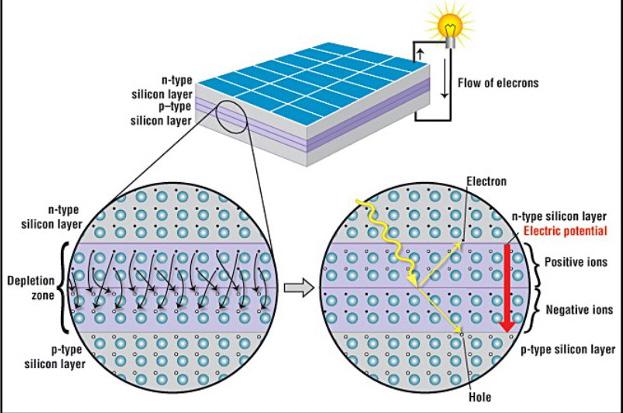
6 minute read
A window to a renewable future
from Issue 29
BY TIA BÖTTGER
silicon can be heavy and bulky, making them difficult to widely install into existing architectural spaces (6). Emerging technologies of transparent solar cells help address the challenge of a very large amount of space required, as any already existing sheet of glass could be converted into photovoltaic cells. The prospect of converting windows in buildings, cars, agricultural sheds, and even the glass panels of electronic devices into energy harvesting devices is an exciting challenge, which would help overcome the current limitations of solar panels and address the high demand for energy (7).
Advertisement
There is an ever-increasing demand for energy in an expanding economy with a continuously growing population. Electricity production generates the largest share of global greenhouse gas emissions, with 60% of our electricity in the U.S. coming from burning fossil fuels, mostly coal and natural gas (1),(2). To continue to meet energy consumption needs while replacing fossil fuels, a rapid expansion of clean renewable energy infrastructure is imperative. Solar energy received on Earth’s surface per year is approximately 120,000 TW, which is 6-7 thousand times more than the current global energy consumption (3). Research into solar energy has been a priority, as it is considered the most abundant source of energy that can satisfy the demands of continual economic development (4). As of 2020, only around 1% of global energy demand could be satisfied by installed solar energy plants, however (5). A large surface area is required for solar energy to be an efficient source of electricity, as photovoltaic cells rely on absorbing light (photons) to create a current. For photovoltaic cells to fulfill energy demand, they must be adopted on a wide scale. Traditional solar panels made from
An understanding of a traditional photovoltaic cell is necessary before exploring emerging technology into transparent photovoltaic cells. Most basically, a photovoltaic cell functions by creating a potential difference, which will drive current to flow through the cell so that energy can be transported to electrical devices. A semiconductor material is used to achieve this, and a photovoltaic cell functions under the same working principle as a semiconducting diode. Semiconductors are crystalline solids, so they are neither metals nor insulators, and their conducting properties are determined by impurities or dopants (4). Silicon is by far the most widely used semi-conducting material, with around 80% of solar cells in the world made using silicon-based materials (4),(8). A slab or film of Si is n- and p- doped in its two surface regions (8). The p-type silicon is produced by adding atoms, such as boron or gallium, which have one less electron in their outer energy level than silicon does. Because one less electron is present than is required to form bonds with the surrounding silicon atoms, an electron “hole” is created. In contrast, n-type silicon includes atoms with one more electron in their outer energy level than silicon, such as phosphorous, leaving the electron free to move within the silicon structure. Where the layers meet, the electrons from the n-type layer will fill the holes in the p-type layer, creating a depletion zone as shown in Figure 1. The p-type layer, initially containing holes, now had excess electrons, and is negatively charged. The n-type layer has lost electrons and is positively charged. Hence, an internal electric field is created within the semiconductor (9). The electric field will direct negative electrons through the material, as they will feel a force in the opposite direction of the electric field (F=qE), and be repelled from the positively charged zone and attracted to the negatively charged zone. When photons from sunlight strike the solar cell, electrons within the silicon material will be knocked loose due to the photoelectric effect, as long as the photon energy (hv) is equal to or larger than the work function of the material (10). These free electrons will move across the cell, directed by the electric field, creating electric current that we use as energy.
