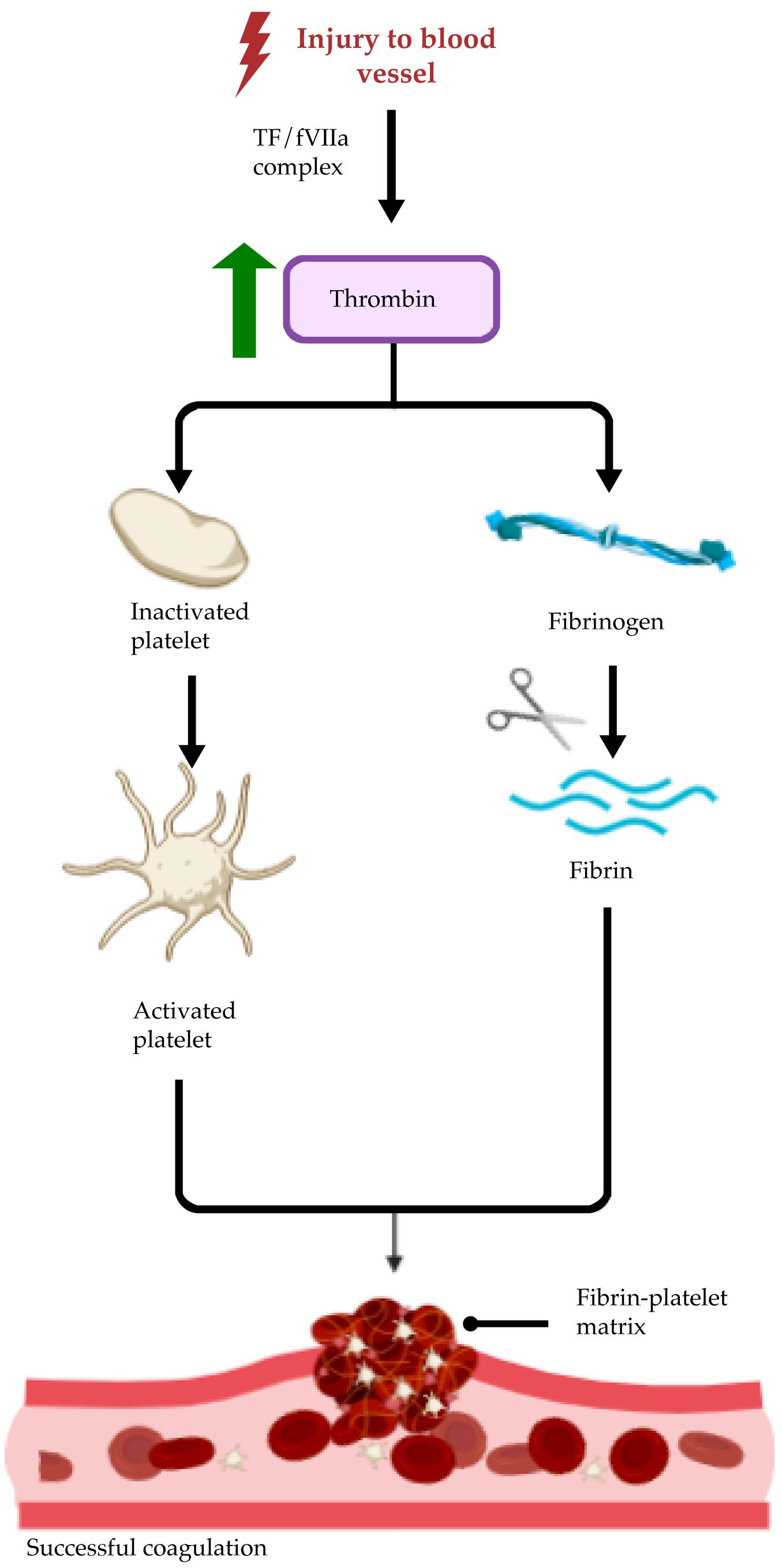
22 minute read
Endothelial Cell Diversity & Heterogeneity
from Scientia 2020
Arvind Muruganantham¹
¹Department of Biology, Baylor University, Waco, TX
Advertisement
Abstract
Given the extensive functional, phenotypic, and genotypic diversity of the endothelium, endothelial cell biology remains a poorly investigated area of research in relation to its relative importance to human health. As the lining of the body’s vasculature, the endothelium heavily mediates the coagulation cascade by controlling the activation of platelets through vWF presentation and formation of the fibrin-platelet matrix through TF secretion. Apart from maintaining proper hemostasis, the endothelium is also largely responsible for mediating immune response via leukocyte trafficking. Endothelium response to local cytokine signaling prompts leukocyte extravasation through chemoattraction (via cytokines), rolling adhesion (via CAMs), tight adhesion (via integrins), and transmigration (via PECAM). Furthermore, tumor angiogenesis, a process highly investigated due to its importance to tumor cell proliferation and metastasis,is also endothelium-mediated. By hijacking proangiogenic pathways, such as VEGF and cytokine signaling, cancer cells promote sprouting angiogenesis to the site of the tumor to obtain a steady supply of nutrients and growth factors. In order to carry out the endothelium’s wide-ranging functions, vascular endothelial cell phenotypes are influenced by a cocktail of developmental influences arising as early as amniotic development and environmental cues from the surrounding tissue. Adding another layer of heterogeneity, recent studies have shown phenotypically equivalent endothelial cells engaging in noise-mediated stochastic phenotype switching to create mosaic heterogeneity in endothelial cells arising from the same vascular bed. Further understanding the diverse and complex functions of endothelial cells, at both the cellular and population level, will provide insight into drug development for a myriad of diseases.
