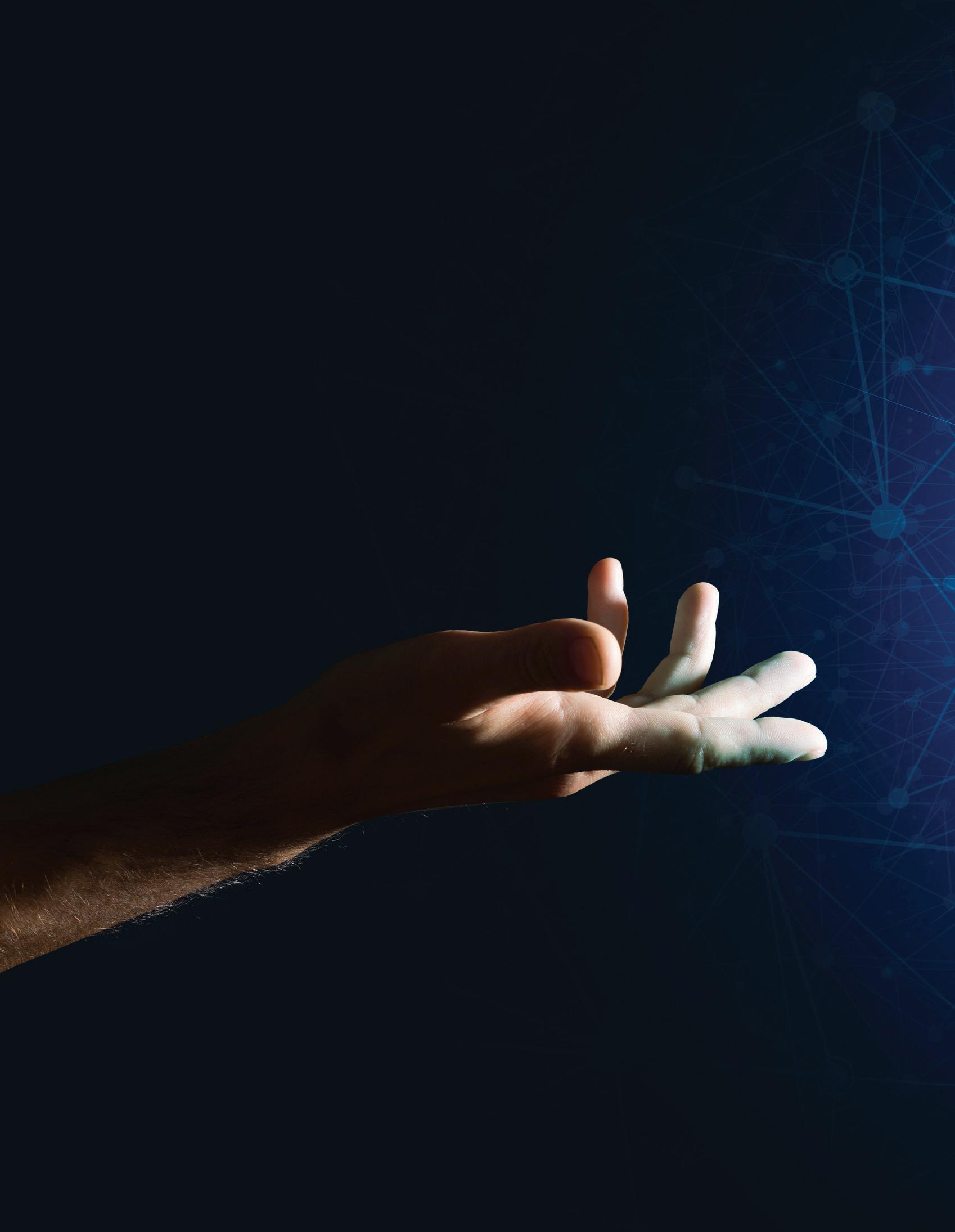
9 minute read
The sPiriT oF survivAl - PArT 2
from "Got Rum?" July 2022
by gotrum
The Spirit of Survival
By Luis Ayala Part 2
This article was originally written for, and published by, Artisan Spirit Magazine.
introduction
in last month’s issue of “got rum?” we explored the origin of yeast and the reasons behind its evolutionary adaptation to produce ethanol (see “The spirit of survival - Part i” on pg. 50). we also reviewed trees’ use of tannins as protection mechanism against predators and we touched on how humans have taken up the roles of both protectors of yeast and trees, and consumers of alcohol and tannins.
in this article we will explore additional mechanisms employed by yeast in order to maximize their probabilities of survival, as well as, the ways in which their competing organisms have adapted.
