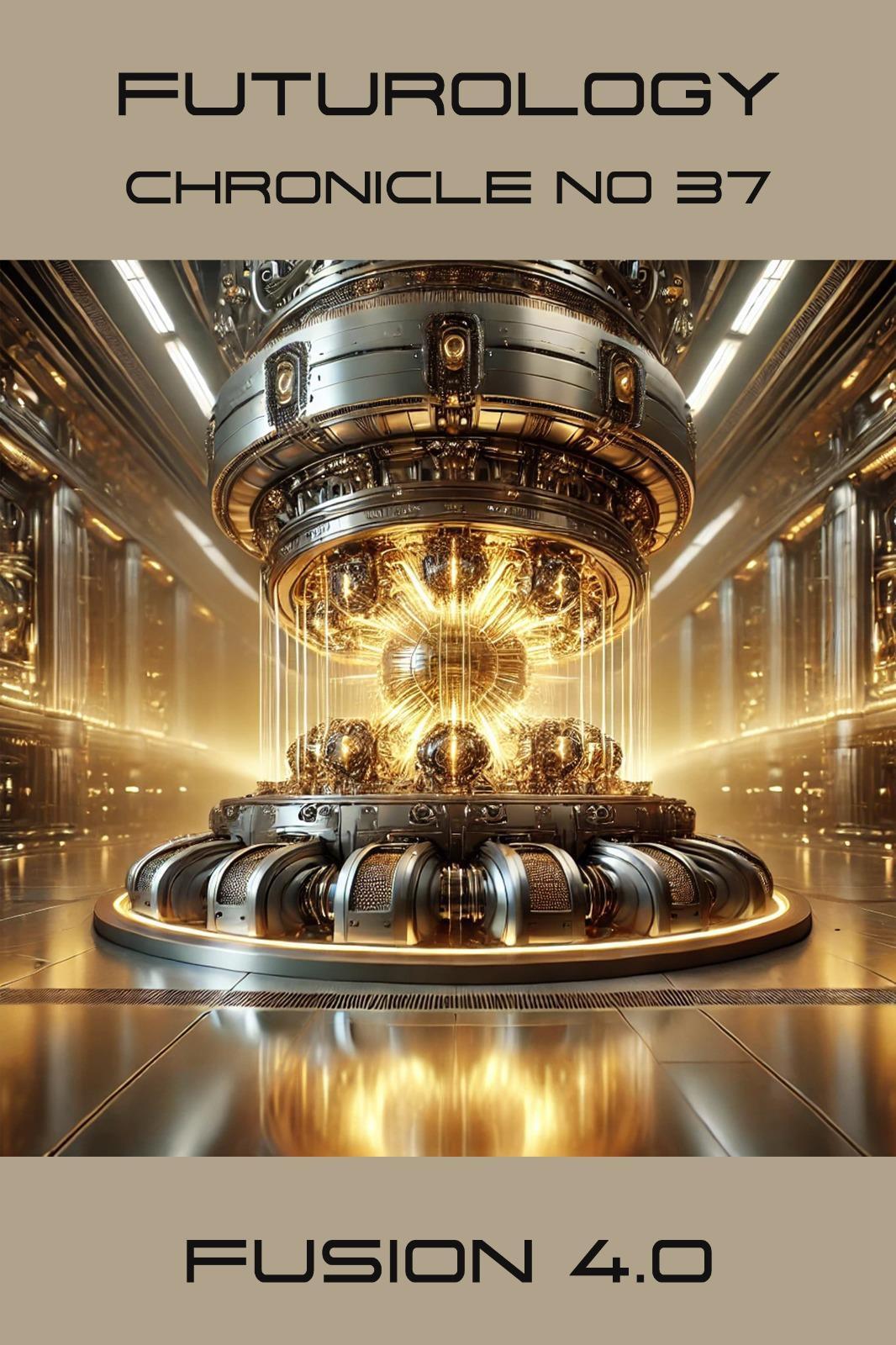

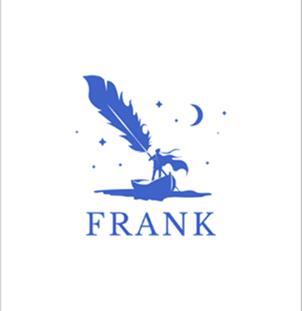
Your Editor of the
Future realism with a scientific flair and an entertaining twist -Independent and Sponsor free
January 2025 – Edition - 5th Year-


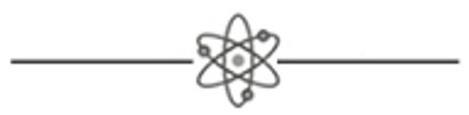

Your Editor of the
Future realism with a scientific flair and an entertaining twist -Independent and Sponsor free
January 2025 – Edition - 5th Year-
Let's cut through the noise: Yes, fusion is too late to save us from the current catastrophe climate. The comfortable fiction of a 1.5°C ceiling has shattered like countless other environmental promises.
Forget the 2050 net-zero goals—warming is racing toward a catastrophic +4°C trajectory.
