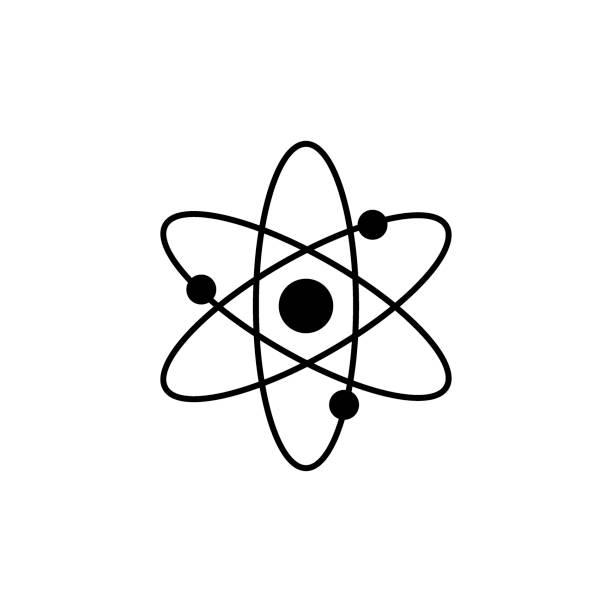
9 minute read
QUANTUMANIA
From Marvel to medical physics, everyone and their grandma seems to have quantumsomething these days. Is it just hype, or will new quantum physics really enable radically different ways of diagnosing and treating disease?
Not long after the Fulbright orientation this year I received a message from a US scholar that I’d spoken to about my work as a quantum systems engineer.
Alongside a blurb for the latest Ant Man and the Wasp movie, it read:
“Apparently they journey into the quantum realm. ‘Quantumania.’ Read it and weep”.
Trolling new acquaintances with silly job titles is an important cultural skill for Americans to learn when visiting Australia, so I was impressed by the efficiency with which my research was ridiculed, but it also prompted me to think more carefully about how the tag “quantum” is used and perceived. In the next few paragraphs I want to give my perspective, focusing on medical applications of my work, but touching briefly upon a few other areas where the role of quantum physics is either underappreciated or exaggerated.
I’m going to begin with a "quantum leap"
The phrase occupies a confusing space between metaphor and misnomer. To a physicist (or at least this physicist), a “quantum” usually means a discrete packet of stuff, often energy, but also sometimes charge, momentum, magnetic flux and anything else that is “quantized”, which turns out to be practically everything (except maybe space and time but please let’s not go there).
In this context, a quantum leap might be a proton flipping spin states or an electron transitioning between atomic orbitals.
These leaps are tiny in terms of the energy and length scales over which they occur, but quantum leap does not mean "tiny hop".
Usually, someone saying quantum leap means an enormous jump in progress. If a quantum leap is actually miniscule, it’s ridiculous that it has ended up meaning something gigantic.
That said, there’s something fundamentally and profoundly different between one quantum state and another, and in many ways our inability to properly comprehend or articulate that difference is the issue I’m doing a bad job trying to talk about.
Scientists are quite pedantic creatures and generally very careful about what they say and how they say it.
Each scientific field has its own jargon that provides shorthand for complicated ideas.
Quantum physics is an extreme field where even the fundamental building blocks have weird names because the concepts are so counterintuitive and alien.
Unfortunately that makes some ideas very hard to translate into everyday speech, and as a result it’s very easy to start using lazy analogies and bad metaphors that totally disregard any nuance conveyed by the original words.
Quantum tech companies are particularly guilty of this -- it seems simply replacing any C or K with a Q in an otherwise ordinary word to make it more quantum-y is considered par for the course, even if the result is that nobody can tell anymore which words have genuine scientific meaning and which ones are marketing.
Before going any further I need to make my own confession. I am guilty, at least by association, of a lack of nuance in the way I describe my work.
After two years of being called a quantum systems engineer I still don’t know what that means.
Similarly, our quantum sensor, while relying on quantum physics for its fundamental function, doesn’t carry out a measurement with many tell-tale characteristics of quantum physics phenomena.
To try to make sense of this mess I’m going to start by describing a quantum sensor.
Quantum sensors take advantage of quantum physics to measure signals that occur over energy or length scales inaccessible by conventional means.
Quantum sensing is linked to intrinsic physical constants and allows measurements to approach fundamental limits in sensitivity and accuracy. Quantum technologies harnessing such measurement approaches have the potential to enable applications impossible or impractical with traditional devices.