Weapons of Yeast Destruction
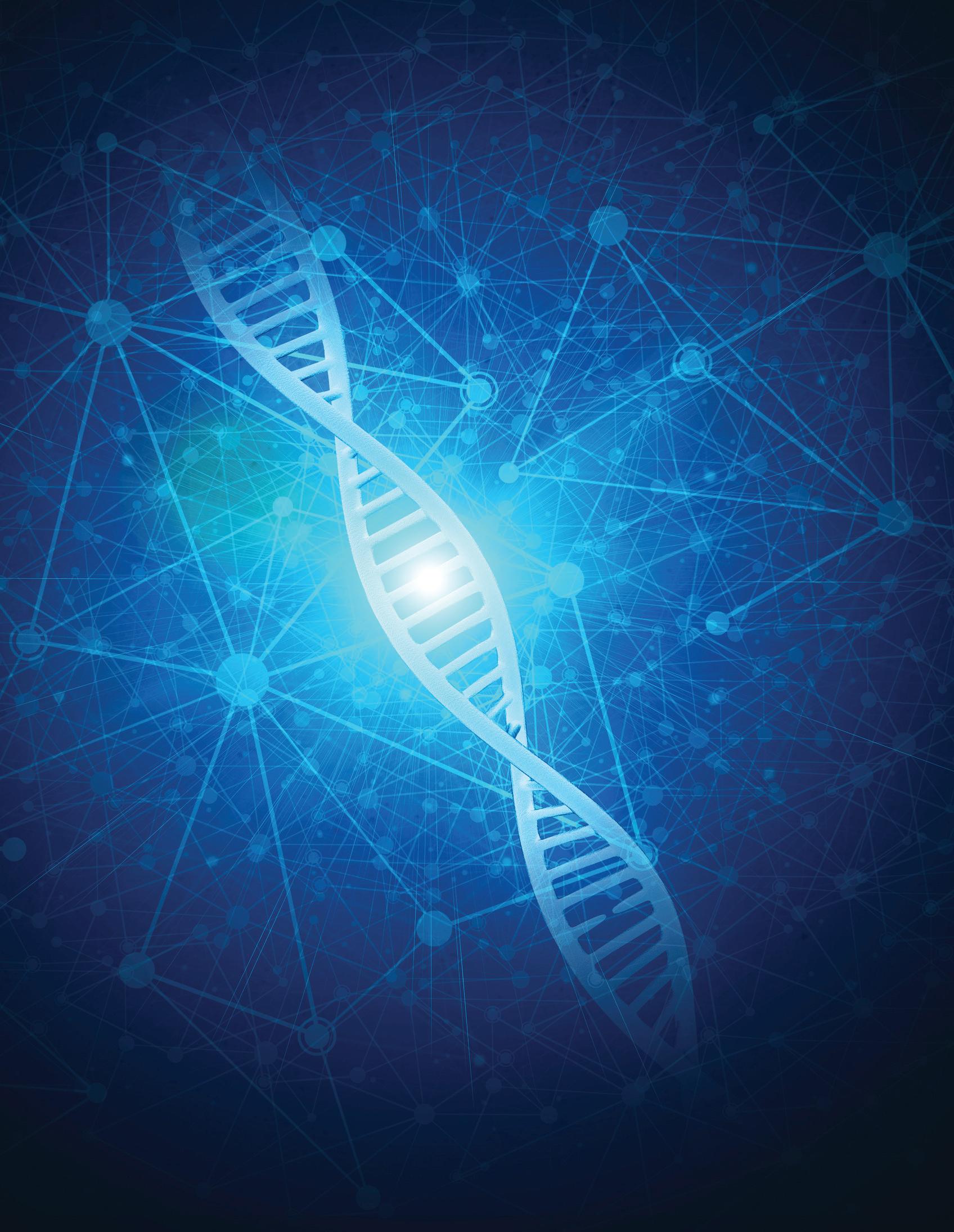
it is easy for us to think that yeast are all members of the same ‘team’ of organisms, all wearing the same proverbial uniform and working in tandem to achieve a collective goal. while this notion embodies aspirational images of collaboration, the reality is that yeast populations are highlycompetitive across strains. when different communities of fermenting yeast produce ethanol, they do so to ‘cleanse’ their environment by killing potential competitors that have not developed a resistance to alcohol. But what happens when the competitor is a different strain of fermenting yeast, one that has developed a resistance to it?
The answer is simple: chemical warfare in the form of killer toxins! The discovery of yeast killer toxins dates back to 1963, when Bevan and Makover(1) described the secretion of molecules by a certain isolate of brewer’s yeast (Saccharomyces cerevisiae) that inhibited growth of other yeast strains. The secretions were subsequently identified as a protein(2) which was named killer factor or killer toxin and the producing strain termed killer yeast. Production of killer toxins has now been identified in over 100 ascomycetous and over 50 basidiomycetous yeast species(3). There are many classes of killer yeast strains differing