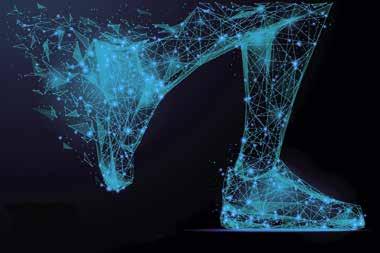
12 minute read
4 page CPD article
Essentials of Biomechanics
Principles of gait: Using the Acceleration Lever System Effectively
Advertisement
By Andy Horwood
Visiting Lecturer & Fellow Staffordshire University Product Designer & Research at Healthy Step Ltd
INTRODUCTION
Levers are simple machines that can be used to move loads. Every lever system needs a relatively solid beam that can rotate around a fulcrum, which in biomechanics, means an instantaneous joint axis. Remember joint axes change position with joint motion for they are not stable points of rotation but momentary and instantaneous, constantly moving with changing joint positions. Long bones make perfect levers for animal motion, as they form a single long, narrow structure that provides good rigidity with very limited compliance to permit bending deflection. The more deflection within a beam, the more effort power lost within the lever system. For a beam to move or resist motion it requires effort. In biomechanics this derives from muscular contractions that are usually interacting with gravitational forces to create or resist motion. Finally, there is the resistance or load to move. For lower limb motion, this consists of either proximal or distal body masses, derived from either segments within the lower limb or above it from the head, arms, and trunk. Most levers used within the lower limb consist of long bones acting as beams within class three lever systems to create flexion or extension motions at low metabolic costs, and yet they are using lever arm mechanics that compromise mechanical efficiency. Heel lift is different for several reasons.
Complications in Using the Foot as a Beam
The foot does not make a simple beam. This is because it is constructed from multiple bone components between the ankle and metatarsal phalangeal (MTP) joints, only five being long bones (metatarsals). Thus, to function in a beam-like manner requires the use of soft tissues to create stability across the foot’s articulation. A disproportionate amount of metabolic energy is used during the acceleration phase compared to other periods of human gait (Gottschall and Kram, 2003; 2005). This tells us that heel lift and early terminal stance is energetically more costly and therefore more difficult to perform compared to loading response, midstance weight transfer, or swing phase. There could be a good reason for this. Using a single long bone, such as the femur or tibia, to replicate a beam is relatively easy as these are biologically stiffened structures ideal for the purpose. Under loading, long bones are subjected to bending moments, which tends to focus different stresses to different surfaces. When beams are bent downwards a deformation occurs, known as ‘sagging’ deflection. This causes tension forces to focus on the inferior surface of the beam, while compression forces focus onto the superior surface. In the middle will be a neutral zone where neither tension nor compression exists. When beams are deflected upwards, known as ‘hogging’, forces reverse with tension now focused onto the superior surface (see figure 1 overleaf). Having a highly erect posture, human lower limb long bones avoid most of these sagging and hogging deflections because they are primarily loaded in a vertical superior-inferior direction, more like a column than a beam. However, this tends to lead to anterior-posterior and medial-lateral bending stresses instead.
Figure 1: When a beam is loaded horizontally it will either bend downwards (sagging deflection - left image) or deform upwards (hogging deflection –right image) depending on the direction of the load. This deflection direction effects where compression and tensional forces are concentrated.
Image from the upcoming text ‘Clinical Biomechanics in Human Locomotion: Origins and Principles’, with permission of www.healthystep.co.uk).
