Sierre Ternoey
Senior Thesis | 2024
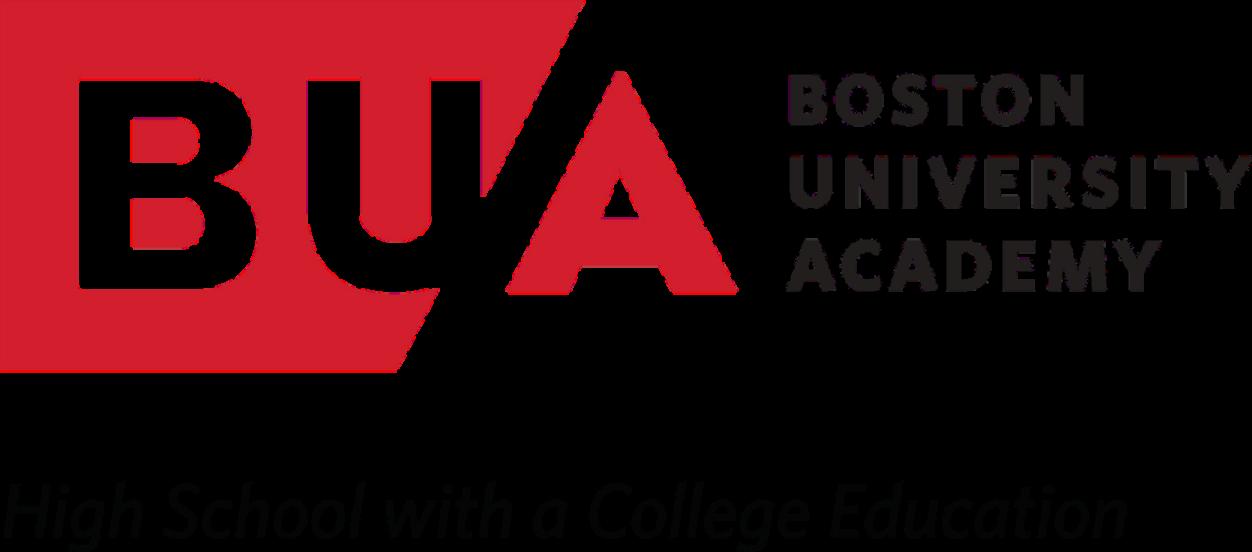
Abstract
Modeling Rhizobacterial Colonization of Roots in COMETS
Sierre Ternoey
The rhizosphere is the region of soil surrounding a plant’s roots that is affected by the plant’s chemical processes and growth. It is home to a great many species of bacteria that collaborate and compete with each other for resources to survive. Plant roots provide bacteria colonies with a physical home as well as nutrients in the form of exudates. In return, many bacteria greatly benefit their host plant. This thesis dives deeper into the colonization of Brachypodium distachyon, a model grass species, by Pseudomonas simiae, a keystone bacteria species. The relationship between these species is currently being studied as part of the Microbial Community Analysis and Functional Evaluation in Soils (mCAFEs) project at the Lawrence Berkeley National Lab using in vitro methods. In collaboration with the research team at Segrè lab and Berkeley lab, computational models and corresponding in vitro models of the colonization of B. distachyon by P. simiae were created to advance research on this relationship. Computation Of Microbial Ecosystems in Time and Space (COMETS), an opensource modeling platform developed by Segrè lab, was used to
construct the computational models. In vitro models currently in development at Berkeley lab will serve as comparisons to improve the computational models. Eventually, the COMETS models will accurately simulate the chemical exchanges and spatial structure of root colonization in the rhizosphere, potentially laying essential groundwork for cultivating a more complete understanding of the relationship between rhizobacteria and their host plants.
Introduction
1. Soil Microbiome
The rhizosphere is the region of soil around a plant’s roots, affected by secretions and processes of its roots. It is home to a plethora of microorganisms, including thousands of rhizobacteria and fungi species that colonize the root to use as a home and food source. The plant provides colonizing bacteria with a home as well as nutrition in the form of exudates, which are metabolites that the plant releases through its roots periodically. In exchange, many rhizobacteria species benefit their host plant in a number of ways. In this way, microorganisms and their host plants form compelling, mutually beneficial relationships. While rhizobacteria are very proactive in the bacteria-root relationship, the plant also controls many aspects, particularly the root colonization process (Chen 2024). This thesis deeper investigates the colonization of the plant species Brachypodium distachyon by the rhizobacteria species Pseudomonas simiae.
Brachypodium distachyon is a grass species that has grown popular in research as a model for more agriculturally relevant plants, such as rice, barley, and wheat. In this thesis, Brachypodium distachyon, or rather its roots, will act as a representation of the roots of these grass species. Brachypodium
distachyon first emerged as a model organism in the early 2000s, following a huge breakthrough in the world of plant research: the sequencing of the genomes of rice and Arabidopsis. Unfortunately, the methods used to sequence these species’ genomes could not be applied to larger genomes with copious amounts of repetitive DNA. This was when Brachypodium distachyon was presented as a solution. Its genome is compact, with little repetition, and could, therefore, easily be sequenced. Brachypodium distachyon was also found to share a lot of genetic similarities with related but more complex species. This meant B. distachyon could act as a good representation of these species in research going forward. Furthermore, obtaining an accurate B. distachyon genome laid the key groundwork for the construction of the more complex genomes of rice, wheat, barley, etc. (Hasterok et al. 2022). B. distachyon’s short generation time and extensive available germplasm makes it a favorable candidate for experiments. It has quickly become a central species in the world of plant research for the study of plant development and root morphology (Sasse et al. 2019). Additionally, in the context of this thesis, its steady growth rate and simple root architecture make it a great species for computational modeling.
