Electrocardiography of the dog and cat Diagnosis of arrhythmias II Edition Santilli
Visit to download the full and correct content document: https://textbookfull.com/product/electrocardiography-of-the-dog-and-cat-diagnosis-ofarrhythmias-ii-edition-santilli/
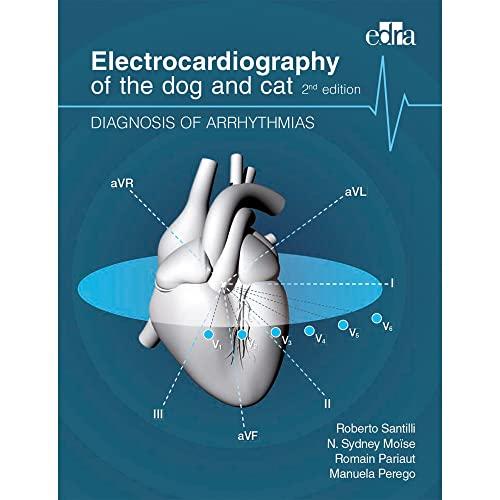
More products digital (pdf, epub, mobi) instant download maybe you interests ...
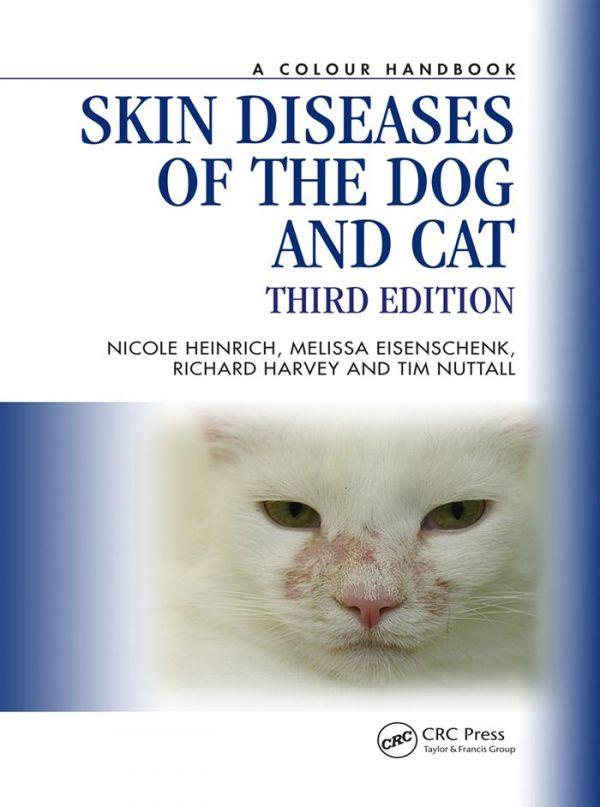
Skin diseases of the dog and cat Third Edition
Eisenschenk
https://textbookfull.com/product/skin-diseases-of-the-dog-andcat-third-edition-eisenschenk/
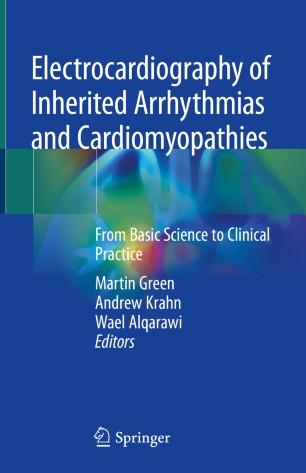
Electrocardiography of Inherited Arrhythmias and Cardiomyopathies From Basic Science to Clinical Practice Martin Green
https://textbookfull.com/product/electrocardiography-ofinherited-arrhythmias-and-cardiomyopathies-from-basic-science-toclinical-practice-martin-green/
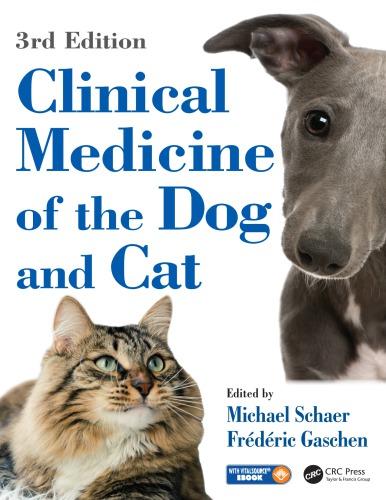
Clinical Medicine of the Dog and Cat Third Edition
Michael Schaer
https://textbookfull.com/product/clinical-medicine-of-the-dogand-cat-third-edition-michael-schaer/
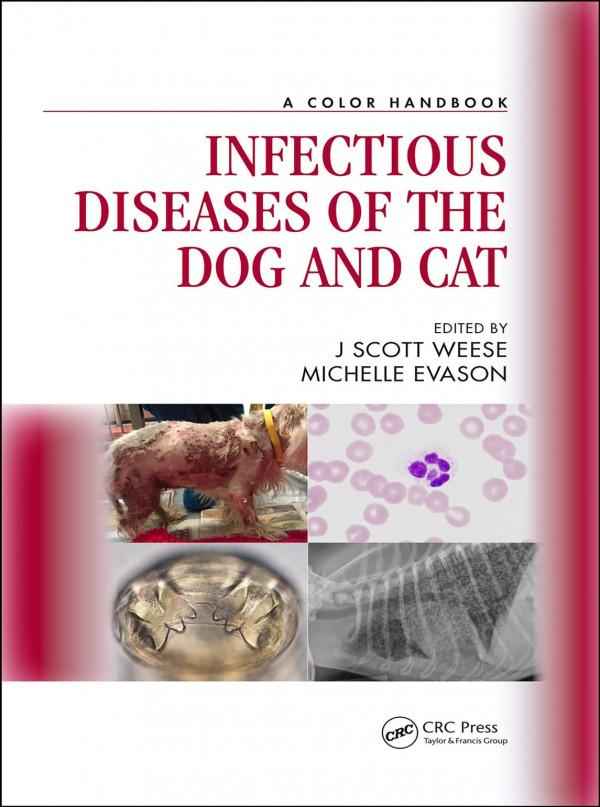
Infectious Diseases of the Dog and Cat-A Color Handbook 1st Edition Scott Weese (Editor)
https://textbookfull.com/product/infectious-diseases-of-the-dogand-cat-a-color-handbook-1st-edition-scott-weese-editor/
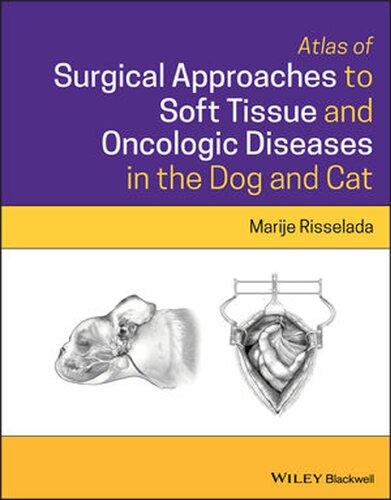
Atlas of Surgical Approaches for Soft Tissue and Oncologic Diseases in the Dog and Cat Marije Risselada
https://textbookfull.com/product/atlas-of-surgical-approachesfor-soft-tissue-and-oncologic-diseases-in-the-dog-and-cat-marijerisselada/
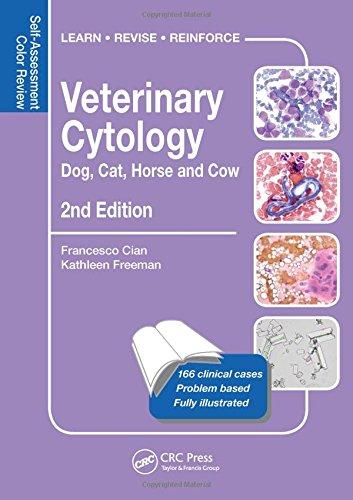
Veterinary Cytology: Dog, Cat, Horse and Cow: SelfAssessment Color Review, Second Edition Cian
https://textbookfull.com/product/veterinary-cytology-dog-cathorse-and-cow-self-assessment-color-review-second-edition-cian/