Confirming the 2012 World bank report “Turn down the heat. Why a +4°C warmer world must be avoided”
“For a thorough exploration of science and answers to this fundamental issue, explore my two books on climate disruption: Earth Polycrisis (2023) and Net Zero-Reality Check (2024)”
For those who would use this truth to dismiss fusion research, they are committing an even graver sin than the polluters who brought us here.
What follows in these pages isn't just another technical manual – it's a battle plan for humanity's energy future.
To the naysayers who suggest we abandon ninety years of fusion research, billions in investment, and countless hours of scientific brilliance, I ask:
Since when did human progress come with an expiration date?
As you'll discover in the chapters ahead, fusion technology has evolved from a scientific dream to engineering reality.
The race to harness the power of the stars has accelerated beyond our wildest expectations, even as skeptics cling to outdated objections.
The delicious irony isn’t lost on me: the same generation that once chanted “power to the people” now stands in the way of the ultimate power source a generation I watched as a teen, not fully grasping their message in those days.
Today's environmental movement, led by aging activists who cut their teeth protesting nuclear weapons, seems incapable of distinguishing between the atomic bomb's destructive force and nuclear fission and fusion's clean potential.
Their arthritis-riddled fingers still point accusingly at anything bearing the word "nuclear," while their grandchildren – armed with physics degrees and environmental conscience – fight to save the very future these protesters once claimed to champion.
As you navigate through the FUSION TECH section, you'll understand why these brilliant young minds are so passionate.
The FUSION RACE chapter will reveal how the global competition for fusion supremacy has transformed from a scientific pursuit into a technological gold rush.
And yes, the REALITY CHECK section will confront the hard truths about our timeline and challenges – because sugar-coating helps no one.
The young engineers and scientists you'll meet on these pages aren't deluded optimists. They’re clear-eyed realists who understand physics and basic math.
Our future will make our current climate disasters look like gentle warnings. Yet these NextGen fusion warriors persist, not because they believe they can prevent the climate crisis –
that ship has sailed – but because they refuse to leave future generations without options.
Think of fusion research as planting an oak tree you'll never sit under.
Our grandchildren's children may inherit a world wracked by climate chaos, but they deserve more than our apologies.
They deserve tools – powerful ones – to fight their way back from the brink. Fusion energy could be their lifeline, their chance to rebuild while finally breaking free from the fossil fuel addiction that brought us here.
The choice before us isn't whether to solve today's crisis – we have already failed at that. The choice is whether we will compound our failure by abandoning tomorrow's solutions.
As you read through these chapters, you'll understand why this technology matters, who's leading the charge, and what stands in our way.
You'll see why the fusion reactors of tomorrow may not save us, but they might just save them.
In a world burning with present troubles, that future possibility isn't just worth fighting for – it's our moral obligation to pursue it. The pages that follow will show you why it matters more than ever.
Nuclear fusion has been a subject of scientific research for decades. The potential for fusion to provide a significant source of energy has driven continuous advancements in the field.
This timeline explores the four generations of nuclear fusion research, highlighting key milestones that have contributed to our current understanding and capabilities in fusion technology.
Generation 1.0: First Laboratory Fusion (1932) The Dawn of a New Era
The story begins in the halls of Cambridge University in 1932. Here, a brilliant physicist named Mark Oliphant (Australian born) was about to make history.
Using an improved version of Ernest Rutherford's (New Zealand born) particle accelerator, Oliphant set out to explore the fundamental nature of atomic nuclei.
The Chapter Illustration depicts Ernest Rutherford personal lab in 1926 where the young Mark Oliphant worked alongside him.
In a groundbreaking experiment, Oliphant fired deuterium particles (a heavy isotope of hydrogen) at each other.
To his amazement, he observed the production of helium-3 and tritium clear evidence of nuclear fusion occurring in a laboratory setting for the first time in human history.
This moment marked the birth of nuclear fusion research. Oliphant's work demonstrated that it was possible to recreate, albeit on a tiny scale, the same process that fuels the sun and stars.
It was a small step, but one that would inspire generations of scientists to come.
The implications were enormous. If fusion could be harnessed, it promised an almost limitless source of clean energy. However, the road ahead would prove long and challenging.
The temperatures and pressures required to sustain fusion reactions were far beyond what 1930s technology could achieve.
For decades following Oliphant's discovery, progress in fusion research was slow. Scientists experimented with various methods to confine and heat plasma - the super-hot state of matter needed for fusion.
But it wasn't until 1968 that the next breakthrough occurred, this time in the Soviet Union. Soviet scientists, led by Lev Artsimovich and Igor Nikolaevich Golovin , had been working on a unique device called a tokamak. The name "tokamak" stands for “Toroidalnaja Kamera i Magnitnyje Katushki” i.e. "toroidal chamber with magnetic coils". This doughnut-shaped machine used powerful magnets to confine and compress plasma.
The team achieved a major milestone with their T-3 tokamak device. They reported plasma temperatures an order of magnitude higher than any other fusion device at the time - a stunning achievement that caught the attention of the global scientific community.
Initially, western scientists were skeptical of the Soviet claims. However, when a team from the UK's Culham Laboratory visited Moscow to verify the results, they confirmed the breakthrough. This led to what became known as the "tokamak revolution".
The success of the T-3 tokamak shifted the focus of fusion research worldwide. Countries around the globe began building their own tokamaks, and international collaboration in fusion research intensified.