Introduction
The endothelium, the single layer of cells that lines the cavities of the body and its vasculature, is involved in many critical biological processes and disease pathologies. Endothelial cells are products of mesoderm-derived hemangioblasts that form cell clusters, known as blood islands, during amniotic development. The outer cells of the blood islands further flatten to differentiate into vascular endothelia while the core of the blood cells become hematopoietic cells (Dyer & Patterson, 2010). As the vascular endothelium progresses in the developmental process, it gains exposure to signals that results in the cell type’s widespread diversity and heterogeneity via environmental cues. Through a combination of paracrine signaling and cellular sensors, cells modulate their gene expression patterns to match that of their respective environments (Zhang & Friedman, 2013). Given the endothelium’s multifaceted roles throughout the body, the ability of endothelial cells to become highly specialized for their environment is crucial to maintaining proper vascular physiology.
Endothelium Mediated Coagulation
Serving as the lining of the body’s vasculature, naturally, the endothelium is heavily involved in many of the hemostatic pathways triggered by vascular injury. Typically, coagulation is thought to occur in three distinct phases (Versteeg, Heemskerk, Levi, & Reitsma, 2013). First, sub-endothelial collagen is exposed at the site of injury and mediates the initial round of platelet adhesion to the collagen surface via von Willebrand factor (vWF). Activated endothelial cells then secrete tissue factor (TF) to combine with activated circulating coagulation factor VII (fVIIa) to form the TF/fVIIa complex, which in turn increases thrombin levels to form activated platelet aggregates. Finally, thrombin proteolytically cleaves various coagulation factors and fibronectin to interlock fibrin chains into a cohesive fibrin matrix, thus creating an effective barricade to protect against infection and rapid blood loss (Figure 1). Endothelial cells serve as master regulators of the coagulation cascade through a two-fold mechanism: presentation of vWF on the luminal surface of vasculature to prompt activated platelet adhesion to the collagen surface and TF secretion to create a cross-linked fibrin matrix upon which activated platelets can form aggregates (Yau, Teoh, & Verma, 2015).
After the injury has been healed, endothelial cells also contribute to the breakdown of the dense platelet-fibrin matrix through the release of fibrinolytic enzyme activators, such as tissue plasminogen activator (t-PA)(Rajendran et al., 2013). In unison with other serine proteases, t-PA catalyzes the
Figure 1. Summary of the endothelium-mediated coagulation cascade. Endothelium-secreted tissue factor (TF) and circulating activated coagulation factor VII (fVIIa) form a complex to upregulate thrombin expression.
conversion of inactive plasminogen to its clot degrading isoform, plasmin (Tsurupa & Medved, 2001). Endothelial cell mediated t-PA release from internal Weibel-Palade bodies is essential to restoring tissue health and maintaining hemostasis (Hubel et al., 2002). Dense platelet aggregates bound to endothelial cell presented vWF are unlinked through the metalloprotease, ADAMTS13 (Turner, Nolasco, Tao, Dong, & Moake, 2006). In addition, constitutive ADAMTS13 secretion by endothelial cells remains an important source of circulating plasma ADAMTS13 during the post-injury repair response.