particularly in the spectrum of their activity against sensitive strains, in their cross reactivity, genetic determination of killer toxin, killer toxin immunity and the mechanisms of killer toxin action(4). Here is a classification of killer yeast according to genetic basis: 1. Cytoplasmically inherited encapsulated double-stranded RNA (dsRNA) viruses 2. II-Linear double strand DNA plasmid 3. Killer phenotype associated with chromosomal genes
While these killer toxins may appear to affect only specific yeast strains, there are numerous potential commercial applications for them in the real world: 1. Food and fermentation industries 2. Killer yeast as potential antimicrobial agents 3. Yeast killer system in bio-typing 4. Killer yeasts in recombinant DNA technology and transgenic plants
As you can imagine by now, we should not expect for these killer toxins and
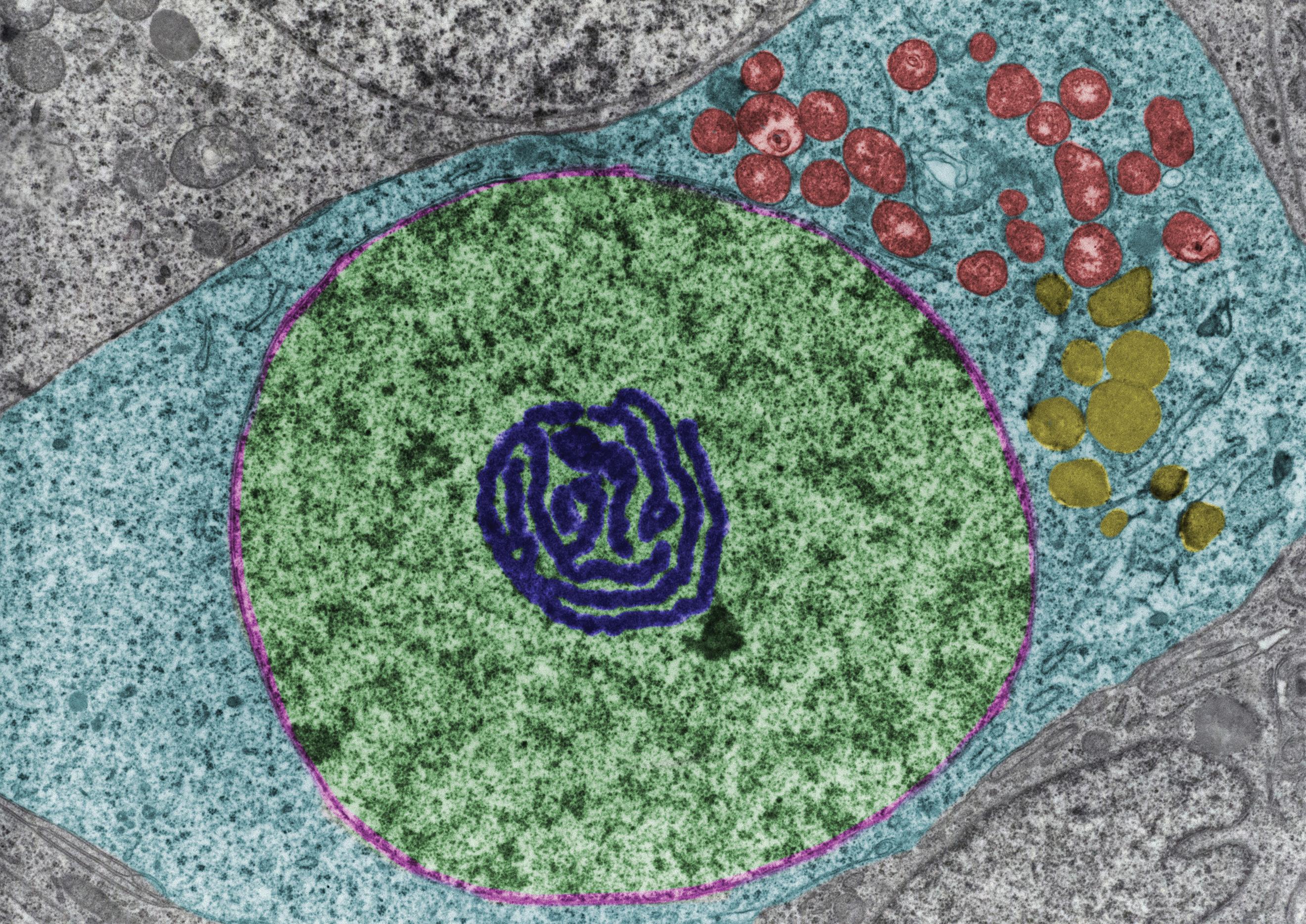
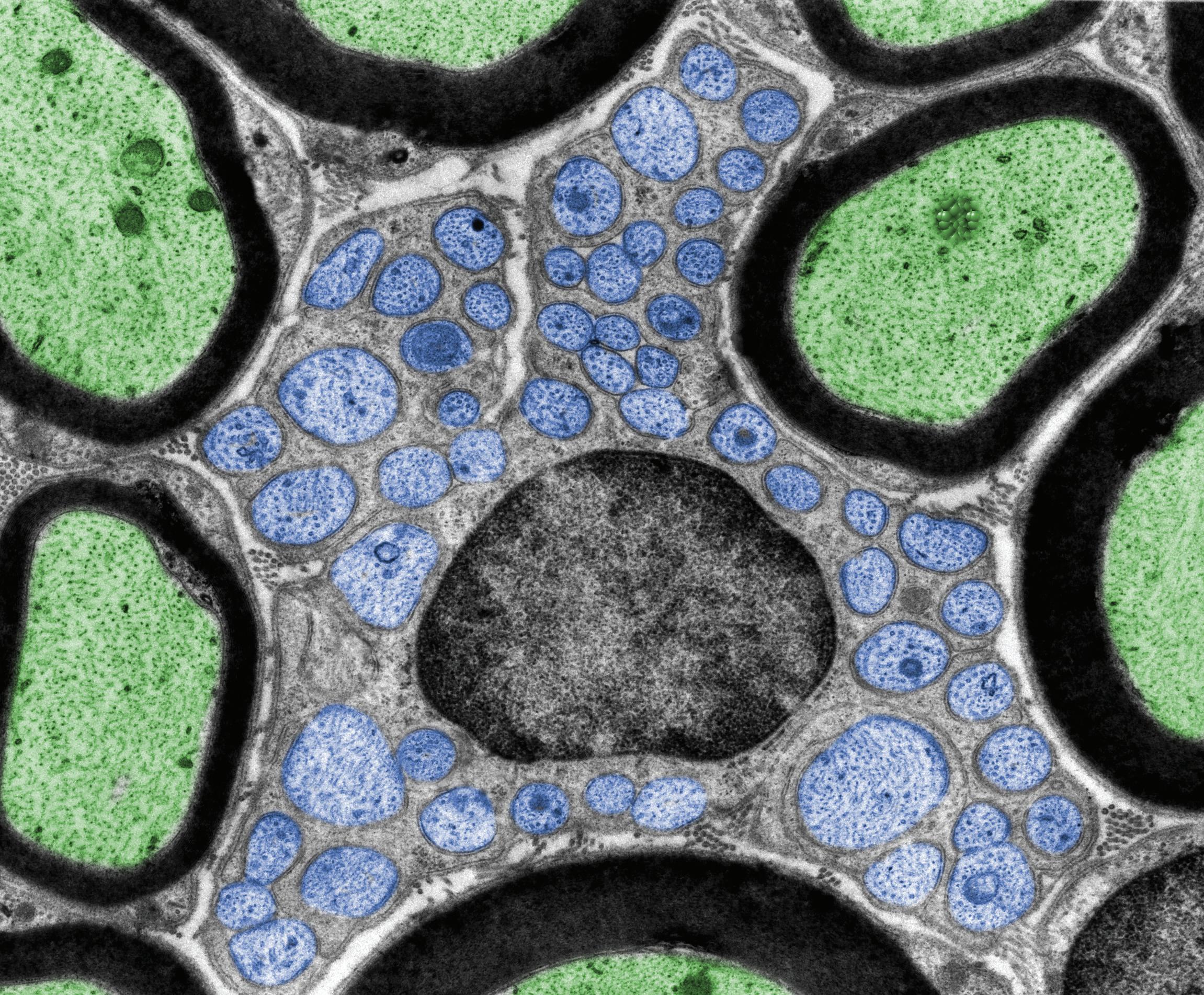
their victims to remain static: history has shown us that it is only a matter of time before organisms develop immunity to the toxins, which then sets off the production of new and improved toxins by future generations of yeast. In other words, for every weapon developed, there is a matching neutralizing defense and for every defense an improved weapon is eventually produced.