Pseudomonas simiae is a keystone rhizobacteria species that colonizes roots and uses them as a nutrient source and physical home for the colony. It is a highly competitive bacteria species in the rhizosphere. P. simiae was first classified when scientists discovered it as the cause of death in a female monkey and her offspring (Vela et al. 2006). Since then, further studies of its genome have revealed compelling properties of the relationship between P. simiae and the plants it colonizes. In 1988, a group of scientists at the Willie Commelin Scholten Phytopathological Laboratory isolated a strain of P. simiae that had colonized the roots of their wheat plant (Triticum sativum) and named it WCS417. The researchers discovered this strain of P. simiae while comparing the efficacy of different biological soil environments in suppressing take-all disease in wheat (C. M. J. Pieterse et al. 2020).
“Take-all” is a disease caused by the soil-borne fungus Gaeumannomyces graminis var. tritici. This disease is widely considered to be the most important root disease of wheat and is responsible for 5 to 20% of annual crop loss in the United Kingdom. Furthermore, these percentages will likely increase as climate change improves environmental conditions for fungal diseases. Primary infection occurs when the fungus enters a plant’s
roots, penetrating the root epidermis and invading the root cortex. Secondary infection follows as the disease is transmitted from one plant to another through root-to-root contact, spreading through the crop. Once the fungus has successfully entered the root, it destroys the vascular tissue, preventing the plant’s water and nutrient uptake in the soil. Currently, there are no optimal solutions to this problem, and only crop rotation, an unreliable control measure, is used in practice. Several fungicides have been developed and tested, each with their own pitfalls. At the moment, host-induced gene silencing (HIGS), the silencing of wheat genes that interact with the fungus, has been the most successful method for reducing infection (Palma-Guerrero et al. 2021). However, the disease remains a significant difficulty in farming.
A promising new weapon against take-all disease arrived with the discovery of WCS417. The bacteria strain stood out because of its success in suppressing take-all disease in its wheat plants while simultaneously increasing grain yield. Follow-up studies have been carried out to further investigate the effects of WCS417 on plant yield and take-all disease severity, which confirmed these properties. Inoculating seeds with P. simiae prior to sowing reduced take-all disease severity caused by the inoculation with G. graminis var. Tritici in the fields from 27% to
6% while significantly improving wheat yield. Additionally, WCS417 was shown to control other diseases, such as bacteria wilt from Ralstonia solanacearum and nematode Xiphinema index. WCS417-root signaling was shown to improve the plant’s defense efficiency overall, making its immune system stronger while also preventing it from activating unnecessary and costly defense mechanisms. Furthermore, WCS417 was shown to help with drought prevention and salt tolerance in peppermint plants, and improve water, iron, and nutrient uptake (C. M. J. Pieterse et al. 2020). However, research has shown that these benefits depend highly on a successful colonization of the rhizosphere by the P. simiae colony (Compant 2010).
WCS417 is particularly successful in colonizing plants mainly due to its ability to keep competing microorganisms in check and its adaptation to plants’ defense systems. P. simiae outcompetes other microbes that are attempting to colonize the root area by dominating the iron supply, using pyoverdine, a molecule released by microorganisms to capture iron from the rhizosphere. Additionally, a closer examination of its secondary metabolite biosynthetic gene clusters reveals WCS417 can release metabolites which affect other competing microbes, as well as suppress the defense mechanisms in the host plant intended to
prevent bacteria from colonizing it. This presents a tradeoff in the relationship: In exchange for many benefits, the plant’s control of its defense system is compromised (C. M. J. Pieterse et al. 2020). However, the relationship between P. simiae and its host plants can be regarded as symbiotic, as it reduces plant disease while increasing crop yield. Therefore, the prospect of fully understanding and enhancing it presents an attractive opportunity for improving agriculture.
Another important process in rhizobacterial root colonization is chemotaxis. Chemotaxis is the navigation by bacteria in response to a chemical gradient in their environment in order to optimize their metabolic activity. Bacteria move in the direction of an increasing gradient if the chemical is a chemoattractant, and in the direction of a decreasing gradient if the chemical is a chemorepellent. Chemoattractants are often food sources themselves, such as glucose, for bacteria to feed on. However, other molecules can also be chemoattractants if the bacteria associate them with a food source. In the context of the rhizosphere, the source of chemical gradients that allow rhizobacteria to locate the plant are the plant’s exudates. Exudation is when plants periodically release byproducts of photosynthesis through their roots. These exudates contain specific
chemoattractant signal molecules, which help bacteria like P. simiae to navigate towards the root using chemotaxis. A study investigating the role of chemotaxis in rhizosphere colonization found that major chemoattractants for Pseudomonas species also include amino acids, organic acids, polyamines, gammaaminobutyric acid, and inorganic phosphate. Pseudomonas fluorescens, a close relative of Pseudomonas simiae, even displayed chemotactic responses to Streptomyces spores, which is itself a bacteria species most commonly used in antibiotics (Feng et al. 2021).
Once bacteria are in close enough range of the root, they no longer require chemotaxis. However, chemotaxis is decidedly an essential component in the colonization process and has yet to be fully understood, in particular because it can be difficult to determine which exact molecules bacteria are responding to (Feng et al. 2021). Root colonization involves many complex biochemical and biophysical processes. This is where computational modeling becomes a valuable tool for furthering the research of rhizobacteria. In this thesis, Computation Of Microbial Ecosystems in Time and Space (COMETS) was used to create a computational model of a soil environment and a root cross section in the rhizosphere.
2. Computational Modeling with COMETS
Computation Of Microbial Ecosystems in Time and Space (COMETS) is an open-source modeling platform launched in 2021, dedicated to stoichiometric modeling of metabolism in microbial communities. Essentially, this program simulates the behavior of diverse bacteria communities over time in spatially and chemically complex environments using the bacteria species’ genome-scale stoichiometric models (Dukovski et al. 2021).