However, the function of the endothelium stretches far beyond simply serving as a lining for blood vessels and as a central mediator of the coagulation cascade. The endothelium’s role in inflammation has been a heavily investigated area of research in the past decade. Moving away from the notion that the endothelium serves only a menial role in the immune response, recent studies have shown that the endothelium plays a crucial role in regulating leukocyte transmigration and homing (Muller, 2013). Using the vasculature as a ‘highway’ for transport to sites of interest, circulating leukocytes are able to swiftly counter pathogenic threats present in the plasma. However, luminal membrane protein presentation by endothelial cells is responsible for the movement of leukocyte infiltration beyond the endothelial basement membrane to mitigate pathogenic threats past the endothelium (Sans et al., 1999). Immune response-mediated cellular adhesion molecule (CAM) presentation is controlled by paracrine cytokine signaling (such as interleukin-1 and tumor necrosis factoralpha) from the site of inflammation or infection. In response to cytokine signaling, endothelial cells increase the presentation of leukocyte ligand CAMs, such as P-selectin, to prompt rolling adhesion (Jung, Norman, Scharffetter-Kochanek, Beaudet, & Ley, 1998). Rolling adhesion is characterized by the slowing down of leukocytes on the endothelium as attractions between endothelium presented CAMs and leukocyte ligands are rapidly formed and broken. Once rolling leukocytes have decreased their movement considerably, tight adhesion takes place. During this stage, chemokines secreted by activated macrophages cause leukocyte surface integrins to switch from their rested low-affinity conformation to their activated highaffinity conformation (Sun et al., 2014). High-affinity integrinreceptor interactions lock the leukocyte in a tight adhesion, rendering the leukocyte immobile. Once tight adhesion has been achieved, leukocytes extend their pseudopodia and recruit platelet endothelial cell adhesion molecule (PECAM) to pull the leukocyte through gaps between endothelial cells (Vaporciyan, 1993). When it encounters the endothelial basement membrane, a combination of mechanical force and proteolysis is used to force the leukocyte deeper into the surrounding tissue. Since the recruitment of leukocytes past the endothelium is heavily mediated by presentation of leukocyte ligands on the luminal endothelial surface, various endothelial disorders can impact leukocyte movement throughout the body. For instance, in diabetic kidney disease, where high blood glucose levels diminish endothelial nitric oxide synthase (eNOS) levels, blood vessels are vasoconstricted in response to reduced nitric oxide production (Garcia-Garcia, 2014). As a result, in the glomeruli of the kidney, miniscule blood vessels are destroyed through sustained damage to the glomerular basement membrane via mechanical force induced by leukocyte infiltration. Thus, this greatly hinders the kidney’s ability to effectively filter blood.
Endothelium Mediated Leukocyte Trafficking
Role of the Endothelium in Cancer
Perhaps one of the most important and therapeutically
relevant functions the endothelium commands is angiogenesis. Angiogenesis is the process by which cells signal the endothelium in response to changing tissue requirements to sprout new blood vessels (Walsh, 1997). This process is relatively common in the body, taking place during development and during periods of rapid tissue growth. However, during cancer, tumor cells hijack the angiogenic pathway to siphon nutrients and blood via the vasculature (Jahroudi & Greenberger, 1995). Once the tumor has grown larger than a few millimeters in diameter, it begins to experience hypoxia and nutrient deprivation, as the existing blood supply is insufficient to satisfy the rapidly proliferating tumor’s needs. Thus, the tumor begins to release proangiogenic signals to the surrounding tissue in an effort to recruit vasculature to the area (Hida, Maishi, Annan, & Hida, 2018). Along with vascular endothelial growth factor (VEGF), the key growth factor responsible to recruiting endothelial sprouting and growth, tumor cells also secrete other cytokines and guidance factors to further remodel the surrounding vasculature such that the tumor is adequately nourished (Figure 2).
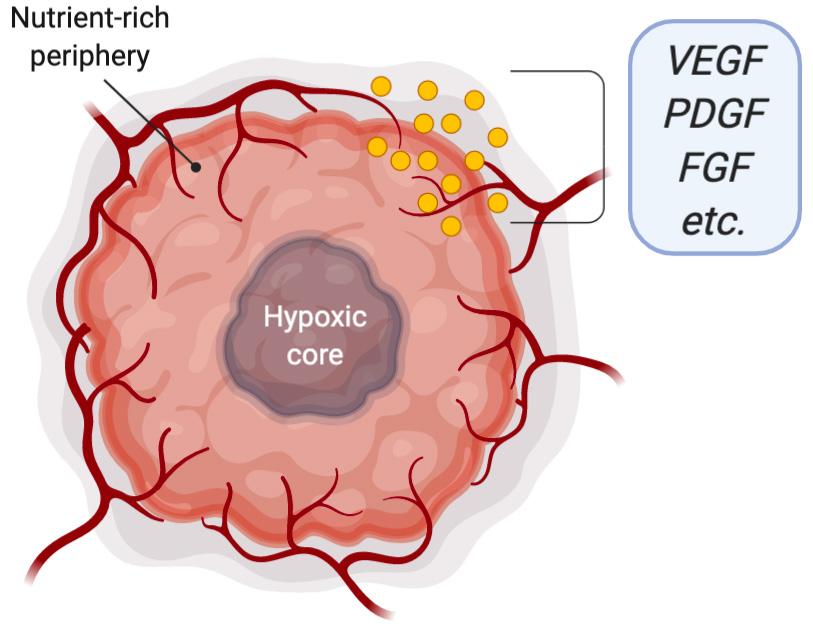
Figure 2. Tumor cells directly adjacent to vasculature are adequately nourished while the core of the tumor is hypoxic and nutrient-deficient. The tumor releases proangiogenic growth factors (such as VEGF, PDGF, FGF) to increase nutrient availability. VEGF, vascular endothelial growth factor; PDGF, platelet derived growth factor; FGF, fibroblast growth factor.