The tokamak design became the leading contender in the quest for fusion energy, a position it still holds today.
As tokamak technology improved through the 1970s and 1980s, researchers were able to achieve higher temperatures and better plasma confinement. But the goal - producing more energy from fusion than was input to heat the plasma - remained elusive.
In 1997, a major step towards this goal was achieved at the Joint European Torus (JET) facility in the UK. JET was (and still is) the world's largest tokamak, a collaborative project involving scientists from across Europe.
Using a 50-50 mix of deuterium and tritium fuel, the JET team set a world record for fusion output. They produced 16 megawatts of fusion power from an input of 24 megawatts of heating power.
This represented a fusion energy gain factor (Q) of 0.67 - the closest anyone had come to net energy gain from fusion.
While still falling short of breakeven (where fusion energy output equals input power), this achievement was a major milestone.
It demonstrated that significant fusion power production was possible and provided valuable data for future fusion reactor designs.
The JET results also validated the choice of fuel for future fusion power plants. The deuterium-tritium mix proved far more effective than other potential fuel combinations, producing much higher energy outputs.
This success spurred on plans for even larger fusion experiments. The international ITER project, an enormous tokamak designed to achieve sustained net energy production, was conceived in part based on the promising results from JET.
While tokamaks continued to make steady progress, another approach to fusion was quietly advancing. Inertial confinement fusion, which uses powerful lasers to compress and heat fuel pellets to fusion conditions, had been under development since the 1970s.
On December 5, 2022, this approach yielded a historic breakthrough at the National Ignition Facility (NIF) of Lawrence Livermore National Laboratory in California.
Scientists there achieved fusion ignition for the first time in a laboratory setting, marking the dawn of Generation 4.0 in nuclear fusion research.
The NIF team used an array of 192 powerful ultraviolet lasers to deliver 2.05 megajoules (MJ) of energy to a tiny fuel capsule. This resulted in a fusion energy output of 3.15 MJ - more energy than was used to initiate the reaction. It was the first time in history that a controlled fusion reaction had produced net energy gain. The significance of this achievement cannot be overstated. While the energy gain was small and the experiment far from a practical power plant, it proved that net energy gain from fusion is possible. It represented a fundamental breakthrough that had eluded scientists for nearly nine decades.
This success has reinvigorated the fusion research community and sparked renewed interest from governments and private investors. It's seen as a crucial step towards practical fusion energy, offering hope for a future of abundant, clean power.
Nuclear fusion, the process of merging two atomic nuclei into a single, more stable nucleus, releases a tremendous amount of energy. This energy, in the form of fast-moving particles, shall be captured in a fusion power plant to produce electricity.
Today, fusion research focuses on four primary fuels options, with deuterium and tritium, the isotopes of hydrogen leading the field.
Deuterium contains one proton and one neutron, while tritium has one proton and two neutrons, distinguishing them from ordinary hydrogen, which has just a single proton and a promising protonboron approach.
To trigger fusion, the fuel must first be heated to a point where electrons break free from the nuclei-forming plasma- a state in which ions and electrons move independently.
This plasma must then be heated further, reaching temperatures exceeding 100 million degrees Celsius (200 million degrees Fahrenheit), and confined at high density for a long enough period to enable fusion.
To achieve these conditions, scientists have designed various containment methods, with twenty-two reactor approaches currently under investigation.
The most recognized is the tokamak, a donut-shaped device that uses powerful magnetic fields to hold plasma. However, alternatives, such as stellarators, inertial confinement reactors, and other innovative designs, contribute to the diversity of experimental approaches aimed at mastering fusion.
Beyond the challenge of designing a viable reactor, there are ten critical challenges to overcome before constructing the first pilot "demo" plant and the sixteen innovative attempt to solve them.
These obstacles range from achieving and maintaining extreme temperatures, to handling materials that can withstand intense neutron bombardment, to managing the intricate engineering requirements of large-scale fusion systems.
Addressing each of these hurdles is essential to turning fusion into a reliable energy source. Fusion reactors work by creating and confining plasma in devices like tokamaks, where magnetic fields act as invisible tracks, guiding ions and electrons around the chamber.
By injecting additional energy, scientists accelerate the fuel particles to high speeds, causing them to collide and fuse, releasing energy primarily in the form of fast neutrons. However, fusion particles tend to drift from the core and impact on the inner walls, risking structural damage and fuel contamination.
To counter this, reactors employ a divertor a special chamber that channels stray particles and removes excess heat to safeguard the reactor.
The fusion process then relies on what scientists call the “triple gain”—a combination of plasma density, confinement time, and temperature—as a benchmark for success.
This concept, initially proposed by physicist John D. Lawson in 1955, established the key conditions for achieving an energy output greater than the input. Modern fusion research uses this "triple gain" approach to guide reactor designs toward sustained, self-sufficient energy production.
In the following chapters, I will cover these topics in detail, factchecking each one with objectivity and without getting carried away by the optimistic hype that surrounds the fusion sector.
Nuclear fusion and nuclear fission stand as two distinct paths toward power generation, each with unique characteristics that highlight the strengths and challenges of their respective approaches.
