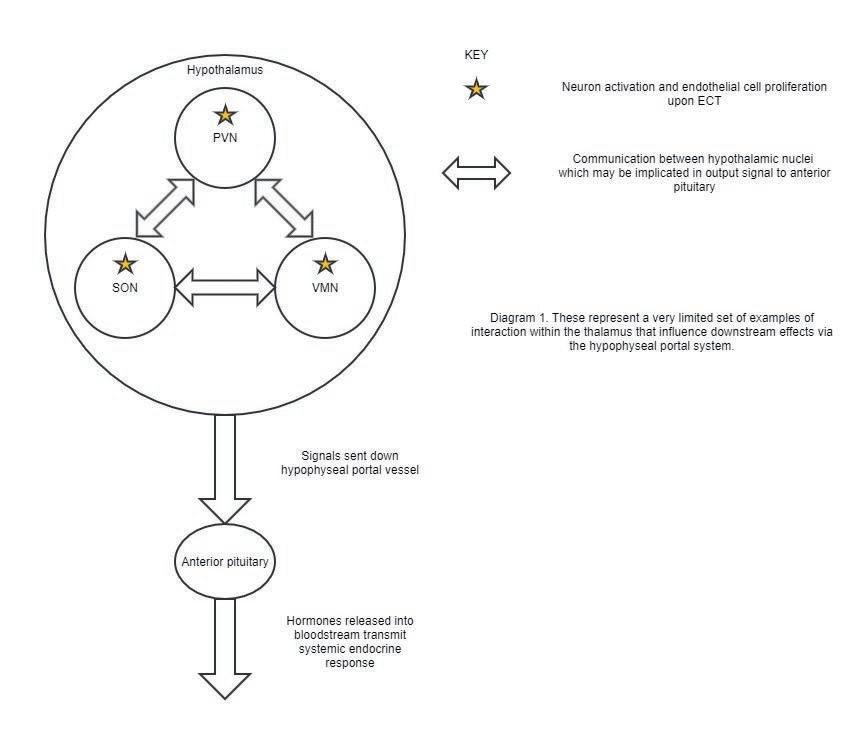
28 minute read
Electroconvulsive Therapy Uses and Its Ability to Induce Neurogenesis: A Literature Review
Kylar J. Harvey1*, Gwyneth J. Harris1*, Alexander I. Greenstone1*, Catherine L. Falzone1*, and Sami R. Hasan1*
¹Geisinger Commonwealth School of Medicine, Scranton, PA 18509 *Master of Biomedical Sciences Program Correspondence: shasan@som.geisinger.edu
Abstract
Electroconvulsive therapy (ECT) has been used historically to treat depression, seizures, and many other psychiatric symptoms. With the investigation of the mechanisms of action of ECT, physiological changes were noted in patients treated with ECT where neurogenesis was consistently linked to reductions in symptoms of many psychiatric illnesses. Neurogenesis is a prolific area of clinical neuroscience research with a wealth of current research and possibilities for future research. Also linked to the therapeutic benefits of ECT are neuronal activation and endothelial proliferation in the midhypothalamic nuclei. The hypothalamus is a key regulator of endocrine function, so further research investigating the implications of ECT-induced mid-hypothalamic changes on endocrine functions of patients is warranted and may illuminate some potential therapeutic pathways for ECT. In addition, these hypothalamic changes do not represent the sum of all neurological changes following ECT, so interactions between the mid-hypothalamic changes and other neuro-anatomical changes after ECT should be considered to develop a more complete model of ECT’s therapeutic action in treating depression and epilepsy. ECT has promise for diseases of aging as, with aging, drug metabolism is altered, making pharmacotherapeutic intervention less consistent within this population. With an understanding of ECT comes more confident use of the treatment in the clinical setting and could help physicians decide when ECT is appropriate as the first line of treatment.