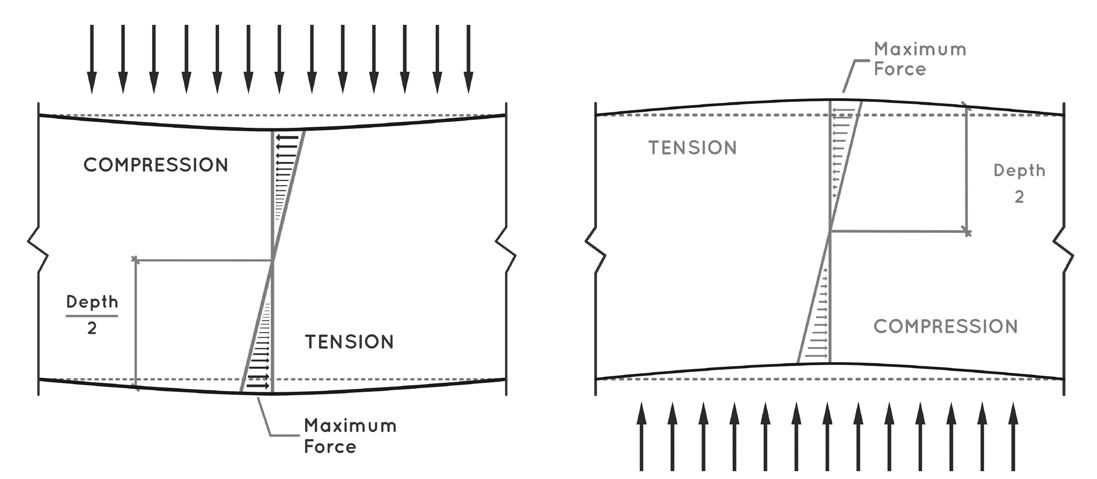
The foot is not made of a single long bone beam. Modelling the foot as a solid structure dramatically underestimates the power dissipated within the foot and overestimates the power derived from the foot during gait (Farinelli et al, 2019). This is important because it tells us that a structure with twenty-eight bones (remember the two sesamoids) is not going to be rigid at any point during gait. Indeed, it is important that the foot can dissipate energy passing through it by permitting deformation and motion within it. As a structure supplied with (usually) twenty-five articulations (from ankle to the MTP joints), some ten extrinsic muscles, around nineteen intrinsic muscles, and an extensive network of strong ligaments and fascia, the foot certainly possesses the capacity to provide structural property adaptability. For the application of acceleration power, the foot needs to behave as much as it can as a single rigid structure running from the ankle to the MTP joints. During late midstance and at heel lift, the foot is lying horizontally over the support surface, with body weight driving down above and under the influence of the ankle dorsiflexion moment. Therefore, it is subjected to sagging deflection at the all-important heel lift boundary. Thus, the dorsum of the osseous foot is subjected to compression while the plantar foot is exposed to tension strains before and as the heel starts to lift. How well it copes and resists the sagging deflection depends on how stiffened the foot is at heel lift, remembering that the healthy foot is going to be in some degree of a semi-rigid state, never totally rigid.
How Heel Lift Occurs in the Healthy Foot
Let us consider how the foot is behaving towards the heel lift boundary during the normal compliance-stiffness cycle of the walking stance foot. During late midstance, the midfoot starts to stiffen due to the fall under gravity of the body’s mass over the foot at the ankle as part of the inverted pendulum’s motion (Horwood, 2021). As forces on the foot start to rise as a percentage of body mass, the foot stiffens exponentially (Stolwijk et al, 2014; Bjelopetrovich and Barrios, 2016; Takabayashi et al, 2020). Forces at heel lift tend to peak at around 120% (1.2x) of body weight. This exponential stiffening under higher loading is the classic behaviour of a viscoelastic structure. These are properties which all biological tissues constructed from collagen conform to.
However, both intrinsic and extrinsic plantar muscle become increasingly active throughout late midstance (Ferris et al, 1995, Kokubo et al, 2012, Murley, et al, 2014; Farris et al, 2019), providing effort that is compressing the midfoot articulations together. This active stiffening compliments the passive connective tissue stiffening to create an adaptable level of stiffness across the vault of the foot in all three orthogonal planes.
The foot reaches its peak of pronation-induced vault depression (sagging deflection / lowest profile) at the heel lift boundary (Hunt et al, 2001). This should have induced maximal passive stance phase vault stiffness. Foot stiffening muscular actions of tibialis posterior and peroneus longus (Kokubo et al, 2012) also reach their peak activity at the heel lift boundary (Murley et al, 2014).
Thus, the mobile midfoot should achieve a semi-rigid state so that it can act as an acceleration beam to induce heel lift via energy applied from the Achilles, rather than providing a zone of mobility that dissipates plantarflexion power. During late midstance, the Achilles has become increasingly loaded with energy derived from the breaking activity of triceps surae acting on the body’s centre of mass (CoM) while it is rotating forward under gravity via ankle dorsiflexion. This action in turn has been increasingly moving the centre of the ground reaction force (CoGRF) to the forefoot, while applying a dorsiflexion moment across the midfoot. The ankle and midfoot dorsiflexion moments are forcing the vault to depress under sagging deflection, stretching and widening connective tissues such as the plantar ligaments and plantar aponeurosis. This process should stiffen the foot vault to help resist this sagging deflection passively, while the plantar muscles assist in an adaptable manner. As energy storage rises within the Achilles, a point is reached where the elastic power inside the Achilles is greater than the body mass keeping the heel on the ground. At that point, the heel ‘pops’ off the ground under the elastic recoil power of the Achilles, generating a powerful ground reaction force (GRF) under the forefoot (see figure 2).
