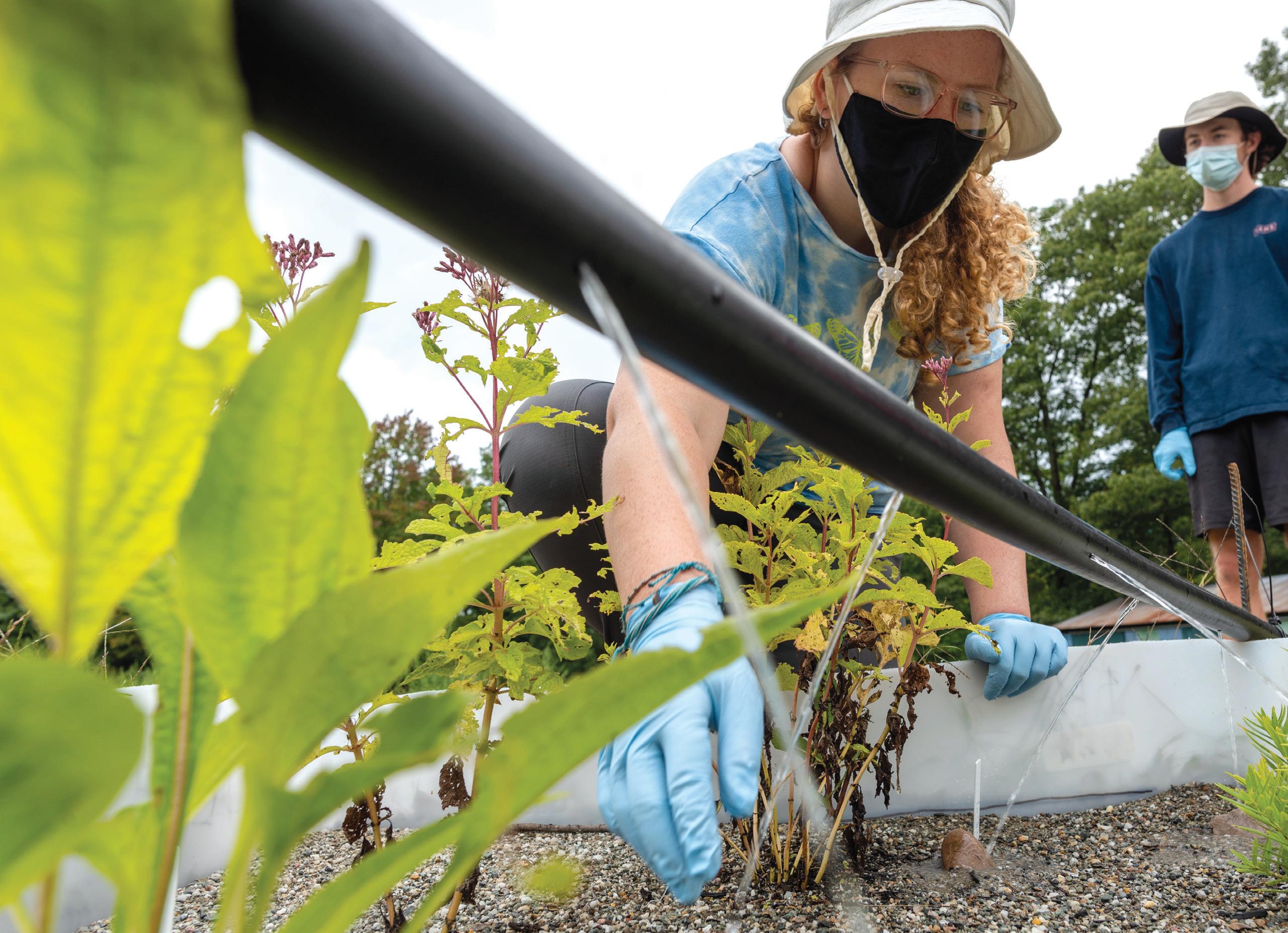
tank that reaches near the ceiling on the other side of the shop. “That’s enough quantity that we can bag it and send it to the farm.”