Introduction
The generation of new cells from stem cells is a lifelong process sustained in a multitude of cells. In the case of neurons, they arise from a resident population of progenitors throughout adulthood via neurogenesis, proliferation, and differentiation of adult stem cells (1, 2). Neurogenesis is heavily studied in rodents due to ethical concerns and its conservation in mammals. There are three classes of neural stem cells and progenitor cells in rodent nervous systems: neuroepithelial cells, radial glial cells, and basal progenitors. With each cell type, there is a respective type of division-symmetric, proliferative division; asymmetric, neurogenic division; and symmetric, neurogenic division (3). Symmetric proliferation is defined as producing two daughter cells of the same fate, while asymmetric proliferation generates a single daughter cell that is identical to the mother cell and a second nonidentical cell. Asymmetric divisions, interestingly, may continue to replenish the stem cell pool but lack the ability to regulate adult neurogenesis (4). Neuroepithelial cells are considered true stem cells due to their ability to differentiate and self-renew, while the radial glial cell and basal progenitors are restricted to a single cell fate and are unable to self-renew (3). The type of division and proliferative or differentiation direction is determined by many factors relating to specific epithelial cell characteristics: the apical-basal polarity and cell cycle length (1, 5). Neural progenitors may be influenced by their microenvironments, mainly through the influence of distal and proximal neurons, making them subject to extrinsic regulation (3). Gamma-aminobutyric acid (GABA) signaling is essential for both early neuron depolarization and mature neuron hyperpolarization; the latter is crucial for initiating proper reception of glutamatergic inputs (6). The subgranular zone (SGZ) progenitors, namely the dentate granule cells, receive excitatory glutamatergic and inhibitory GABAergic signals from the local interneurons while also being influenced by different neurotransmitters from distal brain areas (7). Cell fate determination is influenced by neurotransmitters, specifically GABA, glutamate, and nitric oxide (NO), prior to neurogenesis. Recent observations have indicated that GABA and glutamate also play a role in the control of neurogenesis (6, 8, 9). Neurogenesis can occur in the hippocampus and olfactory bulb of adult mammals, whereas it was previously thought to not take place outside of embryonic and early postnatal periods (1, 10). The process occurs in the SGZ of the dentate gyrus within the hippocampus, where it is relevant for some forms of learning and memory, and the subventricular zone (SVZ) of the lateral ventricles where ependymal cells are suspected of being the resident adult NSCs (5, 10, 11). Just like the rest of the body, neurogenesis is influenced by aging through a loss of homeostasis in stem cells, including telomere shortening, DNA damage, and cell cycle interruptions, to name a few pathways (4, 12). Neurogenesis is heavily influenced by both positive and negative factors, including epigenetic components of hippocampal neurogenesis that also need to be considered (13). Positive factors may include exercise and environmental enrichment (mating, diverse foods) while negative factors may be generalized as acute and chronic stress (14–19). Hippocampal neurogenesis can be enhanced by hormones, growth factors, drugs, physical exercise, and neurotransmitters and suppressed by aging, glucocorticoids, and stimuli that activate the pituitary/adrenal axis (1, 20, 21). While many models convey that neurogenesis may be considered a cellreplacement method, newer models show appreciation for the neuroplasticity that ensues from the continuous addition of new neurons, also emphasizing the structural plasticity contributions that result (21). In this review, we examined the effects between neurogenesis and electroconvulsive therapy (ECT).
Methods
An examination of the literature was conducted in search of ECT’s uses and ability to generate neurogenesis. References were obtained from Google Scholar, Academic Search Ultimate, EBSCOhost, APA PsycArticles, and ScienceDirect. References were considered acceptable and reliable for inclusion as they were drawn from reputable databases and include peer-reviewed research articles. Articles' publish dates range from 1985 to 2020. In addition, National Center for Biotechnology Information StatPearls online textbook pages were referenced, copyright 2021. Keywords screened for included: electroconvulsive therapy, ECT, neurogenesis, seizure, depression, hypothalamic-pituitary axis, neuroendocrine disorder. Peer-reviewed articles cited are dated from 1985 to 2020 and include research articles as well as StatPearls online textbooks.
Discussion
The link between neurogenesis and ECT ECT is psychiatry’s oldest behavioral psychiatric treatment, having been around since the 16th century, though its first documented use in a controlled clinical setting was in 1938 for general psychosis (22–25). ECT is a procedure administered with electrodes that are placed on the head to stimulate a specific portion of the brain using electric current applied through the electrodes via sine-wave current which has alternating frequencies (23, 24). The first medically documented use of ECT for a specific psychiatric illness was in 1941 to treat schizophrenia, depression, and seizures along with the use for general psychosis (23, 25). Today, ECT is administered to over 1,000,000 patients around the world each year for schizophrenia, depression, seizure disorders, and other psychotic symptoms (23). The use of ECT is not a widely adopted approach due to concerns of the possible side effects of memory impairment and possible brain damage (26). These concerns are noted due to mixed results from ECT experiments in rodent models that were conducted without the proper safeguards currently upheld today on human subjects (26). The concerns regarding memory impairment and the potential for brain damage are negated by the American Psychiatric Association (APA) 2001 guidelines along with the Royal College of Psychiatrists’ guidance for the use of a brief pulse-wave current instead of a continuous current (23). This brief pulse stops the damaging potential that is associated with the overproduction of glutamate caused by prolonged applications of constant electric current (22, 23). How ECT helps with the management of psychiatric diseases is suggested to be the mechanism of neurogenesis within the dentate gyrus of the hippocampus and the SVZ, as well as an increase in neuroplasticity (25, 27–35). Many mental health disorders are noted to exhibit decreased volume of the hippocampus and the SVZ, indicating there is impaired neurogenesis in these two regions (28, 31, 32, 35–38). ECT can modify monoamine transporters, promote increased neurogenesis, increase neuroplasticity, and aid in regulating the hypothalamic-pituitary-adrenal (HPA) axis (34). Neuroplasticity and neurogenesis were shown to be positively correlated in many scientific experiments and reviews suggesting some common factors that ECT may modulate neuroendocrine responses including, angiogenesis, epigenetics, ATP release, immune response, increased number of immature neurons, and many other factors (27–30, 39). A large focus has been put on the modulating effects of ECT on neuroendocrine responses, as they have been shown to increase efficacy of medication and reduce need for medication in patients suffering from epilepsy and depression. (27, 28, 31, 33, 40–42).