With a p-n junction such as described, photons must have an energy greater than or equal to the energy band gap in the semiconducting material for an electron to be freed for an electric circuit (4), (11). This is a limiting factor in the efficiency of solar cells, as lower-energy photons from sunlight will not be used in energy generation. Multijunction cells are a common way to achieve a higher total conversion efficiency. As the name implies, they are comprised of a stack of single-junction cells in descending order of their energy band gaps, so that lower energy photons which aren’t captured by the top cell can still be absorbed by lower-band-gapcells (11). Furthermore, synthesized nanocrystalline dyes are used to expand the range of the wavelength of electron excitation, and efficiency in the excitation process (4). When a photon is absorbed, moving an electron to a higher energy level, little light passes through the material. Light passes through a material when the energy gap of the electrons is higher than the photons, and so the arrangement of atoms and electrons in a material determines its transparency. Therefore, it is a particular challenge to create an efficient solar cell which is also mostly transparent to the human eye.
To maintain a current, a solar cell also needs a thin film of electrode material, such as highly conductive platinum. When a conductor attaches the positive and negative ends of the cell an electrical circuit is formed (11). Electrons in a solar cell are transferred from a front surface electrode to a counter electrode. Finding a transparent counter electrode is a main challenge of transparent solar cells. Most existing transparent solar cell technologies use a conductive oxide glass such as fluorine-doped tin oxide (FTO), and indium-doped tin oxide (ITO), using a thin film with a thickness of less than 20 nm. Combined with the intrinsic optical losses of the glass itself, these layers reduce transparency by approximately 15-20%. Complete transparency in a solar cell is an unrealistic endeavor (4). Instead, researchers aim for near transparency, and there have been some promising developments towards this goal.
There are two main categories of transparent photovoltaic technologies: those which are wavelength selective, making use of compounds which selectively absorb ultraviolet (UV) and/or near-infrared (NIR) light invisible to the human eye, and those which generate electricity from a wide wavelength absorption range and achieve near transparency by segmenting opaque devices or making use of very thin photoactive material (5). For the purposes of this paper, I will only detail one particularly successful development as recent as this year, in which a near-invisible solar cell was created with high efficiency utilizing photon energy in a large range of wavelengths, including visible light.
A team of researchers in Japan led by Toshiaki Kato at Tohoku University exposed an ITO electrode to a vapor of tungsten disulphide (WS2). Under the right conditions, the vapor deposited an atom-thick layer of WS2 onto the ITO conductive glass surface. The monolayer of WS2 acts as a semiconductor, making up the photoactive layer, while the indium-doped tin oxide ITO is the transparent electrode. The researchers were able to precisely control the energy band gap, which electrons need to pass from one material to the other, by coating the ITO in thin metals and placing an insulating layer between the ITO and WS2. In this case, the difference in the work function of the electrode and semiconductor materials could produce a built-in potential, because as the electrons absorbed photons and crossed the WS2 layer, they jumped between the semiconductor’s two energy bands, leaving behind a positively charged hole before moving to the conducting ITO electrode. The voltage difference created allows for electrical energy to be harvested. Furthermore, the team increased the contact barrier height in the ITO-WS2 junction, increasing the voltage difference to be 1,000 times more effective at creating electrical energy than existing ITO-based solar cells. Additionally, their choice of materials was far more transparent, allowing for up to 79% of average visible transmission (7), (12).
Kato’s team also demonstrated how their solar cell could be fabricated on larger scales, expanding the material’s surface area. In other ITO-based solar cells, this led to a voltage drop and decrease in efficiency. With this design, however, a high performance could be maintained in solar cells as large as 1 cm2 in area (7), (12). If the material becomes commercially available, it would have a huge impact. If all the buildings with 90% glass on their surface, such as sky-scraper office buildings, used transparent solar cells on the surface of the glass, the solar cells would have the potential to power more than 40% of that building’s energy consumption, depending on factors such as geographic location and tree cover (4). Another case study of a typical office building found that the energy savings for installation on one glass facade could have a return on investment in just a couple of years (5). Kato’s team imagines that implementing transparent solar cells into electronic devices could allow them to run without the need to ever be plugged into an external grid or power supply. Overall, these advances would revolutionize the use and availability of solar energy and are exciting milestones towards a renewable future.