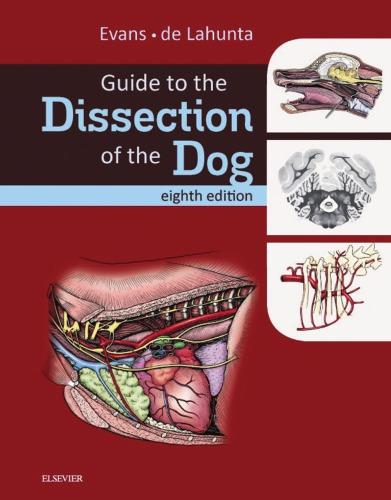
Guide to the dissection of the dog Evans
https://textbookfull.com/product/guide-to-the-dissection-of-thedog-evans/
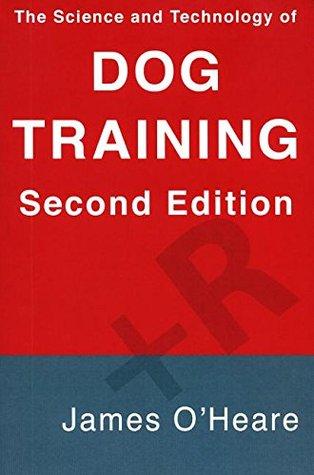
The Science and Technology of Dog Training 2nd Edition
James O’Heare
https://textbookfull.com/product/the-science-and-technology-ofdog-training-2nd-edition-james-oheare/
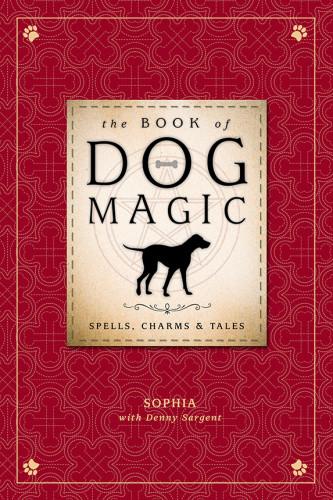
The book of dog magic spells charms tales First Edition
Sophia
https://textbookfull.com/product/the-book-of-dog-magic-spellscharms-tales-first-edition-sophia/
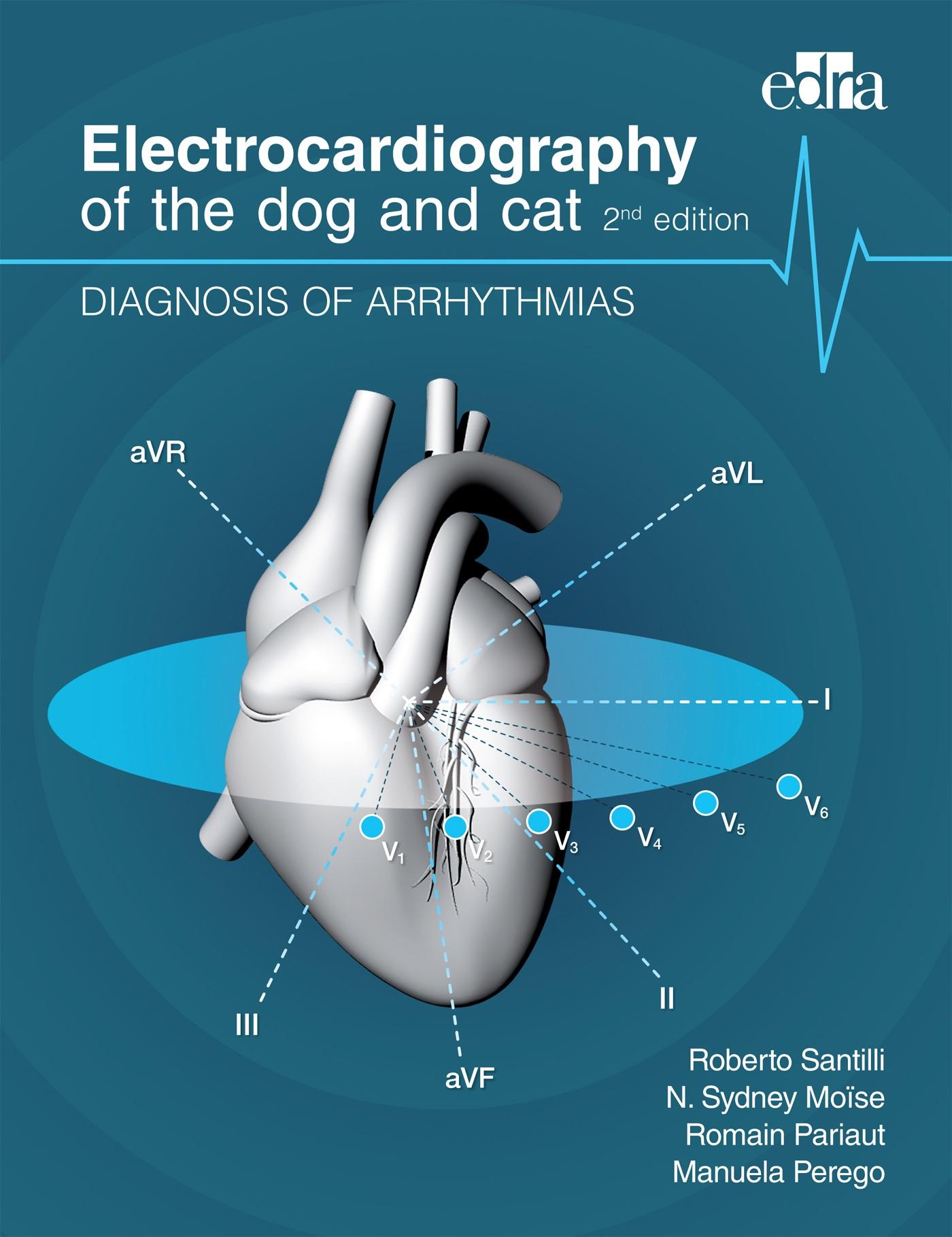
Electrocardiography of the dog and cat
DIAGNOSIS OF ARRHYTHMIAS
2nd edition
Electrocardiography of the dog and cat
DIAGNOSIS OF ARRHYTHMIAS
2nd edition
Roberto Santilli
N. Sydney Moïse
Romain Pariaut
Manuela Perego
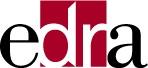
Senior Editor: Alessandra Muntignani
Project Manager: Mercedes González Fernández de Castro
Design and layout: Grupo Asís Biomedia, S.L.
Cover artwork: Federica Farè, Veronica Santilli, and Roberto Santilli Paper, Print and Binding Manager: Michele Ribatti
© 2018 Edra S.p.A.* – All rights reserved
ISBN: 978-88-214-4784-6
eISBN: 978-88-214-4785-3
No part of this publication may be reproduced, stored in a retrieval system, or transmitted, in any form or by any means, electronic, mechanical, photocopying, recording, or otherwise, without prior written permission from the publisher.
Knowledge and best practice in this field are constantly changing: as new research and experience broaden our knowledge, changes in practice, treatment, and drug therapy may become necessary or appropriate. Readers are advised to check the most current information provided (i) or procedures featured or (ii) by the