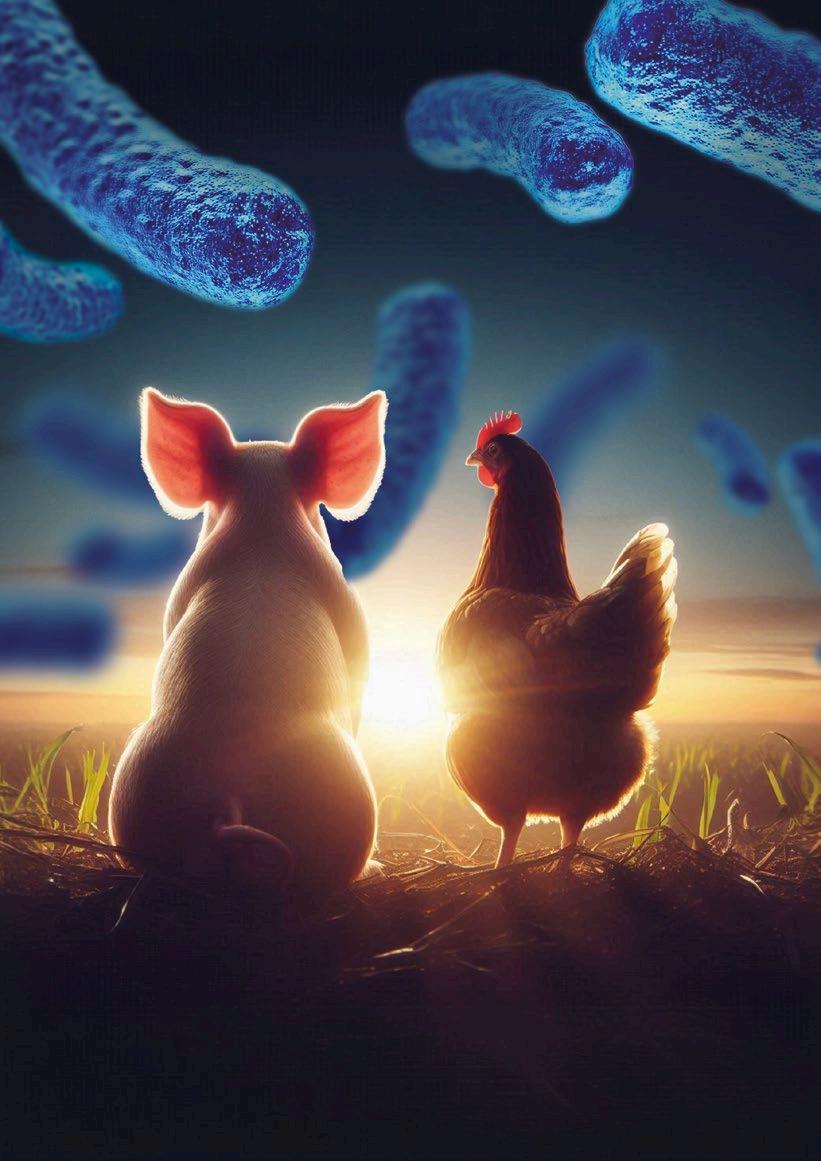
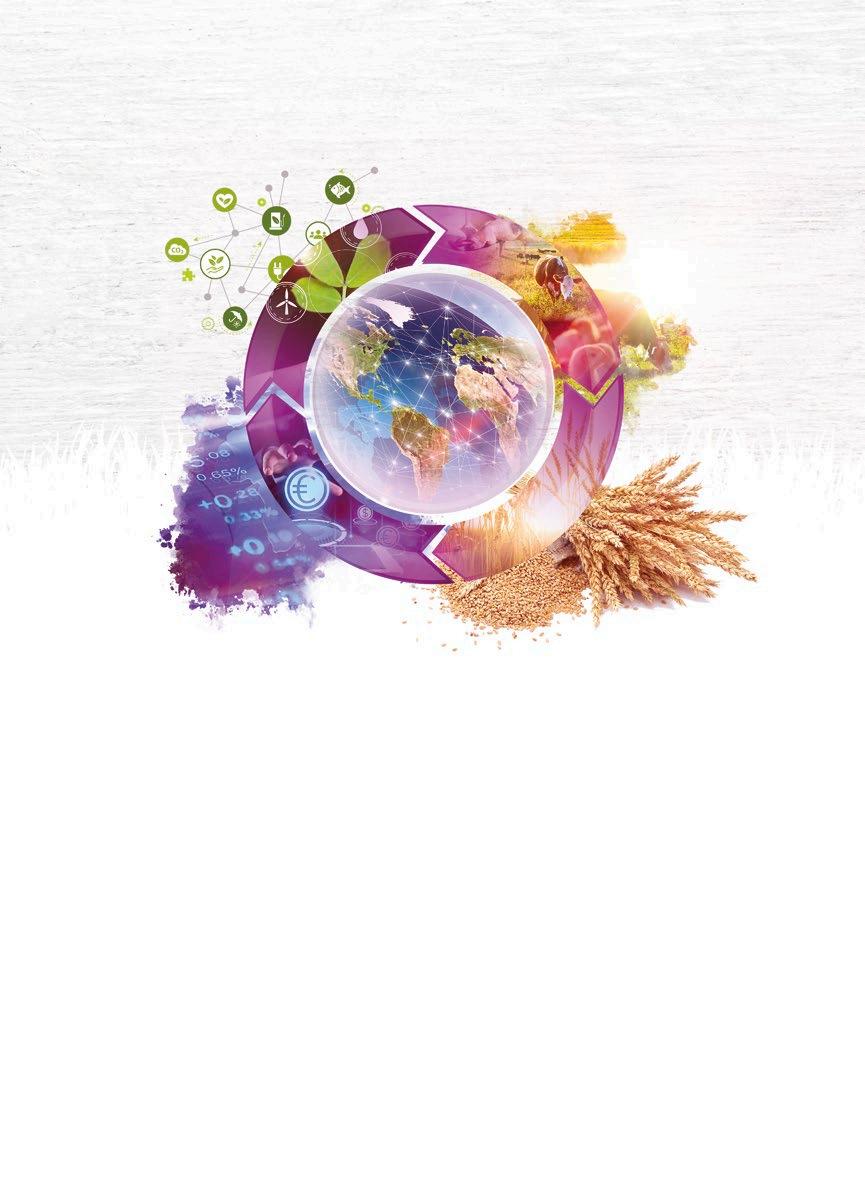
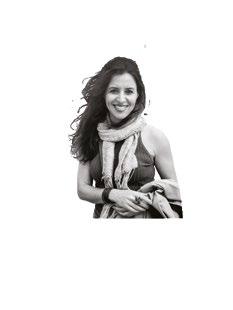

It is time to present our second edition of nutriNews International, June 2024. With full speed into summer for most of our international readers, we have several interesting topics to share with all of you.
In recent years, the importance of gut health has become increasingly recognized, with the spotlight shining on the role of probiotics and prebiotics in maintaining a healthy digestive system. These two classes of supplements, while similar in name, play distinct roles in promoting gut health and overall well-being.
Together, pre- and probiotics form a powerful duo for gut health. While prebiotics provide the nourishment for beneficial bacteria need to flourish, probiotics introduce these beneficial bacteria into the gut. By incorporating both into the feed, you can support a healthy gut microbiota and promote overall wellness in livestock. Discover some more information on this interesting topic.
“The Brazilian Tables” is a project designed and coordinated by professors and editors Horacio Santiago Rostagno and Luiz Fernando Teixeira Albino. This year, the 5th edition of the book for poultry and swine features an update on the chemical composition of feeds, energy values and digestible amino acids; the nutritional requirements of broilers, breeders, layers, quail and swine in production and reproduction; and recommendations for mineral and vitamin supplementation.
In this edition of our nutriNews International we share with you two articles, one for poultry and one for swine, were we share some highlights of the content of the freshly released book. Find out some of the updates that were presented in Brazil.
With a focus on ruminants
We have several trending topics to share with you, from a nutrimental approach to cope with heat stress in high producing dairy cows, application of amino acids in the feeding of cattle, to the use of exogenous β-mannanase to improved FEC in lactating dairy cows. All these titles were selected for you to bring to the table different forms to achieve better production results in a difficult industry sector, dairy.
This and many other interesting subjects can be found in this new edition.
Enjoy your reading.
EDITOR COMMUNICATION GROUP AGRINEWS LLC
ADVERTISING
Luis Carrasco +34 605 09 05 13 lc@agrinews.es
CUSTOMER SUPPORT
Mercé Soler
EDITORIAL STAFF
Álvaro José Guzman
Viviana Schroeder
TECHNICAL DIRECTION Dr. Edgar Oviedo (poultry)
info@grupoagrinews.com nutrinews.com grupoagrinews.com
Free distribution magazine AIMED AT VETERINARIANS AND TECHNICIANS Legal Deposit Nutrinews B11597-2013
Subscription price 125$
Legal Deposit: B15281-2024 ISSN 2938-8929
Noun Project / Freepik/Dreamstime
Use of pre and probiotics in the feeding of monogastric
Márcia Gabrielle Lima Cândido Veterinarian and Doctor in Rural Constructions and Ambience, Department of Agricultural Engineering of the Federal University of Viçosa, Brazil
The 5th Edition of the Brazilian Tables for Poultry and Swine: 1. Feedstuff Description and Poultry Requirements
Edgar O. Oviedo Rondón Prestage Department of Poultry Science, NC State University TechnoCare Dual Power for Efficient Poultry Production
Can NATU-B4 reduce the cost of pig production
Nuproxa Technical Team
Feeding for high milk quality in dairy cows under heat stress
The 5th Edition of the Brazilian Tables for Poultry and Swine: 2. Swine Nutritional Requirements
Edgar O. Oviedo Rondón Prestage Department of Poultry Science, NC State University
Carolina Kyriacou Dairy Consultant at Vettaky Ltd
Amino acids in the feeding of cattle (Part 2)
Beatriz de Magalhães Ceron and Breno Luis Nery Garcia
Exogenous β -mannanase improved FEC in lactating dairy cows.
Kebreab, E., J. Mendez, P. Ji, J-J. Lee, and S. Seo§ Technical Team CTCBIO
Márcia Gabrielle Lima Cândido Veterinarian and Doctor in Rural Constructions and Ambience, Department of Agricultural Engineering of the Federal University of Viçosa, Brazil
The gastrointestinal tract (GIT) microbiota of mammals comprises approximately 10^14 microorganisms and includes a wide diversity of microbial species (Míguez et al., 2016; Yang and Xu, 2018; Yazad et al., 2020). The GIT is the most colonized organ in the body.
The microbiota is associated with a wide range of functions within the host, including the fermentation of complex macronutrients, production of nutrients and vitamins, protection against pathogens, and maintenance of immune system balance (Han et al., 2018; Li et al., 2020; Yin et al., 2018, 2020).
Recent studies have indicated that diet has a considerable effect on the modulation of intestinal microbiota (Donaldson et al., 2016; Lalles, 2016). Some of the main additives used in animal nutrition for the modulation of intestinal microbiota are probiotics and prebiotics.
Although probiotics have been used for many years, it was in the 1960s that it was first demonstrated that strains of Lactobacillus were able to improve the growth performance of pigs (Ahasan et al. 2015).
Since then, the most frequently used probiotics in monogastric animals are yeasts (Saccharomyces boulardii and S. cerevisiae) and bacteria (Lactobacillus spp., Enterococcus spp., Pediococcus spp., Bacillus spp.) that act in the cecum and colon.
The most common benefits of using probiotics for monogastric animals are:
Increased body weight,
Reduced incidence of diarrhea,
It is important to highlight that probiotics play an important role in the prevention of diarrhea in piglets.
Additionally, probiotics such as Enterococcus faecium and Bacillus subtilis can reduce the concentration of ammonia in bird excreta (Dhama et al. 2008), which contributes to maintaining litter quality, air quality in the barns, and reducing respiratory problems and lesions in the foot pad.
Production of antimicrobial substances, such as organic acids (mainly lactic, acetic, and formic acid), bacteriocins, hydrogen peroxide, and other compounds that inhibit intestinal pathogens (Corcionivoschi et al. 2010; Murali e Kavitha, 2010);
Production of enzymes (for example, proteases, amylases, lipases, and glycosidases) by the microbiota. Bifidobacterium bifidum produces a DNA polymerase that has been described as important in the repair of damaged cells;
Reduction of toxic amines, which are produced by some intestinal microorganisms and have irritating and toxic activity or cause diarrhea;
Most commonly used probiotics as additives in animal nutrition
Some species of the genus Lactobacillus used as feed additives have demonstrated beneficial abilities, particularly in reducing mortality in fish, improving growth performance in piglets, and enhancing egg production and quality in poultry
Competition for nutrients and/or for attachment sites (competitive exclusion) in the intestinal mucosa with potentially pathogenic bacteria, thus preventing pathogenic bacteria from colonizing the intestinal tract;
Stimulation of the immune system, either directly or indirectly, by modulating the commensal flora or the immune system. Probiotics play a role in the host’s specific and nonspecific immune responses and in stimulating the production of pro and anti-inflammatory cytokines (O’Hara y Shanahan, 2006; Walsh et al., 2008; Wang et al., 2009)
Some probiotic strains act as immunomodulators, increasing macrophage activity and local antibody levels, inducing interferon production, and thus activating Natural Killer cells (Yasui et al. 1989; Perdigon et al. 2001).