A good example showing how performing a measurement based on fundamental physics leads to improved accuracy can be found in the field of metrology.
Atomic clocks have been around since the middle of the 20th century, when it was still cool to name your tech as if it was radioactive. They are orders of magnitude more precise than their predecessors because the oscillation by which they measure time (their 'tick' if you will) is based on a fundamental property of the atoms inside them, whereas classical oscillators such as pendulums, springs and even quartz oscillators, have their tick set by some bulk material property that alters depending on lots of different variables that don’t stay the same over time, leading to different length ticks and causing the clock to eventually run fast or slow.
Most atomic clocks perform their measurements with millions of atoms at once inside a vapour cell at microwave frequencies. The latest type of atomic clocks developed in the 2000s, referred to as “quantum clocks”, probe laser-cooled single atoms at optical frequencies.
This is like performing a quantum measurement many times within a single tick, or, in other words, doing an ensemble of experiments on a single quantum object. So, while quantum clocks really are more quantum than the atomic clocks they seek to outperform, I think the argument that atomic clocks are a form of quantum sensor is still compelling.
Extending that logic into the field of medical imaging I want to talk about magnetic resonance imaging (MRI). MRI is based on the principle of nuclear magnetic resonance, but because the technique was developed into a clinical tool in the 70s “nuclear” wasn’t such a popular term anymore and the sub-atomic nature of the particles that generated the signal wasn’t emphasised. It’s even more disguised because when you do a quantum experiment on enough particles all at once you can get results that are well explained by classical physics. This means an MRI scan doesn’t look like a quantum experiment.
But fundamentally, every hydrogen nucleus in your body acts as a tiny quantum sensor, precessing about the external magnetic field at a rate proportional to the field strength.
MRI applies magnetic field gradients to encode spatial information with that precession frequency to make images.
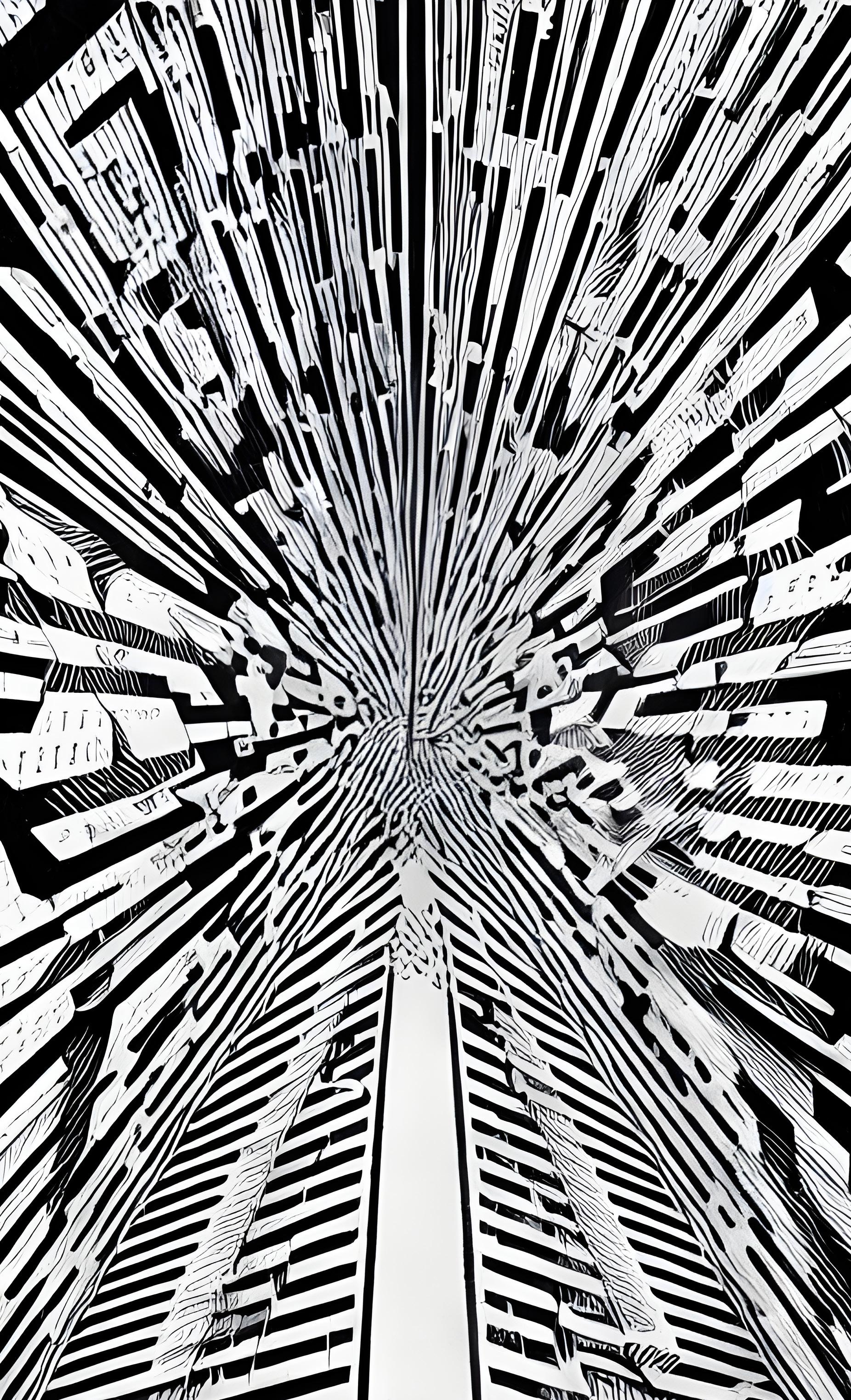
Depending on microscopic conditions, the way nuclear spins in the human body precess and get in and out of phase with each other varies, so if you’re clever you can make images that look different – that have contrast – depending on specific biological processes that affect local spins.
One such type of MRI, called functional MRI (fMRI), visualises changes in blood oxygenation by looking for the way that affects nearby hydrogen nuclei. By detecting areas of blood oxygenation with fMRI, doctors can infer that cells are doing lots of work in that area and this can give information about biological processes such as brain function. Using these non-invasive imaging techniques provides valuable insights into neuron activity, enabling us to detect impairment or serious illness without the need for surgery.