Figure 2: Heel lift uses a biomechanically rare class two lever-like system to initiate acceleration. The effort (E) is further from the fulcrum (F) than the resistance (R). This is probably because myofascial fibre shortening occurs via elastic recoil, rather than via active muscular contraction, which would be metabolically expensive. The ankle plantarflexion effort provided by Achilles tendon elastic recoil requires midfoot stiffness and ankle and midfoot plantarflexion, to apply power to the forefoot as a large ground reaction force (black arrows). The upper body’s centre of mass (CoM-black circle) falls forward under gravity and the centrifugal forces of the swing limb, but heel lift adds extra momentum to opposite heel contact. Loss of midfoot semirigid stability loses any semblance of an efficient class two lever at heel lift.
Image from the upcoming text ‘Clinical Biomechanics in Human Locomotion: Origins and Principles’, with permission of www.healthystep.co.uk).
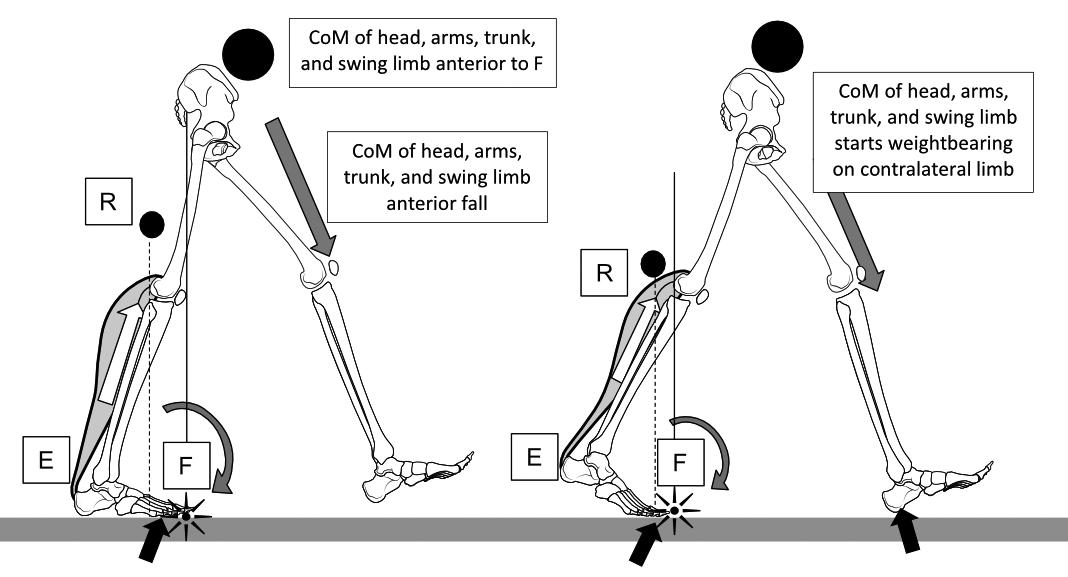
Safe Heel Lift
Vault depression (pronation) during single-limb support of midstance achieves three primary roles: that of increased contact surface area and thereby reducing peak plantar pressures (the mobile adaptor), that of energy dissipation and storage via tissue deformation, and that of passive foot stiffening. By passively stiffening the foot under increasing loads during late midstance, while also providing energy dissipation and storage, the foot vault creates a highly energetically efficient way of supplying a number of essentials roles for safe gait. Muscle activity allows this process to be adaptable, permitting increased energy dissipation or providing more energy storage for acceleration, as each step’s kinetics and kinematics requires it. Adaptability, not consistency, is the most important capability of the foot and lower limb.
A number of factors need to come into play for a safe heel lift to provide efficient acceleration. Requirements in any give step will reflect the spatiotemporal parameters (gait angle, step length, gait speed, etc.) of each step. The contralateral swing limb should be applying maximal centrifugal forces during late swing and be entering its terminal swing just before ipsilateral heel lift. The heel of the swing foot should be around 1 cm above the ground at ipsilateral heel lift.