manufacturer of each product to be administered, to verify the recommended dose or formula, the method and duration of administration, and contraindications. It is the responsibility of the practitioners, relying on their own experience and knowledge of the patient, to make diagnoses, to determine dosages and the best treatment for each individual patient, and to take all appropriate safety precautions. To the fullest extent of the law, neither the Publisher nor the Editors assume any liability for any injury and/or damage to persons, animals or property arising out of or related to any use of the material contained in this book.
Edra S.p.A.
Via G. Spadolini 7, 20141 Milano Tel. 02 881841 www.edizioniedra.it
(*) Edra S.p.A. is part of
The authors
Roberto A. Santilli, Dr. Med. Vet., PhD, DECVIM-CA (Cardiology)
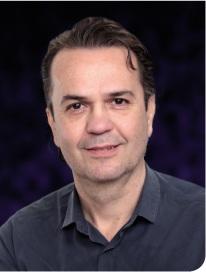
Roberto Santilli graduated from the College of Veterinary Medicine of the University of Milan in 1990. He became a diplomate of the European College of Veterinary Internal Medicine-Companion Animals (Specialty of Cardiology) in 1999. Between 2004 and 2006, he completed a Master in Electrophysiology and Electrical Stimulation at the University of Medicine of Insubria. He then obtained a PhD at the University of Turin, College of Veterinary Internal Medicine in 2010. Roberto Santilli is the head of the cardiology departments of the Clinica Veterinaria Malpensa in Samarate, Varese (Italy) and of the Ospedale Veterinario I Portoni Rossi, Bologna (Italy). Since 2014, he has been an Adjunct Professor of Cardiology at the Cornell University College of Veterinary Medicine, where he is actively involved in the development of a cardiac electrophysiologic laboratory. His main research activities include the diagnosis and treatment of arrhythmias in dogs and cats.