It is a Gram-positive bacterium belonging to the group of lactic acid-producing bacteria. .
Additionally, Lactobacillus has been associated with improving immune defense mechanisms in fish and reducing Salmonella contamination in broiler chickens.
Bifidobacteria are found in large numbers in the intestines of animals and humans. Their presence does not always indicate good intestinal health in the host. When used as a feed additive in piglets, the species Bifidobacterium pseudolongum showed significant results with improved feed conversion rate.
In poultry, the species Bifidobacterium animalis, Bifidobacterium thermophilum, and Bifidobacterium longum demonstrated their ability to reduce coccidiosis in chickens infected with Eimeria tenella, protective activity against Salmonella and Listeria in vitro, and against E. coli in chickens.
Overall, bacteria belonging to the genus Bifidobacterium have potential as additives for feed and as alternatives to conventional antibiotics
Some bacteria of this genus, such as Bacillus subtilis, are regularly used as a dietary supplement, especially in aquaculture and poultry farming. Various studies have shown that these species have a high potential for immunomodulation and protection against diseases
Bacillus licheniformis has also shown probiotic abilities when used as an additive in pigs and has been effective against diarrhea occurring in piglets 3-10 days after weaning, caused by enterotoxigenic strains of E. coli.
However, some species such as Bacillus cereus can cause problems due to the endotoxins and emetic toxins they produce.
Enterococcus is a common member of the endogenous intestinal microbiota of humans and animals. Enterococcus strains have been used as feed additives in poultry and pigs as alternatives to the use of antibiotics in feed.
The E. faecium strain has demonstrated its ability to stimulate other lactic acid bacteria (especially Lactobacillus) in the small intestine of turkeys and to improve feed conversion, intestinal morphology, and beneficial manipulation of cecal microflora in chickens
However, the Enterococcus genus has some disadvantages as these bacteria can participate in the transmission of antibiotic resistance.
Additionally, this genus is frequently associated with pathologies such as infections of the urogenital tract and endocarditis.
Despite having demonstrated good results, the use of probiotics belonging to this genus should be verified beforehand to use only strains that pose no danger to the health of the animals
Saccharomyces is a genus of yeast that is also part of the intestinal microbiota. Within this genus, S. cerevisiae is the most well-known species and is most commonly used as a probiotic.
It is recognized for improving the reproductive performance of sows, increasing the concentration of immunoglobulin G (IgG) in colostrum and subsequently in piglets’ plasma IgG to improve growth performance and promote “healthy” intestines in pigs.
Positive results from the use of S. cerevisiae have also been observed in fish. It is capable of improving the growth, hematological, antioxidant, and immunological responses of Nile tilapia, enhancing Nile tilapia’s resistance against a pathogenic fungus, as well as increasing the innate immune response of golden dorado.
Other species belonging to this genus, such as Saccharomyces carlsbergensis, are also used as probiotics in animal nutrition.
The concept of prebiotics was first described in 1995 by Gibson and Robertfroid (1995) and has undergone some changes over time (Table 1)
Table 1
Definitions of Prebiotics
1995 “Undigested food/feed components that, by stimulating the growth and/or activity of a single type or a limited number of microorganisms residing in the gastrointestinal tract, improve the health condition of a host ” (Gibson and Roberfroid, 1995)
2004 “A selectively fermented component that allows specific changes in the composition and/or activity of microorganisms in the gastrointestinal tract, being beneficial for the health and well-being of the host” (Gibson et a., 2004)
Prebiotics are indigestible carbohydrates, considered functional fibers that are not digested by pathogenic bacteria, nor by birds or pigs, remaining available only for beneficial bacteria
2007 “Non-viable food/feed component that confers a health benefit on the host associated with the modulation of the microbiota” (FAO, et a., 2007)
2010 ‘Dietary prebiotics’ as “a selectively fermented ingredient that results in specific changes in the gastrointestinal composition and/or activity of the microbiota, thus conferring benefit(s) to the health of the hos” (Gibson et al., 2010)
2015 “Undigestible compound that, through its metabolism by microorganisms in the intestine, modulates the composition and/or activity of the intestinal microbiota, thereby conferring a beneficial physiological effect on the host” (Bindels et al., 2015)
Adapted from Markowiak et al., 2018
2016 “Substrate that is selectively utilized by host microorganisms, conferring benefit(s) to health” (Gibson et al., 2017)
Enhancement of epithelial barrier
and defensins
Probiotics
Pathogens
Mucus
Increased adhesion to intestinal mucosa
1. Main action mechanisms of probiotics
There are several prebiotics that can be used in animal nutrition, such as:
Mannanoligosaccharides (MOS)
Fructooligosaccharides (FOS)
Gluco-oligosaccharides (GOS)
Inulin
Arabinoxylans (AXOS)
Chito-oligosaccharides (CHOS)
Isomalto-oligosaccharides (IMOS)
Glucomannan (OGM)
Alginate oligosaccharides (AOS)
Pectin oligosaccharides (POS)
Inhibition of adhesion
Production of antimicrobial substances
Competitive exclusion of pathogen microorganisms
Modulation of the immune system
Source: adapted from Bermudez-Brito et al. (2012)
MOS, GOS, and FOS are the most studied and used prebiotics as additives in animal nutrition.
According to Wang (2009), there are five basic criteria for classifying feeds as prebiotics:
1. Resistance to digestion in the upper sections of the gastrointestinal tract
2. Fermentation by the intestinal microbiota
3. Beneficial effect on the health of the host
4. Selective stimulation for the growth of probiotic microorganisms
5. Stability under various feed processing conditions
The mechanisms of action of prebiotics include blocking the receptor portion for bacterial adhesion; modulation of immunity produced by antibacterial compounds; increasing exudation in the intestinal lumen; as well as inducing morphological alteration in the intestinal structure (Pourabedin y Zhao., 2015; Hammed, 2021)
Prebiotics are not digested in the upper GI tract and are believed to be fermented by specific bacteria when they reach the colon. The environment in the colon is suitable for fermentation and growth of commensal bacteria due to its slow transit, availability of nutrients, and pH.
In this way, the acidic environment in the colon can alter the composition of the microbiota, which helps suppress the growth of some potential pathogens such as E. coli, Clostridium, Streptococcus faecalis, and Proteus, and increases the growth of some beneficial bacteria, including bifidobacteria, lactobacilli, and Eubacterium (Morrison and Preston, 2016; O’Callaghan and van Sinderen, 2016; Zhang et al., 2015)
Prebiotics can stimulate or modulate the immune system. One of the mechanisms of immunological modulation by prebiotics is attributed to the stimulation of humoral immunity through the interaction of sugar with specific receptors located on macrophages and dendritic cells, which induce the release of cytokines and the proliferation of lymphocytes (Saad et al., 2013)
The fermentation of carbohydrates in the colon leads to the production of short-chain fatty acids (SCFAs), primarily acetate, propionate, butyrate, and other metabolites such as lactate, pyruvate, ethanol, and succinate (Janssen y Kersten, 2015; Sarbini y Rastall, 2011; Slavin, 2013).
SCFAs are important because they can reduce protein fermentation in the intestinal tract. Butyrate, for example, regulates cell growth and induces differentiation and apoptosis in the small intestine, resulting in improved cell proliferation as well as better digestion and absorption in the small intestine (Linberg, 2014; van der Aar et al., 2017).
The presence of prebiotics such as oligosaccharides in the intestine can also increase the resistance of probiotic strains due to bacterial adhesion properties. Oligosaccharides also help maintain the integrity of the intestinal mucosa, increasing villi height, mucin release, and mucosal biofilm composition (Wan et al., 2018a; Yasmin et al., 2015)
Some probiotics can modulate the immune system by binding to the G protein receptors within the gut-associated lymphoid tissue (GALT). Another mode of action is via stimulation of mucus production, decreasing the connection between bacteria and the epithelial barrier, increasing cellular resistance, and secretion of immunoglobulin A (IgA) (Bischoff et al., 2014; Celi et al., 2019)
Figure 2. Main action mechanisms of prebiotics
Stimulating the growth of probiotics
Prebiotics
Probiotics
source: adapted from Fong et al. (2020)
Selective fermentation by probiotics
Selective fermentation
being absorbed by the intestine and exerting an antiinflammatory action
interacting with pathogens preventing their colonization
Some specific types of prebiotics, such as COS, inulin, AXOS, and AOS, have been associated with immunomodulatory effects in monogastric animals (Ding et al., 2018; Vogt et al., 2015; Wan et al., 2018b; Xiong et al., 2015).
A review by Seifert and Watzl (2007) concludes that the addition of inulin and FOS to the diet has an effect on the immune system, with a positive impact on immunological biomarkers in intestine-associated lymphoid tissues..
Several studies have shown that prebiotics positively affect the functionality of the immune system, altering the expression of proinflammatory cytokines (Hu et al., 2018; Wan et al., 2018a; Wang et al., 2016a; Xiao et al., 2016).
It can be observed that the use of pre- and probiotics is beneficial for the health, well-being, and productive efficiency of poultry and pigs.
These additives can bring various benefits to animal production, especially with the increasing restrictions on antibiotics.
On the other hand, prebiotics can affect the intestinal microenvironment and the utilization of other nondigestible ingredients and dietary compounds, such as antibiotics, minerals, and vitamins. Supplementation of inulin (1%, 2%, and 3%) in pigs increased plasma concentrations of zinc and iron (Samolinska y Grela, 2017).
In turn, higher concentrations of XOS (between 0.1 and 0.5 g/kg) demonstrated an improvement in bone mineralization (Wang et al., 2017).
Use of pre and probiotics in the feeding of monogastric animals.
DOWNLOAD PDF
>> Bibliography available upon request
COUNTRIES OF DISTRIBUTION
Worldwide
First probiotic on the market with proven in vivo intestinal germination. Probiotic resistant to pelleting and gastric pH , with precise germination in the intestines of birds. Action in modulating intestinal microbiota, even favoring the control of pathogens such as E. coli , Clostridium perfringens and Salmonella spp . It promotes intestinal integrity and has an antiinflammatory action that promotes a greater balance of intestinal health, decreases dysbiosis, and provides higher zootechnical gains. Product with proven compatibility with the main market promoters and anticoccidials.
CELMANAX has several benefits for animals. Firstly, it helps to prepare their immune system to anticipate potential threats, enabling them to respond quickly. Additionally, it has the ability to bind bacteria and toxins, leading to improved feed efficiency. As a result, CELMANAX can help boost profits while reducing expenses on balanced feed. Lastly, it helps to maintain intake and performance, even during periods of increased heat and humidity. It helps reduce the number of withdrawals due to illness, helping to increase the producer’s profits
CELMANAX is a unique blend that contains a rich supply of fermentation metabolites. These metabolites are produced by fermenting a specific medium using Saccharomyces cerevisiae CELMANAX is an excellent source of yeast material, which provides essential nutrients for all types of livestock and poultry.
CELMANAX offers numerous immunity benefits to boost animal performance and productivity, surpassing any single yeast culture, monoand oligosaccharide, or glucan ingredient in feed. Worldwide
Improves pellet quality, feed efficiency, and mycotoxin binding while stimulating daily earnings. BG MAX is a biologically active product that contains Bentonite and Refined Functional Carbohydrates (RFCs).
BG MAX combines our RFC technology with processed bentonite to help animals maintain performance in the face of mycotoxins
INGREDIENTS CONCENTRATION
COMERCIAL NAME
COMPANY/ MANUFACTURER
Product for incorporation into premixes that will be used for the production of feed for broilers, turkeys, breeders, and layers. 10 g/t of feed
Product for direct incorporation into feed for chicken, turkeys, breeders and layers. 500 g/t of feed
Alterion® NE Bacillus subtilis DSM 29784 1×10 10 UFC/g prod
Alterion® NE50 Bacillus subtilis DSM 29784 2×10 8 UFC/g prod
Calf starter: 1 g/hd/day Milk replacer: 1 g/hd/day
Heifer: 3 g/hd/day
Pre-fresh dry cows: 6 g/hd/day Lactating cows: 3 g/hd/day
Newborn to 110 kg: 1 g/hd/day
Beef Calf, 110-225 Kg: 2 g/hd/day Feedlot: 2 g/hd/day Cows: 3 g/hd/day
Dairy
Beef
Swine Sow: 0,20 kg/ton Nursery: 0,20 kg/ton Grow/Finish: 0,20 kg/ton
Chickens Layer: 0,05 kg/ton Broiler: 0,05 Kg/ton
Turkeys Breeder, Poult, Grow/Finish: 0,05 kg/ton
Goats Kids: 0,4 g/hd/day Goats: 0,7 g/hd/day
Sheep Lambs: 0,5 g/hd/day Sheep: 1 g/hd/day
Dairy cattle 14-28 grams / head / day
Poultry 1 Kg / Metric Ton of Complete Feed
Swine Start 2 Kg / Metric Ton of Complete Feed Development 1 Kg / Metric Ton of Complete Feed
Hydrolyzed yeast, yeast extract and yeast culture ( Saccharomyces Cerevisiae ) RFC (REFINED FUNCTIONAL CARBOHYDRATES).