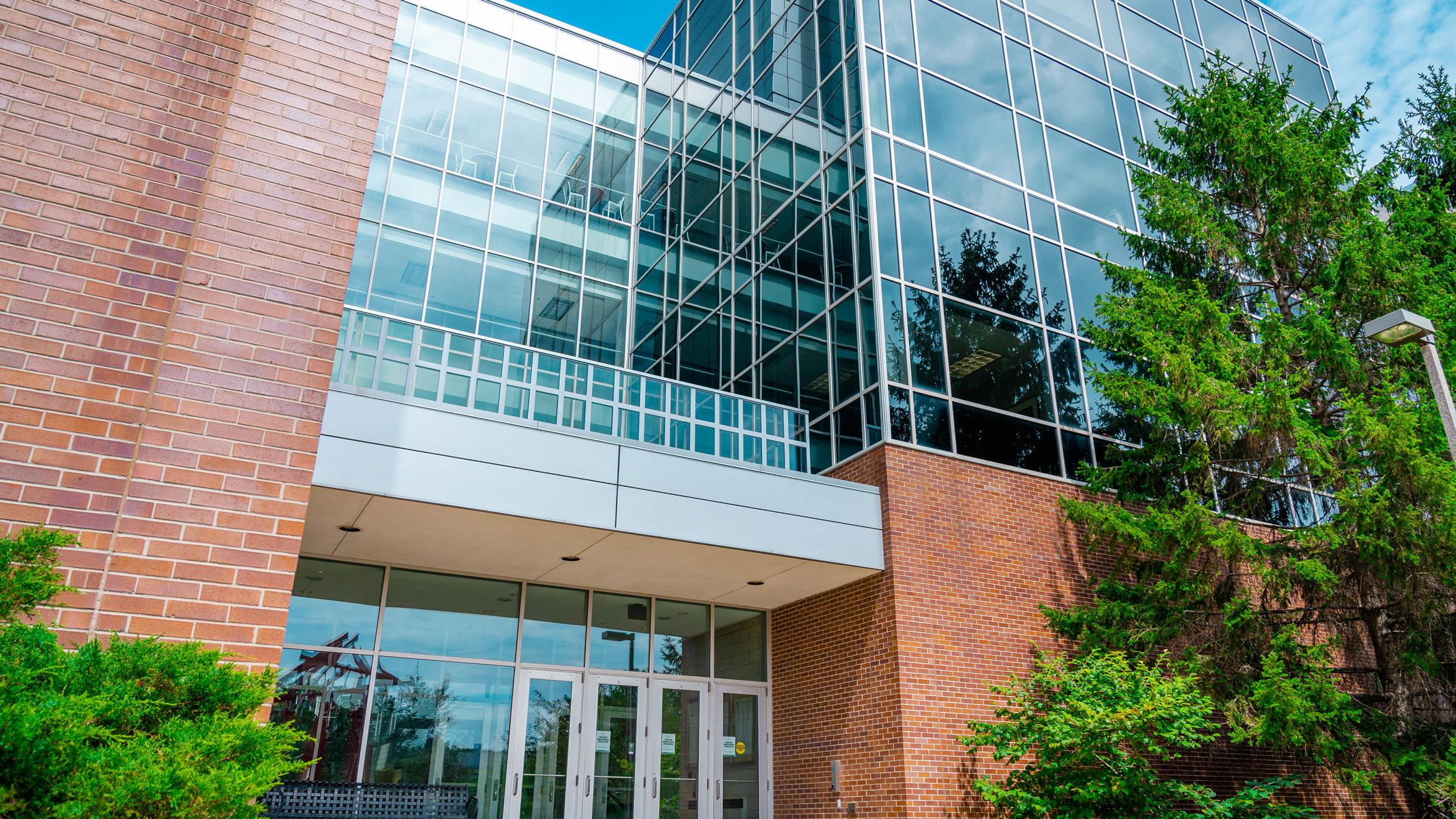