How ECT can cause neurogenesis to treat seizures For the past decade, ECT has been looked at as a potential treatment for reducing the effects of epilepsy (43–47). Long exposure epilepsy is shown to disrupt hippocampal granule cells and their production. Initially, there is an increase in the rate of granule cell neurogenesis of the hippocampus in the early stages of epilepsy development (43, 44). The onset of chronic seizures is thought to be caused by too little neurogenesis resulting in prolonged recurring seizures (43, 44). The contribution of ECT to amplifying neurogenesis is the targeted factor that is deemed critical for the reduction in the severity and duration of chronic seizures. The area of the brain that is of neurogenesis interest is the hippocampal dentate granule cells, as this area is seen to be diminished in epilepsy (45). This, in return, strongly affects stem cell-associated plasticity in the dentate gyrus (46). The importance of dentate granule cells is their involvement in the regulation of relayed information to the hippocampus (47). Chronic temporal lobe epilepsy is associated with neurodegeneration and inhibition in the hippocampal regions (44). As observed in animal models and rodents, an initial response to the development of epilepsy is an increase in neurogenesis, but because of long chronic exposures, a decrease in neurogenesis is observed (48, 49). Furthermore, persistent chronic seizures were shown to deplete progenitor cells, leading to reduced neurogenesis (48). As ECT is shown to induce a brief seizure, it demonstrated a correlation between how short, induced seizures may increase neurogenesis and ultimately help treat epilepsy (50, 51). The mechanism of action for ECT is still not yet understood, but according to a systematic review and meta-analysis, animal models appeared to demonstrate that ECT induces neuroplasticity, which ultimately increases the hippocampal volume (52). To increase the volume and neurogenesis of the hippocampal region as well as producing an anticonvulsant effect, ECT was administered to bypass the need for any medications (53, 54). As seen in many patients, chronic seizures and depression may sometimes be triggering risk factors for one another (55). As seen in specific cases, such as treatment-resistant depression and epilepsy, the reduction of these occurrence frequencies may be tackled by the utilization of ECT as it is seen to help increase the neurogenesis in many hippocampal subfields and the amygdala (55, 56). These areas are correlated with the occurrence of depression and anxiety (55). Some of the anticonvulsant effects in the improvement of these regions include decreasing seizure duration, increasing the seizure threshold, and decreasing spontaneous seizure frequencies after multiple ECT sessions (53, 54, 57–59). In some cases, ECT was used to reduce the frequency of seizures in patients with seizure disorders who did not optimally respond to antiepileptic drugs (58). ECT as a form of therapy may be favorable for those who are in the refractory status epilepticus (SE). Refractory SE patients are shown to be partially resistant to treatment medications for epilepsy and therefore need an
alternative form of treatment. SE patients experience inordinate recurring and prolonged seizures that affect their quality of life. Although there is a lack of clinical studies on ECT for refractory SE patients, there were 11 SE patients who reported being treated with ECT. These patients were assessed for their efficacy and were shown to succeed in decreasing epileptic episodes and, in some cases, a cessation in seizures (59). Long exposure high-intensity ECT provided temporary cessation of refractory SE for a few months from the point of the last ECT session (60). The main take from these findings is that ECT treatment has been shown to alter the neurogenesis frequency in the hippocampal regions (61). Graph theory is a quantitative analysis of complex networks to study the brain network organization, represented in a graph-like manner (61, 62). This theory in hand with the utilization of functional and structural MRI can be used to observe the neuroimaging of ECT test subjects to confirm the pre-ETC and post-ETC networking differences. To obtain a vast beneficial database on treating epilepsy with ECT, there needs to be more targeted clinical studies addressing its potential therapeutic improvements and effects.
Figure 1. This figure illustrates a very limited set of examples of interaction within the thalamus that influence downstream effects via the hypophyseal portal system.
Neurogenesis generated by ECT in the treatment of depression ECT has been a major topic of interest in the therapeutic treatment of depression. The key issue is to what degree structural changes can be viewed as trait-dependent, indicative of susceptibility to depression, or state-dependent, and therefore an important therapeutic target. Preclinical experiments have demonstrated that the initiation of pathways contributing to improved hippocampal plasticity is part of the anti-depressive therapeutic mechanism of action, indicating a potential state-dependent structural-level counteracting mechanism (63). A further area of interest is the placement of the electrodes (25) and the possible combination of pharmacotherapy in neurogenesis (25, 64). High-dose right unilateral (RUL) ECT was found to have higher efficacy than bilateral ECT when above the threshold level (65, 66). Regarding the duration of a pulse, ultra-brief RUL ECT had a greater efficacy on remission in those who experience psychotic features (67). ECT has been found to be a more effective measure over pharmacological interventions (68), because it acts directly on the central nervous system (69). ECT is usually not used as the first line of treatment, but one study suggests that if ECT was used earlier in those with long-term chronic depression, there could be an increase in remission (70). Despite these suggestions, a randomized controlled trial study found no significant difference between ECT and pharmacotherapy (71). Late-life depression therapies have a different effect because of the biological changes associated with aging (72). ECT may be superior to pharmacological interventions for depression in the elderly, given that drugs experience decreased absorption, increased amount of distribution, decreased metabolism, and diminished excretion with aging (72). Also, patients can undergo age-related increases in drug susceptibility in later life. Elderly patients may have pharmacodynamic modifications that render them more sensitive to anticholinergic and noradrenergic side effects owing to age-related receptor vulnerability and age-related alterations in cholinergic and monoaminergic neurotransmission (72). However, such pharmacotherapies have been shown to act on amplifying neural progenitors (25) and provide protection against volume reductions caused by diseases (69). Historically there has been a lack of evidence that ECT may produce higher rates of remission than drug therapy (73, 74). More recently, several studies provide evidence suggesting an increase in the volume of gray matter with ECT among elderly patients and did not cause a significant difference in rates of remission (74, 75). Contrary to this, one study of elderly patients found no difference in brain volume between those treated with RUL and those treated with bitemporal ECT (76). Though high-dosage RUL has been found to be just as effective as bilateral ECT and is associated with a decrease in long-term amnesia (73), additional analysis is merited to define appropriate formula-based dosages for RUL ECT in elderly patients (77). While most studies have found a correlation between neurogenesis and remission of depressive episodes (69, 78, 79), there is a strong indication of relapse after 6 months (63, 76, 80). A better understanding of how ECT can achieve neurogenesis to treat depression may lie in optimized neuroplasticity in the HPA axis (81).