CELMANAX
Natural fermentation of yeast culture with aluminum silicate
BG-MAX
COUNTRIES OF DISTRIBUTION
ADDITIONAL INFORMATION
Worldwide
Chile & Perú
TechnoCare® is a probiotic combination of two potent bacterial strains, the multifaceted lactic acid producer
Bacillus coagulans DSM 32016, and a specialist in pathogen inhibition Bacillus licheniformis DSM 33806. It enhances animal performance and maintains overall health through multiple complementary modes of action.
DOSAGE
400 g / t of feed
SPECIES
TARGET
CONCENTRATION
INGREDIENTS
COMERCIAL NAME
COMPANY/ MANUFACTURER
Product recommended for inclusion in all feeds, especially in early ages and phases of high digestive problems. Helps to balance the intestinal microbiota through the adhesion of flagellated microorganisms and stimulates the immune system in a synergistic way. Natural solution to complement medication reduction programs in feed.
From 400 g/t feed, depending on the objectives.
All animal species
Bacillus coagulans (DSM 32016) 2.5 x 10 9 CFU/g
TechnoSpore®
Bacillus coagulans (DSM 32016) 2 x 10 9UFC/g of additive
Bacillus licheniformis (DSM 33806) 8 x 10 9UFC/g of additive
TechnoCare®
Yeast fractions from 3 strains of Saccharomyces cerevisiae Cyberlidnera jadinii)
Synergistic combination of different inactivated yeast strains and yeast fractions
YANG
Worldwide
Yeast hydrolyzed from a controlled hydrolysis process that stimulates yeast cells breakdown by specific exogenous and endogenous enzymes, releasing a high nutritional and functional value. It is a highly palatable alternative protein source that offers a well-balanced level of highly digestible amino acids, which contribute to animal growth and maintenance of proper intestinal health.
From 2.5 Kg/t depending on the species and production stage.
MOS are able to bind numerous undesirable bacteria by limiting their fixation in the digestive tract, ß-glucans are particularly interesting for their power to stimulate the non-specific immune system of the animal and the yeast cell walls have a certain capacity to absorb mycotoxins.
Starting at 0.5 kg/t, recommended doses vary depending on the target species and the level of challenge.
Balanced level of beta-glucans and mannanoligosaccharides (MOS)
All species
Saccharomyces cerevisiae
Specifically designed hydrolyzed yeast
YELA PROSECURE
Saccharomyces cerevisiae
Selected and optimized yeast cell wall
OPTIWALL
COUNTRIES OF DISTRIBUTION
Regulates intestinal microbiota, helps limit negative impact of enteric pathogen toxins ( C.difficile, E.coli ), reinforces intestinal integrity and modulates inflammatory response. Overall improvement of productive performance and during critical periods of stress such as piglet weaning, sow peripartum or heat stress periods. First feed additive with a food safety claim for its benefits on salmonella contamination reduction on broiler carcasses
Worldwide
Liquid feed hygiene enhancer: Authorized as a technological additive for its ability to regulate acidity and improve the hygienic conditions of liquid feed, for all animal species. Gut microbiota stabilizer: Lactic acid producing bacteria Lowers the intestinal pH , favoring the development of positive microbiota, protects the intestinal barrier function and improves digestibility. Improves performances, contributes to reduced risk of enteric mortality and maximizes profits.
Recommended for use in drinking water to help reinforce intestinal microbiota during periods of digestive stress, after antibiotic treatments, vaccinations, etc.
50 g/t of feed
100 g/t of feed
All pigs throughout the production cycle and all minor swine species. Chickens and turkeys for fattening, and minor poultry species for fattening.
INGREDIENTS CONCENTRATION
COMERCIAL
COMPANY/ MANUFACTURER
2 x 10¹⁰CFU/g
1 x 10¹⁰CFU/g
100 g/t of feed
Gut microbiota stabilizer: All fattening and breeding swine other than sows. All poultry species. All species of fish and all crustaceans. Liquid feed hygiene enhancer: all animal species
1 x 10¹⁰CFU/g
Saccharomyces cerevisiae boulardii CNCM I-1079
LEVUCELL SB 20
LEVUCELL SB 10ME TITAN
Pediococcus acidilactici CNCM I-4622
200g/m³ of drinking water
All fattening and breeding swine other than sows. All poultry species.
2.5 x 10⁹CFU/g
Pathogens are constantly attacking your cows. They come in many forms—viruses, bacteria, mycotoxins and more and continuously have the ability to rob your cows of their health and productivity.
Of the samples analyzed, fecal samples and 84.7% of feed samples contained detectable clostridia.
This same survey also found that 78.5% and 33.6% of fecal and feed samples, respectively, contained , a toxin-producing known to contribute to hemorrhagic bowel syndrome (HBS)
contamination during feed-out, feed mixing or delivery.
If spoilage occurs in forages, high levels of yeasts and molds can end up in the TMR. Yeasts and molds can steal valuable nutrients from the cow and may cause inconsistent intakes or impact performance.
Mycotoxins are secondary metabolites produced in feeds by various species of molds. Although the rumen microbial community is able to detoxify many different mycotoxins, a dairy cow’s high rate of feed intake and feed passage out of the rumen make it unlikely for complete detoxification of mycotoxins that find their way to the small intestine.
Since mycotoxins are too prevalent to completely mitigate, it’s important to focus on a proactive defense that creates resiliency in your cows to help them avoid, or minimize, the effects of pathogen challenges. Now more than ever, preparing your cows to help them fight off disease challenges is critically important. The following are three important steps to build resiliency:
In research trials, CELMANAXTM has shown to bind pathogens such as E. coli and Salmonella enterica, preventing them from creating health challenges in the cow. Cows fed CELMANAXTM also had lower somatic cell counts and this stronger immunity has resulted in improved milk production.
A healthy immune system is one of the cow’s best chances to avoid a health challenge, and the proactive strengthening of the cow’s’ immune system is the main driver of establishing herd resilience. In your ration, consider including products such as CELMANAX™ from Arm & Hammer Animal and Food Production.
EFFECT OF CELMANAXTM ON BACTERIAL PATHOGENS IN VITRO Control
CELMANAXTM contains Refined Functional Carbohydrates™ (RFCs™) that support growth of beneficial bacteria and prevent certain pathogens from attaching to the intestinal wall and cause disease. CELMANAXTM combines the benefits of multiple feed additives in one consistently high-quality formula.
Graphic 1. CELMANAX TM was shown to bind pathogens such as E.Coli and Salmonella enterica.
CelmanaxTM
Graphic 2. In three studies, cattle fed CELMANAX TM had numerically lower somatic cell count than the control groups.
Graphic 3. In four trials shown above, cows fed CELMANAX TM produced more milk per day than the control group.
The gut microbiome— from the rumen through the large intestine— is a key driver of the health and productivity of the cow.
Gut bacteria are specific to your dairy, and it’s important for beneficial bacteria to thrive while reducing the impact of pathogenic organisms.
Specific Bacillus strains can produce antimicrobial peptides that inhibit pathogen growth.
Research has also shown that Bacillus can impact tight junction proteins to help create tighter barriers between cells in the small and large intestine to prevent pathogens from entering the bloodstream.
CERTILLUSTM from Arm & Hammer
Animal and Food Production offers dairy-specific Bacillus strains to enhance the microbial environment inside your cows. This creates a better gut environment and improved rumen function by enhancing the role of beneficial bacteria to help cows deliver more consistent production and withstand various health challenges.
With Bacillus products, producers can create more resilient animals that can overcome health and stress challenges. Including a Bacillus product in the ration can help cows overcome reoccurring stressors and focus more on maintaining health and productivity.
Work with your nutritionist to create and feed Bacillus strains specific to your cows’ environment to make their impact even more effective.
TMR Sample Number
Graphic 4. Total Clostridia enumeration results from TMR samples.
Because they are ever-present on your dairy, protecting cows from the impact of mycotoxins is more important than all-out removal. Building resilience inside the cow is key to preventing mycotoxin challenges, which include strengthening immunity and building a thriving gut environment.
But another component is the process of actually detoxifying mycotoxins to block their toxic effects and prevent them from crossing the gut barrier to do their damage.
Add these ingredients to take a step beyond just binding mycotoxins to achieve more broad-spectrum efficiency.
One of those ingredients is BG-MAX & Hammer Food Production. is the only product that does more than just bind mycotoxins, instead taking a comprehensive approach to managing the issue.
BG-MAX includes RFCs to create a combination of binding mycotoxins while also strengthening gut integrity. A stronger gut means greater resilience. Also, BG-MAX contains highly refined bentonite that targets key mycotoxin strains without impacting essential nutrients the cow needs.
Your nutritionist and veterinarian can help create a program to build resiliency in your herd and keep viruses, bacteria, mycotoxins and other pathogens at bay. Work with them to put together a strategy that includes key nutrition components to strengthen immunity and create a robust environment inside the gut.
Edgar O. Oviedo-Rondón
Prestage Department of Poultry Science, North Carolina State University, Raleigh, NC
On March 26, the 5th edition of the Brazilian Tables for Poultry and Swine was released in Viçoca, Minas Gerais, Brazil. The 5th edition was dedicated in memory of Professor Luiz Fernando Teixeira Albino, one of the editors of all previous editions, who passed away last February 13.
This document was mainly developed at the Federal University of Viçosa but includes important contributions of researchers from São Paulo State University (UNESP), the Federal University of Paraíba, and the Federal University of Lavras.
These Tables become an additional reference for practical feed formulation of monogastric animals worldwide.
In a couple of articles, we will summarize the main contents of this publication to stimulate readers to obtain the publication and evaluate its values and equations.
To simplify the reading of this article, most of the references were omitted and all can be found in the 575 pages of this well-documented book. These Tables can be acquired with calculators or software to apply the mathematical models used.
This publication includes a description of nutrient composition, amino acid (AA) digestibility, and energy content of most feed ingredients used in poultry and swine diets in Brazil.
However, it can be a reference for other countries with feed ingredients of similar origin or characteristics. It also contains the methodologies, equations, and diverse examples to estimate the energy content of feedstuffs, and nutritional requirements for broilers, broiler breeders, layers, and swine.
These tables contain a description of Brazilian feed ingredients. This edition contains two categories of corn grain and soybean meal in addition to the average values.
The two additional corn categories were calculated using the average dry matter value plus or minus one standard deviation. The soybean meals included higher or lower content of hulls.
The phytate phosphorus (P) content of Brazilian plant feedstuffs was determined in addition to total P, and the non-phytic P was considered as available P. In animal feedstuffs, the P availability was considered 100%, except for meat and bone meals which had 90% P availability.
Values of standardized digestible P content determined in broiler
To estimate standardized digestible P values, the authors used endogenous P excretion values of 0.138 mg P/kg of dry matter
The amount of P that phytases can make available for broilers and pigs was estimated using metaanalyses.
The average P equivalence (available and digestible P) values of fungal and bacterial phytases were estimated according to the inclusion level (FTU/kg) from 250 to 1,500 FTU/kg.
Linear responses and equations are provided, but it is important to remember that several non-linear responses have been reported.
Two metabolizable energy (ME) values for poultry are presented for each feedstuff. One for general poultry and another for mature poultry or hens.
It was considered that hens metabolize better (0.3 kcal/g ME more) the nitrogen-free extract and crude fiber components. Net energy (NE) values for poultry feedstuffs were also calculated using the coefficients obtained by Carré et al. (2014).
This considers 76% for digestible protein and 80% for digestible starch, considered as digestible nitrogen-free
Digestible energy (DE) and ME values for swine feedstuffs were determined in growing pigs with weights between 20 and 75 kg. The NE values were calculated using the Noblet et al. (1994) equation. Two values of DE and ME are presented for growing pigs and sows.
Equations to estimate ME and NE for poultry feedstuffs, and DE, ME, and NE values for growing pigs and sows were included to correct these energy values according to the chemical composition. There are digestibility coefficients of
In these Tables, the energy and AA nutrient requirements were determined by using integrated factorial and empirical models. The models presented use Gompertz equations to estimate average body weight (BW) and daily body weight gain (BWgain) to describe growth rates.
One hundred feed ingredients are described and for most of them, the practical recommended inclusion levels are presented for each feeding phase
The non-starch polysaccharide content of some feedstuffs is presented using the methodology of Englyst et al. (1994) and presented as-fed.
Using the estimated growing data, models estimate daily ME and standardized ileal digestible (SID) lysine requirements. The factorial models consider the ME for maintenance and production. The coefficients are different for males and females, but the calculations are similar.
The ME for maintenance was 113 kcal/kg BW0.75 and the ME for growth was estimated with a quadratic equation related to BW. The basic equations to estimate ME requirements for broilers of high performance are:
ME males = (113*BW(kg)0.75) + ((2098 + 961.31*BW – 167.87*BW2) *BWgain (kg/bird/d))
ME females = (113*BW(kg)0.75) + ((2158.2 + 1154.4*BW – 227.78*BW2)*BWgain (kg/bird/d))
Corrections for temperature were proposed. Additionally to ME, the NE levels of broiler feeds were mentioned in this edition.
The requirements are calculated for broiler flocks of high performance. For broiler flocks with standard performance, it is necessary to increase the requirements by 4%.
The correction for environmental temperature (T) effects above thermoneutral (TN) T is = 2.6*BW0.75 (TN – T)
It was considered TN 23 oC between 17-33 days of age, and 20 oC between 34-56 days.