Genome-scale stoichiometric models consist of all the metabolic reactions encoded in an organism’s genome and are used to predict the organism’s behavior in different metabolic contexts (Passi 2021). COMETS has developed several genome-scale stoichiometric models of microorganisms, including various bacteria and even some fungi, such as yeast, and even a WCS417 P. simiae model, which is still in the testing stages of development. For the beginning of this research, the COMETS E. coli core model will be used as a placeholder in simulations. While Escherichia coli is not a rhizobacterium, The “e_coli_core” stoichiometric model consists of only the key reactions of the more complete E. coli stoichiometric model. These key reactions, like glycolysis, the tricarboxylic acid cycle and the pentose phosphate shunt, are well representative of other bacteria species, including
P. simiae. The nutrient requirements vary between E. coli and P. simiae, but can easily be adjusted. This thesis centers around relationship models and scenarios that require only that metabolites can be exchanged as food sources and that the attraction of the colony to the root can be modeled. Therefore, the E. coli model was used in place of P. simiae for the testing stages of the models. Once finalized, the P. simiae model will be implemented and the model will be translated to reflect the chemical profile corresponding to P. simiae metabolism.
Recent versions of COMETS utilize dynamic flux balance analysis (dFBA) to accurately predict the biomass growth, evolutionary dynamics, and enzyme activity of bacteria colonies based on their genome-scale stoichiometric models (Dukovski et al. 2021). Dynamic FBA is an extension of regular flux balance analysis, which is a method for modeling the metabolism of a cell in microbiology. Regular FBA predicts the changes to metabolite weight and proportion before and after various chemical reactions in a stoichiometric matrix. However, this method only accurately predicts the growth of cells for steady state conditions and constant uptake rates. Dynamic flux balance analysis allows for the modeling and optimization of a microbial community’s metabolism in the context of a complex environment that is
changing over time. While more accurate, dFBA is more costly and requires more information than regular FBA in order to construct a dynamic model of the community (Henson et al. 2014). Therefore, there is a tradeoff in using a more complex modeling tool for COMETS. As the grid size of a model is increased, the run-time of a simulation grows too rapidly, so COMETS simulations are currently restricted to 2-dimensional grids.
The Segrè lab has developed a soil model that accurately emulates soil conditions. Users can adjust soil to contain nutrients, rocks, and metabolite levels according to their specific experiments. This paper describes how COMETS was used to create a model of a grainy soil environment and a model of a root cross section in order to examine two levels of the root colonization process more closely. Both COMETS models have a corresponding in vitro model that simulates the same scenario in a wet lab experiment as part of the Microbial Community Analysis and Functional Evaluation in Soils (m-CAFEs) project at the Lawrence Berkeley lab. The m-CAFEs project is a multiinstitutional research project with the goal of developing “complementary approaches to precisely edit microbiomes with foundational tools and develop the molecular ecosystems biologybased understanding required to predict, manipulate, and design
rhizosphere microbial communities impacting DOE relevant activities” (m-CAFEs 2020). The in vitro counterparts will serve as references as the models continue to be tested and improved.
Methods
In COMETS, Python notebook scripts are used as an interface for users to engineer the spatial and chemical environment of their microbial simulation. There are several components to a COMETS simulation script. First, the dimensions of the grid in which the simulation will take place are defined. Then, the parameters for the metabolite environment are set. Users decide the quantity and location for any and all metabolites relevant to the simulation, such as nitrogen, oxygen, ammonia, glucose, etc. For a well mixed, constant environment, the metabolites can be set to be abundantly available throughout the grid. However, metabolites can also be set to be available only at certain locations, they can be set to diffuse through the environment at specific rates, and they can be set so their abundance varies periodically. Users can also choose to place barriers throughout the simulation, to create obstacles that might occur in nature, like rocks, branches, or in the case of this thesis,
roots and soil grains. And lastly, a microorganism is added to the model in the form of a stoichiometric model.
As previously mentioned, chemotaxis plays an important role in the root colonization process. A chemotaxis module has been developed for COMETS, based on growth and diffusivity rates recorded through extensive in vitro experimentation using chemoattractant filled agar plates. Users set a desired metabolite to be a chemoattractant or a chemorepellent in the model environment by calling the “set_chemotaxis” function and inputting the metabolite name, chemotaxis coefficient, and nutrient parameters. The metabolite name and nutrient parameters work the same way here as in the general environment. The chemotaxis coefficient indicates the strength of the chemical gradient in bacterial motility. Although setting up chemotaxis does not require very many inputs from the user, the algorithm for the chemotaxis module does take into account the many additional factors that can play a role in chemotaxis and the movement of bacteria, including the density of the bacteria population, density of the chemoattractant or chemorepellent, bacterial sensitivity, diffusion of the attractant, etc.
Another important process that takes place during root colonization is near-wall hindered diffusivity. Root colonization
dynamics vary depending on the species, age, and even the location on the plant’s root system. At younger areas of the root, exudates contain more mucilage in order to lubricate the growing roots, whereas closer to the seed, the roots are less viscous (McNear Jr. 2013). Furthermore, the toughness and composition of the root surface itself varies at different locations of the root, as it is covered in ridges and hairs. Researchers at m-CAFEs hypothesize that the rhizobacteria collect primarily in these root crevices due to near-wall hindered diffusion. Near-wall hindered diffusion is the slowing of Brownian motion due to an increase in the viscosity of a substance as it interacts with a wall. Essentially, the diffusion of bacteria through liquid is hindered near solid walls. In the context of the rhizosphere, bacteria diffusing through a layer of secreted metabolites are slowed as they get closer to the root surface and struggle to access the root cell wall. Berkeley researchers hypothesize that as a result, bacteria attempting to navigate towards the wall accumulate in the small open regions within crevices, where the viscosity remains lower. Near-wall hindered diffusion is being implemented in COMETS based on a mathematical model published by researchers at Oxford University (Eloul & Compton 2016), and is expected to be available in May of 2024.