ECT and neuroendocrine responses ECT is associated with the activation of neurons and proliferation of endothelial cells (82) in three mid-hypothalamic nuclei: the paraventricular nucleus (PVN), supraoptic nucleus (SON), and ventromedial nucleus (VMN) (83). The PVN controls autonomic function via numerous inputs and projections (84), and is influenced by oxytocin, vasopressin, and corticotropinreleasing hormone (CRH) (84). The SON synthesizes oxytocin and vasopressin and communicates with the medial preoptic nucleus of the thalamus (85). The SON also communicates with the PVN (86). Both the PVN and the VMN are responsive to oxytocin and orexin (87–89). The VMN generates aggression (90), regulates satiety (91), and mediates sexual behavior (92), and secretes neuropeptide Y (93), which is known to activate CRH neurons in the PVN (94). Given the extensive crosstalk between these three nuclei, their communication is essential in coordinating a joint output signal. This signal is relayed via "hypophyseal portal vessels'' to the anterior pituitary (95), which releases adrenocorticotropic hormone, growth hormone (GH), prolactin, thyroid-stimulating hormone, folliclestimulating hormone, and luteinizing hormone into circulation (96) (Figure 1). These hormones are indicators of anterior pituitary and hypothalamic function and have been used to study the endocrine consequences of ECT-mediated mid-hypothalamic cell proliferation. Prolactin and GH levels increase with repeated ECT (97). ECT recipients given naloxone, a putative opioid receptor antagonist (98), had no significantly different response in prolactin or GH, suggesting an opiate-independent mechanism for ECT-stimulated hormone release (97). Thyroid hormone, known to improve mood, memory, and executive function (99), may be decreased with ECT, as low thyroid hormone patients have poor recall accuracy after ECT, while ECT patients receiving adjunctive triiodothyronine have statistically significant improvements to memory (100). These endocrine responses to ECT may be partly explained by neuronal activation and epithelial proliferation in the PVN, SON, and VMN. However, neurological effects of ECT — for instance, activation of the mesocorticolimbic dopamine system and frontotemporal glutamate-GABA processes (101) — may also contribute to ECT response. Characterization of ECT’s neuroendocrine effects requires further research into both neurological and endocrine impacts of the treatment (102). More complete knowledge of these mechanisms may in turn improve how ECT is used to treat seizure patients, depressed patients, and other diseases in the future.
Conclusion
ECT has been used since 1941 to treat depression, seizures, and many other psychiatric symptoms, and the treatment has improved with time. As ECT has been explored, it was discovered that neurogenesis was repeatedly linked to decreases in symptomologies of many psychiatric illnesses. Neurogenesis is a prolific area of clinical neuroscience that applies to current and future studies of many psychiatric pathologies. A key area of ECT research is neuronal activation and endothelial proliferation in the mid-hypothalamic nuclei. Future research could characterize the implications of these mid-hypothalamic changes for endocrine balance. Additionally, interactions between the mid-hypothalamic changes and other neuro-anatomical changes after ECT should be considered to develop a more complete model of ECT’s therapeutic action in treating depression and epilepsy. Due to the propensity of ECT to circumvent pharmacodynamics, especially in older patients, future clinical interventions using ECT should be explored as the first line of treatment. In efforts to improve ECTgenerated remission, studies could investigate how to sustain neurogenesis. This sustained neurogenesis may lead to new insights into minimizing relapse.
Disclosures
The authors have nothing to disclose.
References
1. Götz M, Huttner WB. The cell biology of neurogenesis.
Nat Rev Mol Cell Biol. 2005;6(10):777–88. 2. Zhao C, Deng W, Gage FH. Mechanisms and functional implications of adult neurogenesis. Cell. 2008;132(4):645–60. 3. Sahay A, Hen R. Adult hippocampal neurogenesis in depression. Nat Neurosci. 2007;10(9):1110–5. 4. Eisch AJ, Petrik D. Depression and hippocampal neurogenesis: A road to remission? Science. 2012;338(6103):72–5. 5. Wang H, Warner-Schmidt J, Varela S, Enikolopov
G, Greengard P, Flajolet M. Norbin ablation results in defective adult hippocampal neurogenesis and depressive-like behavior in mice. Proc Natl Acad Sci. 2015;112(31):9745–50. 6. van Wijngaarden P, Franklin RJM. Ageing stem and progenitor cells: Implications for rejuvenation of the central nervous system. Development. 2013;140(12):2562–75. 7. Berg DA, Belnoue L, Song H, Simon A. Neurotransmittermediated control of neurogenesis in the adult vertebrate brain. Development. 2013;140(12):2548–61. 8. Duman RS, Aghajanian GK. Synaptic dysfunction in depression: Potential therapeutic targets. Science. 2012;338(6103):68–72. 9. Obernier K, Alvarez-Buylla A. Neural stem cells: Origin, heterogeneity and regulation in the adult mammalian brain.