The Pe-Phlo project aims to create a portable and affordable device that can work on Vermont-scale wastewater treatment plants—and on farms too. First, the scientists repurposed a commercially available device normally used to prevent pipes from getting clogged. With its oscillating electrical fields, they precipitate crystals of phosphorus as struvite. At the same time, they apply another electrical field to the water in front of a customized membrane preventing it from clogging with these crystals. Out pours phosphorus-free water ready to be released—while, inside, fertilizer piles up. “Our approach takes a few minutes, versus the current standard which takes a few days,” says Badireddy, who directs UVM’s Water Treatment and Environmental Nanotechnology Lab.
Khan again climbs the stepladder and begins to adjust the mixer motor on the top of the tank. “Phosphorus is, right now, a non-renewable product. We collect it from mining,” he says. About 70 percent of the planet’s extractable phosphorus lies under the ground in Morocco where huge mines supply the raw material for fertilizer, and livestock feed, to many parts of the world. “The thing is, we’re going to run out of phosphate rocks,” Khan says. “If we can use it more efficiently, if we can recycle it, if we can get to net-zero phosphorus—that would be good,” he says. But, over the loud machinery, it sounds like, “that would be gold.”
A homemade downpour washes across the asphalt of a big city parking lot— under a blue sky at the pavement-free fields of the UVM Horticulture Research and Education Center. “You’re looking at the storm, right here,” Professor Stephanie Hurley says, smiling. She points at a hose streaming water from five holes into a low plastic basin full of flowering plants. The plants are half-submerged by this kneehigh rain burst. Behind her, Bryce Carleton ’22 adjusts the hose where it connects to a tall Rubbermaid tub raised on cinder blocks. “We filled this tank with a stormwater cocktail we developed in the lab,” explains Samantha Brewer (at left), a graduate student in plant and soil science. “There’s a predetermined concentration of four pollutants: nitrate, zinc, copper—and phosphate.”
Rain gardens and their fancy cousins—bioretention systems with underground pipes, like these here at the research station— have been increasingly used since the 1990s to catch stormwater runoff from rooftops, roadways, and the many impervious surfaces found in cities around the world. They use plants, layers of rocks, and engineered soil mixes to mimic nature—slowing and filtering the pulses of water and sediment (plus heavy metals and oils from cars, and those ubiquitous bags of dog waste) on their journey to the nearest stream, lake, or sea. “Basically, they create pockets in the landscape to soak up water the way it would have been before people paved the world,” says Hurley, who runs UVM’s Ecological Landscape Design Laboratory.
For this miniature rain garden, and eleven others just like it in a row, this is the first of twenty simulated storms they’ll have to endure over the next two years. Each will dump into the plastic basins the equivalent of one inch of rain sluicing across a parking lot. So far, the handsome-looking native plants—Joe-Pye weed, purple coneflower, and butterfly milkweed—look in fine form.
“We know Lake Champlain has a problem: too much effluent reaches the lake with phosphorus in it. Lots of rain gardens have been installed to try to help,” Hurley says. “We also know the sponge effect is happening; they’re soaking up the stormwater. But there’s a question we must figure out: are these rain gardens really solving the phosphorus problem?”
Hurley has good reason to think that many rain gardens may, actually, be adding to the problem. “One of the reasons is that people have been focused on the garden component of rain gardens,” she says, “and most gardeners, including me at home, use a lot of compost.” This compost is great for plant growth and beautiful flowers. “But most composts available, especially in Vermont, are manure-based. So they’re super-rich in nutrients like phosphorus and nitrogen.” Hurley’s research shows that rain gardens do, indeed, trap phosphorus particles attached to sediment. But then, without careful design, the water filtering through can pick up phosphorus from the rich soil and send a large dollop of dissolved pollution downstream. “The liquid phosphorus, that’s in soluble form, is very readily available for algae growth,” Hurley says.