At the moment, near-wall hindered diffusion and chemotaxis modules are still in the testing stages. For the time being, two models of the rhizosphere have been developed without chemotaxis or near-wall hindered diffusion. However, these modules are crucial in answering the questions at hand building a simulation of the root colonization process. Once the version of COMETS that implements them is released and the models are in appropriate conditions to be transitioned to the updated COMETS version, these processes will be implemented in the simulations.
1. Soil Environment
The first relationship model is a soil environment with barriers the bacteria have to get around in order to reach a food source. The barriers represent the grains of sand in soil that the bacteria have to navigate in real life to access the root. In soil with coarser sand, there are fewer grains, but their size is greater, and thus present larger obstacles to navigate around. Finer soil has smaller obstacles, but far more which are more densely packed together. To replicate soil grains, a Voronoi diagram was used in this model. A Voronoi Diagram can be defined as the partitioning of a Euclidean plane into regions close to each of a given set of objects, such as a set of points distributed throughout the plane.
This can also occur in 3-dimensional space. In the rhizosphere, the tiny grains in the soil are packed together, approximately forming a Voronoi diagram (Zhang 2021). The objective of this model was to compare the navigation of rhizobacteria through grainy soil environments of various densities in COMETS. To imitate the grainy soil environment, a Voronoi diagram would be constructed, and the partitioned regions would be blocked off by placing barriers in the grid, leaving open a path that bacteria navigate through. Several models would be created, increasing the number of partitioning points and decreasing the width of the path in the Voronoi diagram to vary density. The main goal of this model is to compare the diffusion and mobility of the bacteria in different densities of soil to determine whether using coarser or finer soil plays a significant role in the root colonizing process.
To construct the computational model, a 61 cell by 61 cell grid was created in COMETS to represent .37 cm2 of soil space. Global parameters were set to create a growth-enabling environment consistent with that of soil, with unlimited oxygen, ammonia, phosphate, water, and protons. The standard glucose concentration for an E. coli colony feed is approximately 0.5 grams/liter, equivalent to 2.8e-8 mmol/cell in a COMETS model. However, because the majority of the grid is blocked off by
barriers imitating grains, additional glucose is needed in order to enable colony growth. In order to keep the average glucose concentration through the whole grid at 2.8e-8 mmol/cell, the global glucose was set to be 2.9e-5 mmol/cell.
To create the sand grains for the bacteria to navigate around, barriers were placed to block out the space where the grains would lay. To accurately depict this, a Voronoi diagram was constructed by randomly generating 10 points around the grid as the region partitioning objects. Once partitioned, a path was carved out by isolating and removing the cells along the borders of the partitions. Barriers were placed at every cell that was not a part of the path, while leaving an additional strip of width three cells wide at the top and bottom of the grid to make space for the initial population and glucose food source. A Voronoi diagram of 10 points was created, with a path of width 5 pixels, as well as a diagram of 20 points, with a path of width 3 pixels, to emulate basic coarse and fine grainy soil environments that cover an approximately equivalent proportion of the grid. Finally, the initial population of bacteria was placed. 1.2 e-4 grams of E. coli was distributed evenly along one edge of the grid. 6e-2 mmol of glucose was evenly distributed along the opposite edge of the grid as the food source to attract the bacteria colony. A diagram
depicting the spatial set-up of the grainy soil model is provided in does not implement chemotaxis. Images of the biomass distribution through the grid were recorded after 0 cycles, 100 cycles, 200 cycles, 300 cycles, and 400 cycles. With a time step set to 0.1 hours, these intervals are equivalent to 0, 10, 20, 30, and 40 hours of real time, respectively. COMETS models with finer grains, i.e. with more points used to generate the Voronoi diagram, are pending and expected to be run in June of 2024. The m-CAFEs
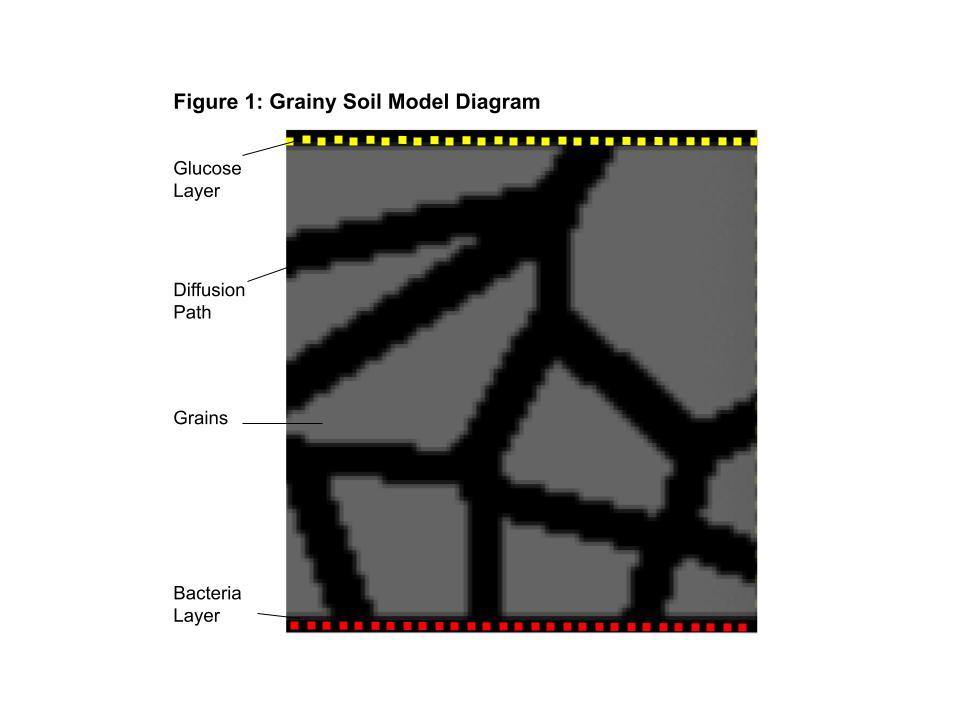
team has developed an in vitro simulation of the grain obstacle model by 3d printing devices that are essentially identical to the COMETS model. These physical models consist of a 2.5 cm by 2.5 cm chamber with polymer barriers 3D printed in the form of a Voronoi diagram, leaving a path open. The chambers will be shipped to the Segrè lab, where P. simiae will be placed on one edge, and the food source on the opposite side, as was done in the computational model. Results from the in vitro experiments can be expected in June, 2024.