The SID Lys requirement for broiler maintenance was determined as 0.045 mg of SID Lys per kg of metabolic weight (W0.75).
The SID Lys requirements for production were based on a compilation of results of dose-response experiments evaluating diverse Lys levels.
Weekly and total experimental period data were considered to calculate the SID Lys intake and the optimum levels or responses observed. Then, requirements for maintenance and growth were calculated. The total Lys requirements were calculated considering an SID Lys of 90.7%
These are the equations to estimate the SID Lys requirement of broilers with high performance:
SID Lys Req. male (g/day) =
(0.045*BW0.75) + ((14.574 + 2.7554*BW – 0.286*BW2)*BWgain (kg/bird/day))
SID Lys Req. female (g/day) = (0.045*BW0.75) + ((14.143 + 4.0337* BW – 0.5709*BW2)* BWgain (kg/bird/day))
The standard performance is calculated by increasing these values by 4%.
The requirements for other essential AAs were calculated by applying the ideal protein concept (Table 1).
Three growing phases (1 to 17, 17 to 35, and 35 to 56 days of age) were considered. This is an update taking into consideration advances in genetic selection.
The total sulfur AA requirements were established to ensure that at least 55% corresponds to methionine. In the same way, 55% of the phenylalanine + tyrosine should correspond to phenylalanine.
But, glycine and serine requirements are presented together since these two AAs are interchangeable.
Going one step beyond in animal nutrition means ceaseless research and development. Going one step beyond means anticipating developments in the livestock industry and offering the highest quality products and services. Going one step beyond means total commitment to overcoming all our customers’ challenges.
Table 1. Ideal protein ratios used in the Brazilian Tables 2024. Amino acid / Lys ratio (%) used to estimate amino acid requirements (Table 2.20 page 260)
These tables include recommendations for the formulation of low crude protein (CP) diets. A 50% ratio between essential Nitrogen (Ne) or essential AAs and Total N was recommended.
The Ne was determined by calculating digestible essential N and total essential N, considering the N content of essential AAs.
The essential AAs considered were Lys, Met, Thr, Trp, Arg, Gly+Ser, Val, Ile, Leu, His, and Phe.
Additionally, it was recommended to meet the minimum digestible protein requirement to prevent the metabolic use of Ne to synthesize nonessential AA, which impairs broiler performance.
The digestible protein requirement and the total CP requirement can be estimated based on the SID Lys requirement. A proportion of 5.5 or 6.0% can be used to calculate total CP and digestible protein requirements, respectively.
For example for a broiler flock with an estimated 1.22 SID Lys requirement, the total CP requirement will be 22.18% (1.22 x 100/5.5) and the digestible protein requirement will be 20.33 (1.22 x 100/6.0).
The P requirements were also set as a factorial model. The maintenance requirement was established considering the endogenous P losses of 0.026*W
The P requirements for growth were determined with the results of doseresponse experiments for three production phases.
The available P requirement is estimated with coefficients of 6, 5, and 4 for 1 to 21 d, 22 to 42 d, and 43 to 56 d, respectively.
The digestible P requirements had coefficients of 5.4, 4.5, and 3.7 for each of the periods.
Authors recommend using digestible P requirements more than available non-phytic P. The dietary available and digestible P content are expressed in percentage as a function of feed intake.
Available or Digestible P requirement (g/day) = (0.026*BW(kg)0.75) + Coeff. P by period * BWgain (kg/day)
The total calcium (Ca) requirement is estimated by multiplying by the ratios (2.10 and 2.32) of available and digestible P.
Sodium, potassium, and chlorine requirements (mg/bird/day) were calculated as a function of broiler age, and the value obtained was multiplied by dietary ME content expressed in Mcal.
The ME requirements were also calculated with similar factorial models. The ME maintenance requirement was the same as used in broilers 113 kcal ME per kg BW0.75 and 92.3 kcal NE.
The ME requirements for growth are estimated with quadratic functions that vary for each bird category due to the differences in body chemical composition.
The ME requirements for egg production were calculated as 2.4 kcal per gram of egg mass for leghorn and brown hens, and 1.54 kcal/g egg mass for broiler breeders.
The equations used to estimate ME requirements for replacement pullets are:
ME (kcal/day) 1 to 15 weeks = (113*BW(kg)0.75) + ((3.77 + 1.65*BW –0.923*BW2)*BWgain (kg/bird/d))
ME (kcal/day) 16 to 18 weeks = (113*BW(kg)0.75) + ((3.77 + 1.65*BW – 0.923*BW2)*BWgain (kg/bird/d) + (2.4*Egg mass (g/bird/day))
The equations to estimate NE requirements for replacement pullets of laying hens are:
NE (kcal/day) 1 to 15 weeks = (92.3*BW(kg)0.75) + ((2.40 + 1.36*BW –0.706*BW2)*BWgain (kg/bird/d))
NE (kcal/day) 16 to 18 weeks = (92.3*BW(kg)0.75) + ((2.40 + 1.36*BW – 0.706*BW2)*BWgain (kg/bird/d) + (1.54*Egg mass (g/bird/day))
The equations to estimate ME and NE of laying hens are:
ME (kcal/bird/day) = (113*BW(kg)0.75) + (6.68*BWgain (kg/bird/d)) + (2.4*Egg mass (g/bird/day))
NE(kcal/bird/day) = (92.3*BW(kg)0.75) + (4.34*BWgain (kg/bird/d)) + (1.54*Egg mass (g/bird/day))
The equations to estimate ME and NE for broiler breeder pullets are:
ME (kcal/day) 1 to 15 weeks = (113*BW(kg)0.75) + (4.0 + 0.56*BW)*BWgain (kg/bird/d))
ME (kcal/day) 16 to 18 weeks = (113*BW(kg)0.75) + (4.0 + 0.56*BW)*BWgain (kg/bird/d)) + (2.4*Egg mass (g/bird/day))
NE (kcal/day) 1 to 15 weeks = (92.3*BW(kg)0.75) + (2.24 + 0.486*BW)*BWgain (kg/bird/d))
NE (kcal/day) 16 to 18 weeks = (92.3*BW(kg)0.75) + (2.24 + 0.486*BW)*BWgain (kg/bird/d) + (1.54*Egg mass (g/bird/day))
ME (kcal/bird/day) = (113*BW(kg)0.75) + (7.62*BWgain (kg/bird/d)) + (2.4*Egg mass (g/bird/day))
NE(kcal/bird/day) (3.58*BWgain
The corrections for environmental T are similar for all bird categories. Temperature above the TN affects ME for maintenance = 113 + (0.88 x (T – TN)). In T below the TN the correction is = 113 + (6.73 x (TN – T))
The AA requirements were also calculated with the same methodology used for broilers, but the ideal protein profiles are different for pullets, laying hens, and broiler breeders. Those ratios of each AA related to SID Lys levels are described in several Tables in this new publication.
SID Lys Req. 1 to 15 weeks (g/bird/day) = (45.1*BW0.75) + ((24.13 + 14.60*BW –17.44*BW2)*BWgain (kg/bird/day)
SID Lys Req. 16 to 18 weeks (g/bird/day) = (45.1*BW0.75) + ((24.13 + 14.60*BW – 17.44*BW2)* BWgain (kg/bird/day) + (9.46*Egg mass (g/bird/day))
The equation to estimate the SID Lys of table-egg laying hens is:
SID Lys Req. (g/bird/day) = [(45.1*BW0.75) + ((55 + 12 * BWgain (kg/bird/day))/0.80) + (11.5*Egg mass (g/bird/day))]/1000
The Brazilian tables also have the description to estimate the requirements of minerals of table-egg and broiler breeder hens. There are complete descriptions of nutritional requirements for Japanese and European quails, also based on factorial models for Energy and AAs.
The 5th Edition of the Brazilian Tables for Poultry and Swine: Feedstuff Description and Poultry Requirements DOWNLOAD ON PDF
The equations to estimate the SID Lys of broiler breeder pullets are:
SID Lys Req. 1 to 15 weeks (g/bird/day) = [(45.1*BW0.75) + (14.7 + 5.3*BW –0.444*BW2)*BWgain(kg/bird/day)]/1000
SID Lys Req. 16 to 18 weeks (g/bird/day) = [(45.1*BW0.75) + (14.7 + 5.3*BW – 0.444*BW2)*BWgain(kg/bird/day)] + (9.46*Egg mass (g/bird/day))]/100
The equation to estimate the SID Lys of broiler breeder hens is very similar to laying hens, but the requirement for egg production is higher:
SID Lys Req. (g/bird/day) = [(45.1*BW0.75) + ((55 + 12 * BWgain (kg/bird/day))/0.80) + (14.5*Egg mass (g/bird/day))]/1000
In the next article, we will describe the nutrient requirements for Swine estimated in this new version of the Brazilian Tables. For those not familiar with the use of these equations the Book of the Brazilian Tables has multiple examples, and the calculators can aid in understanding.
We hope these summaries contribute to the awareness, understanding, and motivate the study of this excellent source of nutritional information.
Dr. Lydia Zeibich
R&D | Microbiome Specialist | Product Manager Probiotics
Biochem – Feed Safety for Food Safety
B. coagulans DSM 32016 has manifold features to enhance animal performance and support overall health.
For example, this lactic acid-producing strain can comprehensively modulate the host-microbiota by inhibiting harmful gram-negative gut bacteria such as Escherichia coli while promoting health-beneficial bacteria such as Lactobacillus spp
Furthermore, immunological analyses demonstrated that B. coagulans DSM 32016 boosts the immune defense by enhancing animal IgA production.
The positive impact of TechnoCare®200 on performance and different health parameters was tested in one day old broiler chickens (Abor Acres, Thailand 2021).
The control group was fed a diet without TechnoCare®200, whereas the other group received the same diet but containing TechnoCare®200.
TechnoCare®200 successfully improved animal performance, including a +2% higher daily body weight gain (BWG) and a significant -1.4% reduction of the feed conversion ratio (FCR) (Figure 1).
B. licheniformis DSM 33806 has the property to directly suppress harmful gram-positive gut bacteria such as Clostridium perfringens. In this regard, it has been scientifically proven that TechnoCare® reliably lowers the risk of intestinal disturbances and associated serious diseases, including necrotic enteritis in poultry.
The extraordinary high concentration of Bacillus spores in TechnoCare® is a key factor for its outstanding success. They are also well-known for their storage stability and remarkable heat resistance, making TechnoCare® suitable for pelleting processes up to 95 °C. TechnoCare® can be used in premixes, mash, and pelleted feed and is compatible with other feed additives such as organic acids and coccidiostats.
Figure 1. Effect of TechnoCare®200 on performance, digestibility, production efficiency, and flock uniformity. DWG, daily weight gain; FCR, feed conversion ratio; CP, crude protein; GE, gross energy; EPEF, European production efficiency factor; FU, flock uniformity. *, p ≤ 0.05.
The improved feed conversion was reinforced by an increased feed digestion.
Broilers fed with TechnoCare®200 showed a +4% improvement of crude protein (CP) digestibility and were able to extract +3% more energy (GE) from ingested feed (Figure 1).
As result, broilers fed with TechnoCare®200 showed a +4% better European production efficiency factor (Figure 1), although no differences in livability were observed.
Furthermore, TechnoCare®200 significantly improved flock uniformity (FU) by up to +1.9 % (Figure 1), an important parameter when it comes to optimizing feed formulation or carcass processing and final sales.
Improved performance and feed conversion is strongly associated with intestinal health including an efficient gut microbiota as key factor.
Microbiological analysis of fecal samples at day 35 demonstrated the microbiota-modulating properties of TechnoCare®200
The supplementation of the probiotic led to an increase of health-beneficial bacteria such as Bifidobacteria (+43%) and Lactobacillus (+23%), whereas harmful bacteria such as E. coli (-26%), C. perfringens (-34%), and Salmonella (-19%) were clearly reduced in feces of the broilers fed with TechnoCare®200.
These data were reinforced by the health status of the intestinal epithelium determined by necrotic enteritis (NE) scoring.
In the first section of the small intestine, the duodenum, the dual-strain probiotic was able to significantly reduce necrotic appearance by -64% and thus improved gut integrity (Figure 2).
Also, other gut sections of broilers fed TechnoCare®200 had a better health status (Jejunum [-3 %] and Ileum [-6%]).
1.0E+06
0.0E+06
Improved intestinal health and digestibility have also the potential to improve fecal consistency, thus enhancing litter quality and foot health.
Related data indicated, independent of the sampling time (day 24 [-5%] or day 35 [-3%]), a better fecal score in birds that were fed TechnoCare®200 (Figure 3).
Likewise, the moisture content of the litter was reduced by -3% and -1% at day 24 and day 35, respectively (Figure 3)
Figure 2. Effect of TechnoCare®200 on intestinal microbial balance and NE Scoring. Ileal digesta was used for bacterial analysis. NE Scoring: 0 = No lesions; 1 = Thin intestinal wall; 2 = Few areas of necrosis; 3 = Moderate to severe necrosis; 4 = Fibrinous necrotic debris. *, p ≤ 0.05
The collective results suggest an improved litter quality which is known to support foot health of birds and lowers the occurrence of painful foot pad lesions.