N. Sydney Moïse DVM, MS, DACVIM (Cardiology and Internal Medicine)
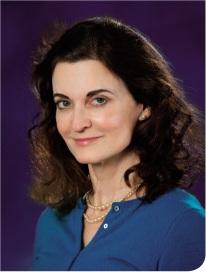
Sydney Moïse graduated from the College of Veterinary Medicine, Texas A&M University (DVM) and Cornell University (MS). She became a diplomate of the American College of Internal Medicine in 1982 and the subspecialty of Cardiology in 1986. She established the Cardiology Service at Cornell University and is
currently Professor in the Department of Clinical Sciences. She has been involved in the clinical practice of cardiology, teaching and research. Her research has primarily focused on arrhythmias and their underlying mechanisms. For six years she served as Editor-in-Chief for the Journal of Veterinary Cardiology. Throughout her career she has been involved in international veterinary medicine with regards to teaching and collaboration. For her work she has been awarded the American Veterinary Medical Association Research Award (American Kennel Club) and the British Veterinary Medical Association Bourgelat Award.
Romain Pariaut Dr. Vre., DACVIM (Cardiology), DECVIM-CA (Cardiology)
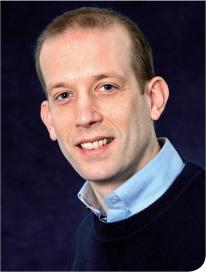
Romain Pariaut graduated from the School of Veterinary Medicine of Lyon, France, in 1999. He became a diplomate of the American College of Internal Medicine (Cardiology Subspecialty) in 2005, and a diplomate of the European College of Internal Medicine- Companion Animals (Specialty of Cardiology) in 2006. Between 2007 and 2015, he was a faculty member in the School of Veterinary medicine at Louisiana State University. He then joined Cornell University as an Associate Professor in the Department of Clinical Sciences and is actively involved in the development of a cardiac electrophysiology laboratory. Since 2007, Romain Pariaut has been an Associate Editor for the Journal of Veterinary Cardiology.
Manuela Perego, Dr. Med. Vet.
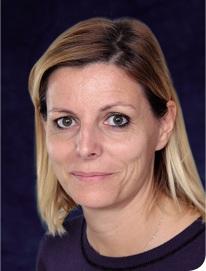
Manuela Perego graduated from the College of Veterinary Medicine of the University of Milan in 2003. She completed a residency program of the European College of Veterinary Internal Medicine-Companion Animals (Specialty of Cardiology) between 2010 and 2013 under the supervision of Dr. Roberto Santilli. She currently works in the Cardiology Departments of the Clinica Veterinaria Malpensa in Samarate, Varese (Italy) and the
Ospedale Veterinario I Portoni Rossi, Bologna (Italy). Her main research activities include the diagnosis and treatment of arrhythmias in dogs and cats.
Preface
“It is the theory which decides what we can observe.”
Albert Einstein
The main objective of this book is to give both clinical and theoretical information to interpret simple and complex electrocardiograms in dogs and cats. Detailed description of normal rhythm variations and arrhythmias is provided to meet the readers’ needs whether they are just beginners or experts. Consequently, veterinary students and busy general practitioners will find the information sought to categorize electrocardiograms, while cardiology residents and specialists will discover additional depths for understanding the complexities of the electrophysiology behind the electrocardiogram. For all, the goal of this book is to increase knowledge to meet the challenge of electrocardiographic interpretation. We have made the deliberate choice to use the same terminology and electrocardiographic planes used for the analysis of the human electrocardiogram. We have done so because the main source of information concerning the clinically applicable electrophysiologic studies of arrhythmias is from those performed in people.
In recent years, research to understand the electrophysiological mechanisms behind cardiac arrhythmias in veterinary patients has increased. Although these studies remain limited, the advancements are real and give us a better understanding of the clinical arrhythmias we commonly and uncommonly diagnose. Such information improves not only our ability to make an accurate diagnosis, but to deliver better treatment.
The first three chapters of this book include a detailed description of the anatomy and electrophysiology of the conduction system, the theory behind the formation of the electrocardiographic waveforms, and the recording techniques available to the clinician. The following chapters first focus on the characteristics of normal rhythm in dogs and cats, the impact of cardiac chamber enlargement on electrocardiogram, and then detail the portfolio of atrial and ventricular arrhythmias described in veterinary medicine. Specifically, chapters 7 to 10 are dedicated to ectopies and tachyarrhythmias. Chapters 11 and 12 describe bradyarrhythmias and conduction abnormalities. Chapter 13 is an overview of the effects of systemic diseases and drugs on the electrocardiogram. Finally, chapter 14 describes the electrocardiogram in the presence of an artificial pacemaker, and the signs of device malfunction. We have included at the end this book a series of electrocardiograms that readers can use to test their knowledge.
Initially published in Italian, this publication is now accessible to the English-speaking readership in an expanded and updated version. This project was possible by the addition of two co-authors. Notably this work stems from a common passion for the fascinating complexity of arrhythmias and it is the product of friendship, collaboration and mutual academic respect.
We hope that the readers will find it helpful in their daily clinical activities, and rather than feeling overwhelmed by the intricacies of electrocardiography, will develop their interest in deciphering the tracings they record.