Fusion, the same process that powers our sun, involves combining light elements like deuterium and tritium. In contrast, fission, the backbone of today’s nuclear reactors, splits heavy elements like uranium and plutonium. While fusion aims to recreate stellar reactions here on Earth, fission relies on splitting atoms for energy a fundamental difference that shapes the technology, safety, and waste of each method.
Fusion’s light-element fuels are available and inherently safer, primarily using isotopes of hydrogen. Fission, however, requires rarer heavy elements, such as uranium and plutonium, which have higher radioactivity and complex handling requirements.
One of fusion’s biggest advantages is its waste output: helium, a nonradioactive gas that poses no long-term storage issues. Fission, on the other hand, generates highly radioactive waste, creating storage challenges that can last for centuries. This stark contrast has pushed fusion into the spotlight as a cleaner energy source.
Fusion is inherently safe; if a reactor is damaged, the reaction will naturally stop. Fission, however, poses greater risks, as the reaction can continue even after a reactor shutdown. This fundamental difference underscores fusion’s appeal as a safer option for largescale energy production.
Despite its promise, fusion technology faces enormous challenges in achieving and maintaining the high temperatures and pressures needed for sustained reactions. Fission, while established, still requires advancements to improve efficiency and lower costs. Fusion may hold the key to a safer, cleaner energy future, but reaching commercial viability remains a complex hurdle.
As an early conclusion nuclear fusion and nuclear fission are very distant cousins, each with its own approach to generating pair. But the differences between them could not be more striking.
Fusion advocates see it as the safer option: if a reactor faces trouble, the reaction halts naturally. However, achieving and sustaining the intense temperatures and pressures needed for fusion has been a monumental challenge for now.
On the other hand, present conventional nuclear reactors rely on fission, where heavy elements like uranium and plutonium are split to release energy.
While this technology is ill-established, it comes with significant tradeoffs. Fission generates highly radioactive waste, requiring careful handling and storage.
Safety is another issue: if things go wrong, a fission reaction can persist, making accidents potentially catastrophic.
The promise of fusion is clear: cleaner and safer energy. But for now, fission dominates as fusion continues its journey from lab experiments to a hopeful energy future.
In essence, while fission technology powers today’s reactors, fusion continues to inspire with its potential for a safer and cleaner tomorrow.
The journey from concept to reality for fusion may be long, but the outcome could redefine a “safe” future of energy.
Fusion research has traditionally revolved around deuterium-tritium (D-T) as the go-to fuel. However, a broader examination of other options deuterium-deuterium (D-D), deuterium-helium-3 (D-He3), and proton-boron-11 (p-B11) reveals alternative pathways.
This expanded view is crucial to addressing the limitations of D-T while pursuing more sustainable and innovative energy solutions.
D-T is favoured for its lower ignition temperature of 100 million Kelvin and high energy yield, making it the leading choice for nearterm fusion reactors like ITER. Deuterium is abundant and nonradioactive, while tritium must be bred within the reactor, typically using lithium-based breeder blankets.
This introduces engineering complexities, including tritium extraction systems and managing neutron-induced material degradation. Despite its challenges, D-T remains the most viable option for immediate progress.
D-D fusion eliminates reliance on tritium, utilizing the abundant deuterium isotope found in seawater. However, it demands much higher temperatures (400-500 million Kelvin) and produces lower energy yields. The less energetic neutrons generated reduce material damage compared to D-T, offering potential cost savings in reactor maintenance.
While D-D fusion simplifies fuel sourcing and waste management, achieving the required temperatures remains a significant technical barrier.
D-He3 reactions produce minimal neutrons, reducing radioactive waste and material degradation. This allows for direct energy conversion from charged particles, bypassing conventional heat systems. However, helium-3 is scarce on Earth, with lunar mining as a speculative solution.
The extreme ignition temperature of over 600 million Kelvin further complicates its feasibility. While promising in theory, D-He3 fusion faces significant logistical and technical obstacles.
p-B11 fusion produces no neutrons, yielding only helium nuclei. This allows for direct electricity generation and reduces radioactive byproducts. However, it requires over 1 billion Kelvin to initiate, the highest among fusion fuels, and generates intense x-ray radiation that challenges reactor material integrity.
While experimental setups like field-reversed configurations show potential, p-B11 remains a distant goal, requiring breakthroughs in plasma confinement and radiation management.
In conclusion none of the four fuels has yet proven fully conclusive. Each presents a mix of advantages and challenges, from D-T's nearterm practicality to p-B11's long-term potential.
My personal scientific analysis leans toward proton-boron-11 for its aneutronic promise, but I continue to follow all research progress step by step, recognizing the importance of exploring every pathway toward achieving sustainable fusion energy.
While the magnetic confinement tokamak and stellarator dominate discussions about fusion energy, a broad spectrum of innovative designs exists. These designs, ranging from incremental improvements to groundbreaking concepts, are vital to accelerate fusion energy development.
This summary introduces 22 experimental approaches, shedding light on their unique methodologies and contributions.
The tokamak remains a cornerstone of fusion research. Utilizing powerful magnetic fields to confine plasma, tokamaks like ITER aim to achieve a net energy gain. Key advancements include superconducting magnets and AI-driven predictive controls. However, challenges such as plasma instability and extreme material stresses remain significant barriers.