However, despite the elaborate signaling efforts of the tumor, tumor cell-recruited vasculature is often disorganized and prone to hemorrhaging due to the internal competition among tumor cells to obtain nutrients (Weis & Cheresh, 2011). As a result of this, tumor cell-recruited vasculature is organized only according to the intensity of proangiogenic signal released by cells that lie in certain areas of the tumor, partially explaining why cells at the core of tumors are hypoxic in nature (Muz, Puente, Azab & Azab, 2015). In response to this leaky new vasculature, the immune system prompts its wound healing response and deploys immune cells to the area (Weis & Cheresh, 2011). The accompanying inflammatory response further contributes to tumor angiogenesis via immune cell secreted cytokines. In unison with VEGF and chemokine signaling, tumor cells also release proteolytic enzymes that cleave segments of the extracellular matrix (ECM), exposing proangiogenic integrins and allowing cellular mobility for metastasis. After loss of cellular adhesion (primarily through VE-cadherin loss, ECM cleavage, and connexin loss), cancer cells that are able to infiltrate the blood supply often migrate to metastatic sites in a non-random fashion (Ben-Baruch, 2009). For example, breast cancers tend to metastasize to the bone and brain while colon cancers tend to metastasize to the lung and liver. In order to survive and thrive at the metastatic site, the cancer cell must be able to adapt to the difference in microenvironment between the site of origin and the metastatic site. Thus, while cancer cells are often able reach diverse areas of the body, only a small subset of those extravasions result in successful growth of the cancer cell colony due to microenvironment compatibilities.
Conventional forms of chemotherapy routinely used in clinical settings tend to lack specificity against cancer cells. As a result, cancer treatment is extremely demanding and greatly diminishespatient quality of life (Bhugwandass, Pijnenborg, Pijlman, & Ezendam, 2016). However, modern cancer therapies are attempting to inhibit tumor angiogenesis instead of directly targeting cancer cells that can easily be evaded by the host immune system and gain drug resistance. This approach seems particularly attractive since it serves as a method of conferring specificity on cancer treatment that has never been accomplished before (Weis & Cheresh, 2011). Since intense angiogenesis observed at the site of tumors is not highly typical in the body, targeting neovascularization in the body may be more effective at destroying tumors than conventional chemotherapy. However, there are limitations to this approach. Targeting proangiogenic pathways, such as through VEGF inhibition, is highly feasible, although consequences sustained through inhibition of non-canonical pathways must also be considered. In addition, cancer cells are often able to adapt and compensate for subtle changes in their microenvironment; for instance, an anti-VEGF therapy might prompt the tumor to upregulate chemokines and proangiogenic integrins to maintain a net proangiogenic status (Jin & Mu, 2015). Thus, targeting the endothelial cells responsible for angiogenesis may be a highly effective strategy for starving tumors of their nutrient supply, although conferring specificity exclusively to tumor-induced vasculature proves to be challenging.
Phenotypic Diversity of the Endothelium
Given the endothelium spans all areas of the human body, it must be able to cater to a wide variety of demands dependent on the tissue surrounding the vasculature. Morphological changes induced by the cellular microenvironment prompts a compensatory response from individual cells of the endothelium (Sumpio, 1991). To this extent, each cell reacts to its microenvironment in a unique way, largely independent to the influences of neighboring cells. However, since neighboring cells are exposed to roughly the same environmental conditions, inevitably they tend to exhibit similar gene expression profiles. Exemplary of the highly dynamic state of endothelial cells, individual segments of the vascular endothelium are able to
gauge shear stress and prompt the activation of transcription factors (such as Kruppel-like factor-2, KLF-2) to express proteins to ameliorate shear stress (Hierck, 2008). For instance, regions of heavy laminar stress, such as arterial vasculature where blood pressure is high, contain endothelial cells that heavily express VE-cadherin, a junctional endothelial-specific adhesion molecule that packs endothelial cells tightly and elongates cells to reduce shear stress (Kondapalli, Flozak, & Albuquerque, 2003). In contrast, regions of less strenuous shear stress, such as venous vasculature where blood pressure is lower, contain endothelial cells that heavily express P-selectin and vWF.
Although dynamic gene expression is by no means exclusive to endothelial cells, it is unique in its magnitude of plasticity; in making gene expression highly plastic, endothelial cells are able to display a wide range of phenotypic diversity specifically suited for their respective cellular microenvironments (Dejana, Hirschi, & Simmons, 2017). For instance, the degree of compactness between endothelial cells in capillaries has three levels (Betts, 2013) (Figure 3).