Development. 2019;146(4):dev156059. 10. Jacobs B. Adult brain neurogenesis and depression.
Brain Behav Immun. 2002;16(5):602–9. 11. Seib DRM, Martin-Villalba A. Neurogenesis in the normal ageing hippocampus: A mini-review. Gerontology. 2015;61(4):327–35. 12. Drapeau E, Nora Abrous D. Stem cell review series: Role of neurogenesis in age-related memory disorders. Aging Cell. 2008;7(4):569–89. 13. Kumar A, Pareek V, Faiq MA, Ghosh SK, Kumari C. Adult neurogenesis in humans: A review of basic concepts, history, current research, and clinical implications. Innov
Clin Neurosci. 2019;16(5-6):30–7.
14. Tang SW, Helmeste D, Leonard B. Is neurogenesis relevant in depression and in the mechanism of antidepressant drug action? A critical review. World J Biol Psychiatry. 2012;13(6):402–12. 15. Akers KG, Chérasse Y, Fujita Y, Srinivasan S, Sakurai T, Sakaguchi M. Concise review: Regulatory influence of sleep and epigenetics on adult hippocampal neurogenesis and cognitive and emotional function: Sleep, epigenetics, and adult neurogenesis. Stem Cells. 2018;36:969–76. 16. Christian KM, Song H, Ming G. Functions and dysfunctions of adult hippocampal neurogenesis. Annu Rev Neurosci. 2014;37(1):243–62. 17. Lieberwirth C, Pan Y, Liu Y, Zhang Z, Wang Z. Hippocampal adult neurogenesis: Its regulation and potential role in spatial learning and memory. Brain Res. 2016;1644:127–40. 18. Spurny B, Seiger R, Moser P, Vanicek T, Reed MB, Heckova
E, et al. Hippocampal GABA levels correlate with retrieval performance in an associative learning paradigm.
Neuroimage. 2020;204(116244):116244. 19. Elder GA, De Gasperi R, Gama Sosa MA. Research update: Neurogenesis in adult brain and neuropsychiatric disorders. Mt Sinai J Med. 2006;73(7):931–40. 20. Drew M, Hen R. Adult hippocampal neurogenesis as target for the treatment of depression. CNS Neurol Disord Drug
Targets. 2007;6(3):205–18. 21. Bergmann O, Spalding KL, Frisén J. Adult neurogenesis in humans. Cold Spring Harb Perspect Biol. 2015;7(7):a018994. 22. Sienaert P. What we have learned about electroconvulsive therapy and its relevance for the practising psychiatrist.
Can J Psychiatry. 2011;56(1):5–12. 23. Leiknes KA, Schweder LJ, Høie B. Contemporary use and practice of electroconvulsive therapy worldwide. Brain
Behav. 2012;2(3):283–344. 24. Koga K, Furue H, Rashid MH, Takaki A, Katafuchi T, Yoshimura M. Selective activation of primary afferent fibers evaluated by sine-wave electrical stimulation. Mol Pain. 2005;1:13. 25. Rotheneichner P, Lange S, O’Sullivan A, Marschallinger
J, Zaunmair P, Geretsegger C, et al. Hippocampal neurogenesis and antidepressive therapy: Shocking relations. Neural Plast. 2014;2014:723915. 26. Dwork AJ, Christensen JR, Larsen KB, Scalia J,
Underwood MD, Arango V, et al. Unaltered neuronal and glial counts in animal models of magnetic seizure therapy and electroconvulsive therapy. Neuroscience. 2009;164(4):1557–64. 27. Nuninga JO, Mandl RCW, Froeling M, Siero JCW, Somers
M, Boks MP, et al. Vasogenic edema versus neuroplasticity as neural correlates of hippocampal volume increase following electroconvulsive therapy. Brain Stimulat. 2020;13(4):1080–6. 28. Giacobbe J, Pariante CM, Borsini A. The innate immune system and neurogenesis as modulating mechanisms of electroconvulsive therapy in pre-clinical studies.
J Psychopharmacol. 2020;34(10):1086–97. 29. Ueno M, Sugimoto M, Ohtsubo K, Sakai N, Endo A, Shikano
K, et al. The effect of electroconvulsive seizure on survival, neuronal differentiation, and expression of the maturation marker in the adult mouse hippocampus. J Neurochem. 2019;149(4):488–98. 30. Ma DK, Marchetto MC, Guo JU, Ming G, Gage FH, Song
H. Epigenetic choreographers of neurogenesis in the adult mammalian brain. Nat Neurosci. 2010;13(11):1338–44. 31. Perera TD, Dwork AJ, Keegan KA, Thirumangalakudi
L, Lipira CM, Joyce N, et al. Necessity of hippocampal neurogenesis for the therapeutic action of antidepressants in adult nonhuman primates. PLoS One. 2011;6(4):e17600. 32. Malberg JE. Implications of adult hippocampal neurogenesis in antidepressant action. J Psychiatry
Neurosci. 2004;29(3):196–205. 33. Jun H, Mohammed Qasim Hussaini S, Rigby MJ, Jang M-H.