2. Root Cross Section
The second relationship model is a cross sectional view of the root surface. The main interest of this model is the motion and accumulation of bacteria in the context of the root environment’s geometric layout. The root cross section will be represented with a barrier through the middle of the COMETS model grid in the form of a sine wave to emulate the ridges visible in the photographs.
Metabolites that the B. distachyon root would release as part of exudation will then be programmed to appear just above the barrier, the way they would be released through a real root using the set_specific_metabolite_at_location() function. The function allows users to specify a location in the grid, the desired
metabolite, and amount of the metabolite to be placed at the location. COMETS also has functions that allow users to set metabolites to be replenished periodically if they are uptaken by bacteria. A study on root exudation dynamics observed a diurnal signature in the metabolic profile of model plant species’ root exudates, including B. distachyon. That is, the amount and type of compound found in the collected exudates varied at different timepoints over the course of the day, as well as at different stages in the plant’s development (McLaughlin et al. 2023). Therefore, COMETS’ time-specific functions will be important to take advantage of in the future as the simulation of exudation is improved.
It should be noted that the endosphere, which is the region inside of the root, can also be colonized by bacteria. However, the microbial community of the endosphere differs from the microbial community of the rhizosphere or that of bulk soil. This is because in order to reach the endosphere, bacteria must penetrate the root. While some bacteria, including P. simiae, do travel across root domains during their life cycle, motion between the two is not fluid. Therefore, the nutrient composition and bacterial population look different on the inside compared with the outside of the root (Liu 2023). Therefore, in order to focus on the rhizosphere rather
than the endosphere for this model, the root border was programmed to be an impenetrable barrier.
To construct the computational model, a 61 cell by 61 cell grid was created in COMETS to represent .37 cm2 of soil space. Global parameters were set to create a bacteria friendly environment consistent with soil, with unlimited oxygen, ammonia, phosphate, water, and protons. The global glucose was set to be 2e-8 mmol per cell, which is the standard concentration of glucose per cell in COMETS models for E. coli colony growth. This is equivalent to around 2e-4 mmol per cm2 and within the standard range of 4-8 mg C g−1 that promotes colony growth and respiration (Reischke et al. 2014). Barriers were then placed to cover the entire area that the root cross section lays. With a space step of .05 in the COMETS model, 1 cell is equivalent to .05 centimeters. Barriers were placed in the shape of a sine wave with a wavelength of 20 cells, or 1 centimeter, and an amplitude of 5 cells, or .25 centimeters. 5e-5 mmol of glucose was then evenly distributed in a layer of cells running along the entire sine wave using the set_specific_metabolite_at_location() function to act as the chemoattractant exudates. More specific metabolites will be programmed in the future. Finally, the initial population of bacteria was placed. 4.4 e-6 grams of E. coli was then distributed evenly
around the open space remaining in the grid. A diagram depicting the spatial set-up of the root cross section model is provided in Figure 2.
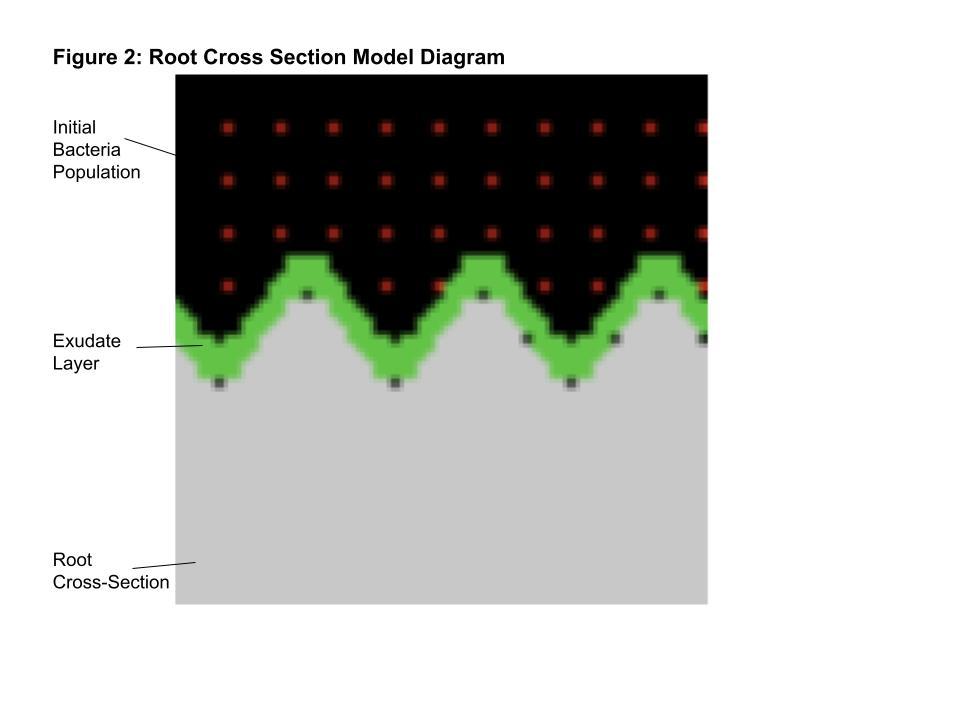
version of COMETS which does not implement chemotaxis. Images of the biomass distribution through the grid were recorded after 0 cycles, 100 cycles, 200 cycles, 300 cycles, and 400 cycles. With a time step set to .1 hours, this is equivalent to 0, 10, 20, 30, and 40 hours of real time, respectively. The models will be run in COMETS with chemotaxis once available and results are expected by the end of May, 2024. A physical counterpart to the
computational model is in development at the Lawrence Berkeley National Laboratory as part of the lab’s m-CAFEs project, using agar plates to imitate the root surface, covered in a growth media to imitate the exudates.