Meanwhile in Gotham City . . .
While yeast genera are occupied with production of killer toxins and with the development of chemical resistance to such poisons, other organisms are busy at work evolving ways to consume the ethanol manufactured during fermentation. Of these organisms, acid-producing bacteria are the most notorious, not only because of their abundance in nature, but also because of the key roles they play in humans’ own survival. Here are a few examples.
• Acetic Acid Bacteria (AAB): Acetic
Acid Bacteria are a classification of bacteria which are capable of oxidizing ethanol into acetic acid during fermentation. Currently there are 10 genera in the family Acetobacteraceae,
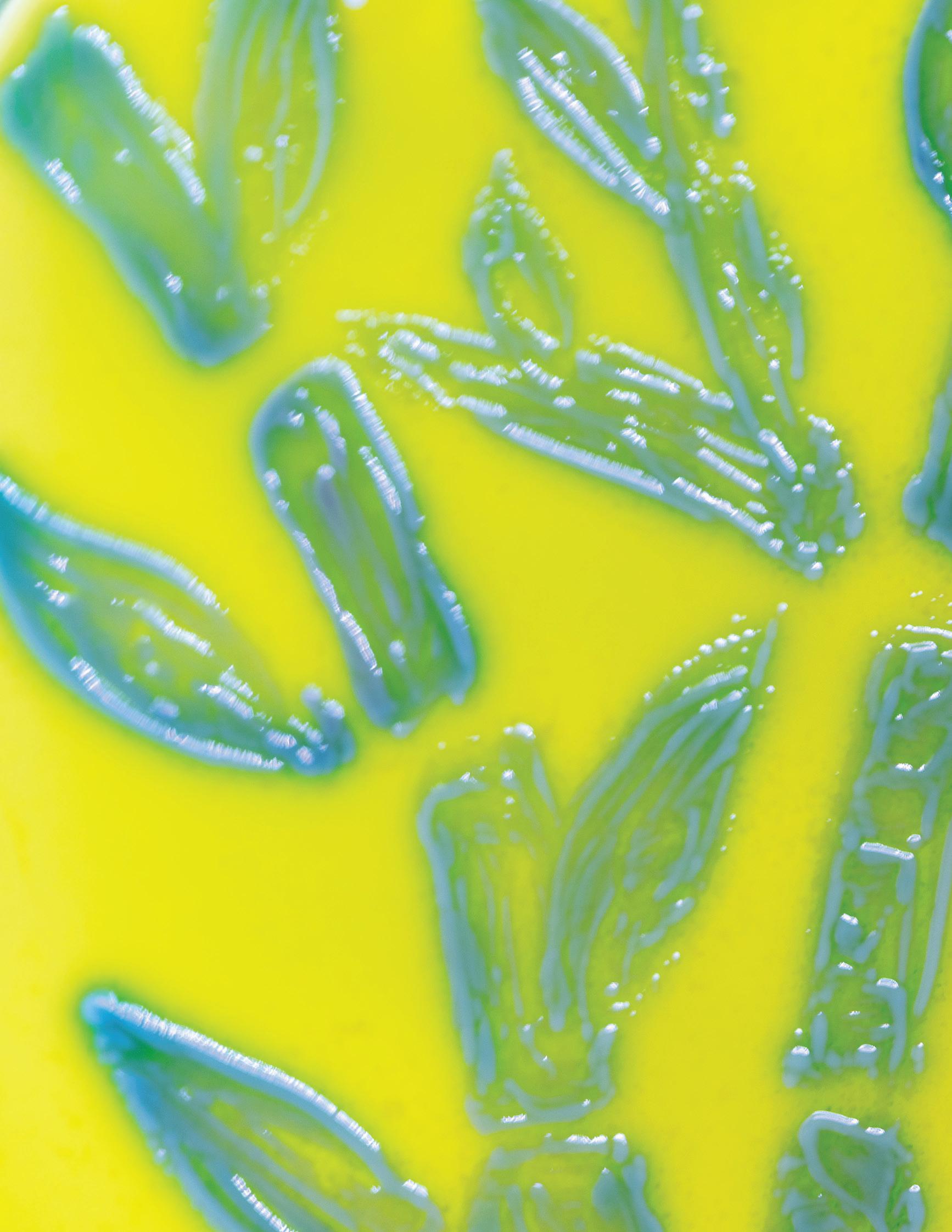
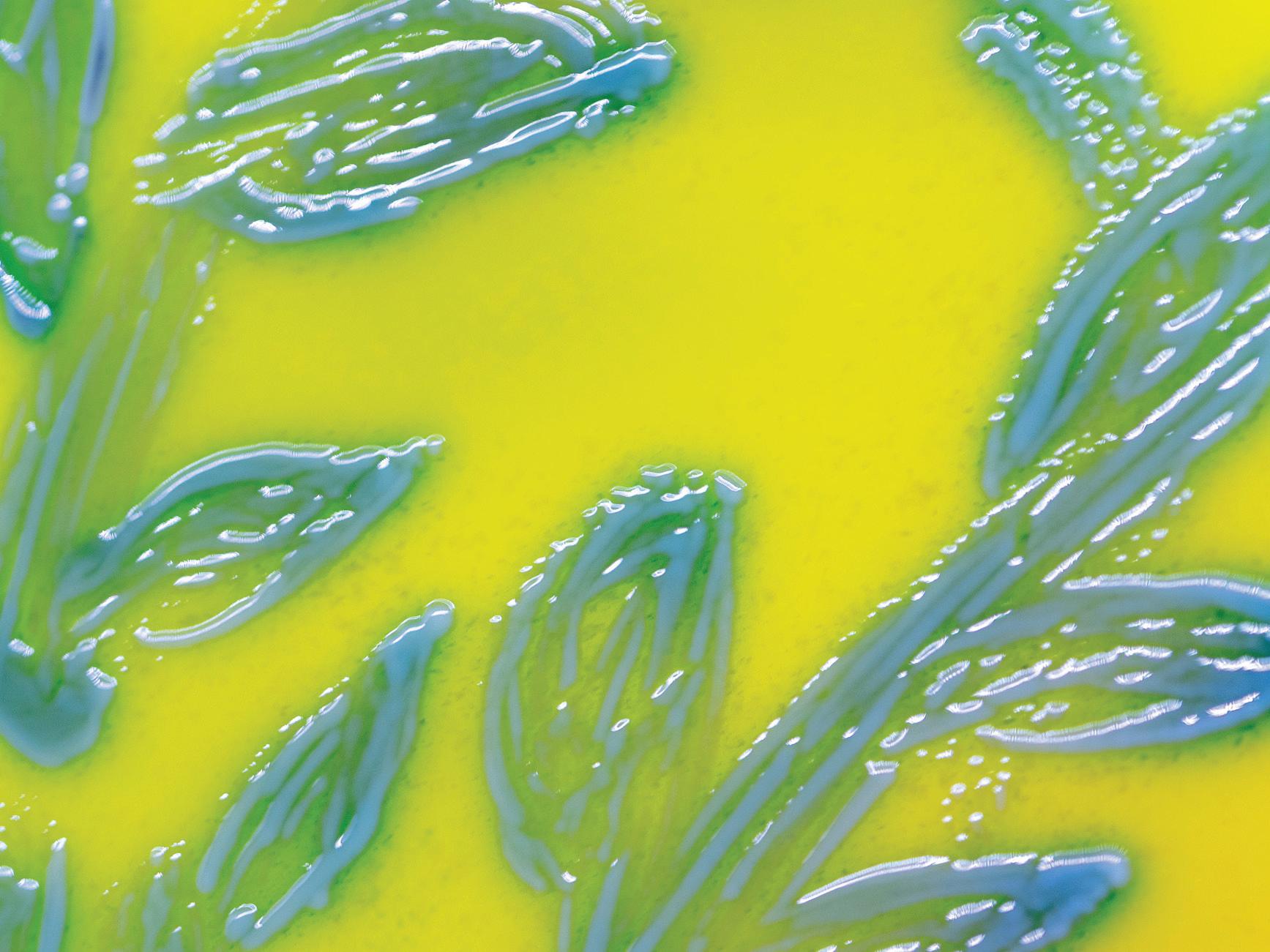
one of these is Acetobacter, which can oxidize ethanol to carbon dioxide and water using Krebs cycle enzymes. One example of Acetobacter is Acetobacter diazotrophicus, which can be isolated from coffee plants or from sugarcane stalks. It is an acidproducing, nitrogen-fixing bacteria. In fact, the A. diazotrophicus -sugarcane relationship, first observed in Brazil, was the first report of a beneficial symbiotic relationship between grasses and bacteria through nitrogen fixation. Nitrogen-fixing bacteria are important in modern agriculture - exploiting these bacteria would decrease the present dependency on nitrogen fertilizers, which would have positive results for the ecosystem and the health of humans and other
animals. Other strains can be found in samples from Japanese rice vinegar (komesu) or unpolished rice vinegar (kurosu)(5). • Lactic Acid Bacteria (LAB): Lactic
Acid Bacteria are bacteria capable of fermenting glucose primarily to lactic acid, or to lactic acid + CO2 + ethanol. All LAB grow anaerobically, but unlike most anaerobes, they grow in the presence of O2 as “aerotolerant anaerobes”. Although they lack catalase, they possess superoxide dismutase and have alternative means to detoxify peroxide radicals, generally through peroxidase enzymes.