That’s why she and post-doctoral scientist Paliza Shrestha G’17 are recommending revisions to the state’s stormwater manual—and are running this experiment to test different materials for rain garden plants to grow in: sand, a low-phosphorus top soil, wood chips, and an aluminum-rich material left over from drinking water treatment that binds well with phosphorus. “We’re looking for the least nutritious soil mix we can have for the plants, and still get them to grow and be healthy,” Hurley says, as she and Shrestha climb down into a trench where pipes collect the water that has flowed down through the rain gardens. “We’ll be able to see how much phosphorus comes out,” Shrestha says, “versus what we put in.”
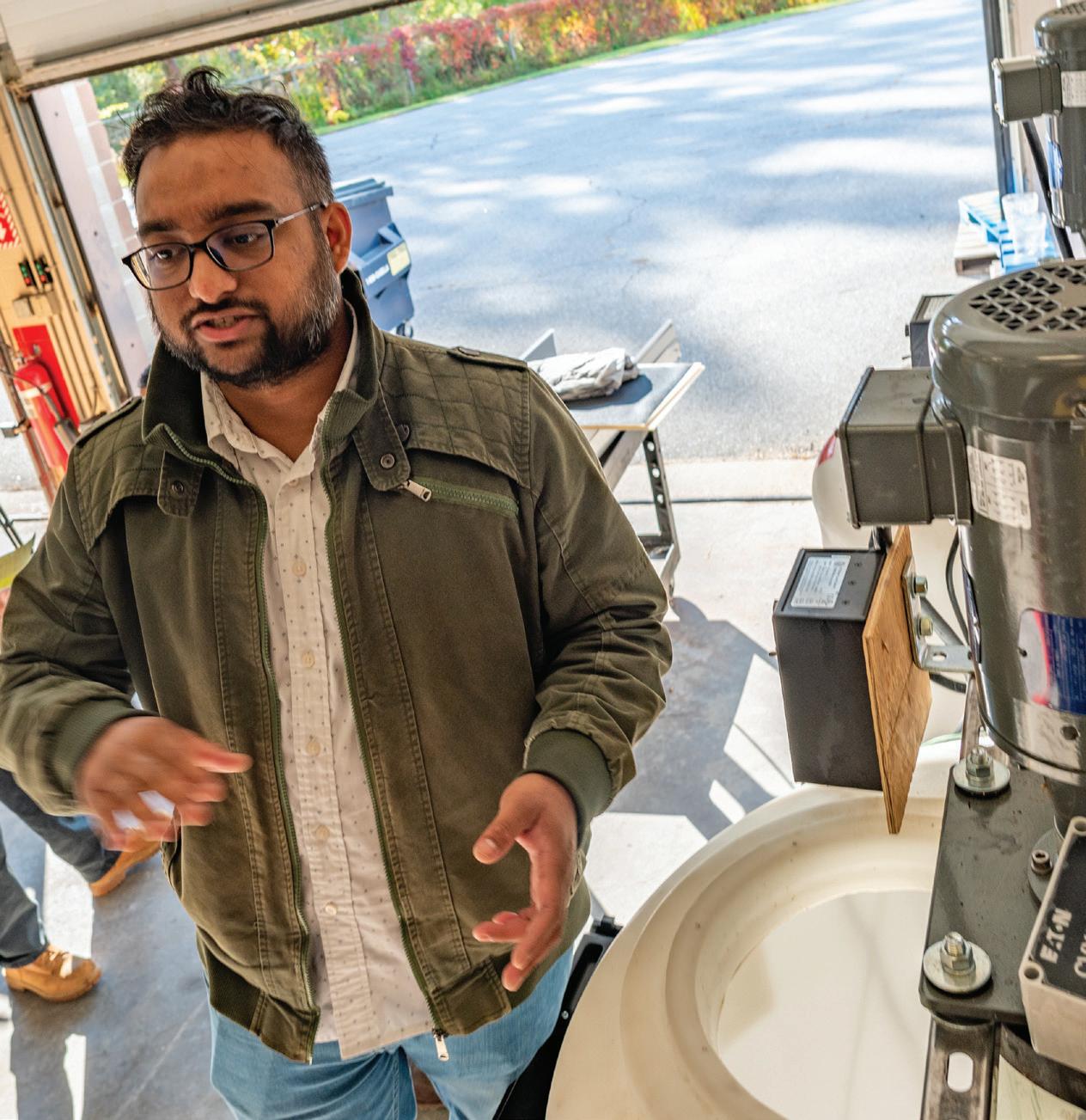
RAIN GARDEN
South Burlington, VT
EPSCOR RESEARCH BUOY
Missisquoi Bay, Lake Champlain
In the middle of Missisquoi Bay, an aluminum motorboat, a floating yellow platform covered with monitoring equipment, a research technician named Saul Blocher, a doctoral student named Katelynn Warner, and a seasick photographer—all bob up and down in rough chop. Perched starboard on the front of the boat, Blocher lowers a long blue tube on a cable into the lake. On the port side, Warner fills tiny bottles from a hose siphoning out of the water.