Indeed, although none of the birds had severe foot pad lesions, initial discolorations were significantly less observed (-40%) at feet of broilers fed with TechnoCare®200 (Figure 3).
A healthy, productive livestock animal starts with taking care of its insides, the intestinal tract. TechnoCare® specially developed by Biochem is doing just that. The collective findings indicate the multifaceted beneficial properties of TechnoCare®200 with positive effects on:
Animal performance and feed digestibility
Flock uniformity and production efficiency
Microbial balance and gut integrity
Litter quality and foot health
Figure 3. Effect of TechnoCare®200 on fecal consistency, litter moisture and foot health. Fecal Scoring: 1 = Hard and dry; 2 = Firm and formed; 3 = Soft and moist; 4 = Soft and unformed; 5 = Watery liquid. Lesion Scoring: 0 = smooth, no lesion; 1 = very small and superficial lesion with slight discoloration over small surface. *, p ≤ 0.05.
This dual-strain probiotic is not only a reliable and well-proven environmentally friendly alternative to antibiotic growth promotors, but also suitable to maintain farm profitability in challenging times by taking care of your animals’ health and welfare.
TechnoCare® : Dual Power for Efficient Poultry Production DOWNLOAD ON PDF
A productive livestock animal starts with taking care of its insides, the gut. TechnoCare® is a powerful dual-strain probiotic developed to resolutely fight against harmful gut bacteria. It is a reliable alternative to antibiotic growth promoters while protecting animal health and improving performance for a more profitable animal production.
Contact us: info@biochem.net
biochem.net
OBJECTIVES
MATERIALS & METHODS
Natu-B4® is a polyherbal-based product that is recommended to replace choline chloride (CC) in swine feeds. Although it’s use and acceptance has increased over the years during the starter and grower phase, recent field evidence supports the benefits of Natu-B4® supplementation in the finisher phase.
This trial was conducted to validate the hypothesis that Natu-B4® could benefit pig performance during the last phases of growth.
Evaluate the effect of Natu-B4® supplementation in grower-finisher pigs on zootechnical performance.
» The study was conducted at Zootests. France.
» One hundred and seventy-six (176) male pigs (Topigs TN70 sows*Excelium boar) of 34 kg body weight (BW)
» They were allocated to 2 treatments with 4 replicates each
» The pigs were individually identified with RIFO tags for the trial and they were fed using Self Automatic Feeder (SAF) and Orinkers (SAO) during the trial.
» Daily measurements were taken on individual BW and feed intake per animal.
» The study was divided in two phases: phase one from 34 to 85 kg BW and phase two from 85 kg to slaughter.
Table 1. Description of treatments
Feeds were based on corn and soybean meal. The levels of crude protein, digestible lysine and net energy (NE) used during the study are presented in Table 2.
MATERIALS & METHODS
RESULTS
Age at 100 kg (days)
The statistical model used to analyze the results included the average response, the fixed effects of treatment and pen and the residual. All performed by the GLM procedure of SAS.
The utilization of Natu-B4® resulted in a significant reduction (p = .048) of 2.9 days in the time required for pigs to reach 100 kg (Figure 1). Additionally, the use of Natu-B4® showed a noticeable improvement (p = .078) in the daily growth rate of pigs at 100 kg (Figure 2).
The feed conversion rate (FCR) at 100 kg was not different between treatments (p >.05). However, the pigs fed with Natu-B4® presented a numerical advantage of 0,05 (5 points) in average in comparison to the control animals (Figure 3).
FCR at 100 kg
a,b Bars with different letters indicate a significant difference (p < .05).
Daily growth to 100kg
CONCLUSIONS
a,b Bars with different letters indicate a significant difference (p < .10).
a,b Bars with different letters indicate a significant difference (p < .05).
The findings of this study prove how the addition of Natu-B4® to the diet of pigs (at a rate of 250 g per ton) has the potential to enhance zootechnical performance during the finishing phase.
Moreover, it presents an economically viable alternative for reducing the overall cost of pig production.
Copyright©: 2007-2024 Nuproxa Switzerland Ltd. AII Rights Reserved. Any reproduction (total or partial) of this content without prior authorization is forbidden. Version update: January 13. 2023
Edgar O. Oviedo-Rondón Prestage Department of Poultry Science, North Carolina State University, Raleigh, NC
In this second article related to the new edition of the Brazilian Tables for Poultry and Swine (BTPS), we will continue describing the methodologies and equations used to determine energy and nutrient requirements for swine.
We recommend acquiring this publication as a reference for nutritionists. In the present summary, the citations of literature were omitted to simplify reading, but the complete documentation can be found in these new report of the BTPS.
Integrated factorial and empirical models were also used to estimate the nutritional requirements of pigs.
In this 5th edition, the genetic improvements impacted the growth curves with final heavier live body weights (BW) at similar age. These faster growth rates caused improvements in the feed conversion ratios.
The Gompertz equation was also used to describe growth. In this article, we will present some equations as examples of the process used to estimate the requirements. However, the BTPS contains more equations and multiple practical examples and values calculated for each growing or reproductive phase or condition.
Most of the equations depend on the BW of growing pigs, gestating gilts or sows, lactating sows, and piglets. Then, an accurate, practical, and expedited estimation of BW and the BW gain becomes even more important. Technologies like machine vision to predict the BW of individual pigs with more frequency may contribute to obtaining a better prediction of nutrient requirements.
The nutritional recommendations were estimated for herds of high genetic potential. This includes values for barrow, gilts, entire males up to 100 kg and immunocastrated from 100 kg onwards, and mixed-sex pigs of high and standard performance.
The daily metabolizable energy (ME) requirements were determined based on daily ME for maintenance (MEm) and for production (MEp) or growth.
The MEm (kcal/day) = 106*BW0.75 with BW expressed in kilograms. The MEp was obtained from experiments and the data included performance, feed intake, and ME intake by gender, age, and rearing phases.
Consequently, the ME requirements for males of high genetic potential can be estimated with the following equations:
MEentire males up to 105 kg = (106*W0.75) + (1782.4 + 71.426*BW –0.4552*BW2)*BWgain(kg/day)
MEimmuno-castrated males 105-155 kg = (106*W0.75) + 5875.5*BWgain(kg/day)
The entire males had lower requirements and feed intake than the immuno-castrated and two equations were adjusted to estimate daily ME requirements.
The requirements can be corrected for environmental temperature (T) with this equation 2.4*BW0.75*(TN – T) using the thermoneutral T (TN).
The TN was indicated in the text of the BTPS for each BW range from 15 to 30 kg pigs with 25 oC as TN, to finisher pigs of 100 to 130 kg with 21 oC TN.
This is the same correction indicated in the 4th edition of these tables.
The requirements for standard performance were calculated by increasing 3% the values estimated for high genetic potential animals.
The reasoning is the potential higher challenges in production, handling, environment, and health that may occur in those production systems.
The requirements of amino acids (AA) for growing pigs were calculated by determining first the need for standardized ileal digestible (SID) for lysine (SID Lys) with the factorial method.
The SID Lys for maintenance was established as 0.039 g of SID Lys BW0.75. The SID Lys for growth were estimated with a quadratic equation based on the results of multiple experiments.
Then, the following is the final equation to estimate SID Lys requirements for entire growing males with high genetic potential:
SID Lys (g/day) = (0.039*BW0.75)
+ (16.074 + 0.1531*BW –
0.0012*BW2)*BWgain(kg/day)
All other AA are estimated using ideal protein profiles for phases during growth as described in the text of these BTPS. Based on the SID Lys requirement crude and digestible protein can be calculated by dividing the value (SID Lys *100) by 6.2 or 7.0%, respectively.
The digestible phosphorus (P) requirements for entire and immunecastrated males can be estimated with the following equation:
0.046*BW0.75 + 5.42*BWgain (kg/day).
The available P requirement can be estimated as 0.046* BW0.75 + 5.68*BWgain (kg/day).
The total calcium requirement is recommended as 2.04 times the digestible P requirement or 2.10 times the P available requirement.
Requirements for sodium, chlorine, and potassium are estimated with quadratic equations as a function of BW.
The genetic progress of swine breeders to be more prolific was taken into consideration in this 5th report. The effects of parity order, the number of piglets born, and weight loss during lactation, among other factors were accounted for.
All nutrient requirements were established in amounts needed per day per animal for optimum performance.
Equations to estimate nutrients and energy considered for the gestation phase are the gilt or sow BW, the BW gain, the number of piglets, reproductive gain, mammary gland development, maternal growth, and recovery from BW loss during the previous lactation.
Requirements for the lactation phase included the litter size, BW, BW loss, litter BW gain, mammary gland involution, and BW loss during the weaning-to-estrus interval.
The maternal BW gain (kg/day) is obtained with the following equation: (0.01503 – 0.00011*average days) * total maternal BW gain (kg)
The reproductive BW gain (kg/day) labeled in the BTPS as RWG needs to be calculated for two periods 0 to 85 days and 86 to 115 days.
RWG 0-85 days (kg/day) = (0.000246 * average days in gestation) * Number of piglets.
RWG 86-115 days (kg/day) = (-0.0307 + 0.000726 * average days in gestation) * Number of piglets
For gilts and sows in gestation, the RWG information that describes the growth of the uterus, fetuses, fluids, placenta, and mammary tissue, helps to calculate the ME requirement using the following equation:
ME = 106*BW0.75 + 4915 * maternal weight gain + 1826*RWG
Four categories were used for the gestation sows: nulliparous, primiparous, secundiparous, and multiparous.
1 1 3 3 2 2 4
Three categories were used for lactating sows: primiparous, secundiparous, and multiparous.
The dietary feed intake was estimated using the ME equation and the dietary energy content to calculate the percentage of dietary nutrients.
The ME requirement (kcal/day) for lactation is calculated using the following equation:
ME (kcal/day) = 106*BW0.75 + 6230*litter BWgain - 4600*Female BW loss postpartum(kg/day)
A correction for environmental T was included when sows were housed at temperatures outside the TN range (14 to 26 oC) with this equation 2.4 * 60.976*(TN-T).
pigs
The SID Lys (g/day) requirement for gestation sows can be calculated using the following equation:
SID Lys (g/day) = 0.036*BW0.75/0.7 + 22.6* maternal weight gain + 22.6*RWG
In lactation, the SID Lys (g/day) requirement can be calculated as
SID Lys (g/day) = 0.036*BW0.75 + 23.6* litter BWgain – 7.0*Female BW loss postpartum (kg/day)
The total Lys requirement was obtained considering an average SID Lys digestibility of 88%. All other AA were calculated using ideal protein profiles for two periods during gestation from 0 to 85 days and 85 to 115 days, and one profile for lactation sows.
To formulate low crude protein diets where synthetic AA are included and nonessential AA can become limiting, a 35% ratio of Non-essential to Total Nitrogen (Ne: Total N) ratio was considered for the gestation phase and a 39% ratio for the lactation phase.
Examples in the text of the BTPS indicate how to make the calculations and the content of N for each AA used for this estimation.
We expect to motivate interest in exploring this 5th edition of the Brazilian Tables for Poultry and Swine. The application of the equations and practice will reveal the applicability and factors to improve through more research and control.
Minimum levels needed of dietary crude and digestible protein can be obtained by dividing the SID Lys (SID Lys *100) by 4.7 or 5.3, respectively, for gilts or sows up to 85 days of gestation.
For gestation between 86 and 115 days, the SID Lys values (SID Lys *100) should be divided by 4.5 and 5.0 to obtain crude protein and digestible protein (%) levels to use in the formulation.
Finally, for a sow in gestation, the SID Lys should be divided by 5.1 and 5.7.
A reduced feed intake is usually observed when pigs are exposed to heat stress during a long period of time.
As they have a limited capacity to evacuate the heat, and due to the thermic effect of feeding, they modify their feeding behavior to limit their body heat production.
Main physiological and metabolic consequences of heat stress are impaired intestinal barrier function, perturbations to the hormonal system, changes in thermoregulation responses...
The sensitivity of insulin, a key hormone involved in the regulation of multiple metabolic processes such as the energy metabolism, may also be affected under heat stress.
LEVUCELL SB is the specific probiotic yeast Saccharomyces cerevisiae boulardii CNCM I-1079. More than 50 scientific publications in monogastric feeding have documented its beneficial effects on gut health.
Under heat stress conditions, LEVUCELL SB helps maintain optimal gut barrier integrity functions and reduces the risk of pathogen endotoxin translocation into the blood stream with associated inflammation.
Supplemented pigs with LEVUCELL SB better cope with heat stress. Lactation performance are improved, and fattening pigs are more efficient converting feed into growth.
LEVUCELL SB EFFECTS UNDER HEAT STRESS
Thermoregulation response
Water intake
Body temperature
LEVUCELL SB BENEFITS
Maintenance of healthy gut functions
Metabolic response adaptations
Insulin sensitivity
Heat loss capacity increase
Pigs can better evacuate the heat
Adaptation of feeding behavior
LEVUCELL SB supplemented pigs eat more often with smaller meals for an increased daily feed intake
OVERALL BETTER ADAPTATION TO HEAT STRESS
Carolina Kyriacou, nutritional consultant from Vettaky LTD, Cyprus
MSc from the University of Wageningen, Netherlands
I am a dairy nutritionist in Cyprus, where the weather is among the hottest anywhere in Europe. Another fun fact about Cyprus is that in 2022 it was among the top 5 leading countries with the highest production of cheese (Figure 1).