Despite the fact that many genera of bacteria produce lactic acid as a primary or secondary end-product of fermentation, the term Lactic Acid
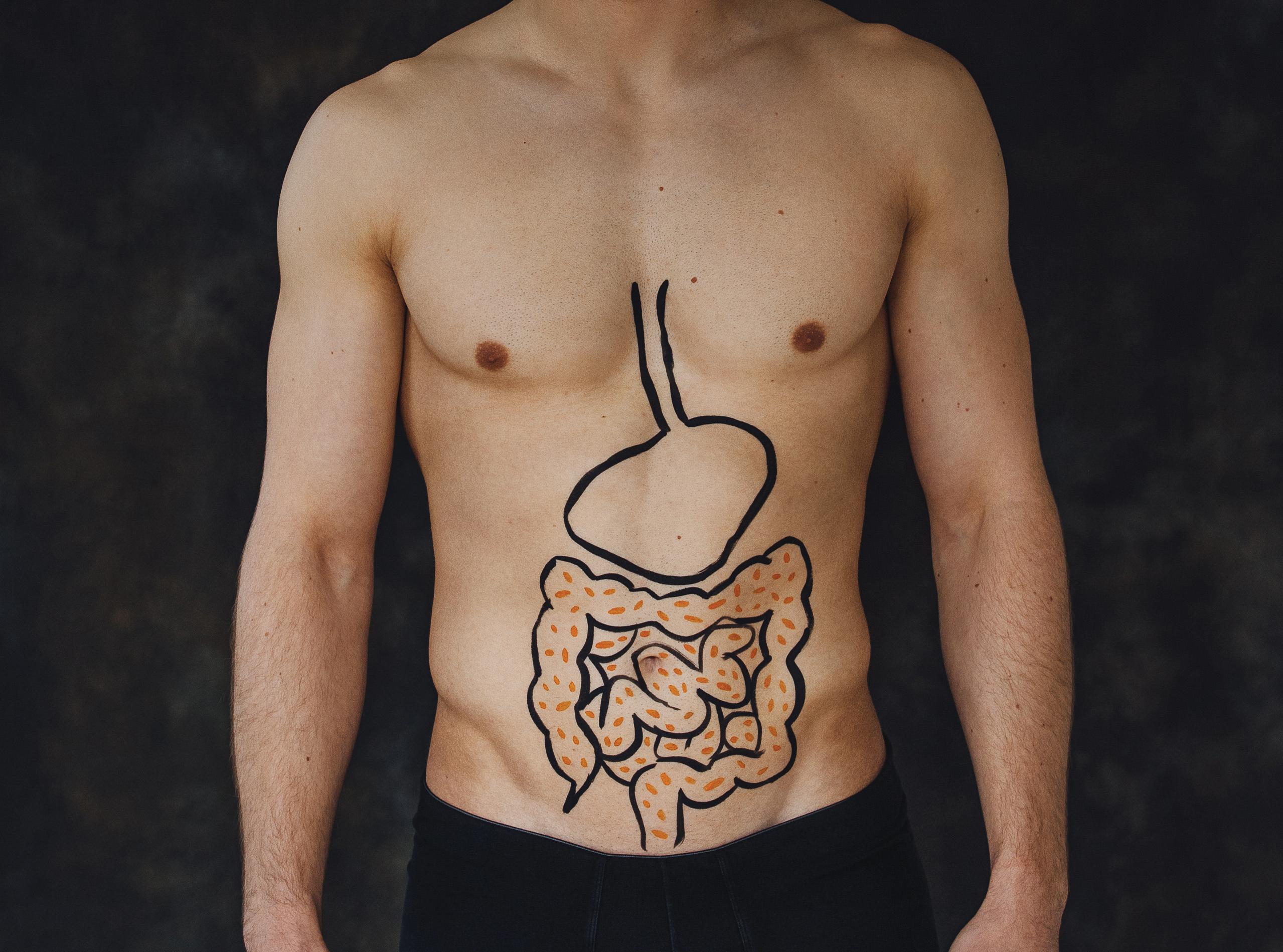
Bacteria is conventionally reserved for genera in the order Lactobacillales, which includes Lactobacillus, Leuconostoc, Pediococcus, Lactococcus and Streptococcus, in addition to Carnobacterium, Enterococcus, Oenococcus, Tetragenococcus, Vagococcus, and Weisella. Because they obtain energy only from the metabolism of sugars, lactic acid bacteria are restricted to environments in which sugars are present. They have limited biosynthetic ability, having evolved in environments that are rich in amino acids, vitamins, purines and pyrimidines, so they must be cultivated in complex media that fulfill all their nutritional requirements. Most LAB are free-living or live in beneficial or harmless associations
with animals, although some are opportunistic pathogens. The human gut contains a highly diverse microbial community that is essentially an open ecosystem, despite being deeply embedded within the human body. Food-associated fermentative bacteria, including probiotics, are major sources of ingested bacteria that may temporarily complement resident microbial communities, thus forming part of our transient microbiome(6). • Ingested bacteria can temporarily complement resident bacterial communities as part of our transient microbiome. • The extent of integration is highly species -and strain- dependent and
may vary depending on dietary context and baseline microbiota structure. • Ingested bacteria can cause major shifts in the composition of the microbiome of the small intestine, whereas alterations in the colon are mostly of limited extent. • Clinical data have provided evidence that ingested bacteria may stimulate production of short-chain fatty acids and inhibit some opportunistic pathogens. Our dependence on acid-producing bacteria to ferment our foods (cheese, sauerkraut, kimchi, etc.) has resulted in our preservation and propagation of desired strains. But the same strains can also wreak havoc when they appear in operations where the acidity is seen as an undesirable trait or where its production compromises yields, like in the alcohol production industry.
Penicillin: Is What’s For Lunch!
When it was discovery in 1928 by Dr. Alexander Fleming, penicillin marked a turning point in human history: for the first time ever, doctors had a tool with which they could cure patients infected by a plethora of deadly infectious diseases. Not too long after its revolutionary introduction into the medical field, microbiologists and scientists found uses for penicillin outside hospitals as well, including its use as an inoculant in fermentation tanks at ethanol plants.