Stellarators like Germany’s Wendelstein 7-X employ intricate magnetic configurations to confine plasma without requiring external current. Their steady-state operation and inherent stability make them promising, though manufacturing complexities and lower performance compared to tokamaks present challenges.
This approach compresses fuel pellets using lasers or impact, creating the extreme conditions necessary for fusion. Facilities like the National Ignition Facility (NIF) have achieved significant milestones, but achieving consistent net energy gain remains elusive.
Compact designs like the UK's MAST Upgrade offer enhanced efficiency through innovative geometry and features like the Super-X divertor, which mitigates heat loads. These advancements could pave the way for smaller, economically viable fusion reactors.
Using particle accelerators to propel ions at fusion targets, this approach mirrors high-energy physics experiments. Facilities like GSI in Germany explore its potential for high repetition rates, offering continuous operation possibilities.
Distinct from other inertial methods, laser-driven systems use ultraintense laser beams for plasma compression. While demanding in precision and complexity, these systems hold promise for highenergy physics applications beyond power generation.
Compact and cost-effective, DPF devices generate plasmoids capable of high-energy densities. Companies like LPP Fusion are exploring aneutronic reactions for neutron-free energy conversion, though stability and scalability remain hurdles.
8. Plasma Liner Compression Fusion (PLCF)
This hybrid uses converging plasma jets to compress fusion fuel. Modular and adaptable, PLCF appeals for its potential to simplify reactor designs and expand fuel options.
Using X-rays generated within a hohlraum to compress fuel capsules, this method prioritizes uniformity. Breakthroughs at NIF highlight its potential, but energy conversion inefficiencies persist.
RFPs rely on self-generated magnetic fields for plasma confinement. Their compact designs and higher plasma currents offer advantages, though they are less mature than tokamaks or stellarators.
Z-pinch systems use electrical currents to compress plasma. Advances by Zap Energy promise to address historical instability issues, presenting a simplified alternative to complex magnetic confinement designs.
Inspired by planetary magnetospheres, this system uses a suspended superconducting ring to confine plasma. New Zealand’s Open Star Technologies is pioneering this approach with modular, hightemperature superconductors.
Combining magnetic and inertial confinement techniques, MTF compresses magnetized plasma with mechanical or plasma liners. General Fusion’s unique piston-driven model exemplifies this hybrid's practical potential.
Distinct yet related to MTF, magneto-inertial methods operate within an intermediate density regime. Helion Energy’s compact, aneutronic reactors exemplify its innovative application.
With its self-contained plasma structure, the FRC promises compact designs and compatibility with advanced fuels. Companies like TAE Technologies lead this field with beam-driven plasma innovations.
Using muons to overcome nuclear repulsion at room temperatures, this approach offers low-temperature fusion possibilities. However, its energy balance challenges limit practical applications.
Studying energy release during magnetic reconnection, this Princeton-based initiative informs both astrophysical phenomena and fusion energy advancements.
Using magnetic mirrors to trap plasma, these linear devices face challenges like end losses but influence broader fusion research, particularly in plasma heating.
Combining magnetic and electrostatic confinement, the Polywell leverages a high-density plasma core for fusion. While promising, technical and engineering hurdles remain.
Popularized by Farnsworth, IEC uses electrostatic fields to accelerate ions for fusion. Despite scalability issues, its simplicity offers educational and research benefits.
Accelerating particle beams to collide directly, this method simplifies containment systems. TAE Technologies integrates this with plasma techniques for niche applications.
Historically controversial, LENR has regained interest for its low-cost, low-temperature methodology. Emerging technologies like Japan’s QHe and U.S.-based Brillouin Energy point to industrial applications.
My scientific instinct finds LENR a compelling pathway, combining cost-effectiveness and practical scalability.
While tokamaks and stellarators dominate the fusion energy narrative, the array of approaches under experimentation worldwide offers untapped potential.
LENR, with its simplicity and low cost, resonates with my scientific instinct as a practical complement to mainstream fusion research, demanding further exploration and rigorous validation.
This diverse ecosystem of ideas may collectively lead to the realization of sustainable fusion power.
The "triple gain" concept is central to achieving viable nuclear fusion energy. It refers to the delicate balance of three factors temperature, plasma density, and confinement time required to produce more energy than the input.
Fusion demands extreme temperatures exceeding 100 million degrees Celsius, where atomic nuclei can overcome their natural repulsion, known as the Coulomb barrier, and fuse together.
High plasma density ensures sufficient collisions between nuclei, while confinement time dictates the duration these conditions are maintained to achieve net energy gain.
Despite significant advancements, ten core challenges persist in translating this theoretical framework into practical fusion power.
Sustaining High Temperatures Achieving and maintaining the extreme temperatures required for fusion reactions remains a daunting task. Plasma heating methods must balance energy input with losses from radiation and conduction.
Stable Plasma Confinement Plasma confinement through magnetic or inertial methods faces turbulence and instabilities, such as Edge Localized Modes (ELMs), which can disrupt operations and damage components.
Energy Balance Reaching ignition, where fusion reactions selfsustain and produce surplus energy, is critical. Current designs struggle with maintaining the necessary energy balance.