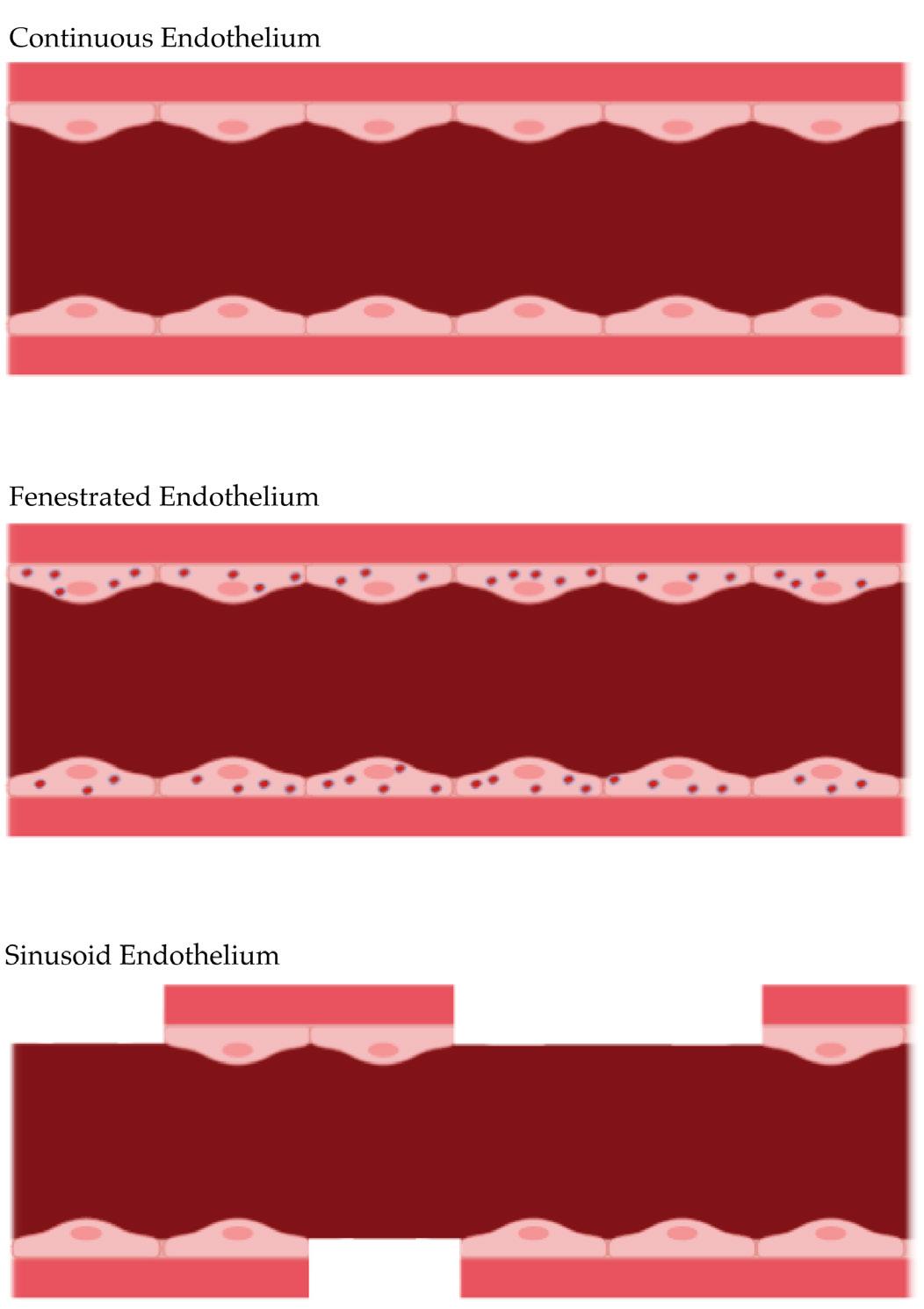
Figure 3. The three types of endothelia. The pink layer below the endothelial cells is the basement membrane.
Continuous endothelium is the most common type found in nearly all vasculature. As its name suggests, it is characterized by a continuous array of endothelial cells held together firmly by tight junctions. The continuous endothelium scarcely participates in adsorption and secretion due to its lowcell permeability. Water, ions, and other small molecules travel past the continuous endothelium through tiny gaps in the tight junctions between endothelial cells. The blood-brain barrier, a complex system of miniscule filtering capillaries that allows selective permeability for neuronal tissue, contains continuous capillaries supported by astrocytes and a thick basement membrane (Abbott, 2013). As a result, very few substances are able to cross the blood-brain barrier. Thus, the extreme selectivity of the blood-brain barrier poses numerous therapeutic challenges, as low blood-brain barrier permeability drastically limits the repertoire of drugs that can be used for neuronal diseases. Contrastingly, in areas where extremely high permeability is necessary, such as the kidney and small intestine, fenestrated endothelia exist (Betts, 2013). Fenestrated endothelia feature porous endothelial cells in addition to the gaps between tight junctions found in continuous endothelia. These 60-80 nm diameter fenestrations serve to increase the degree of permeability from the vasculature to the surrounding tissue by creating a route for molecular transport that does not involve intercellular travel. To aid in size-based sorting, fenestrations are supplemented by a network of radially aligned fibrils that allow small molecules and moderate amounts of protein to diffuse through the endothelial lining (Pavelka & Roth, 2010). In renal glomeruli though, specialized cells called podocytes extend foot processes through the endothelial fenestrations to confer solute specificity in the absence of fibrils (Pavenstadt, 2000). The negatively charged basement membrane is also able to selectively discourage the diffusion of negatively charged solutes such as albumin (Betts, 2013). Moreover, fenestrated endothelia are able to modulate their degree of permeability given their respective microenvironment by controlling the number of fenestrations present. The third type of endothelium, and the least common, is the sinusoid endothelium. Sinusoid endothelia are characterized by flattened rows of endothelial cells with large intercellular gaps. These large intercellular gaps are present in the basement membrane as well, allowing for the easy passage of large proteins or even cells. Compared to the other types of endothelia, sinusoid endothelia have the slowest blood flow, allowing for copious exchange of nutrients, waste, and other molecules. Sinusoid endothelia are extremely useful in organs that secrete very large biomolecules, such as the liver and spleen. In the bone marrow, sinusoid endothelia are crucial since new blood cells can only enter the blood supply through the massive intercellular gaps characteristic of sinusoid endothelia.