Finally, we would like to thank all the individuals who have directly or indirectly contributed to this book: architect Federica Farè who designed several new figures; architect Federica Farè and Veronica Santilli for the ideation and realisation of the cover; Dr. Lucia Ramera and Dr. Maria Mateos Panero who prepared the material needed to proceed with the first translation from Italian to English; Dr. Gianmario Spadacini and Dr. Paolo Moretti from the Instituto Clinico Humanitas Mater Domini Castellanza (Varese) for their guidance and leadership in the field of clinical cardiac electrophysiology; Dr. Alberto Perini for his expertise in anesthesiology and his assistance during electrophysiologic studies and pacemaker implantation procedures; Dr. Silvia Scarso and the Anesthesia Section of Cornell University for consulting on the effects of sedatives and anesthetics on the cardiac rhythm; our colleagues of the Veterinary Clinic Malpensa for their help in the management of our canine and feline patients with arrhythmias; Shari Hemsley for extensive Holter analysis at Cornell University; the cardiology residents at Cornell University; the many veterinarians who continue to refer us cases and help us expand our database of electrocardiograms and Holters by using the cardiology services of the Veterinary Clinic Malpensa and Cornell University; finally, Edra Publishing House for technical support, and Dr Carlo Scotti for believing in this work and supporting its development.
Roberto A. Santilli, N. Sydney Moïse, Romain Pariaut, Manuela Perego
Table of contents
CHAPTER 1
ANATOMY AND PHYSIOLOGY OF THE CONDUCTION SYSTEM
Anatomy of the conduction system
Anatomical substrates for arrhythmias
The action potential
Correlation between the phases of the action potential and electrocardiographic waves
Electrical properties of the myocardium and their relationship with the action potential
Spontaneous automaticity of pacemaker cells
Atrioventricular conduction
Propagation of the cardiac electrical impulse
Cardiac nervous control
Other factors that influence cardiac activity
CHAPTER 2
PRINCIPLES OF ELECTROCARDIOGRAPHY
Historical notes
The surface electrocardiogram
CHAPTER 3
FORMATION AND INTERPRETATION OF THE ELECTROCARDIOGRAPHIC WAVES
The electrocardiograph
Recording and calibration of the electrocardiographic tracing
Formation of the electrocardiographic waves
Electrocardiographic analysis
Tools for interpreting the electrocardiogram
Electrocardiography during the first weeks of life
Artifacts
CHAPTER 4
NORMAL SINUS RHYTHMS
Sinus rate
Sinus rhythm
Sinus arrhythmia
Sinus rhythms with other arrhythmias
CHAPTER 5
CHAMBER ENLARGEMENT
Atrial enlargement
Ventricular enlargement
CHAPTER 6
BACKGROUND TO THE DIAGNOSIS OF ARRHYTHMIAS
Mechanisms of arrhythmias
Abnormalities of impulse formation: automaticity and triggered activity
Abnormalities of impulse conduction
Classification of arrhythmias
Hemodynamic consequences of arrhythmias
Arrhythmia-related clinical signs
Diagnosis of arrhythmias
CHAPTER 7
SUPRAVENTRICULAR BEATS AND RHYTHMS
Ectopic atrial beats and rhythms
Junctional ectopic beats and rhythms
Patterns of ectopic supraventricular beats
Relationship between atrial and ventricular activation
Atrial parasystole
Atrial dissociation
CHAPTER 8
SUPRAVENTRICULAR TACHYCARDIAS
Sinus tachycardia
Atrioventricular nodal reciprocating tachycardia
Focal junctional tachycardia and non-paroxysmal junctional tachycardia
Atrioventricular tachycardias mediated by accessory pathways
Focal atrial tachycardia
Multifocal atrial tachycardia
Macro-reentrant atrial tachycardia (atrial flutter)
Atrial fibrillation
Differential diagnosis of narrow QRS complex tachycardias in the dog
CHAPTER 9
VENTRICULAR ECTOPIC BEATS AND RHYTHMS
Ventricular ectopic beats
Ventricular parasystole
CHAPTER 10
VENTRICULAR ARRHYTHMIAS
Defining tachycardia
Describing tachycardia
Monomorphic ventricular tachycardias
Polymorphic ventricular tachycardia
Bidirectional ventricular tachycardia
Ventricular fibrillation
Ventricular tachycardia without structural cardiac disease
Ventricular tachycardia during familial dilated cardiomyopathy
Ventricular tachycardias during arrhythmogenic cardiomyopathy
Ventricular tachycardias during myocarditis
Ventricular tachycardia during ischemic cardiomyopathy
Ventricular tachycardia during ventricular hypertrophy
Ventricular tachycardia during congestive heart failure
Ventricular tachycardias during systemic diseases
Differential diagnosis of wide QRS complex tachycardia
Determining the danger of ventricular tachycardias
CHAPTER 11
BRADYARRHYTHMIAS
Sinus bradycardia
Sinus arrest
Sinus standstill
Sinus node dysfunction or sick sinus syndrome
Atrial standstill or atrioventricular muscular dystrophy
Sino-ventricular rhythm
Asystole or ventricular arrest
Pulseless electrical activity or electromechanical dissociation
CHAPTER 12
CONDUCTION DISORDERS
Disorders of atrial conduction
Inter-atrial conduction block
Atrioventricular blocks
Intraventricular conduction disorders or bundle branch blocks
Aberrant conduction
Linking or sustained aberrant conduction
Gap phenomenon and supernormal conduction
CHAPTER 13
ELECTROCARDIOGRAPHIC CHANGES SECONDARY TO SYSTEMIC DISORDERS AND DRUGS
Hypoxia
Electrolytic disorders
Pericardial diseases
Abdominal diseases
Chronic respiratory diseases
Endocrine diseases
Intracranial diseases
Hyperthermia and hypothermia
Electrocution
Autoimmune diseases
Drugs
CHAPTER 14
ELECTROCARDIOGRAPHY AND PACING
Basic components of the pacemaker
Pacing modes
Pacemaker malfunction
GUIDED INTERPRETATION OF ELECTROCARDIOGRAPHIC TRACINGS
Anatomy and physiology of the conduction system
Under normal conditions, the electrical impulse generated by the pacemaker cells of the sinus node propagates to the atria, atrioventricular node and ventricles along bundles of specialized cardiomyocytes and triggers muscle contraction, a mechanism known as electro-mechanical coupling.