Functional role of adult hippocampal neurogenesis as a therapeutic strategy for mental disorders. Neural Plast. 2012;2012:854285. 34. Xiao W, Zhan Q, Ye F, Tang X, Li J, Dong H, et al. Elevated serum vascular endothelial growth factor in treatmentresistant schizophrenia treated with electroconvulsive therapy: Positive association with therapeutic effects.
World J Biol Psychiatry. 2019;20(2):150–8. 35. Wang J, Wei Q, Bai T, Zhou X, Sun H, Becker B, et al. Electroconvulsive therapy selectively enhanced feedforward connectivity from fusiform face area to amygdala in major depressive disorder. Soc Cogn Affect
Neurosci. 2017;12(12):1983–92. 36. Kranaster L, Blennow K, Zetterberg H, Sartorius A.
Electroconvulsive therapy does not alter the synaptic protein neurogranin in the cerebrospinal fluid of patients with major depression. J Neural Transm. 2017;124(12):1641–5. 37. Svensson M, Grahm M, Ekstrand J, Höglund P, Johansson
M, Tingström A. Effect of electroconvulsive seizures on cognitive flexibility: ECS and cognitive flexibility.
Hippocampus. 2016;26(7):899–910. 38. Singh A, Kar SK. How Electroconvulsive Therapy works?:
Understanding the neurobiological mechanisms. Clin
Psychopharmacol Neurosci. 2017;15(3):210–21. 39. Gosnell SN, Meyer MJ, Jennings C, Ramirez D,
Schmidt J, Oldham J, et al. Hippocampal volume in psychiatric diagnoses: Should psychiatry biomarker research account for comorbidities? Chronic Stress. 2020;4:2470547020906799. 40. Maruyama S, Boku S, Okazaki S, Kikuyama H, Mizoguchi
Y, Monji A, et al. ATP and repetitive electric stimulation increases leukemia inhibitory factor expression in astrocytes: A potential role for astrocytes in the action mechanism of electroconvulsive therapy. Psychiatry Clin
Neurosci. 2020;74(5):311–7.
41. Inta D, Lima-Ojeda JM, Lau T, Tang W, Dormann C,
Sprengel R, et al. Electroconvulsive Therapy induces neurogenesis in frontal rat brain areas. PLoS One. 2013; 8(7):e69869. 42. Becker S, Wojtowicz JM. A model of hippocampal neurogenesis in memory and mood disorders. Trends Cogn
Sci. 2007;11(2):70–6. 43. Cho KO, Lybrand ZR, Ito N, Brulet R, Tafacory F, Zhang L, et. al. Aberrant hippocampal neurogenesis contributes to epilepsy and associated cognitive decline. Nat. Commun. 2015;6(1):6606. 44. Hattiangady B, Shetty AK. Implications of decreased hippocampal neurogenesis in chronic temporal lobe epilepsy. Epilepsia. 2008;49(5):26–41. 45. Hester MS, Danzer SC. Hippocampal granule cell pathology in epilepsy - a possible structural basis for comorbidities of epilepsy? Epilepsy Behav. 2014;38:105–16. 46. Jessberger S, Parent JM. Epilepsy and adult neurogenesis.
Cold Spring Harb Perspect Biol. 2015;7(12):a020677. 47. Danzer SC, Kotloski RJ, Walter C, Hughes M, McNamara
JO. Altered morphology of hippocampal dentate granule cell presynaptic and postsynaptic terminals following conditional deletion of TrkB. Hippocampus. 2008;18(7):668–78. 48. Danzer SC. Neurogenesis in epilepsy: Better to burn out or fade away? Epilepsy Curr. 2016;16(4):268–9. 49. Varma P, Brulet R, Zhang L, Hsieh J. Targeting seizure - induced neurogenesis in a clinically relevant time period leads to transient but not persistent seizure reduction.
J Neurosci. 2019;39(35):7019–28. 50. Salik I, Marwaha R. Electroconvulsive Therapy. StatPearls,
Treasure Island (FL): StatPearls Pub; 2021. 51. Parent JM, Lowenstein DH. Seizure-induced neurogenesis:
Are more new neurons good for an adult brain? Prog Brain
Res. 2002;135:121–31. 52. Wilkinson ST, Sanacora G, Bloch MH. Hippocampal volume changes following electroconvulsive therapy: A systematic review and meta-analysis. Biol Psychiatry Cogn Neurosci
Neuroimaging. 2017;2(4):327–35. 53. Regenold WT, Weintraub D, Taller A. Electroconvulsive therapy for epilepsy and major depression. Am J Geriatr
Psychiatry.1998;6(2):180–3. 54. Lunde ME, Lee EK, Rasmussen KG. Electroconvulsive therapy in patients with epilepsy. Epilepsy Behav. 2006;9(2):355–9. 55. Gryglewski G, Baldinger-Melich P, Seiger R, Godbersen
GM, Michenthaler P, Klöbl M, et. al. Structural changes in amygdala nuclei, hippocampal subfields and cortical thickness following electroconvulsive therapy in treatmentresistant depression: Longitudinal analysis. Br J psychiatry. 2019;214(3):159–67. 56. Abbott CC, Jones T, Lemke NT, Gallegos P, McClintock SM,
Mayer AR, et. al. Hippocampal structural and functional changes associated with electroconvulsive therapy response. Transl Psychiatry. 2014;4(11):e483. 57. Griesemer DA, Kellner CH, Beale MD, Smith GM.