Milk quality matters to cheese production and dairy farm profitability. Therefore, an important role for me on dairy farms is to develop cost-effective feeding strategies for high milk quality even under high temperatures in the summer season.
Let’s look at how adequate supplementation of trace elements in dairy rations can play an important role to alleviate the negative effects of heat stress on milk composition.
The temperature humidity index (THI) (Figure 2) has been established as an indicator for heat stress risk. Collective data from research has shown that milk production in dairy cows can be affected when daily average THI exceeds 68.
However, this also depends on the level of milk production in the dairy herd, as higher producing dairy cows are more susceptible to heat stress (Figure 3).
Recent studies show that modern high producing dairy cows become heatstressed at an average THI of 68. High producing dairy cows have a higher metabolic heat load due to processes such as lactogenesis and milk secretion.
Consequently, as milk production and metabolic heat production rise genetically, heat stress will increasingly limit the expression of genetic potential. High producing dairy cows are also more prone to oxidative stress, which can further be exacerbated by heat stress with enhanced consequences for milk yield and quality.
Calculating the THI can provide a good indication for the risk of heat stress in dairy cows at any given time.
However, what this value does not consider are differences in the type of farming system the cows are kept in. This means the real heat exposure the dairy herd experiences may differ from the calculated THI. Farming systems may or may not include cooling systems or shading. Thus, the real heat exposure may be lower than reported by nearby weather stations.
Heat stress not only reduces the milk production but also affects the quality of milk by altering various components of milk. Most studies aiming to quantify the effect of heat stress conditions on cow performance report a decrease in protein and fat percentage, as well as milk yield.
Many milk payment systems rely on milk quality traits such as fat and protein content to determine the price per unit milk. These traits influence coagulation and technological properties of milk and consequently, cheese production.
It is therefore of great interest to farmers to produce large volumes of highquality milk, particularly in countries in which the majority of supplied milk is directed to cheese production.
In those countries with a high proportion of cheese production prolonged periods with elevated THI will not only have detrimental effects at the farm-level, but also to the dairy industry at large.
Research has shown a general decline in milk fat levels as well as reduction in milk protein between the winter and summer months. Furthermore an increase in somatic cell counts (SCS) was observed during the warm months.
More recent research from Italy reported that milk composition is more sensitive to milder heat-loads than milk yield.
This means that even mild heat stress conditions could induce adverse changes in milk quality in dairy cows.
The authors concluded that if farms would take into account the effect of heat stress on milk quality on top of that of milk yield estimated economic losses of dairy farms under heat stress would be far greater than previously thought.
Milk fat content has been shown to decrease with heat stress in dairy cows. This can to some extent be explained by an increased risk of acidosis under those conditions. Factors that can contribute to rumen acidosis problems are a decreased dry matter intake with a lower proportion of forage and higher levels of fermentable carbohydrates, a slow down in rumination and a decrease in the amount of saliva reaching the gut.
Milk protein is reduced by heat stress. Many studies attributed this reduction to a decline in feed intake.
However, recent research revealed that decreased feed intake in response to heat stress only partially accounts for the reduction in both milk production and milk protein, suggesting additional mechanisms that disturb milk protein synthesis during heat stress.
Results of these studies suggest that oxidative stress as a result of heat stress contributes to a reduction in milk protein content.
The explanation for this is an increase in apoptosis (cell death) in mammary gland tissues that directly reduces the number of mammary epithelial cells. Furthermore elevated levels of free radicals also damage milk protein synthesis by regulating signaling pathways.
Dairy herds experience a seasonal increase in somatic cell counts (SCC) in milk during the summer months. SCC tends to rise with temperature and humidity levels during summer.
On the one hand as a result of cows lying down more frequently in the heat, increasing the risk for environmental mastitis because of the enhanced exposure of the teat end to bacteria.
On the other hand, heat stress increases the level of oxidative stress in cows, which again is known to reduce the resistance of cows to mastitis infections further exacerbating the risk for mastitis.
Heat stress stimulates the production of reactive oxygen species (ROS) in body cells. Oxidative stress occurs when there is an imbalance between ROS production and antioxidative defences in the cells.
In dairy cows oxidative stress results in a negative impact on the immune system, increased mastitis frequency, higher somatic cell counts in milk, a reduction in milk protein content and reduced milk production.
To prevent oxidative damage, animals are equipped with endogenous defence mechanisms including antioxidants, to counterbalance the effects of ROS, and these important compounds can be divided into enzymatic as well as nonenzymatic antioxidants.
Consequently, the supplementation of diets with antioxidants can help to enhance the antioxidative capacity oft the cow and alleviate the extent of oxidative stress. Dietary antioxidants include vitamins, trace-elements, polyphenols from plant extracts and carotenoids.
There is a considerable amount of evidence that trace minerals such as selenium (Se), zinc (Zn), copper (Cu), and manganese (Mn) have antioxidative properties and can help minimize the negative effects of oxidative stress.
The organic form of Se (selenomethionine and yeast Se) performs better than inorganic Se (sodium selenite or selenate). Supplementation of 0.3 ppm/kg DM of organic selenium was effective in reducing oxidative stress in cattle. Selenium was also shown to increase milk protein synthesis.
Supplementation of rumenprotected zinc-methionine at 0.131% of diet dry matter resulted in better oxidative status in lactating high producing Holstein dairy cows under heat stress compared to control cows. It further helped to maintain milk production and composition during periods of heat stress (THI 75).
Milk protein and fat levels were significantly higher in treatment cows vs. control. On top of that animals fed the rumenprotected zinc-methionine had significantly lower SCC during the experimental period than their counterparts in the control group.
There is no single approach to maintain high milk quality in dairy cows under heat stress conditions. It requires a holistic approach encompassing both management and nutritional strategies.
However, supplementing the dairy ration with a balanced mix of key trace elements with antioxidative properties can be a successful part of these strategies to help counteract the negative effects of heat stress.
References upon request.
Feeding for high milk quality in dairy cows under heat stress DOWNLOAD ON PDF
Beatriz de Magalhães Ceron, Breno Luis Nery Garcia Faculty of Veterinary Medicine and Animal Science, Departmento of Nutrition and Animal Production , University of Sao Paulo, Brazil
Considering that each protein synthesized by the animal organism is genetically determined and has a structure with a unique and invariable composition of amino acids (AAs), the requirement varies according to each AA, therefore, the balance of AAs in the diet of ruminants is essential (SCHWAB; WHITEHOUSE, 2021).
Out of the 20 AAs present in proteins, 9 are classified as nutritionally essential (indispensable), and one (arginine [Arg]) is considered conditionally essential.
Essential AAs cannot be synthesized in the body or are inadequately produced endogenously and must be obtained through the diet.
The essential AAs include: histidine (His); isoleucine (Ile); leucine (Leu); lysine (Lys); methionine (Met); phenylalanine (Phe); threonine (Thr); tryptophan (Trp); and valine (Val) (NASEM 2021). 1) 3) 5) 8) 2) 4) 7) 6) 9)
In the case of Arg, even though there is a significant level of de novo synthesis, for high-producing cows, synthesis is insufficient to meet the demand.
Consequently, Arg is traditionally considered an essential AA for ruminants. The other 10 AAs are
For ruminants, AA absorption occurs through three main pathways:
Microbial Proteins (MicP) synthesized in the rumen,
Undegraded Dietary Protein in the Rumen (UDR), and, to a lesser extent,
Endogenous proteins (SCHWAB; WHITEHOUSE, 2021).
Scientific evidence suggests that microbial protein generally accounts for over 50% of the flow of crude protein (CP) to the small intestine (SCHWAB; BRODERICK, 2017).
For a long time, it was assumed that MicP (synthesized in the rumen) combined with a portion of UDR (dietary protein escaping ruminal degradation) would fulfill all AA requirements.
This assumption was likely accurate for low-producing cows used in initial experiments (considering approximately 2,300 kg/year average milk production in the United States).
In a classic experiment using 15N-labeled ammonium sulfate for lactating cows, it was observed that 15 hours after feeding, all amino acids in milk proteins (separated by fractionation after hydrolysis) were labeled.
This demonstrated that MicP could provide all the necessary AAs for milk protein synthesis.
However, the labeling level differed among AAs, with higher labeling for non-essential AAs compared to essential AAs. It is assumed that there was a higher degree of labeling for non-essential AAs because these can be synthesized by the ruminant organism (especially in the liver from ammonia) and not solely from microbial protein, as is the case with essential AAs (VIRTANEN, 1966).
Considering the need to quantify microbial protein synthesis to meet the requirements of lactating cows, the same authors conducted a study by feeding lactating cows diets with urea nitrogen as the only nitrogen source, without protein sources.
Despite no negative impact on milk production or milk protein composition under the experimental conditions tested, there was a lower concentration of essential AAs in the blood plasma of lactating cows fed diets with all protein coming from non-nitrogenprotected (NNP) sources compared to cows in the control group (VIRTANEN, 1966).
Furthermore, it is worth noting that the rapid hydrolysis of urea into ammonia in the rumen may exceed the capacity of ruminal microbiota utilization. When there is an excess of nitrogen relative to energy in the rumen, unused ammonia is absorbed by the rumen epithelium, taken up by the liver via the portal system, and converted into urea for detoxification.
Urea produced from hepatic detoxification circulates in the blood and can be excreted in urine, recycled to the rumen, or diffused in milk, where it can be detected by measuring urea nitrogen in milk (HEIDARI et al., 2022; SCHWAB; BRODERICK, 2017).
Therefore, for the correct supply of AA requirements in the diet of dairy cows, a proper balance between UDR and NDR sources must be considered.
Considering the main absorption pathways, NASEM (2021) recommendations for each essential AA are calculated using a factorial approach, representing a quantitative evaluation based on the sum needed to fulfill the designated metabolic function of each AA.
For estimating post-ruminal flow of essential AAs, a factorial method based on predicting microbial protein, UDR, and endogenous duodenal crude protein is used. Individual AA flow considers the net supply of AAs and is calculated from the corresponding AA composition and digestibility of UDR and crude microbial protein.
In the case of endogenous duodenal proteins, although they contribute to duodenal protein flow, as they are synthesized by the organism itself and therefore do not constitute a new net supply of AAs, their contribution to metabolizable AA flow is not considered (NASEM, 2021).
Individual recommendations are provided for all essential AAs, with the sole exception of Arg. The approach used for AA recommendations follows that used for metabolizable protein, and a combined efficiency goal is used for each AA, assuming that energy requirements are adequately met (NASEM, 2021).
Current NASEM (2021) recommendations use a multifactorial nonlinear function with additive responses to independent variables, unlike the old linear representation model of the relationship between metabolizable protein or AA supply and milk protein production.
For this reason, it is not possible to define a fixed requirement for any of the determining variables. Instead, an infinite combination of variables can be associated with a particular production level.
Therefore, it is crucial to establish biological guidelines and limits to be followed.
Firstly, it is necessary to establish and quantify the direct net requirement for the supply of essential AAs, whether through protein secretion or protein accretion, indicating the essential AAs removed from the net base that must be replenished promptly. Then, an efficiency of utilizing the source of essential AAs to meet each function is assigned.
Recommendations are then calculated from the net protein requirement divided by the utilization efficiency.
In summary, recommendations for metabolizable protein should be used as a guideline only, with greater emphasis on balancing essential AAs individually. Diets that provide sufficient amounts of metabolizable protein may be deficient in one or more essential AAs, while diets with seemingly insufficient amounts of metabolizable protein may be balanced for essential AAs, and individual AA balance presents a better approach to milk protein production (NASEM, 2021).
Table 1. Example of Adequate Supply of Essential Amino Acids (EAAs) for a Lactating Cow with Graduated Milk Protein Production (values expressed as % of protein) and Composition of EAAs in Feeds and Rumen Microbiota
Requirement¹ expressed as a function of milk fat production (g/d)
Animal products
Ruminal Microorganisms
¹Example of Adequate EAA supplies for an empty multiparous cow (650kg BW), consuming 26kg/d of a diet with 34% NDF with graduated milk protein (values expressed as % of protein). Adapted from NRC 2021; ²Composition of EAAs in feeds and rumen microbiota. Adapted from (SCHWAB; WHITEHOUSE, 2021)
Among the essential amino acids whose supplementation is recommended in ruminants, lysine and methionine are the most limiting and, therefore, show the greatest productivity benefit from supplementation (SCHWAB; BRODERICK, 2017).
Lactating cows with high levels of milk production have increased requirements, which may need the use of synthetic essential amino acids (rumen-protected) for a complete amino acid balance (ALVES, 2004). Through supplementation, the absorption of AAs in the post-rumen is expected, requiring the escape of free AAs based on the administered dose.
Studies suggest that an increase in the dose of AAs infused into the rumen leads to a substantial decrease in ruminal degradation (ALVES, 2004; COTTLE; VELLE, 1989; SCHWAB; BRODERICK, 2017).
Additionally, supplementation with protected AAs results in the escape of a significant ammount of AAs from the rumen unchanged (ALVES, 2004; COTTLE; VELLE, 1989).
However, the commercial production of high-quality rumen-protected AA supplements is challenging (SCHWAB; BRODERICK, 2017).