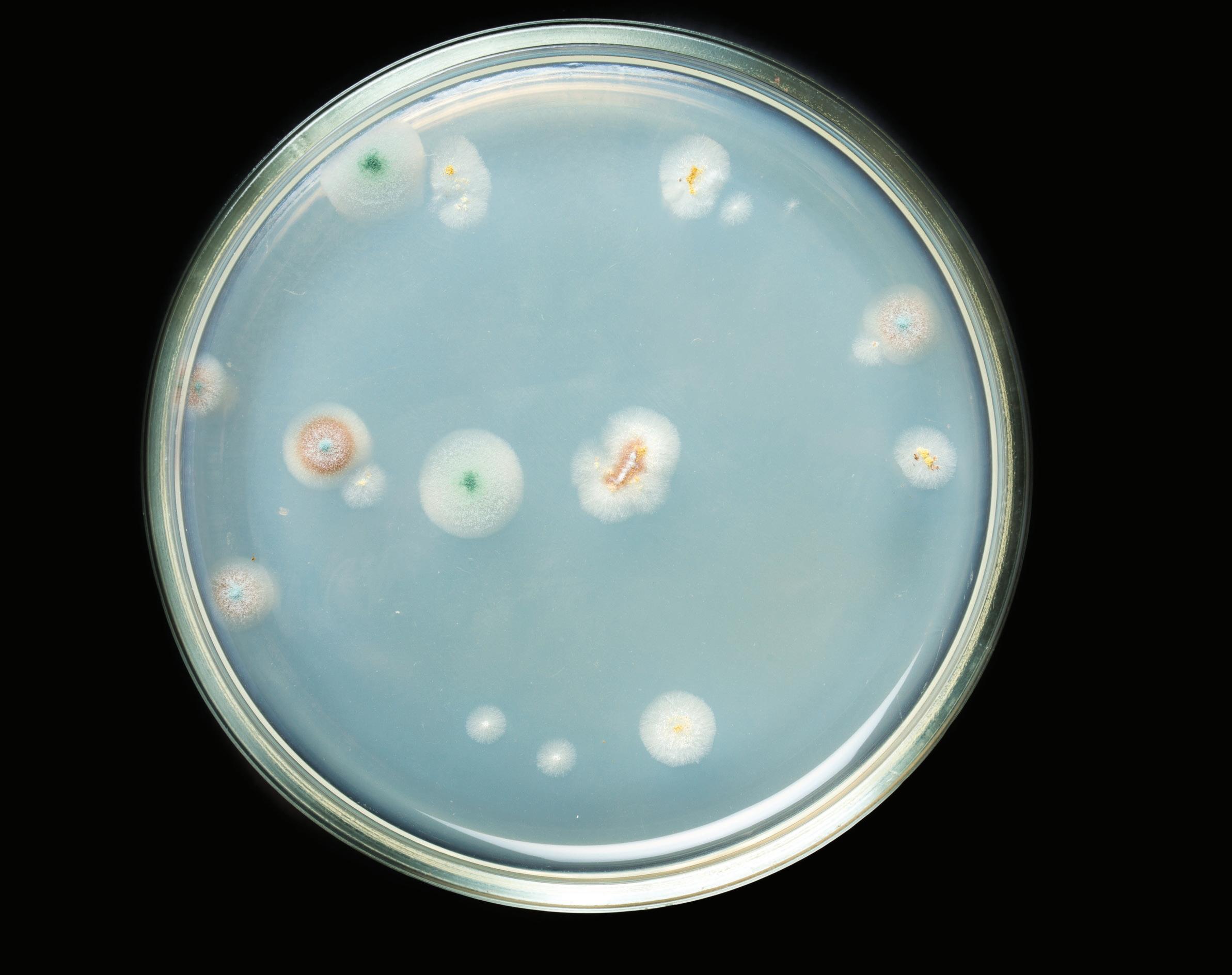
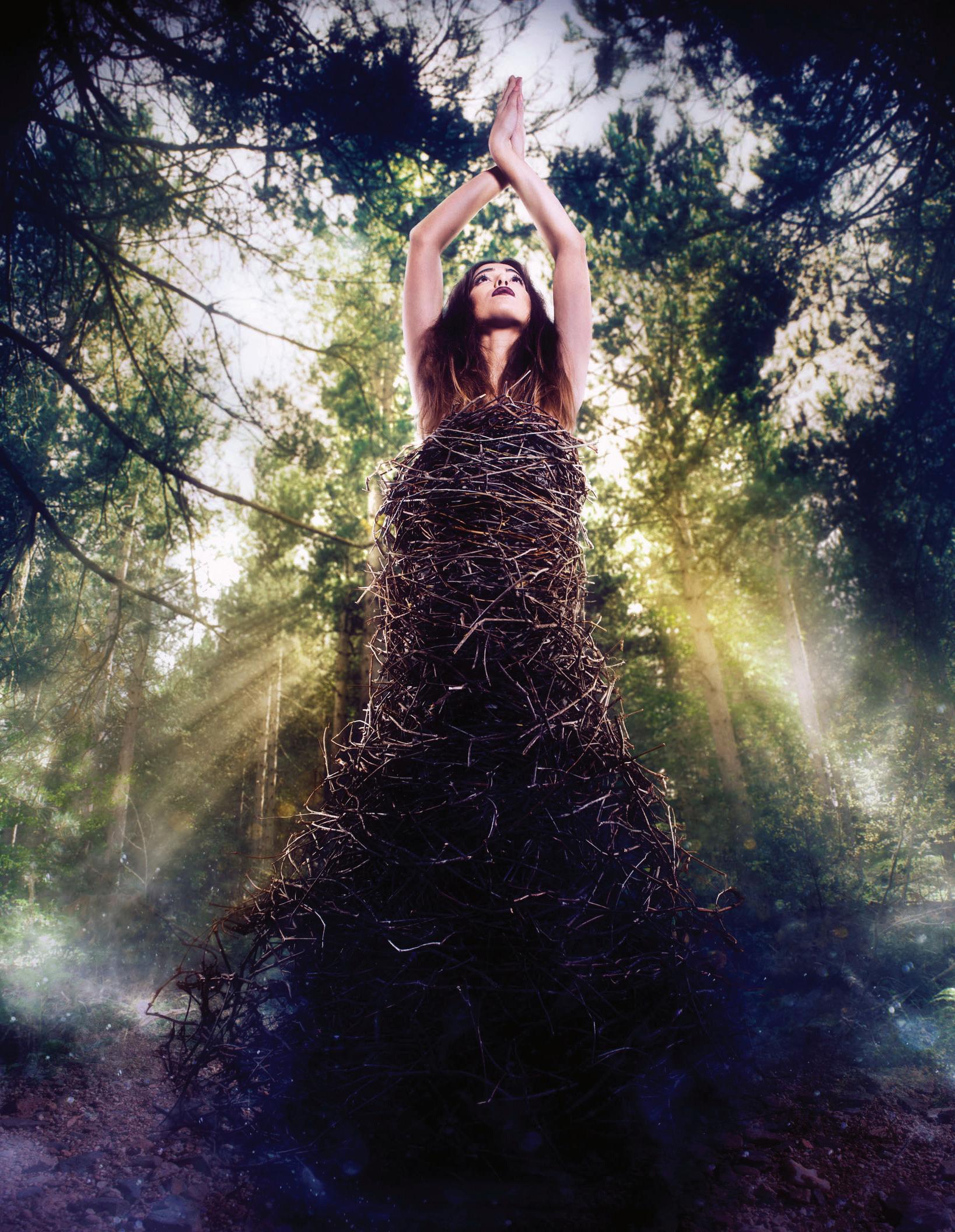
Ever since then, penicillin has been used, even abused, throughout the world, all the while its bacterial victims have been working on survival strategies. According to the World Health Organization(7), “antibiotic resistance is rising to dangerously high levels in all parts of the world. New resistance mechanisms are emerging and spreading globally, threatening our ability to treat common infectious diseases. A growing list of infections – such as pneumonia, tuberculosis, blood poisoning, gonorrhea, and foodborne diseases – are becoming harder, and sometimes impossible, to treat as antibiotics become less effective. Where antibiotics can be bought for human or animal use without a prescription, the emergence and spread of resistance is made worse. Similarly, in countries without standard treatment guidelines, antibiotics are often over-prescribed by health workers and veterinarians and over-used by the public. Without urgent action, we are heading for a post-antibiotic era, in which common infections and minor injuries can once again kill.” If the thought of increasingly-resistant microbes is not scary enough to keep you awake at night, recent studies have highlighted the existence of bacteria that actually eat penicillin. Dr. Guatam Dantas, a microbiologist at Washington University in St. Louis, has spent over a decade deconstructing the steps needed for microbes to break down penicillin. Dr. Dantas suggests that speciallyengineered microbes could be used to break down antibiotic pollutants, for example, in waste from farms where the drugs have been used in livestock or in effluent from hospitals or alcohol plants (some distillers dump fermentation tank sediments straight into municipal sewage, often with yeast and other organisms still alive).
Into the Future