Electroconvulsive therapy for treatment of intractable seizures: Initial findings in two children. Neurology. 1997;49(5):1389–92. 58. Shah N, Pande N, Bhat T, Murke M, Andrade C.
Maintenance ECT as a therapeutic approach to medication-refractory epilepsy in an adult with mental retardation: Case report and review of literature. J ECT. 2012;28(2):136–40. 59. Lambrecq V, Villéga F, Marchal C, Michel V, Guehl D, Rotge
JY, et. al. Refractory status epilepticus: Electroconvulsive therapy as a possible therapeutic strategy. Seizure. 2012;21(9):661–4. 60. Schneegans H, Stetefeld H, Dohmen C, Onur OA,
Lehnhardt FG. Successful treatment of super-refractory status epilepticus with high-intensity electroconvulsive therapy – a case report and review of the current literature.
J. Epilepsy Res. 2019;9(1):76–82. 61. Li Q, Liu S, Guo M, Yang CX, Xu Y. The principles of electroconvulsive therapy based on correlations of schizophrenia and epilepsy: A view from brain networks.
Front Neurol. 2019;10:688. 62. Bullmore E, Sporns O. Complex brain networks: Graph theoretical analysis of structural and functional systems.
Nat Rev Neurosci. 2009;10(3):186–98. 63. Nordanskog P, Larsson MR, Larsson EM, Johanson A.
Hippocampal volume in relation to clinical and cognitive outcome after electroconvulsive therapy in depression.
Acta Psychiatr Scand. 2014;129(4):303–11. 64. Bessa JM, Ferreira D, Melo I, Marques F, Cerqueira
JJ, Palha JA, et. al. The mood-improving actions of antidepressants do not depend on neurogenesis but are associated with neuronal remodeling. Mol Psychiatry. 2009;14(8):764–73. 65. Sackeim HA, Prudic J, Devanand DP, Nobler MS,
Lisanby SH, Peyser S, et. al. A prospective, randomized, double-blind comparison of bilateral and right unilateral electroconvulsive therapy at different stimulus intensities.
Arch Gen Psychiatry. 2000;57(5):425–34. 66. Heikman P, Kalska H, Katila H, Sarna S, Tuunainen
A, Kuoppasalmi K. Right unilateral and bifrontal electroconvulsive therapy in the treatment of depression:
A preliminary study. J ECT. 2002;18(1):26–30. 67. Loo CK, Mahon M, Katalinic N, Lyndon B, Hadzi-Pavlovic
D. Predictors of response to ultrabrief right unilateral electroconvulsive therapy. J Affect Disord. 2011;130(12):192–7. 68. Pagnin D, de Queiroz V, Pini S, Cassano GB. Efficacy of ECT in depression: A meta-analytic review. J ECT. 2008;6(1):155-62. 69. Joshi SH, Espinoza RT, Pirnia T, Shi J, Wang Y, Ayers B, et. al. Structural plasticity of the hippocampus and amygdala induced by electroconvulsive therapy in major depression.
Biol Psychiatry. 2016;79(4):282–92.
70. Dombrovski AY, Mulsant BH, Haskett RF, Prudic J,
Begley AE, Sackeim HA. Predictors of remission after electroconvulsive therapy in unipolar major depression.
J Clin Psychiatry. 2005;66(8):1043–9. 71. Kellner CH, Knapp RG, Petrides G, Rummans TA, Husain
MM, Rasmussen K, et. al. Continuation electroconvulsive therapy vs pharmacotherapy for relapse prevention in major depression: A multisite study from the consortium for research in electroconvulsive therapy (CORE). Arch Gen
Psychiatry. 2006;63(12):1337-44. 72. Stanton L, Kohn R. Depression and the aging brain. Med
Health R I. 2012;95(7):210–1 73. Sackeim HA. Electroconvulsive therapy in late-life depression. Clinical geriatric psychopharmacology. 4th ed.
Baltimore: Lippincott Williams & Wilkins. 2004;385 p. 74. Spaans HP, Sienaert P, Bouckaert F, van den Berg JF,
Verwijk E, Kho KH, et. al. Speed of remission in elderly patients with depression: electroconvulsive therapy v. medication. Br J Psychiatry. 2015;206(1):67–71. 75. Bouckaert F, De Winter FL, Emsell L, Dols A, Rhebergen
D, Wampers M, et. al. Grey matter volume increase following electroconvulsive therapy in patients with late life depression: A longitudinal MRI study. J Psychiatry Neurosci. 2016;41(2):105. 76. Bouckaert F, Dols A, Emsell L, De Winter FL, Vansteelandt
K, Claes L, et. al. Relationship between hippocampal volume, serum BDNF, and depression severity following electroconvulsive therapy in late-life depression.
Neuropsychopharmacology. 2016;41(11):27418. 77. Bjølseth TM, Engedal K, Benth JŠ, Dybedal GS, Gaarden TL, Tanum L. Clinical efficacy of formula-based bifrontal versus right unilateral electroconvulsive therapy (ECT) in the treatment of major depression among elderly patients:
A pragmatic, randomized, assessor-blinded, controlled trial.