Results
1. Model 1: Grainy Soil Environment
The grainy soil simulation runs, which were done without the presence of chemotaxis or near-wall hindered diffusion, showed expected growth and diffusion. The simulation plots of Model A, the coarser grain model, and Model B, the finer grain model, are provided in Figure 3 on the following page and show the distribution of the bacteria population in both models for the 5 time steps. In 40 hours of real time, which was 400 cycles with a time-step of 0.1 in the COMETS model, the colony biomass increased from 1.21e-4 grams to 18.63e-4 grams in Model A and from 1.21e-4 grams to 14.77e-4 in Model B. The plots show that the population remained most concentrated at the initial location, with subtle bright areas at the intersections between paths. There is more open space in these intersections, and thus a higher concentration of glucose available, which likely explains the higher population concentration. In both models, the colony
diffused through the grains and reached the midline of the grid at around cycle 300, meaning the colony covered approximately 3 centimeters in 30 hours. These findings are consistent with what is known about E. coli behavior and diffusion, and do not indicate a difference in population diffusion between the two layouts.
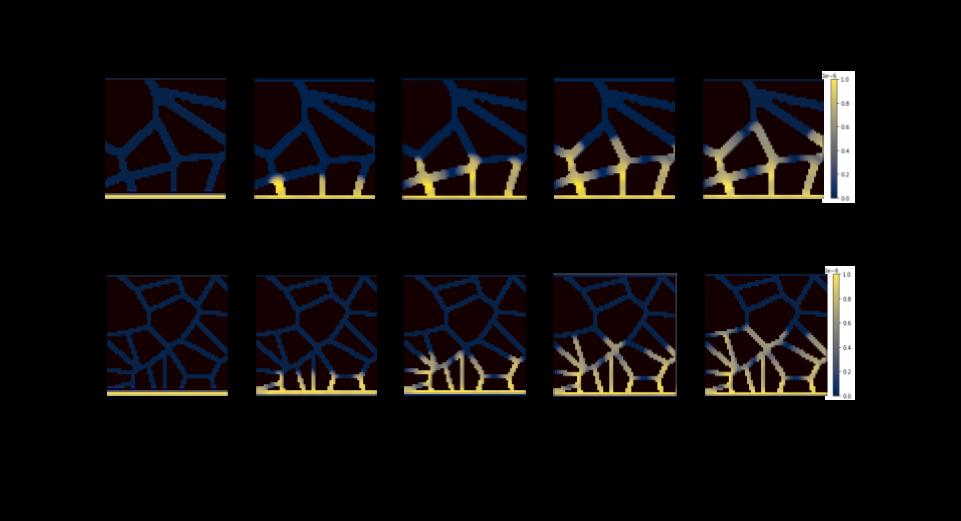
2. Model 2: Root Cross Section
The COMETS runs showed the expected growth and diffusion of the E. coli population in the root cross section model. The simulation plots of the root cross section model are provided in Figure 4 on the following page and show the growth and distribution of the bacteria population for 5 time steps. The total biomass grew from 1.11e-4 grams to 12.38e-4 grams in
approximately 40 hours of real time. In the simulation plots, a clearly discernible gradient in population concentration emerged, with the layer along the root barrier being much more heavily populated than the areas on the edge of the grid. This is consistent with the more abundantly available nutrition around the barrier where the exudates were placed. Slight accumulation of E. coli in the crevices of the sine wave is visible starting at around cycle 200.
Experiments with real 3D printed agar plates have not been conducted yet. This can be expected after the COMETS cross section model has been fully developed with chemotaxis and hindered diffusion.
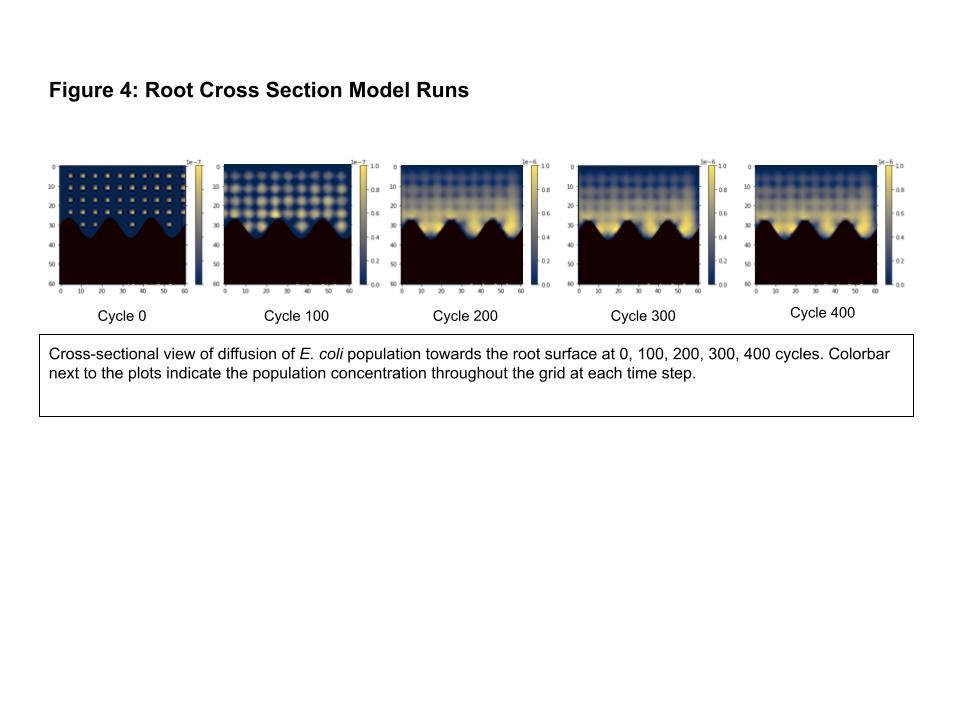
Discussion
1. Model 1: Grainy Soil Environment
Comparing the two models that have been developed and tested, it can not be concluded that coarser soil helps or limits colony movement. Naturally, drawing conclusions based on two samples was not expected. As mentioned, more models with lattices of density 10 and 20, as well as higher densities of 40 or 80 points, will be run once chemotaxis is enabled. Simulation runs will then be quantifiably compared to ensure an accurate assessment of results. For each density treatment, at each time step (0, 100, 200, 300, 400 cycles) the diffusion of biomass will be integrated across the vertical axis (the direction of colony movement). COMETS records the biomass at every cell in the grid, so the biomass in the cells at every level from x = 0 to x = 61 will be summed together, plotting biomass as a function of x. The plot of this integral is expected to approximately be a sigmoidal curve that evens out with each time step as the biomass diffuses through the grid. On each plot, the location of the biomass halfpoint will be recorded, which is the point on the x-axis that divides the total biomass in half, where the area under the curve on the right equals the area under the curve on the left of the half-point. This will be done for each graph at the 5 time steps (0, 100, 200,
300, 400 cycles). This data will allow for an objective comparison of the densities by examining how the half point moves over time for different grainy soil densities.