“Yep, that’s the algae,” Warner says, looking down into the blackish, pea-colored waves. Last week, this thick, green soup of life—largely made of cyanobacteria and other phytoplankton— was in full bloom during some hot days. “There were these speckled patterns and blobs everywhere. It was really cool, but really gross at the same time,” Warner says. “It’s too wavy for that today, but, still, I wouldn’t want to swim in there.”
She and Blocher are part of an extensive research effort, involving dozens of UVM faculty and partner organizations—with support from the National Science Foundation, Senator Patrick Leahy and others—that all aim to improve the water quality here in Missisquoi Bay, in Saint Albans Bay, and other impaired parts of Lake Champlain. And that means taking a deep dive to better understand how phosphorus works in these shallow bays.
Blocher (below) lowers the blue tube, a sophisticated probe called a sonde, under the waves. It takes readings of many aspects of water quality, including temperature, dissolved oxygen, total algae, and phosphorus. Blocher comes out here once a week to collect data—and to calibrate and clean the identical sonde that dangles underneath the research platform. That one lowers itself on a cable, moving through the water column, then sends data by satellite back to a lab on campus. When conditions are right for explosive growth of algae—or for pulses of phosphorus-laden sediment washing into the bay during Vermont’s increasingly common “monster storms,” as Warner calls them—these sondes can collect data every few minutes.
“This high-frequency data we’re collecting in the lake and rivers allow us to capture dynamics—like changing phosphorus concentration—that we would miss if we had to run around in a truck to collect samples,” says UVM geoscientist Andrew Schroth, one of the science leaders for Vermont EPSCoR (the Established Program to Stimulate Competitive Research) that operates this floating research station. The results that he and others have uncovered in this bay point toward solutions to the troubles on Lake Champlain and in algae-plagued waters around the world. The core fact is that phosphorus doesn’t just pour into Missisquoi Bay—it stays in Missisquoi Bay, cycling for decades. This accumulated phosphorus lies in sediment at the bottom of the bay, bound to iron or manganese minerals—“like rust on your car,” Schroth says— as long as there is oxygen. But on hot, windless summer days, the water stratifies into layers, and no fresh oxygen reaches the bottom. Then those minerals can dissolve, releasing their phosphorus into the warm water. “And that can feed a cyanobacteria bloom,” Schroth says. This algae consumes more oxygen as it grows and then more as the overgrowth dies and sinks to the bottom, killing fish and, sometimes, releasing dangerous toxins.