The centrifugal forces of the swing limb help accelerate the CoM of the body towards the next step. As part of this swing limb positioning, the bulk of body mass should be placed anteriorly to the MTP joints before heel lift so that excessive body mass over the single support limb does not delay or overloading heel lifting power. Heel lift itself is a single-limb support event, although it should rapidly be followed by the start this foot’s terminal double-stance phase. At heel lift, the foot must be rigid enough that plantarflexion power derived from the Achilles (aided by that derived from other plantarflexing extrinsic muscle’s tendons) can be applied to the forefoot to make a stable acceleration platform. Most of this acceleration power arises from soleus, especially at slower walking speeds, because soleus provides most of the anterior tibial rotation braking moment during midstance (McGowan et al, 2008; Orselli et al, 2017). Foot stiffening allows the foot to act as a linear spring with the Achilles, rotating the foot and the lower limb mass around the MTP joints, passively driving digits into extension. The activity of tibialis posterior and peroneus longus are essential to this process, for together they profoundly influence the setting of foot stiffeness properties (Kokubo et al, 2012). Setting stiffness correctly for each step allows the plantarflexion power stored within midstance to be converted to acceleration power without excessive energy dissipation across the potentially very mobile midfoot.
SUMMARY
Acceleration power and energy stored across the vault of the foot during late midstance can only be utilised effectively if the foot is stiffened into a semi-rigid beam before heel lift. The position of the body mass, the centrifugal forces of the swing limb, and vault posture all play an important part in permitting safe and efficient heel lift. If performed well, heel lift should release stored energy for acceleration power and mild energy dissipation to prevent injury, while using relatively little muscular activity during acceleration. Efficient heel lift provides
significant momentum into the next footstep that greatly improves gait energetics (Ruina et al, 2005).
In the next issue we will consider the length of the acceleration lever arm and what happens if the foot is too compliant to form a beam at heel lift.
REFERENCES:
Bjelopetrovich A, Barrios JA. (2016). Effects of incremental ambulatory-range loading on arch height index parameters. Journal of Biomechanics. 49(14): 35553558. Gottschall JS, Kram R. (2003). Energy cost and muscular activity required for propulsion during walking. Journal of Applied Physiology. 94(5): 1766-1772. Gottschall JS, Kram R. (2005). Energy cost and muscular activity required for the leg swing during walking. Journal of Applied Physiology. 99(1): 23-30. Farinelli V, Hosseinzadeh L, Palmisano C, Frigo C. (2019). An easily applicable method to analyse the ankle-foot power absorption and production during walking. Gait & Posture. 71: 56-61. Farris DJ, Kelly LA, Cresswell AG, Lichtwark GA. (2019). The functional importance of human foot muscles for bipedal locomotion. Proceedings of the National Academy of Sciences of the United States of America. 116(5): 1645-1650. Ferris L, Sharkey NA, Smith TS, Matthews DK. (1995). Influence of extrinsic plantar flexors on forefoot loading during heel rise. Foot & Ankle International. 16(8): 464-473. Horwood A. (2021). Essentials of Biomechanics. Principles of gait: the inverted pendulum model. Podiatry Review. 78(4): 29-32. Hunt AE, Smith RM, Torode M, Keenan A-M. (2001). Inter-segment foot motion and ground reaction forces over the stance phase of walking. Clinical Biomechanics. 16(7): 592-600. Kokubo T, Hashimoto T, Nagura T, Nakamura T, Suda Y, Matsumoto H, et al. (2012). Effect of the posterior tibial and peroneus longus on the mechanical properties of the foot arch. Foot & Ankle International. 33(4): 320-325. McGowan CP, Neptune RR, Kram R. (2008). Independent effects of weight and mass on plantar flexor activity during walking: implications for their contributions to body support and forward propulsion. Journal of Allied Physiology. 105(2): 486-494. Murley GS, Menz HB, Landorf KB. (2014). Electromyographic patterns of tibialis posterior and related muscles when walking at different speeds. Gait & Posture. 39(4): 1080-1085. Orselli MIV, Franz JR, Thelen DG. (2017). The effects of Achilles tendon compliance on triceps surae mechanics and energetics in walking. Journal of Biomechanics. 60: 227-231. Ruina A, Bertram JEA, Srinivasan M. (2005). A collisional model of the energetic cost of support work qualitatively explains leg sequencing in walking and galloping, pseudo-elastic leg behavior in running and the walk-to-run transition. Journal of Theoretical Biology. 237(2): 170-192. Stolwijk NM, Koenraadt KLM, Louwerens JWK, Grim D, Duysens J, Keijsers NLW. (2014). Foot lengthening and shortening during gait: A parameter to investigate foot function? Gait & Posture. 39(2): 773-777. Takabayashi T, Edama M, Inai T, Nakamura E, Kubo M. (2020). Effect of gender and load conditions on foot arch height index and flexibility in Japanese youths. Journal of Foot & Ankle Surgery. 59(6): 1144-1147.