The behavior of real rhizobacterial colonies in the 3-D printed models will be assessed similarly once in vitro experiments are carried out. The colony distribution through the grid will be analyzed using fluorescent imaging, and the diffusion will be assessed the same way as the COMETS model. Unfortunately, the analysis will be based on comparison between colony images, rather than comprehensive plots of bacterial movement. Therefore, analysis of the real-life results will not be as deeply quantifiable as what COMETS allows. However, the combination of real life results and in depth measurements will hopefully form a helpful, accurate picture that informs understanding of bacterial movement through grainy soil.
2. Model 2: Root Cross Section
The growth and gradient of the bacterial population in the cross-sectional model is consistent with what was expected. The additional glucose along the root barrier increased the population growth along the root surface, which is why the gradient in population density emerges in the plots. Slight collection of E. coli
in the sine wave crevices can be attributed to the combination of increased glucose availability and barriers surrounding the area preventing the diffusion of bacteria through the grid. However, this accumulation is unrelated to the hindered diffusivity caused specifically by liquid-barrier interactions. The models will be run again in COMETS once chemotaxis and barrier-liquid hindered diffusion are finished. It is expected that more colony movement towards the barrier, due to chemotaxis, as well as increased bacterial accumulation in the crevices, due to near-wall hindered diffusion, will be observed once these processes are implemented. This would affirm the hypothesis of m-CAFEs researchers that viscosity and hindered diffusion strongly affect the root colonization process.
Conclusion
The immediate next steps for both models are to run the simulations in the future version of COMETS once it has been finalized by researchers at Segrè lab. Once the testing of stoichiometric models of P. simiae is completed, these models will replace the E. coli core model in the simulation. This thesis focused on research conducted on Brachypodium distachyon, and researchers at Lawrence Berkeley lab have put together a metabolic profile of its exudates (McLaughlin et al., 2023). So next, the glucose layer will be replaced with accurate metabolites according to the exudate profiles of the model plants. Furthermore, just recently in February of 2024, a study on exudate function in root colonization was published by researchers at the Experimental Center of Forestry in North China which specifically looked into Arabidopsis thaliana exudates relevant to WCS417 colonization (Chen et al. 2024). Undoubtedly, there are many opportunities to branch out to other model plants as well as other relevant rhizobacteria species. As root exudation and other relevant processes become more accurately programmed, the bacteria models get fitted more accurately, and more bacteria get incorporated into the model, the models will become more and more realistic.
For Segrè lab, one broader future goal is to expand COMETS to 3-dimensional models. Right now, simulations are limited to 2-dimensional structures. This does allow spatial modeling, but being able to create 3-dimensional geometries presents opportunities for even more intricate geometric models of root systems in space. However, there are many more possibilities for expanding 2-dimensional research. For the cross-sectional model, a potential path forward may be to structure the root surface as a plant cell rather than an impenetrable barrier so that the cycling of bacteria through the root can be simulated. Another model that is currently in development is a layout of an entire root system in an EcoFAB, which is a device designed by researchers at m-CAFEs for observing and experimenting with root growth in a controlled microbial environment (EcoFAB n. d.). Fabricated ecosystems, or EcoFABs, consist of a transparent autoclavable chamber for observing the fabricated ecosystem’s dynamics, in particular the colonization and growth of a target host plant. Additionally, the growth media can be precisely adjusted by users, including but not limited to nutrients, substrates, and microbes. By using the EcoFAB water-based media adjusted to emulate the conditions of soil, researchers can closely observe root growth and morphology without opaque substrates like soil or sand obstructing
the view of the root. The m-CAFEs team has used the devices to specifically research Brachypodium distachyon exudation in different growth environments, which is serving as an in vitro reference for the COMETS model (Sasse 2019). With a model of a full root system, a next step may be to try to simulate the growth of the root over time, extending where the root runs and how the barriers and exudates change correspondingly.
Ultimately, this thesis aims to lay groundwork for the computational exploration of the rhizosphere and future of rootbacteria research initiatives. The goal is that the COMETS models will eventually be developed to the point where they can provide insights on the dynamics of the rhizosphere. This would significantly advance m-CAFEs’ goal to engineer a synthetic community of rhizobacteria using CRISPR that enhances the growth of Brachypodium distachyon plants (m-CAFEs 2020).
References
L. Chen, Y. Liu, The Function of Root Exudates in the Root Colonization by Beneficial Soil Rhizobacteria. Biology (Basel). 2024 Feb 2;13(2):95. doi: 10.3390/biology13020095. PMID: 38392313; PMCID: PMC10886372.