There are various methods, such as Magnetoencephalography (MEG), Electroencephalography (EEG), fMRI, and Near-infrared spectroscopy (NIRS), each with their own strengths and limitations.
MEG and EEG offer high time resolution but limited spatial resolution, while fMRI provides detailed images but operates at a slower time scale. NIRS is cost-effective but only captures surface-level blood-related changes, while fMRI can see those changes throughout the anatomy of the brain.
Combining MEG and MRI would be ideal to capture both fast, regional signals from neurons firing and slow, local, blood-related signals, but it poses challenges in terms of alignment and compatibility between superconducting devices used in MEG and conventional MRI.
To address these challenges, my research is focussed on developing something known as a nitrogen-vacancy (NV) diamond magnetometer.
NV-based sensors take advantage of the quantum properties of tiny imperfections in diamonds to make exquisitely sensitive measurements of magnetic and electric fields, temperature and mechanical strain. They are very robust and can operate at room temperature and high magnetic fields.
Because their sensitivity scales with the number of NVs, like MRI their quantum nature is often disguised in practice by the use of millimetre-sized diamond sensors that measure many NVs simultaneously.
They have so far been used in measurements in fields as diverse as cell biology, microwave engineering, and solid-state physics, but further advancements are needed in sensitivity, coherence times, readout fidelity, and diamond engineering before we can reliably use them in medicine.
The goal for my Fulbright research at the Martinos Center in Boston is to assist in the development of miniaturised and scalable arrays of NV magnetometers for use in MEG/MRI, revolutionising our understanding of brain activity and contributing to new diagnostic and therapeutic techniques.
Stepping back from my own work, and putting to one side the unimportant debate questioning if “quantum” things are really quantum physics, it seems clear that from medical imaging to metrology to computing, there are genuinely exciting emerging technologies that offer real promise.
Whether they are any more or less quantum technology than their predecessors is irrelevant. Because at the end of the day we (thankfully) don’t live in the quantum realm. We live in a real world where real technology makes a real difference.
And a bunch of new devices with elements of quantum physics at their hearts promise to do just that.
DR THOMAS BOELE
is an experimental quantum physicist with a keen interest in building prototype devices, and in the intersection of quantum physics with medical imaging. He received his Bachelor of Arts, Bachelor of Science (Hons I) and PhD from the University of Sydney, where he currently works as a quantum systems engineer. His recent research has focused on developing a quantum magnetometer based on fluorescent defects in diamond.
As a Fulbright Scholar, Thomas will collaborate with researchers at the Martinos Center in Boston to explore how the application of novel quantum sensors in medical imaging can enable new imaging modalities for improved disease diagnosis and treatment.