Our digestive tracts are hosts to trillions of microbes that play a very important role in human biology and disease control. Studies have shown that each milliliter of the large intestine holds approximately 10^11 microbial cells(8). Can you imagine what would happen if we accidentally ingest penicillin-eating bacteria and they set up permanent residence in our guts, allowing other undesired infectious organisms to establish colonies in our microbiota? On the other hand, will human-genome modifications ever allow us to produce additional microbe killer toxins, as part of our immune system? As you read these lines, scientists are also identifying non-Saccharomyces fermentation yeast alternatives, genetically altering them to optimize them for commercial use. These new strands will, undoubtedly, produce their own new versions of killer toxins, which -everyone hopes- shouldn’t have unexpected negative consequences. While the future is not carved on granite, there is one thing we can surely rely on: nature’s chess game will continue to evolve and adapt until the end of time. Hopefully we humans will be around to witness much of it. To the spirit of survival! Luis
References
1. Bevan EA, Makower M “The physiological basis of the killer character in yeast” - 1963 2. Woods DR, Bevan EA “Studies on the nature of the killer factor produced by
Saccharomyces cerevisiae” - 1968 3. Klassen R, Schaffrath R, Buzzini P, Philip
Ganter PF “Antagonistic interactions and killer yeasts” - 2017 4. Tredoux et al.,1986 , Magliani et al.,1997,
Schmitt & Breinig, 2002, Mohamudha
Parveen & Ayesha Begum, 2010 5. Christina Kennedy (Plant Pathology &
Microbiology at The University of Arizona)
“Importance of Biological Nitrogen Fixation” 6. Muriel Derrien, Johan E.T. van Hylckama
Vlieg “Fate, activity, and impact of ingested bacteria within the human gut microbiota” - 2015 7. https://www.who.int/news-room/fact-sheets/ detail/antibiotic-resistance 8. Walter J, Ley R. The human gut microbiome: ecology and recent evolutionary changes.
Annu Rev Microbiol - 2011.