S. Compant, C. Clément, & A. Sessitsch, Plant growth-promoting bacteria in the rhizo- and endosphere of plants: Their role, colonization, mechanisms involved and prospects for utilization. Soil Biology and Biochemistry, 42(5), 669–678 (2010). https://doi.org/10.1016/j.soilbio.2009.11.024
I. Dukovski et al., A metabolic modeling platform for the computation of microbial ecosystems in time and space (comets). Nature News (2021), (available at https://www.nature.com/articles/s41596-021-005933#Sec62).
EcoFAB – Linking genome biology to ecosystem processes through model laboratory ecosystems. (n.d.). https://ecofab.org/
S. Eloul, & R. G. Compton. General model of hindered diffusion. The Journal of Physical Chemistry Letters, 7(21), 4317–4321 (2016).
H. Feng et al., Chemotaxis of beneficial rhizobacteria to root exudates: The first step towards Root–microbe rhizosphere interactions. International Journal of Molecular Sciences. 22, 6655 (2021).
R. Hasterok et al., Brachypodium: 20 years as a grass biology model system; the way forward? Trends in Plant Science. 27, 1002–1016 (2022).
M. A. Henson, T. J. Hanly, Dynamic Flux Balance Analysis for synthetic microbial communities. IET Systems Biology. 8, 214–229 (2014).
Lawrence Berkeley National Laboratory. (24 August, 2020). mCAFEs. https://mcafes.lbl.gov/
S. McLaughlin, K. Zhalnina, S. Kosina et al. The core metabolome and root exudation dynamics of three phylogenetically distinct plant species. Nat Commun 14, 1649 (2023). https://doi.org/10.1038/s41467-023-37164-x
D. H. McNear Jr., The Rhizosphere - Roots, Soil and Everything In Between. Nature Education Knowledge 4(3):1 (2013).
J. Palma-Guerrero et al., Take-all disease: New insights into an important wheat root pathogen. Trends in Plant Science. 26, 836–848 (2021).
A. Passi, J. D. Tibocha-Bonilla, M. Kumar, D. Tec-Campos, K. Zengler, & C. Zuniga, Genome-scale metabolic modeling enables in-depth understanding of Big Data. Metabolites.https://www.ncbi.nlm.nih.gov/pmc/articles/PM C8778254/ (2021).
C. M. J. Pieterse et al., Pseudomonas simiae WCS417: Star track of a model beneficial Rhizobacterium - plant and soil. SpringerLink (2020), (available at https://link.springer.com/article/10.1007/s11104-02004786-9).
S. Reischke, J. Rousk, & E. Bååth, The effects of glucose loading rates on bacterial and fungal growth in soil. Soil Biology and Biochemistry, 70, 88–95. https://doi.org/10.1016/j.soilbio.2013.12.011 (2014).
J. Sasse et al., Multilab EcoFAB study shows highly reproducible physiology and depletion of soil metabolites by a model grass. New Phytologist. 222, 1149–1160 (2019).
A. I. Vela, M. C. Gutiérrez, E. Falsen, E. Rollán, I. Simarro, P. García, L. Domínguez, A. Ventosa, & J. F. FernándezGarayzábal(2006). Pseudomonas simiae sp. nov., isolated from clinical specimens from monkeys (Callithrix geoffroyi). International Journal of Systematic and
Evolutionary Microbiology, 56(11), 2671–2676. https://doi.org/10.1099/ijs.0.64378-0
C. Zhang, S. Zhao, J. Zhao, & X. Zhou, X. (2021). Threedimensional Voronoi analysis of realistic grain packing: An XCT assisted set Voronoi tessellation framework. Powder Technology, 379, 251–264. https://doi.org/10.1016/j.powtec.2020.10.054
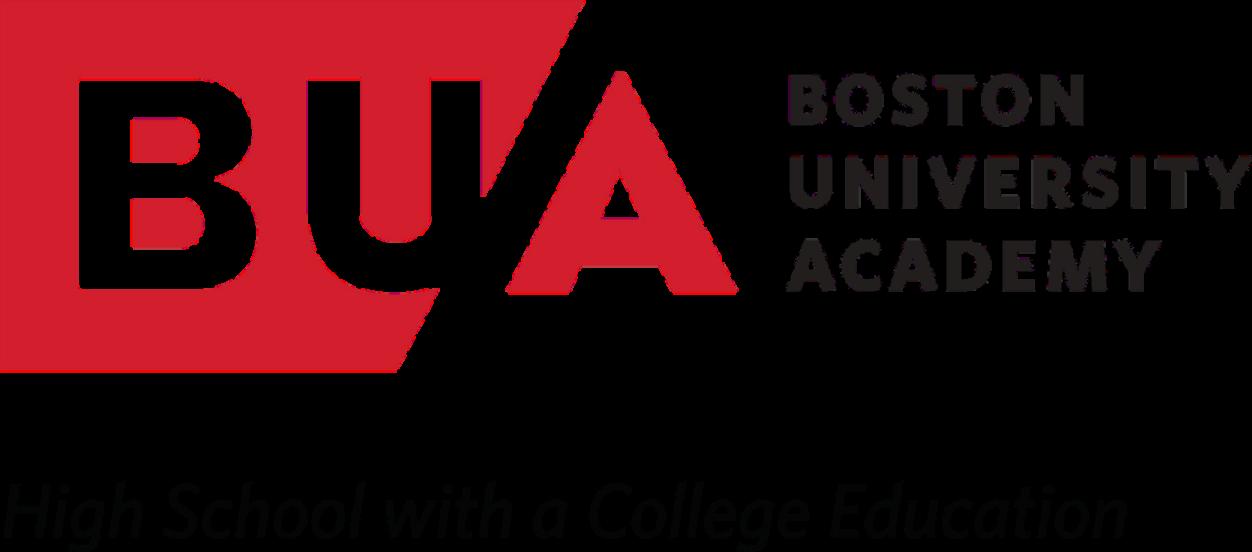