Neutron Damage High-energy neutrons generated during fusion degrade reactor materials, reducing their lifespan and increasing operational costs.
Material Durability Reactor walls must withstand extreme heat, radiation, and particle bombardment. Innovations like tungsten alloys and liquid metals aim to address these issues but are still under development.
Tritium Breeding and Handling Tritium, a key fuel component, is scarce and must be bred in reactors using lithium. Efficient breeding, handling, and recovery systems are essential for sustainable operations.
Fuel Cycle Complexity The fusion fuel cycle involves intricate processes, including isotope separation, impurity removal, and tritium recovery. Managing these efficiently is crucial for commercial viability.
Scaling Up Transitioning from experimental setups to full-scale power plants introduces challenges in size, complexity, and economic competitiveness with existing energy sources.
Energy Extraction Converting fusion energy into electricity through heat exchangers or direct methods like alpha particle capture is not yet optimized.
Economic Viability Fusion must compete with established energy technologies, necessitating cost reductions in reactor construction, maintenance, and fuel sourcing.
Fusion energy's promise lies in its potential to provide a clean and virtually limitless energy source. However, significant technical and economic hurdles remain.
The path to commercialization demands addressing these ten challenges while maintaining incremental progress across all aspects of fusion research.
Building upon the ten challenges outlined in the preceding chapter, the scientific community has developed an array of innovations aimed at overcoming these hurdles.
From plasma stability and material resilience to energy efficiency and scalability, these 16 breakthroughs represent the forefront of fusion energy research.
Each innovation addresses critical gaps, pushing the boundaries of what is possible and redefining the trajectory toward achieving practical, sustainable fusion power.
Together, they form a robust response to the multifaceted challenges facing the fusion sector today.
AI for Reactor Design and Stability Artificial Intelligence accelerates reactor design by replacing traditional simulations with machine learning models, drastically reducing development time. AI also stabilizes plasma in real-time, preventing tearing instabilities through predictive adjustments to reactor parameters.
Breaking the Greenwald Limit New plasma density limits surpass the Greenwald threshold, enabling tokamaks to operate with nearly double the fuel density. This breakthrough enhances energy output and reshapes tokamak scalability.
Boron-Treated Walls Boron coatings on tungsten walls mitigate erosion and plasma contamination, significantly improving reactor durability and efficiency across global fusion facilities.
Attosecond Plasma Diagnostics Advanced diagnostics capture electron dynamics in attosecond intervals, improving plasma behavior understanding and enabling real-time optimization of fusion reactions.
High-Temperature Superconducting (HTS) Magnets HTS magnets, with superior field strength and efficiency, enable compact reactor designs and reduce operational costs. Their quench-resistant properties further enhance reliability.
Iron Silicate Reactor Linings Nanoparticles of iron silicate in reactor walls disperse energy from particle collisions and self-heal under high heat, extending vessel lifespans.
Plasma Pedestal Magnetic Innovations Magnetic perturbations reduce plasma instabilities while boosting overall performance, addressing critical edge-localized mode (ELM) challenges in tokamaks.
Negative Triangularity Configurations Negative triangularity plasma shapes suppress instabilities, paving the way for more stable and efficient compact fusion devices like Spain’s SMART project.
3D-Printed Fusion Targets Dual-wavelength 3D printing techniques produce precise fusion fuel targets at scale, solving bottlenecks in ignition target manufacturing.
Room-Temperature Superconductors While still under scrutiny, room-temperature superconductors could revolutionize magnetic confinement and energy systems, eliminating costly cooling infrastructure.
Nanoresonators for Diagnostics Nanomechanical resonators, capable of precise measurements in extreme environments, enhance diagnostics and optimize fusion fuel dynamics.
Omnigenous Magnetic Fields A novel approach to magnetic field design simplifies stellarator configurations while maintaining plasma confinement efficiency.
Thrusters and Fusion Synergies Insights from plasma thruster development inform turbulence and plasma-wall interaction management, benefiting both propulsion and fusion applications.
Low-Energy Nuclear Reactions (LENR) LENR technology offers pragmatic, scalable energy solutions, particularly suited for off-grid or extraterrestrial applications.
Spin polarisation has emerged as a breakthrough in nuclear fusion, with Princeton Plasma Physics Laboratory demonstrating its potential to enhance tritium efficiency up to tenfold. This advancement could enable smaller, safer, and cost-effective reactors, though technological challenges remain.
Quantum Kinetics' Fusion Milestone Quantum Kinetics
Corporation achieved sustained plasma temperatures of 200 million degrees Celsius for 24 hours, advancing waste transmutation and energy generation.
Kiwi-Led Fusion Commercialization Entrepreneurs in New Zealand capitalize on fusion-born technologies like HTS magnets, applying them across industries while maintaining focus on fusion goals.
Among these transformative innovations, the concept of roomtemperature superconductors stands out for its revolutionary potential.
Although still under scrutiny, its validation could redefine not only fusion energy but also industries far beyond, heralding a technological leap of unprecedented scale.
The International Thermonuclear Experimental Reactor (ITER) stands as a monumental effort in fusion energy research, symbolizing one of humanity's most ambitious scientific collaborations. Conceived during the Cold War as a beacon of peaceful international cooperation, ITER has evolved into a staggering global project.