Developmental Origins of the Endothelium & Morphogen-induced Vascular Specialization
Given the huge amount of diversity among endothelial cells, it is important to understand how these differences arise. Following mature cell differentiation and vascular remodeling via Indian hedgehog (IHH), vascular specialization, that is the differentiation into arterial or venous endothelial cells, is mediated almost solely by a (VEGF) gradient present in the
mesoderm (Dyer & Patterson, 2010). However, it is important to note that although the presence of VEGF and its receptor, VEGFR2, is correlated with arterial cell fate, VEGF signaling may increase endothelial cell survival and propagation but not directly influence differentiation (Atkins, Jain, & Hamik, 2011). Although VEGF-VEGFR1 binding has weaker kinase activity than VEGF-VEGFR2 binding, VEGF-VEGFR1 binding is crucial to maintaining proper vascular morphology by acting as a VEGF sink, mitigating excessive proliferation caused by VEGF-VEGFR2 binding. Acting upstream of VEGF, Sonic hedgehog (SHH) binds to the Smoothened receptor to further induce arterial cell fate. In addition to VEGF activity, vascular specialization is also mediated by ephrinB2 and its receptor tyrosine kinase, EphB4, during vascular plexus remodeling. Interestingly, ephrinB2 secretion is largely exclusive to the arterial endothelium while EphB4 secretion is largely exclusive to the venous endothelium. Thus, this complementary relationship suggests a genetic influence on vascular specialization prior to systemic blood flow. Furthermore, VEGF binding to neuropilin-1 (Nrp-1) previous to systemic blood flow has been shown to trigger Notch signaling, promoting ephrinB2 and subsequently the VEGF-induced arterial phenotype (Dyer & Patterson, 2010). Venous cell differentiation is mediated by suppression of the Notch signaling pathway and arterial identity via chicken ovalbumin upstream-promotor-transcription factor II (COUP-TFII). Ablation of COUP-TFII reverts venous endothelial cells to the arterial phenotype and restores Notch signaling, suggesting COUP-TFII suppresses the default arterial phenotype under venous differentiation (Atkins, Jain, & Hamik, 2011).
From arterial and venous specialization, venous endothelial cells undergo further differentiation to create lymphatic endothelial cells. The lymphatic system serves as the migratory channel by which immune cells carry out their duties and surveil the body. Thus, lymphatic endothelial cells require a distinctive set of differentiation factors separate from those of the arterial and venous endothelia. During development, a subset of cardinal vein cells begins expressing prospero homeobox transcription factor 1 (Prox1) (Atkins, Jain, & Hamik, 2011). Eventually, as the subset of Prox1-positive cells grows in number, a balloon cluster known as the lymph sac arises. The lymph sac then thins and branches to form lymphatic vessels. Formation of Prox1-positive clusters solely at the anterioposterior axis of the cardinal vein suggests that Prox1 expression is closely regulated in venous endothelial cells. SRY-family transcription factor Sox18 and COUP-TFII co-expression has been shown to be necessary and sufficient for Prox1 induction and the subsequent lymphatic endothelial cell phenotype (Atkins, Jain, & Hamik, 2011).
In addition to the astonishing diversity of endothelial cell phenotypes, endothelial cells of the same phenotype also share genotypic differences. For instance, when vWF expression for heart, lung, liver and kidney endothelia was measured through immunofluorescence, vWF expression varied drastically across various organs despite being of the same endothelial type (i.e. continuous, fenestrated, sinusoid) (Marcu et al., 2018). Furthermore, when whole tissue from these organs were stained against VE-cadherin and CD45 and subject to flow cytometry, distinct expression profile clusters were identified among organ-specific tissue; this gene expression heterogeneity was then confirmed once again through RNAseq analysis, revealing unique gene expression profiles for organ-specific endothelial cells.
It is no surprise that organ-specific endothelial cells have distinct gene expression profiles warranted that the endothelium fulfills different functions given the organin which it resides. Gene expression for any cell type, including endothelial cells, lies on a spectrum upon which the most typical ‘pool’ of biologically stable cells is designated as a cell type. However, although they are not given distinction as individual cell types, smaller biologically stable ‘pools’ of cells exist among cell types that can be accessed by noise-mediated changes in DNA methylation (Yuan et al., 2016). Furthermore, using Cre/loxP cell fate mapping, these stochastic switches in gene expression were revealed to be more common in organ tissues requiring more dynamic gene expression (such as the lung and heart) while these switches were shown to be less common in organ tissues requiring static gene expression (such as the liver and kidney) mediated via biologically unstable intermediary states the cell must cross to revert to an alternative gene expression profile. Taken together, these studies suggest a wide range of genotypic diversity among organ-specific endothelial cells as well as endothelial cells arising from the same vascular bed.