Various approaches have been evaluated to physically protect lysine and methionine from the action of ruminal microorganisms (e.g., protection by lipids, often in combination with inorganic materials and carbohydrates as stabilizing agents). The most effective approach has been through a post-ruminal passage system and intestinal release associated with pH differences between the rumen and abomasum, independent of enzymatic function.
The method involves coating the AA surface with polymers resistant to enzymatic degradation but containing synthetic compounds sensitive to pH, insoluble in the ruminal environment, and highly soluble in the more acidic abomasum pH. Compared to other methods, polymer-protected AAs have a higher intestinal release coefficient (SCHWAB; WHITEHOUSE, 2021).
Derivatives of methionine and lysine are also an alternative for supplying these amino acids to ruminants. These refer to free AA analogs to which a chemical blocker has been added to the alphaamino group or the acyl group has been replaced by a non-nitrogenous group.
Derivatives of methionine and lysine are also an alternative for supplying these amino acids to ruminants. These refer to free AA analogs to which a chemical blocker has been added to the alphaamino group or the acyl group has been replaced by a non-nitrogenous group.
Studies evaluating the methionine analog 2-hydroxy-4-(methylthio) butanoic acid (HMTBa), the most studied analog for ruminants, indicate greater resistance of free HMTBa to ruminal degradation compared to free methionine.
Furthermore, free HMTBa has higher ruminal absorption and is converted into methionine in tissues.
However, no increase in methionine concentrations in blood plasma or milk protein concentrations in methioninedeficient cows supplemented with HMTBa was observed, and for this reason, the use of protected AAs remains the most effective alternative.
Supplementation with rumen-protected AAs requires, in addition to analyzing the content and cost of supplements, knowledge of AA absorption when provided according to the herd’s feeding program (SCHWAB; BRODERICK, 2017).
Knowing the ruminal degradability value is crucial for planning the supplementation of diets with synthetic AAs.
Considering this, estimates of bioavailability for supplementation with synthetic AAs must be accurate and reliable (SCHWAB; BRODERICK, 2017).
Analyzing the concentration of free AAs in the rumen, obtained from the proteolysis of diet protein sources, low observed concentrations indicate rapid degradation by ruminal microbiota. AAs obtained by proteolysis are incorporated by microorganisms for multiplication or are deaminated, producing ammonia nitrogen (ALVES, 2004)
In this sense, formulating lower-cost diets using synthetic AAs to meet the demands of high-production cows requires information on the bioavailability of AAs (SCHWAB; BRODERICK, 2017).
The stability of rumen-protected AAs should also be considered when mixed with other feeds in total ration supply, especially when there is high moisture content (SCHWAB; BRODERICK, 2017).
Ji et al. (2016) evaluated the interaction between diet ingredients and rumenprotected AA supplements during total ration preparation in two correlated experiments.
The first experiment aimed to assess the effect of ration moisture content on lysine supplementation, and the second experiment aimed to determine if mechanical mixing of the total ration alters lysine release in the rumen.
Six different rumen-protected lysine supplements were evaluated in both experiments. There was a significant increase in lysine ruminal release in one supplement and a tendency to increase in another after mechanical mixing of the total ration.
For all products tested, the exposure time to the ruminal environment altered lysine release, and a significant interaction between ruminal incubation hours and total ration mechanical mixing was observed for two products.
Additionally, exposure time of the supplements to the diet significantly increased lysine release for all evaluated products, with one product showing a significant interaction between exposure time and dry matter content, resulting in higher lysine release in diets with lower dry matter content.
The data suggest that the farm feeding program can alter the effectiveness of rumen-protected AAs, emphasizing the difficulty of developing stable AA supplements for ruminant nutrition.
Furthermore, the results reinforce that the evaluation of the bioavailability of rumen-protected AA supplements should also involve interaction with the total ration and the farm feeding program (JI et al., 2016; SCHWAB; WHITEHOUSE, 2021).
Supplementing essential amino acids in the diet of lactating cows aims to meet the demand and improve the amino acid balance when dietary protein sources are insufficient (SCHWAB; BRODERICK, 2017).
Effects on milk production, milk protein synthesis (BRAKE et al., 2013), weight gain, and the immune response of dairy cows are observed through supplementation (ABREU et al., 2023).
We share a series of studies conducted to evaluate the correct supplementation of AAs in dairy cows and its effects on productive parameters.
1Brake et al. (2013) evaluated the inclusion of a product containing methionine mixed with soy lecithin and added to mechanically extracted soybean meal (meSBM-Met) in milk production and composition and plasma methionine concentration of lactating cows. The meSBM-Met was compared to rumen-protected methionine supplementation (RPMet) and the control in a Latin square design experiment (5x5; 14 days per treatment, 10 adaptation days, and collections on the last 4 days).
25 multiparous Holstein cows (630 ± 54 kg body weight, 44.9 ± 7.0 kg milk/d, and 87 ± 28 DIM) were used.
Cows were blocked by DIM and distributed into five groups:
inclusion of
inclusion of meSBM-Met
inclusion of
High inclusion of RPMet, according to table 2
Individual milk production per animal was recorded, and milk samples were collected for composition on the last 4 days of each period. Urine and feces samples were collected twice a day on the last 4 days of each period to determine N balance, and plasma samples were collected for AA concentration determination on day 14 of each period.
In vitro methionine degradation determination was also performed for each treatment using ruminal fluid samples from two cows.
Plasma methionine concentration increased linearly with higher RPMet-supplemented amounts, but not when methionine was provided by meSBM-Met.
In vitro methionine degradation results suggest extensive ruminal degradation of methionine with meSBM-Met supplementation, leading to insufficient metabolizable methionine supply. A higher true milk protein concentration was observed when methionine was supplemented as RPMet compared to meSBMMet.
Despite this, N intake and retention did not differ between treatments, contributing to similar amounts of N excreted in urine and feces.
No difference was observed between diets in dry matter intake, apparent digestibility, and average milk production.
In this regard, milk protein content was the production parameter that responded best to the increased supply of metabolizable methionine, making it the most significant effect of supplementation.
Similar to the findings of Brake et al. (2013), an increase in milk protein production was also observed by Socha et al. (2005) in a study evaluating methionine and methionine and lysine supplementation in rumen-protected form in postpartum and early lactation cows.
The study was conducted in a randomized block design, with 84 Holstein cows blocked by calving date and randomly assigned to three diets:
A B C
basal diet (control);
basal diet supplemented with 10.5 g of methionine (Smartamine M)
basal diet supplemented with 10.2 g of methionine and 16.0 g of lysine (Smartamine ML).
In addition to the increase in milk protein production, an increase in energy-corrected milk production and milk fat was also observed in the diet with methionine and lysine supplementation.
It is important to note, however, that in both studies, essential amino acid supplementation occurred in basal diets that met protein requirements, and supplementation might not necessarily have the same effect in low-protein diets.
To understand the effect of limiting essential amino acid supplementation in low-protein diets, Van den Bossche et al. (2023) assessed the supplementation of methionine, lysine, and histidine in lactating cows’ nutrition.
The experiment involved 39 lactating Holstein cows (22 primiparous, 17 multiparous; 638 ± 73.2 kg body weight, average milk production of 32.7 ± 5.75 kg, DIM of 116 ± 29.3).
The experimental design consisted of a 4-week depletion period (control), followed by a 7-week treatment period, and again a control (a 5-week crossover period).
In the first phase, all cows received a control diet (hypoproteic; 14.5% CP), and after this period, cows were blocked by milk production, number of lactations, and plasma histidine concentration (collected along with milk samples on the last day of the pre-experimental period) and randomly assigned to three different diets during the experimental period (7 weeks):
ab c
control diet (n = 13)
control diet supplemented with protected methionine and lysine (MetLys, n = 13);
control diet supplemented with protected methionine, lysine, and histidine (MetLysHis, n = 13).
No effect of supplementation was observed on dry matter intake or productive performance, despite additional supply of dMet, dLys, and dHis evident in the bloodstream.
The authors point out that the observed result may possibly be related to an overestimation of dietary AA supply at the beginning of the experiment, leading to methionine and lysine deficiency despite supplementation affecting the efficiency of AA utilization for milk protein production and synthesis.
Chemical Composition⁹
¹Mechanically extracted soybean meal with added Met during manufacturing. Based on the manufacturing process, low = 3.8 g Met/d total, and high = 7.6 g Met/d total. Based on product analysis, low = 2.7 g total Met/d, and high = 5.3 g Met/d total; ² Mechanically extracted soybean meal with soy lecithins added during manufacturing. Contained by analysis (% DM): Asp, 5.05; Thr, 1.71; Ser, 2.37; Glu, 8.02; Gly, 1.89; Ala, 1.94; Val, 1.97; Ile, 2.23; Leu, 3.41; Tyr, 1.38; Phe, 2.25; His, 1.15; Lys, 2.59; Arg, 2.73; Cys, 0.62; Met not detected; ³ Mechanically extracted soybean meal with Met mixed with soy lecithins and added during manufacturing, containing by analysis (% DM): Asp, 4.95; Thr, 1.70; Ser, 2.32; Glu, 7.88; Gly, 1.87; Ala, 1.92; Val, 1.97; Ile, 2.22; Leu, 3.38; Tyr, 1.29; Phe, 2.24; His, 1.12; Lys, 2.47; Arg, 2.67; Cys, 0.59; Met free, 0.23; ⁵ Calcium soap of long-chain fatty acids (Church and Dwight Co., Princeton, NJ); ⁶ Rumen-protected Lys providing 16.2 g/d of metabolizable Lys (Kemin Industries Inc.; product assumed to contain 21% metabolizable Lys); ⁷ Containing 96% NaCl, 0.35% Zn, 0.2% Fe, 0.2% Mn, 0.03% Cu, 0.007% I, and 0.005% Co; ⁸ Containing 2.58% Zn as Zn-Met; 1.43% Mn as Mn-Met; 0.90% Cu as Cu-Lys; 0.18% Co as Co-glucoheptonate (Zinpro Corp., Eden Prairie, MN); ⁹ Providing in diets (based on DM) 3,300 IU vitamin A/kg, 2,250 IU vitamin D/kg, 35 IU vitamin E/kg, and 0.06 mg Se/kg; ¹⁰ Calculated based on analyses of samples from the basal mixture, meSBM, and meSBM-Met; ¹¹ Estimated from the NRC model (2001). Values for diets containing meSBM-Met were not calculated because ruminal escape and intestinal digestion of escaped Met were unknown.
In addition to productive parameters, the supplementation of essential amino acids (AA) also has an effect on the immune response and, consequently, on the health of dairy cows.
In order to evaluate the effect of rumen-protected Met and Lys supplementation in a low forage diet on milk production, composition, and mammary gland health, Abreu et al. (2023) conducted an experiment on a commercial herd of 4800 lactating cows (11,200 kg/cow [305-day lactation]).
Three hundred and fourteen mid-lactation multiparous cows were randomly allocated to two groups:
Acontrol (107 g of dried distillers’ grains [DDG]); n = 152;
B
RPML (107g of DDG supplemented with Smartamine ML [44% L-Lys; 15% D-Met] and 2.2 g of Smartamine M [n = 75% D-Met]); n = 162.
Blood samples from 22 randomly selected cows were collected to determine the concentration of AA, minerals, and urea nitrogen in plasma.
Additionally, daily milk production (per cow) was measured, and milk samples were collected for composition evaluation (total solids, fat, true protein, lactose, and milk urea nitrogen) and somatic cell count (SCC). A higher plasma concentration of Met and Lys in the RPML group was observed when expressed as a proportion of essential AA and total AA.
There was no observed effect of supplementation on milk production or composition; however, there was an effect on mammary gland health.
Cows in the RPML group had 0.39 times less risk of clinical mastitis than cows in the control group, indicating the impact of essential AA supplementation on the immune system and inflammatory response, possibly indicating a relationship between Met and Lys in the innate immune system response to intramammary infections.
Meeting the requirements of essential AA is fundamental for the optimal productive performance of cattle. Therefore, it is crucial to balance the diet appropriately to meet the requirements of Met, Lys, and His. Properly supplying the requirements of essential AA in the diet of dairy cows should consider the appropriate balance between RUP and RUPN sources.
Furthermore, supplementation with rumen-protected synthetic AA can be an alternative for diets of high-production cows. However, technologies for supplement manufacturing have been a challenge, and for the inclusion of these products in the diet, attention must be paid to the stability and bioavailability of AA.
The results of adequate essential AA supplementation include an increase in milk protein production and improvement in the cows’ immune system response capacity.
References upon request.
Amino acids in the feeding of cattle (Part 2) DOWNLOAD ON PDF
Kebreab, E.,*1 J. Mendez,* P. Ji,† J-J. Lee,‡ and S. Seo§
*Department of Animal Science, University of California, Davis, CA 95616
†Department of Nutrition, University of California, Davis, CA 95616 ‡CTCBio Inc., Seoul, Republic of Korea
§Department of Animal Biosystem Sciences, Chungnam National University, Daejeon 305-764, Republic of Korea
In dairy production systems, feed constitutes the greatest cost and profitability of the operation depends on managing the inputs into the system.
Improving feed conversion efficiency (FCE) not only enhances the viability of a dairy operation but it also reduces excess nutrient excretion into the environment.