Yet, this venture has faced overwhelming criticism for its spiralling costs, constant delays, and geopolitical complexities. Despite these challenges, ITER has inspired over 45 innovative private fusion ventures, a rare bright spot in an otherwise contentious journey.
Initiated in 1985 during the Geneva Superpower Summit, ITER was conceived as a geopolitical and scientific milestone. By 2007, its organizational framework brought together seven major global powers China, the EU, India, Japan, Korea, Russia, and the United States with shared goals of advancing fusion energy. Situated in Cadarache, France, ITER represents over 35 nations and a financial commitment exceeding €20 billion.
The project aimed to achieve its first plasma by 2025, later adjusted to 2035 and, more cautiously, 2039. Delays have been attributed to engineering complexities, material challenges, and global coordination issues. ITER's scale, from its 5,000-ton vacuum vessel to its kilometer-long superconducting magnets, underscores the unprecedented difficulty of this venture.
ITER’s journey has been marked by persistent financial overruns and management challenges. Initial estimates of €6 billion ballooned to €20 billion, with further increases likely. Critics argue that the project epitomizes bureaucratic inefficiency, with delays often framed as
opportunities for innovation. Its reliance on Russian tungsten and magnet technology has also sparked geopolitical concerns amidst ongoing sanctions.
The criticism extends to ITER's timeline, which remains perpetually 15 years from completion. Observers question whether the project can deliver meaningful results or if it is merely a "masterclass in deadline dodging," consuming public funds with limited accountability.
Despite its controversies, ITER has sparked a wave of private sector innovation. Over 45 companies across 15 countries are now actively pursuing fusion energy solutions, exploring smaller, more agile reactor designs.
This private-sector dynamism will be ultimately ITER's most enduring legacy, as it accelerates the commercialization of fusion technologies and diversifies the path toward sustainable energy.
ITER represents a paradox: a titanic scientific endeavour with a monumental risk of failure, yet capable of catalyzing transformative progress. While its future remains uncertain, ITER has undeniably shaped the global fusion narrative.
ITER is a “Titanic build in a car park… in Provence," embodying both the grand ambitions and the glaring pitfalls of humanity's quest for fusion energy by incredible chance leaving a bright spot!.
Since the dawn of fusion research's Generation 4.0 in 2022, marked by the breakthrough at the Lawrence Livermore National Laboratory, the fusion sector has accelerated its development pace. A total of 23 nations, alongside over 45 private ventures, are now vying for supremacy in this transformative field.
This narrative examines the contributions of key nations and private enterprises, highlighting a global race to deliver commercial fusion energy.
• United States: Boasting the largest private fusion sector, with 25 companies like Commonwealth Fusion Systems and TAE Technologies, the U.S. leads in both government and private efforts. Research hubs such as the National Ignition Facility and DIII-D tokamak drive public initiatives, while startups attract significant investment to develop innovative reactor designs.
• China: Rapidly catching up, China has achieved breakthroughs with its Experimental Advanced Superconducting Tokamak (EAST) and plans for the CFETR. Leveraging centralized planning and its prowess in superconducting technologies, China demonstrates both domestic growth and strategic international collaborations.
• European Union: Fragmented but innovative, Europe supports diverse programs through EUROfusion. The UK’s STEP program and private ventures like Tokamak Energy highlight private innovation, while France remains a central figure with ITER and related national strategies.
• Japan: A leader in plasma research, Japan advances with JT60SA, a world-class tokamak developed in partnership with Europe. Japan also plans ambitious DEMO reactors for the 2040s.
• South Korea: With projects like KSTAR and private-public partnerships like Enable Fusion, South Korea combines government investment with private sector ingenuity to emerge as a fusion leader.
• India: Balancing state initiatives such as SST-1 with private ventures like Anubal Fusion, India is positioning itself as a rising power in the fusion sector.
• Spain: A champion of stellarator research, Spain focuses on the TJ-II device and broader international collaborations.
• Sweden: Known for Novatron’s simulation-driven approach, Sweden pioneers elegant, efficient designs.
• Australia: With private ventures like HB11 Energy and university-backed initiatives, Australia aims to establish itself in laser-boron fusion.
• New Zealand: An emerging innovator, New Zealand has gained attention for its work on levitated dipole reactors (LDRs) and recent breakthroughs. Their ingenuity reflects a determination to punch above their weight in the global race.
For a more comprehensive account of these nations and private ventures, refer to my book Fusion 4.0 Reality Check. Available on Amazon.
The fusion sector is entering an unprecedented venture boom, with diverse national strategies and private innovations pushing the envelope.
This momentum is expected to accelerate further as countries and companies strive to achieve the first true demonstration reactor by 2032. This dynamic era underscores a competitive yet collaborative push toward unlocking fusion's potential, heralding a transformative future for global energy.
The global fusion race has entered a phase of unprecedented ambition. The market, valued at $312 billion in 2023, is projected to reach $543 billion by 2033. Investments are surging, with private ventures and governments pouring billions into advancing fusion energy.
Yet, amid these optimistic announcements, the reality is far more nuanced. Beneath the surface of breakthroughs and milestones lie significant challenges that highlight the fragile foundation of these ambitious goals.