Conclusion
Given the multifaceted role of the endothelium in various disease pathologies, understanding endothelial heterogeneity will help confer high levels of specificity when designing therapeutics. Since endothelial cells exhibit heterogeneity at the cellular microenvironment level, they hold promise for high specificity early detection and effective treatment of disease. Further research into mosaic heterogeneity of endothelial cells subject to identical microenvironments will provide great insights into the role of stochastic phenotype switching in disease predispositions such as variable metastatic potential among cells of a tumor. In addition, investigating whether these mosaic gene expression profiles fulfill a higher homeostatic role in their respective global populations will be an interesting line of inquiry. Considering the crucial role of the endothelium in maintaining hemostasis and overall body homeostasis, further investigation into its varied functions across tissue types will provide more insight into how the endothelium works in unison with the kidney to regulate circulating solute levels in the blood. This opens doors for new therapeutic development in vascular disorders, such as hypertension, where new standards of care aside from Losartan are crucially needed. By further elucidating all the molecular effectors responsible for the coagulation response, researchers can engineer better hemostatic agents for use in the clinic and the field. Understanding the dynamism of the endothelium will reveal fundamental new ways to combat a plethora of diseases—from atherosclerosis to cancer.
Acknowledgements
I’d like to thank my former Principal Investigator, Dr. Vivek Bhalla MD, FASN, FAHA, and the Bhalla Lab at the Stanford University School of Medicine, where my previous research on diabetic kidney disease and Esm-1 inspired my interest in endothelial cell and vascular biology.
References
Abbott, N. J. (2013). Blood–brain barrier structure and function and the challenges for CNS drug delivery. Journal of Inherited Metabolic Disease, 36(3), 437–449. doi: 10.1007/ s10545-013-9608-0 Atkins, G. B., Jain, M. K., & Hamik, A. (2011). Endothelial
Differentiation. Arteriosclerosis, Thrombosis, and Vascular
Biology, 31(7), 1476–1484. doi: 10.1161/atvbaha.111.228999 Ben-Baruch, A. (2009). Site-specific metastasis formation
Cell Adhesion & Migration, 3(4), 328–333. doi: 10.4161/ cam.3.4.9211 Betts, J. G. (2013). Anatomy & physiology. Houston, TX:
OpenStax College, Rice University. Bhugwandass, C., Pijnenborg, J., Pijlman, B., & Ezendam, N. (2016). Effect of chemotherapy on health-related quality of life among early-stage ovarian cancer survivors: a study from the population-based PROFILES registry. Current
Oncology, 23(6), 556. doi: 10.3747/co.23.3243 Dejana, E., Hirschi, K. K., & Simons, M. (2017). The molecular basis of endothelial cell plasticity. Nature Communications, 8(1). doi: 10.1038/ncomms14361 Dyer, L., & Patterson, C. (2010). Development of the
Endothelium: An Emphasis on Heterogeneity. Seminars in Thrombosis and Hemostasis, 36(03), 227–235. doi: 10.1055/ s-0030-1253446 García-García, P. M. (2014). Inflammation in diabetic kidney disease. World Journal of Diabetes, 5(4), 431. doi: 10.4239/ wjd.v5.i4.431 Hida, K., Maishi, N., Annan, D., & Hida, Y. (2018). Contribution of Tumor Endothelial Cells in Cancer Progression. International Journal of Molecular Sciences, 19(5), 1272. doi: 10.3390/ijms19051272 Hierck, B. P., Heiden, K. V. D., Alkemade, F. E., Pas, S. V. D.,
Thienen, J. V. V., Groenendijk, B. C., ... Poelmann, R. E. (2008). Primary cilia sensitize endothelial cells for fluid shear stress. Developmental Dynamics, 237(3), 725–735. doi: 10.1002/dvdy.21472 Huber, D., Cramer, E. M., Kaufmann, J. E., Meda, P., Massé Jean
Marc, Kruithof, E. K. O., & Vischer, U. M. (2002). Tissuetype plasminogen activator (t-PA) is stored in WeibelPalade bodies in human endothelial cells both in vitro and in vivo. Blood, 99(10), 3637–3645. doi: 10.1182/blood. v99.10.3637 Jahroudi, N., & Greenberger, J. S. (1995). The role of endothelial cells in tumor invasion and metastasis. Journal of Neuro
Oncology, 23(2), 99–108. doi: 10.1007/bf01053415 Jin, X., & Mu, P. (2015). Targeting Breast Cancer Metastasis.
Breast Cancer: Basic and Clinical Research, 9s1. doi: 10.4137/ bcbcr.s25460 Jung, U., Norman, K. E., Scharffetter-Kochanek, K., Beaudet,
A. L., & Ley, K. (1998). Transit time of leukocytes rolling through venules controls cytokine-induced inflammatory cell recruitment in vivo. Journal of Clinical Investigation, 102(8), 1526–1533. doi: 10.1172/jci119893 Kondapalli, J., Flozak, A. S., & Albuquerque, M. L. C. (2003).