Warner—working with Professor Mindy Morales-Williams in UVM’s Rubenstein School—is out here to discover which cyanobacteria species are the culprits. “That’s why we’re gathering DNA from the water,” she says, carefully labelling the tiny bottles before they get flash frozen in liquid nitrogen. “We’re dealing with an organism that is one of the oldest on earth, that’s survived mass extinctions; it’s a formidable foe,” says Schroth. It may be that running air hoses to the bottom of the bay will help in the fight; Schroth is part of a project doing that now on Vermont’s Lake Carmi. It may be that letting aluminum powder settle to the bottom will help, “so that it’s right there, scavenging, when the phosphorus is released, catching it like a net,” he says. It may even be worth dredging the bay—a multi-million-dollar proposition. But to hit the EPA’s requirement—that phosphorus coming into Missisquoi Bay decrease by 64 percent over the next thirty years—will require more than any of these interventions. “We have to make hard decisions across the landscape and the economy, looking where phosphorus comes from—and investing not just in the lake, but in the whole watershed,” Schroth says. “And then we have to be patient.” UVM
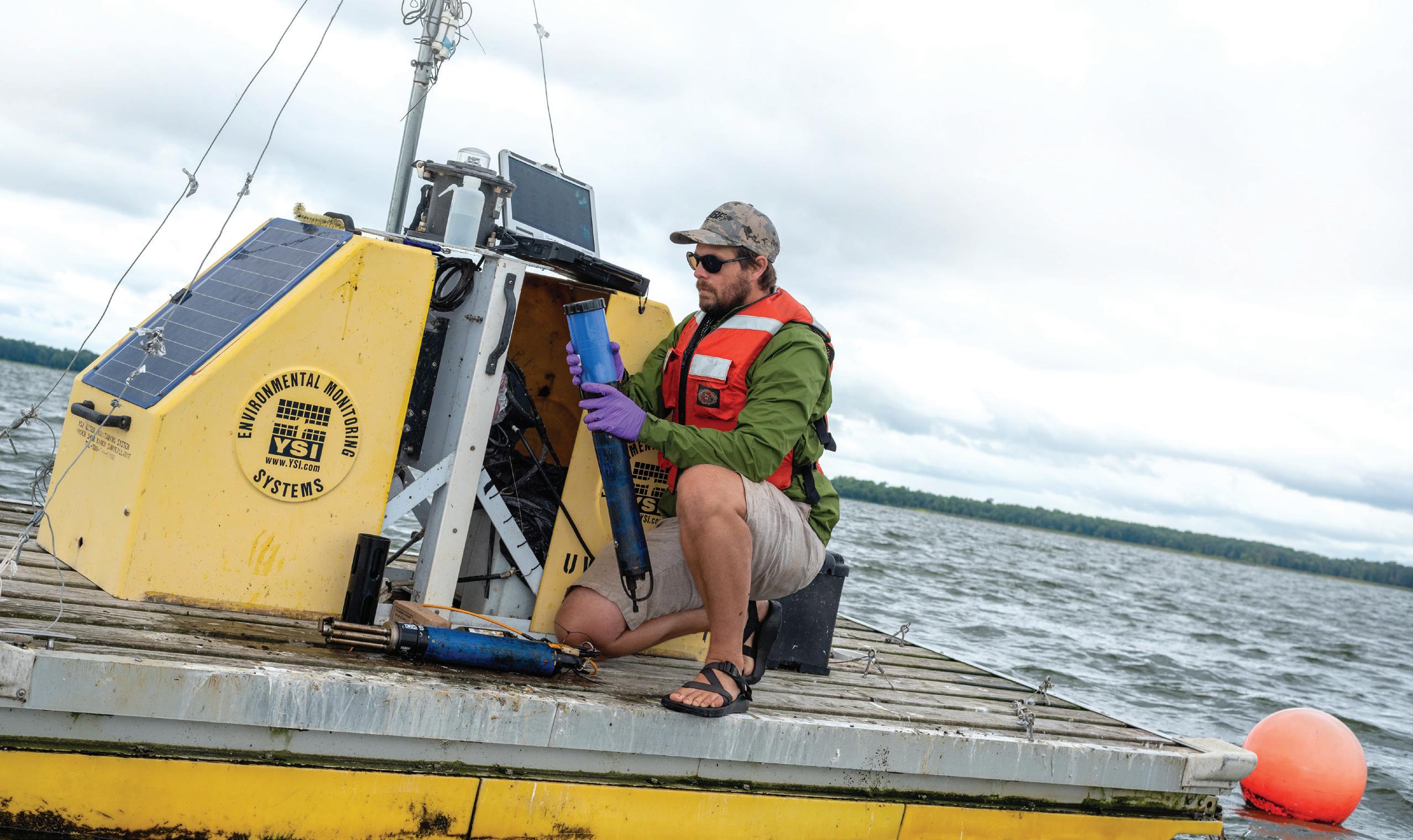
Doctoral student Katelynn Warner collects an algae sample from the shallow waters of Lake Champlain’s Missisquoi Bay.