This chapter critically examines the promises, pitfalls, and paradoxes of fusion energy's progression, offering readers a balanced view of the global pursuit of this transformative technology.
1. The Economic Mirage While the fusion sector boasts rapid growth, its financial ecosystem remains precarious. Despite securing over $7 billion in investments by 2024, the sector faces inefficiencies and high costs. The heavy reliance on government funding and costly infrastructure raises concerns about longterm economic viability.
2. Scientific and Engineering Gaps
Fuel Cycle Complexities: Deuterium-Tritium (D-T) reactions depend on tritium, which is scarce and difficult to handle. Alternative fuels like Deuterium-Deuterium (D-D) require extreme conditions, making them commercially challenging.
Material Limitations: The plasma-facing components of reactors must withstand extreme heat and neutron bombardment. Current materials lack the durability needed for long-term reactor operation.
o
3. The Energy Balance Illusion Recent breakthroughs, like achieving fusion ignition, often omit the full energy balance. For example, the National Ignition Facility's achievement in 2022 produced more energy than injected into the fuel, but not when factoring in the system's overall energy consumption.
4. Geopolitical Realities Nations like China are leveraging industrial ecosystems to dominate the fusion supply chain, creating dependencies that worry Western countries. The parallels with China's control of solar and wind industries highlight the risk of losing technological independence.
5. The Private Sector's Role Over 45 private companies are now engaged in fusion research, but their timelines and achievements remain speculative. While these ventures inspire innovation, their reliance on long-term funding and untested technologies adds uncertainty to the fusion race.
Amid this landscape of progress and scepticism, one bright spot stands out: the transformative inspiration fusion research has drawn from other fields, particularly biotech. Yet, even here, irony reigns. As biotech veterans offer advice, fusion researchers, armed with AI and digital twins, are navigating a much faster-paced innovation cycle.
To borrow from biotech’s well-intentioned but patronizing guidance: "Standardize your milestones, and don’t forget to engage with the public." The fusion sector may smile politely at such advice, but the underlying reality remains fusion’s path to success is uniquely its own.
One hundred years after Sir Marcus Oliphant’s pioneering laboratory demonstration of nuclear fusion in 1932, the dawn of Generation 4.0 has brought fusion energy closer to realization.
With the Livermore ignition success in 2022 and significant advances in both tokamak designs and alternative approaches, a new optimism surrounds the sector.
For the first time, predictions of commercial fusion power are converging around the early 2030s, aligning with the centenary of Oliphant’s groundbreaking experiment.
While challenges persist scaling reactions, advancing materials science, and solving engineering complexities these hurdles appear increasingly surmountable.
A poll of 45 fusion projects reveals a consensus, with most targeting 2030-2035 as the window for delivering grid-ready power.
And very possibly 2032 that will mark the anniversary of Oliphant discovery.
This marks a departure from the infamous “30 years away” refrain, signaling a tangible shift in ambition and confidence.
By 2032, in what would be 100 years after Oliphant’s first experiment, we will likely enter Fusion 5.0, celebrating not only a historic milestone but also the tangible realization of fusion energy’s promise.
This transition will test whether fusion can move from scientific aspiration to practical achievement, delivering on its potential to combat climate change and secure a clean energy future.
In this Futurology Chronicle No. 37 – FUSION 4.0, we explore the global fusion race, contrasting national approaches, technological innovations, and the geopolitical dynamics at play.
My hope is for readers to reflect on just how many more "fusion years" remain before this long-standing dream becomes reality.
While our generation may not fully reap the benefits of fusion energy, our descendants are counting on us. Their future hinges on today’s commitments to solving this energy challenge.
As the clock ticks and climate pressures intensify, the urgency is clear. Will we rise to meet the challenge or risk leaving the promise of fusion unfulfilled?
The time to act is now, and the world is watching. Fusion must deliver—not decades from now, but in time to secure the planet’s future.
ARE YOU NOW A FAN OF FUSION ENERGY AND EAGER TO EXPLORE IN DEPTHS, Then I humbly recommend you check out my book available on amazon
The industry Lobbies
Fusion Industry Association (FIA)-800 Maine Ave SW Suite 223- Washington DC 20024 www.fusionindustryassociation.com CEO : Andrew Holland
EURO fusion-Boltzmannstr.é-85748 Germany www.euro-fusion.org – Professor Ambroglio Fasoli -CEO & Program Manager
Nuclear Business platform-151 Chin Swee Road-Manhattan house - Singapore www.nuclearbusiness-platform.com-CEO- Mr Zaf Coelho
The international Agencies
International Atomic Energy Agency (IAEA:) www.iaea.org
World Nuclear Association: www.world-nuclear.org
The industry publications
World Nuclear New :www.world-nuclear-news.org
Nuclear Engineering International: www.neimagazine;com
Nuclear Newswire: www.ans.org
Ignition News: www.ignition-news;com
Nucnet: www.nucnet.org
Other Media writing regularly on the subject
wwwenergyintel.com www.Interesting engineering.com www.quantamagazine.com www.neozone.org www.livescience.com www.asia.nikkei.com www.theconversation.com www.scientificamerican.com
www.phys.org
www.japantimes.com
www.scmp.com (China)