Laminar Shear Stress Differentially Modulates Gene Expression of p120 Catenin, Kaiso Transcription Factor, and Vascular Endothelial Cadherin in Human Coronary Artery Endothelial Cells. Journal of Biological Chemistry, 279(12), 11417–11424. doi: 10.1074/jbc.m306057200 Marcu, R., Choi, Y. J., Xue, J., Fortin, C.L., Wang, Y., Nagao, R.
J., ... Zheng, Y. (2018). Human Organ-Specific Endothelial Cell Heterogeneity. IScience, 4, 20–35. doi: 10.1016/j. isci.2018.05.003 Muller, W. A. (2013). Getting Leukocytes to the Site of
Inflammation. Veterinary Pathology, 50(1),7–22. doi: 10.1177/0300985812469883 Muz, B., Puente, P. D. L., Azab, F., & Azab, A. K. (2015). The role of hypoxia in cancer progression, angiogenesis, metastasis, and resistance to therapy. Hypoxia, 83. doi: 10.2147/ hp.s93413 Pavelka M., Roth J. (2010) Fenestrated Capillary. In: Functional
Ultrastructure. Springer, Vienna Pavenstädt, H. (2000). Roles of the podocyte in glomerular function. American Journal of Physiology-Renal Physiology, 278(2). doi: 10.1152/ajprenal.2000.278.2.f173 Rajendran, P., Rengarajan, T., Thangavel, J., Nishigaki, Y.,
Sakthisekaran, D., Sethi, G., & Nishigaki, I. (2013). The Vascular Endothelium and Human Diseases. International Journal of Biological Sciences, 9(10), 1057–1069. doi: 10.7150/ijbs.7502 Sans, M., Panés, J., Ardite, E., Elizalde, J., Arce, Y., Elena, M., ... Piqué, J. M. (1999). VCAM-1 and ICAM-1 mediate leukocyte-endothelial cell adhesion in rat experimental colitis. Gastroenterology, 116(4), 874–883. doi: 10.1016/ s0016-5085(99)70070-3 Sumpio, B. E. (1991). Hemodynamic forces and the biology of the endothelium: signal transduction pathways in endothelial cells subjected to physical forces in vitro. Journal of Vascular Surgery, 13(5), 744–746. doi: 10.1016/0741- 5214(91)90372-2 Sun, H., Liu, J., Zheng, Y., Pan, Y., Zhang, K., & Chen, J. (2014). Distinct Chemokine Signaling Regulates Integrin Ligand Specificity to Dictate Tissue-Specific Lymphocyte Homing. Developmental Cell, 30(1), 61–70. doi: 10.1016/j. devcel.2014.05.002 Tsurupa, G., & Medved, L. (2001). Identification and
Characterization of Novel tPA-and Plasminogen-Binding Sites within Fibrin(ogen) αC-Domains†. Biochemistry, 40(3), 801–808. doi: 10.1021/bi001789t Turner, N., Nolasco, L., Tao, Z., Dong, J.-F., & Moake, J. (2006).
Human endothelial cells synthesize and release ADAMTS-13. Journal of Thrombosis and Haemostasis, 4(6), 1396–1404. doi: 10.1111/j.1538-7836.2006.01959.x Vaporciyan, A., Delisser, H., Yan, H., Mendiguren, Thom, Jones,
M., ... Albelda, S. (1993). Involvement of platelet-endothelial cell adhesion molecule-1 in neutrophil recruitment in vivo.
Science, 262(5139), 1580–1582. doi: 10.1126/ science.8248808 Versteeg, H. H., Heemskerk, J. W. M., Levi, M., & Reitsma, P.
H. (2013). New Fundamentals in Hemostasis. Physiological
Reviews, 93(1), 327–358. doi: 10.1152/physrev.00016.2011 Walsh, D. A. (1997). Regulation of Angiogenesis. Angiogenesis, 1(2), 139. doi: 10.1023/a:1018333906081 Weis, S. M., & Cheresh, D. A. (2011). Tumor angiogenesis: molecular pathways and therapeutic targets. Nature
Medicine, 17(11), 1359–1370. doi: 10.1038/nm.2537 Yau, J. W., Teoh, H., & Verma, S. (2015). Endothelial cell control of thrombosis. BMC Cardiovascular Disorders, 15(1). doi: 10.1186/s12872-015-0124-z Yuan, L., Chan, G. C., Beeler, D., Janes, L., Spokes, K. C.,
Dharaneeswaran, H., ... Aird, W. C. (2016). A role of stochastic phenotype switching in generating mosaic endothelial cell heterogeneity. Nature Communications, 7(1). doi: 10.1038/ncomms10160 Zhang, J., & Friedman, M. H. (2013). Adaptive response of vascular endothelial cells to an acute increase in shear stress frequency. American Journal of PhysiologyHeart and Circulatory Physiology, 305(6). doi: 10.1152/ ajpheart.00174.2013