Anatomy of the conduction system
The cardiac conduction system is not directly visible on gross examination of the heart. In order to identify its various components, it is necessary to combine detailed histological studies and molecular techniques that can reveal the differences between myocytes responsible for the initiation and propagation of electrical impulses and myocytes involved in force generation. The cardiac conduction system consists of two types of tissues with different anatomical and electrophysiological characteristics: the nodal tissue and the conduction tissue. The nodal tissue contains cells that can spontaneously depolarize (spontaneous automaticity) and act as pacemakers. The conduction tissue is made of cells organized in bundles and usually separated from the working myocardium by a sheath of connective tissue. These cells are responsible for the rapid propagation of electrical impulses to the rest of the atrial and ventricular myocardium. The conduction system can be divided into three major components: the sinus node (or sino-atrial node), the atrioventricular junction, which includes the atrioventricular node, and the intraventricular conduction system.
Electrical impulses originate in the sinus node. They propagate through the atrial myocardium, the inter-atrial bundles (inferior fascicle and Bachmann’s bundle), and possibly internodal tracts (anterior, middle and posterior tracts). The existence of internodal tracts remains controversial. There are likely several reasons behind this controversy: interspecies variations regarding the organization of the conduction system, difficulties in clearly identifying the path of thin bundles in serial histological sections of atrial tissue, and the lack of consistency in the terminology used to describe the various components of the conduction system. Internodal tracts between the sinus node and the atrioventricular conduction axis have been described in detail in the dog by a single investigator (DK Racker, et al.). Although other experts refute the existence of these tracts, they do agree that preferential pathways, defined by myofiber orientation, ridges, valve annuli and venous ostia, exist between the sinus node and the atrioventricular junction in all mammals commonly studied. As they approach the atrioventricular junction, impulses sequentially reach the atrionodal bundles (superior, medial and lateral bundles), the proximal atrioventricular bundle (or inferior nodal extension) and the compact atrioventricular node. The latter is located on the floor of the right atrium at the level of the atrioventricular fibrous skeleton. The compact atrioventricular node is in continuity with the distal atrioventricular bundle. The penetrating portion of the distal atrioventricular bundle, or His bundle, crosses the right fibrous trigone at the inferior part of the membranous septum, and connects the specialized conduction system of the atrioventricular junction with the specialized ventricular conduction system. Once it reaches the ventricles, the distal atrioventricular bundle divides into left and right branches. The left branch further subdivides into an antero-superior fascicle and a postero-inferior fascicle, which lead electrical impulses from the interventricular septum to the cardiac apex. The Purkinje network finally connects the bundle branches to the working myocardium, enabling rapid and coordinated activation of the entire ventricular mass (Fig. 1.1). All cardiomyocytes are connected by gap junctions. Gap junctions are one component of the intercalated disc and are responsible for electrical coupling of myocytes; in other words, they allow cell-to-cell propagation of electrical impulses. The number of gap junctions is small within the nodes, which are regions of slow impulse velocity. These gap junctions are formed by the assembly of connexin 40 and 45 molecules. Conversely, in the specialized bundles of the conduction system, in which impulse propagation is rapid, there is a large number of gap junctions made of connexin 43.
Sinus node
The sinus node (or sino-atrial node) is a complex structure. Fortunately in recent years the canine sinus node has been studied, providing a more accurate appreciation of its structure and function. The sinus node is located below the epicardial surface at the junction of the cranial vena cava and the right atrium. This positioning is near the upper portion of the sulcus terminalis/crista terminalis. The sulcus terminalis is the name given to the epicardial landmark that corresponds to the endocardial landmark of the crista terminalis. These two landmarks (epicardial view versus endocardial view), which extend between the cavae (intercaval strip), represent the junction of the embryonic sinus venosus and the pectinate muscles of the right auricle. This line of demarcation, which is composed of fibrous tissue, is important in the function of the sinus node. Parallel to the sulcus terminalis/crista terminalis is the sinus node artery. This artery has two branches which surround the central sinus node. Each of these anatomical structures serves to insulate the sinus node from the atrium (Fig. 1.2).