J Affect Disord. 2015;175:8–17. 78. Perera TD, Coplan JD, Lisanby SH, Lipira CM, Arif M,
Carpio C, et. al. Antidepressant-induced neurogenesis in the hippocampus of adult nonhuman primates. J Neurosci. 2007;27(18):4894901. 79. Dukart J, Regen F, Kherif F, Colla M, Bajbouj M, Heuser I, et. al. Electroconvulsive therapy induced brain plasticity determines therapeutic outcome in mood disorders. Proc
Natl Acad Sci U S A. 2014;111(3):1156–61. 80. Jelovac A, Kolshus E, McLoughlin DM. Relapse following successful electroconvulsive therapy for major depression: A meta-analysis. Neuropsychopharmacology. 2013;38(12):2467–74. 81. Schloesser RJ, Orvoen S, Jimenez DV, Hardy NF, Maynard
KR, Sukumar M, et. al. Antidepressant-like effects of electroconvulsive seizures require adult neurogenesis in a neuroendocrine model of depression. Brain Stimul. 2015;8(5):862–70. 82. Bolwig, TG et. al. How does electroconvulsive therapy work? Theories on its mechanism. Can J Psychiatry. 2011;56(1):13–8. 83. Jansson L, Wennström M, Johanson A, Tingström A.
Glial cell activation in response to electroconvulsive seizures. Prog Neuropsychopharmacol Biol Psychiatry. 2009;33(7):1119–28. 84. Ferguson AV, Latchford KJ, Samson WK. The paraventricular nucleus of the hypothalamus: A potential target for integrative treatment of autocrine dysfunction.
Expert Opin Ther Targets. 2008;12(6):717–27. 85. Crespo D, Ramos J, Gonzales C, Fernandez-Viadero C.
The supraoptic nucleus: A morphological and quantitative study in control and hypophysectomised rats. J Anat. 1990;169:115–23. 86. Ježová D, Michajlovskij N, Kvetńanský R, Makara GB.
Paraventricular and supraoptic nuclei of the hypothalamus are not equally important for oxytocin release during stress. Neuroendocrinology. 1993;57(5):776–81. 87. Noble EE, Billington CJ, Kotz CM, Wang C. Oxytocin in the ventromedial hypothalamic nucleus reduces feeding and acutely increases energy expenditure. Am J Physiol Regul
Integr Comp Physiol. 2014;307(6):R737–R45. 88. Liu L, Wang Q, Liu A, Lan X, Huang Y, Zhao Z, et. al.
Physiological implications of orexins/hypocretins on energy metabolism and adipose tissue development. ACS Omega. 2020; 5(1):547–55. 89. Yuko M, Shinichi T, Katsuya T, Seiichi T, Yoichi U,
Kenju S. Orexin action on oxytocin neurons in the paraventricular nucleus of the hypothalamus. Neuroreport. 2017;28(6):330–366. 90. Hashikawa Y, Hashikawa K, Falkner AL, Lin D. Ventromedial hypothalamus and the generation of aggression. Front Syst
Neurosci. 2017;11:94. 91. Gaur A, Pal GK, Ananthanarayanan PH, Pal P. Role of ventromedial hypothalamus in high fat diet induced obesity in male rats: Association with lipid profile, thyroid profile and insulin resistance. Ann Neurosci. 2014;21(3):104–7. 92. Nomoto K, Lima SQ. Enhance male-evoked responses in the ventromedial hypothalamus of sexually receptive female mice. Curr Biol. 2015;25(5):589–94. 93. Chee MJ, Myers MG, Price CJ, Colmers WF. Neuropeptide
Y suppresses anorexigenic output from the ventromedial nucleus of the hypothalamus. J Neurosci. 2010;30(9):3380–90. 94. Dimitrov EL, DeJoseph RM, Brownfield MS, Urban
JH. Involvement of neuropeptide Y Y1 receptors in the regulation of neuroendocrine corticotropinreleasing hormone neuronal activity. Endocrinology. 2007;148(8):3666–73. 95. Smith SM, Vale WW. The role of the hypothalamicpituitary-adrenal axis in neuroendocrine responses to stress. Dialogues Clin Neurosci. 2006;8(4):383–95. 96. El Sayed SA, Fahmy MW, Schwarz J. Physiology, pituitary gland. StatPearls, Treasure Island (FL): StatPearls Pub. 2021. 97. Haskett RF, Zis AP, Albala AA. Hormone response to repeated electroconvulsive therapy: Effects of naloxone.
Biol Psychiatry. 1985;20(6):623–33.
98. US Department of Health and Human Services. Naloxone:
Medical countermeasures database [Internet]. Maryland
USA: National Libraries of Medicine; 2014 [updated 2020
Dec 21; cited 2021 Feb 20]. Available from https://chemm. nlm.nih.gov/countermeasure_naloxone.htm. 99. Samuels MH. Psychiatric and cognitive manifestations of hypothyroidism. Curr Opin Endocrinol Diabetes Obes. 2014;21(5):377–83. 100.Stern RA, Tremont G. Minimizing the cognitive effects of lithium therapy and electroconvulsive therapy using thyroid hormone. Int J Neuropsychopharmacol. 2000;3(2):175-86. 101.Read J, Fosse R. Electroconvulsive treatment: Hypotheses about mechanisms of action. Front Psychiatry. 2013;4:94. 102.Yu, J. Endocrine disorders and the neurologic manifestations. Ann Pediatr Endocrinology Metab. 2014;19(4):184–90.