Although exogenous enzymes have been used routinely in nonruminants to improve nutrient utilization, their use in dairy diets has been limited mainly due to concerns that such enzymes would be ineffective due to hydrolysis in the rumen (Adesogan, 2005).
Several studies have investigated the effectiveness of β-mannanase as an exogenous enzyme supplemented in livestock diets.
However, ruminant diets typically contain relatively greater amounts of forages which contain 70-80% as insoluble cell wall (Van Soest, 1994). This has the potential to be digested through enzymatic activity.
In addition, the dairy industry continues to integrate plant based by-products as part of the animals’ diet to reduce cost, which increases the potentially enzymatically degradable fiber content of the diet.
Fibrolytic enzymes such as β-mannanase hydrolyze non-starch polysaccharides in plant cell walls by targeting plant cell walls.
The polysaccharide β-mannan is an important component of the plant cell walls and includes pure mannan, glucomannan, galactomannan, and galactoglucomannan (Moreira and Filho, 2008).
In nonruminants increases in digestibility of minerals, CP and OM has been reported through reduction of digesta viscosity and increased enzymatic activity in the intestine in swine (Lv et al., 2013).
Supplementation of β-mannanase in broilers was reported to increase FCE, and mitigated ileal viscosity (Lee et al., 2003).
There has been relatively little research conducted on the efficacy of β-mannanase in ruminants.
Supplementation of exogenous β-mannanase increased overall growth, FCE, and nitrogen (N) utilization in goats (Lee et al., 2014) and increased average daily gain in growing beef heifers (Seo et al., 2016).
Tewoldebrahan et al. (2017) investigated the effects of exogenous β-mannanase in dairy cattle fed corn silage and alfalfa hay-based diet.
The authors reported that cows receiving β-mannanase increased milk yield per unit of DMI and milk protein yield per kilogram of CP. They also reported that the percentage of N intake partitioned to urinary N tended to be greater in supplemented cows, perhaps indicating that more N was absorbed than could be supported for milk synthesis.
Our hypothesis was that if cows were fed reduced amount of CP and supplemented with β-mannanase, N use efficiency would further increase resulting in reduced feed cost per unit of milk production.
Therefore, the objective of this study was to determine the effects of exogenous β-mannanase supplementation on feed conversion efficiency (FCE), and milk yield in cows fed relatively low CP levels compared to a standard diet.
The study was conducted at the Dairy Unit of the Animal Science Department’s Teaching and Research Facility at the University of California-Davis.
Twelve post peak-lactation multiparous Holstein cows with an average of 643± 48 kg BW, 40.5± 3.6 kg/d milk yield, and 128± 9.64 DIM were assigned to 3 treatments in a 3-period crossover design, where treatment sequences were balanced using 3 × 3 Latin squares to mitigate possible carryover effects.
The treatments were:
high crude protein (16.1% CP, HCP) TMR diet,
low crude protein (14.6% CP, LCP) TMR diet, or LCP diet supplemented with commercially available β-mannanase enzyme (LCPE) at 0.1% of concentrate DM.
The β-mannanase enzyme (CTCZYME, patent 100477456-0000, CTC Bio Inc., Seoul, South Korea) is a natural enzyme produced by Bacillus subtilis (WL-1).
Kweun et al. (2004) reported that the enzyme had a pH optimum at 6.0 and a temperature optimum at 55oC.
The activity of the enzyme was estimated to be 800,000 U/kg at pH 4.0 and 30oC (Kim et al., 2013). The dose was determined based on the study by Teweldebrhan et al. (2017).
The enzyme was offered to the animals by hand mixing it thoroughly with corn silage first and then mixing the corn silage with rest of the feed.
Each experimental period was 18 d consisting of 14 d adaptation in freestall barn, 1 d adaptation to metabolic stalls and 3 d total collection of urine and feces from cows housed in metabolic stalls individually. Cows were fed twice daily at 0800 and 2000 h at 110% of previous day intake.
Milk yield and composition, and DMI of individual animals were measured. All animals had ad libitum access to water.
Offered feed and refusals by individual cows were weighed and recorded daily.
Feces from individual cows were sampled daily in each period.
Individual feed ingredients were sampled just before (on d 13) and at the end (on d 18) of sampling in each period.
Feed ingredients and feces samples of individual cows were pooled across days within each period and stored at –20 oC until analyzed for chemical composition.
Cows were milked twice daily and samples were collected daily from individual cows and analyzed for milk fat, protein, lactose, milk urea N (MUN) concentrations and milk somatic cell count (SCC).
Daily feces and urine output by individual cows (kg/d) in metabolic stalls were measured on d 16 through to d 18 as described by Teweldebrhan et al. (2017).
It was assumed that digestion and metabolic responses to enzyme would reach homeostasis after a 2 wk adaptation period.
Feces from individual cows were scraped with long hoe scrapers into a plastic tray assigned to each cow before they stepped or rest on it.
The individual feces trays were weighed and a representative fecal sample (100 to 150 g) was drawn every 3 h during the metabolic trial period.
Urine from each cow was collected, sample collection begun after a 24 h acclimatization period.
All urine cans were weighed, sampled and emptied every 3 h. A 5 ml of urine was pipetted into a 250 ml plastic bottle containing 6.5 ml of 2 mol/L hydrochloric acid at each time of sampling. Acidified urine samples were stored at –20oC until analyzed for total N concentration.
Feed ingredients and feces samples were analyzed for DM, CP, NDF, ADF, lignin, starch, total ash and individual mineral contents.
A linear mixed model in R was fitted to determine the effect of β-mannanase supplementation on FCE (milk yield:DMI), DMI, BUN, and somatic cell count with the lmer function. The models were fitted according to Tempelman (2004) for Latin square design.
Details to the analytical methods and statistical analysis upon request.
Dry matter intake was not affected by reduction in dietary protein levels or β-mannanase supplementation, however, there was a tendency for cows in LCPE to have lower DMI compared to HCP treatment (P = 0.068).
In agreement with previous studies that supplemented either β-mannanase (Teweldebrhan et al. 2017) or other exogenous enzymes such as xylanase (e.g., Romero et al. 2016), we did not observe significant differences in milk yield, milk composition, and milk component yields.
However, there was a tendency for greater milk yield in cows supplemented with β-mannanase compared to those on LCP diet (P = 0.09).
Cows on HCP diet had significantly greater milk urea N (MUN) compared to those on LCP and LCPE diets.
Spek et al. (2013) reported that dietary protein level is one of the factors that affect levels of MUN. In our study there was no significant difference in MUN between cows receiving the two low CP diets (P = 0.28) confirming that this is largely due to differences in dietary protein levels.
The somatic cell count in milk was significantly lower in cows receiving LCPE treatment compared to LCP (P = 0.0038) and HCP (P = 0.045) treatments.
The results are in agreement with Teweldebrhan et al. (2017) who also found that supplementation of exogenous β-mannanase to shift cows from close to sub-clinical mastitis to normal conditions (97,000 vs 111,000 vs 59,000 cells/ml for cows consuming HCP, LCP and LCPE diets, respectively).
Dry matter, OM and nutrient digestibilities were not affected by treatment. Teweldebrhan et al. (2017) reported the apparent total tract digestibility of nutrients were not affected in cows supplemented β-mannanase at 0.2% DM but observed lower DM, OM and CP digestibility in cows supplemented at the lower dose.
Feed conversion efficiency was calculated as the ratio of milk yield:DMI.
Efficiencies of converting dietary CP and AACP into milk protein and the efficiency of apparently absorbed OM into milk yield was calculated.
Cows receiving the LCPE compared to HCP diet improved FCE by 190 g/kg DMI (13.4% improvement, P = 0.003).
Compared to cows on LCP diet, those on LCPE also had a significant (P = 0.014) increase in FCE (160 g/kg DMI; 11.0% improvement).
Therefore, the increase in FCE was not only due to reduction in dietary CP content but also due to β-mannanase supplementation.
Although there was no significant differences in efficiency of utilization of apparently absorbed OM, cows receiving the LCPE diet tended to have greater efficiency (P = 0.10).
Cows receiving LCPE diet used dietary CP more efficiently to synthesize milk protein compared to cows on HCP diet (milk protein:CP intake = 0.34 vs. 0.30, respectively).
In this study greater milk N efficiency was achieved through enzyme supplementation partly due to a significant improvement in conversion of AACP to milk protein (0.48 vs. 0.56 for cows fed HCP vs. LCPE, respectively; P = 0.017). There was also a tendency for cows in LCPE to have greater AACP to milk protein conversion compared to cows on LCP diet (P = 0.10).
Improved efficiencies of LCP and LCPE fed cows can partly be due to the possibility that reduced CP caused protein to be supplied close to the requirement avoiding extra energy expenditure for excreting excess N.
Reed et al. (2017) calculated that the metabolic cost of excess N excreted as urea could be 52 to 68 kcal/g N excreted. Therefore, cows on LCPE and LCP diets could spare energy for milk production compared to HCP fed cows.
Teweldebrhan et al. (2017) speculated that the improved milk production efficiency can also be linked to a potentially lower immune response level in the mammary glands indicated by low SCC that can also have a favorable impact on energy efficiency.
As expected, there was a significant reduction in total N intake in cows fed LCP and LCPE diets compared to HCP diet because DMI was not affected. Similarly, because CP digestibility was not affected, the relative reduction in N excreted in feces also reflected the reduced CP intake levels.
Urine N levels were significantly lower in both diets with low CP intake compared to cows fed HCP diet.
The reduction in urine N excretion has a considerable impact on the environment because urine N is more susceptible to leaching than volatile losses of fecal N (Dijkstra et al., 2013b).
On average, 25% of the N excreted in manure (Hristov et al., 2011) and 3 to 15% of urine N in the field or 4 to 52% from urine patches (Oenema et al., 2008) may be lost as ammonia depending on soil type, moisture, temperature, wind speed and urine N concentration and urine composition.
Urine is the main source of volatile N emissions, therefore, manipulating the route of N excretion is an important mitigation tool for nitrous oxide which is a greenhouse gas with a global warming potential 265 times that of carbon dioxide over a 100year horizon (IPCC, 2013).
Proportionally more N was incorporated into milk in LCP and LCPE compared to HCP fed cows. Teweldebrhan et al. (2017) speculated that β-mannanase supplementation may favor amino acid partitioning to mammary gland as β-mannans, the substrates of β-mannanase, have been shown to inhibit insulin secretion in non-ruminants (Morgan et al., 1985; Leeds et al., 1980).
However, supplementation of β-mannanase did not change total N excreted in manure (LCP vs. LCPE treatments).
It did also not affect manure P output, although exogenous β-mannanase has been shown to reduce manure P output in growing pigs (Lv et al., 2013).
The most effective strategy to reduce N excretion and limit impact on the environment is to decrease the dietary CP content (Castillo et al., 2000; Kebreab et al., 2001; Spek et al., 2013).
Feeding a diet that contains CP above the requirement is inefficient because energy must be used to synthesize urea that is excreted in urine (Reed et al. 2017) and is costly to producers because excess N is excreted in manure.
Reduction of N intake decreases the amount of N excreted as demonstrated in our study and may improve N use efficiency (Kebreab et al., 2010).
Furthermore, as milk production per cow increases, N use efficiency has also been reported to increase with concomitant reduction in N excretion per unit of product (Capper, 2011).
In our study cows maintained their level of milk production with reduced dietary CP when supplemented with β-mannanase.
Finally, there is a potential economic benefit to producers in reducing dietary CP concentration and supplementation of β-mannanase.
Using the 2018 feed prices in California, we calculated the cost of feed to produce the same amount milk to be $9.41, $8.65 and $8.38/cow per day for cows fed the HCP, LCP and LCPE diets, respectively.
Therefore, there is a potential daily savings of $1.03/cow during lactation through reducing dietary CP and supplementing β-mannanase.
Supplementing β-mannanase to corn silage and alfalfa hay based diet increased milk yield intensity (i.e., milk yield per kg of DMI and milk protein yield per kg of CP intake) while reducing somatic cell counts in milk of midlactation Holstein dairy cows.
The improvement in FCE is above and beyond that can be gained by reducing dietary CP concentrations.
Supplementation of β-mannanase at 0.1% of concentrate DM did not have a significant impact on milk component yields or composition, however, milk urea N was significantly lower due to reduced N intake.
Apparent total tract digestibility of DM, OM, CP, ADF, NDF, starch and ash were not affected by treatment.
Supplementation of exogenous β-mannanase to low protein diets improved feed conversion efficiency in lactating dairy cows
DOWNLOAD ON PDF
Dietary N intake was reduced in cows fed a diet with lower dietary CP content, which was reflected in lower fecal and urinary N output.
The reduced N in urine has environmental and health benefits because urine is the main source of volatile N emissions such as ammonia and nitrous oxide
Further reduction in dietary CP concentration, perhaps in conjunction with rumen protected essential amino acids maybe possible. Therefore, more research needs to be conducted to investigate how much lower CP we can feed cows without compromising milk yield or animal health.
Our study indicated that it is possible to improve FCE and reduce dietary CP content and somatic cell counts without compromising milk production.
This research was supported by CTC Bio Inc, Seoul, South Korea. The authors also acknowledge Sesnon Endowment fund of University of California, Davis. The authors are grateful to assistance provided by Doug Gisi and several undergraduate interns (Department of Animal Science, University of California-Davis, Davis, CA).
References and result tables with details available upon request.