Although the sinus node is represented in books as a well-defined nodule, it should only be seen as a schematic representation of a very complex structure (Fig. 1.2). Recent studies in dogs and humans reveal that the sinus node should really be thought of as a sinus node complex composed of the compact sinus node, exit pathways, and transitional cells. The compact sinus node is defined anatomically and functionally today with the aid of immunofluorescence staining for specific connexins. As explained above, specific areas of the heart have different concentrations of different types of connexins. The atrial myocardial cells have connexin 43; however, this connexin is absent in the sinus node. Therefore, staining for this protein aids in the proper identification of the sinus node. In addition to the central or compact sinus node there is a transitional region which has a mixture of cell types and varying densities of fibrous tissue and myocardial cells. In mid-size dogs, the length of the compact sinus node ranges between 15 and 20 mm, its width is between 5 and 7 mm and it is approximately 200-µm thick. A layer of atrial myocardium separates the sinus node from the endocardium. In cats, the sinus node is approximately 7-mm long, 2-mm wide and it is approximately 300- to 500-µm thick. Importantly, the entire sinus node complex including the sino-atrial exit pathways encompasses a much larger region. In the dog the sinus node complex can extend from the cranial vena cava to near the coronary sinus. It is this large size that permits the wandering pacemaker. It should be emphasized however that a wandering pacemaker is a reflection of atrial depolarization. The excitation of the atrium is dependent on which exit pathway is used for a given sinus impulse. Studies in the dog reveal that there are at least two such pathways with one located near the cranial vena cava (superior) and the other more inferior and lower on the atrial floor (inferior). The autonomic nervous system largely determines which pathway is used by electrical impulses to exit the sinus node. They exit from the superior exit pathway with high sympathetic tone and from the inferior exit pathway with high parasympathetic tone. It is also important to note that portions of the atria can be depolarized before the sinus node complex itself has completely depolarized.
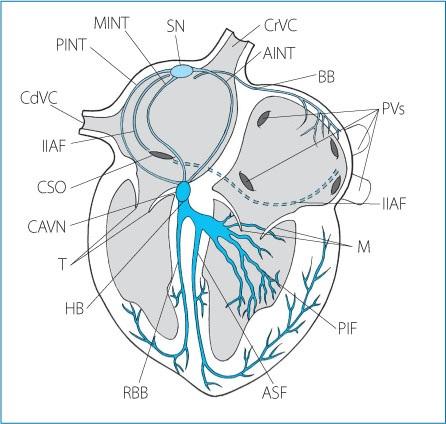
Figure 1.1. The specialized cardiac conduction system in the dog. CrVC: cranial vena cava; CdVC: caudal vena cava; PVs: pulmonary veins; CSO: coronary sinus ostium; SN: sinus node; PINT: posterior internodal tract; MINT: medial internodal tract; AINT: anterior internodal tract; IIAF: inferior inter-atrial fascicle; BB: Bachmann’s bundle; CAVN: compact atrioventricular node; HB: His bundle; RBB: right bundle branch; PIF: postero-inferior fascicle; ASF: antero-superior fascicle; T: tricuspid leaflets; M: mitral leaflets.
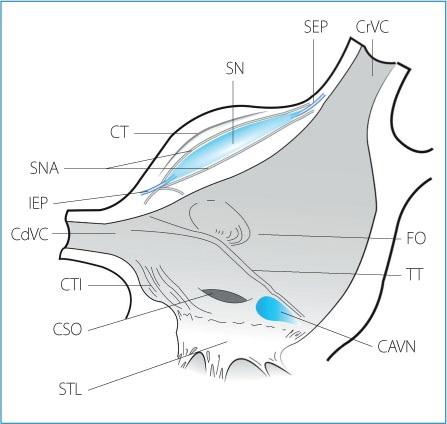
Figure 1.2. Anatomical section of the right atrial cavity and the entrance of the venae cavae after removal of part of the right atrial free wall and the right appendage to show sinus node complex anatomy. CrVC: cranial vena cava; SEP: superior exit pathway; SN: sinus node; CT: crista terminalis; SNA: sinus node arteries; IEP: inferior exit pathway; CdVC: caudal vena cava; CTI: cavotricuspid isthmus; CSO: coronary
sinus ostium; STL: septal tricuspid leaflet; CAVN: compact atrioventricular node; TT: tendon of Todaro; FO: fossa ovalis.
The sinus node is formed by a large amount of connective tissue, and two types of specialized atrial myocytes: the P (pacemaker or “typical” nodal) cells and the T (or transitional) cells.
The P cells are at the center of the sinus node (compact sinus node) and account for approximately 45 % to 50 % of the cell population of the sinus node. P cells are small and sometimes called “empty cells” because they contain fewer myofilaments, mitochondria and sarcoplasmic reticulum than working atrial myocytes. Moreover, they are connected by a low number of gap junctions. At least three morphologies of P cells have been described: the first type is made of ovoid cells containing scattered myofibrils; the second type (or spindle cell) is characterized by an elongated shape with numerous myofibrils; finally, the third type (spider-shaped cell) consists of cells with a central body from which three or more extensions branch out.
T cells are organized at the periphery of the P cells and form a transition zone between the compact sinus node and the working atrial myocardium. T cells have an intermediate morphology between P cells and regular atrial myocytes. All degrees of intermediate morphology can be found, with some cells having almost all the characteristics of the P cells and others resembling working atrial myocytes.
There are interspecies variations regarding the vascularization of the sinus node. Two-thirds of the blood supply is provided by the sinus node artery, which is a terminal branch of the right coronary artery in 90 % of dogs, although it is a branch of the left coronary artery in 10 % of dogs. The remaining one-third is provided by collateral vessels. Venous drainage of the sinus node depends on small veins, called Thebesian veins, which, after traveling through endocardium, open into the right atrium.
Sinus node automaticity is modulated by autonomic tone, and at rest vagal tone predominates. Vagal innervation is provided by discrete vagal efferents and a local (or intrinsic) network of autonomic nerves concentrated in several epicardial fat pads. Sympathetic innervation to the sinus node travels via the left and right subclavian loops, which project from the stellate ganglia. The sinus node mainly receives input from right sympathetic fibers.