FALL 2021 | 37
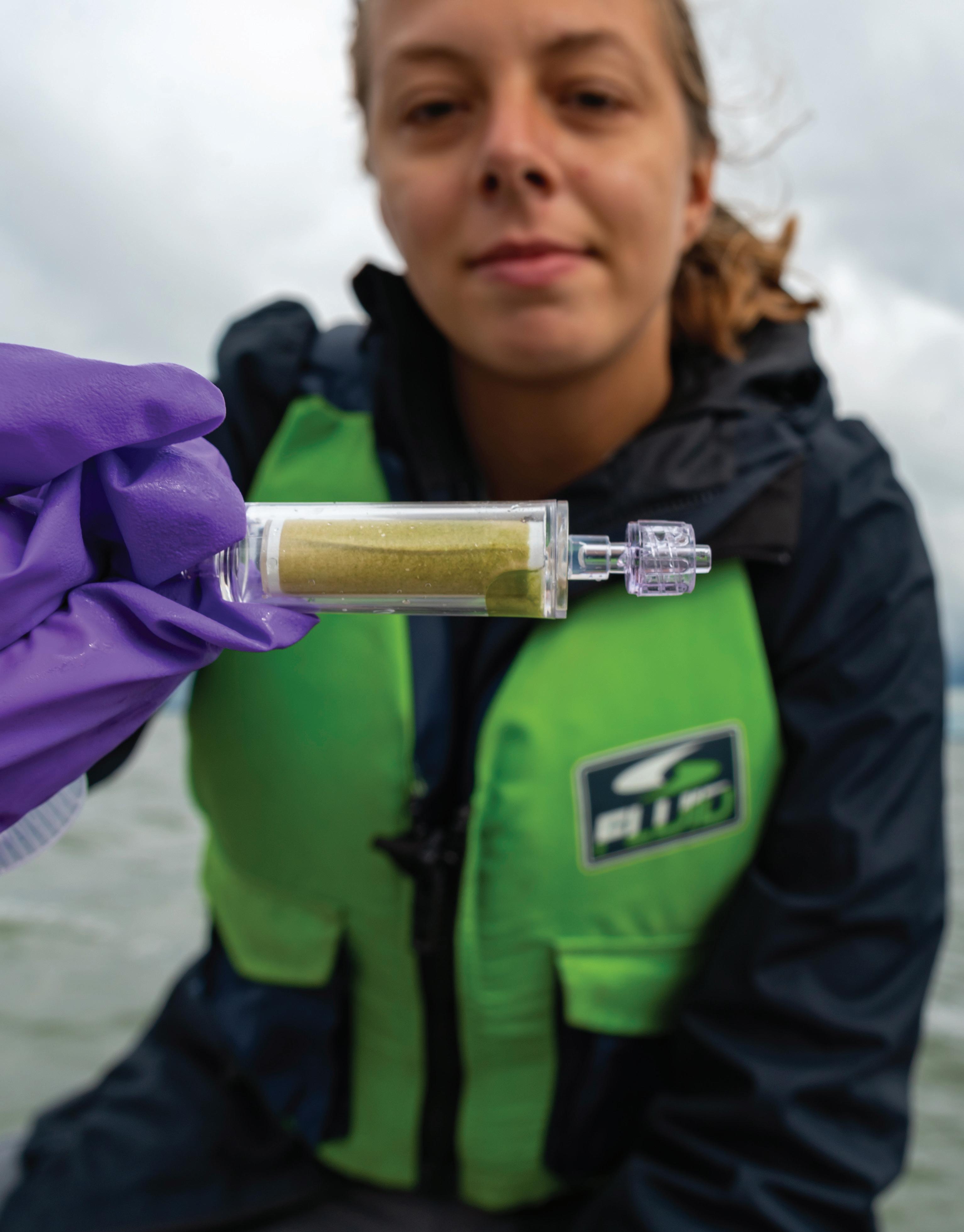