Internodal tracts
As previously stated, the existence of anatomically distinct bundles of specialized myocytes between the sinus and atrioventricular nodes remains controversial, although detailed histopathologic studies of the atrioventricular conduction axis (DK Racker) have provided convincing evidence that they are present at least in the dog. In other species, preferential conduction pathways between the sinus and atrioventricular nodes have been recognized but do not appear to be clearly separated from the adjacent myocardium.
Based on Racker’s description of the intra-atrial conduction system in dogs, there are three internodal tracts (Fig. 1.3):
an anterior internodal tract, a middle internodal tract, and a posterior internodal tract.
The anterior internodal tract arises from the anterior aspect of the sinus node, runs along the anterior margin of the cranial vena cava, crosses Bachmann’s bundle and then continues along the anterior part of the atrial septum. Finally, it joins the superior atrionodal bundle.
The middle internodal tract originates from the sinus node, runs parallel to the posterior internodal tract, anteriorly contours the region of the fossa ovalis and continues in the medial atrionodal bundle.
The posterior internodal tract originates from the sinus node, runs along the crista terminalis and travels along the posterior part of the inter-atrial septum, to reach the coronary sinus ostium. Finally, it continues in the lateral atrionodal bundle.
Inter-atrial bundles
In dogs, inter-atrial conduction occurs along two preferential pathways formed by Bachmann’s bundle and the inferior inter-atrial fascicle. Bachmann’s bundle extends from the region of the sinus node on the right to the left auricle and forms a discrete subepicardial bundle of myocytes where it straddles the inter-atrial groove (Figs. 1.1 and 1.3). The myocytes that form Bachmann’s bundle have some of the characteristics of Purkinje fibers: they conduct impulses at a higher velocity than working atrial myocytes, and they are more resistant to hyperkalemia.
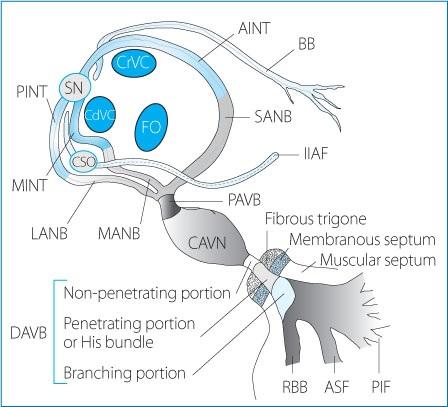
Figure 1.3. Detailed anatomy of the atrial, junctional and ventricular conduction system in the dog. See text for explanation. SN: sinus node; CdVC: caudal vena cava; CrVC: cranial vena cava; CSO: coronary sinus ostium; FO: fossa ovalis; BB: Bachmann’s bundle; IIAF: inferior inter-atrial fascicle; AINT: anterior internodal tract; MINT: middle internodal tract; PINT: posterior internodal tract; LANB: lateral atrionodal bundle; MANB: medial atrionodal bundle; SANB: superior atrionodal bundle; PAVB: proximal atrioventricular bundle; CAVN: compact atrioventricular node; DAVB: distal atrioventricular bundle; RBB: right bundle branch; ASF: antero-superior fascicle; PIF: postero-inferior fascicle.
The inferior inter-atrial fascicle connects the right and left atrium along the path of the coronary sinus. The distal portion of the inferior fascicle terminates in the left atrial myocardium, at the level of the ligament of Marshall (Figs. 1.1 and 1.3). The ligament of Marshall is the remnant of the left cranial vena cava, and extends between the upper and lower left pulmonary veins.
The epicardial portion of the coronary sinus represents another inter-atrial connection between the lower right and left atrium.
Atrioventricular junction
The atrioventricular junction (or atrioventricular conduction axis) includes: 1) preferential pathways (nodal approaches) along the right atrial wall and inter-atrial septum, and possibly discrete atrionodal bundles in dogs; 2) a proximal atrioventricular bundle (or inferior nodal extension); 3) the compact atrioventricular node; and 4) the non-penetrating and penetrating portions of the distal atrioventricular bundle. The membranous interventricular septum represents the distal boundary of the atrioventricular junction.
Atrionodal bundles and proximal atrioventricular bundle (inferior nodal extension)
Controversies remain about the exact nature of these pathways, and whether discrete tracts (atrionodal and proximal atrioventricular bundles) of specialized cells proximal to the compact atrioventricular node exist. DK Racker’s detailed studies of the conduction system in the canine heart support the existence of several atrionodal bundles (superior, medial and lateral) that converge into a proximal atrioventricular bundle, whereas other investigators refute the existence of specialized myocytes insulated from surrounding tissue leading to the compact atrioventricular node. Instead, their results are consistent with functional pathways formed by transitional cells (T cells) and delineated by vein ostia, intra-atrial ridges and the tricuspid valve annulus.
According to DK Racker’s observations these atrionodal bundles (also called nodal approaches) represent the distal continuation of the internodal tracts, and constitute, together with the proximal atrioventricular bundle, the atrionodal region (AN) of the atrioventricular node (Fig. 1.3). Three atrionodal bundles have been described and classified as: the superior atrionodal bundle,
the medial atrionodal bundle, and the lateral atrionodal bundle.
The superior atrionodal bundle is the distal continuation of the anterior internodal tract. It runs in the superoanterior portion of the medial wall of the right atrium, close to the interventricular septal ridge.
The medial atrionodal bundle is the distal continuation of the medial internodal tract. It runs along the supero-medial portion of the coronary sinus ostium, under the epicardial layer of the right atrial medial wall, at the opposite site of the medial portion of the tendon of Todaro.
The lateral atrionodal bundle is the distal continuation of the posterior internodal tract. It runs along the infero-lateral portion of the coronary sinus ostium, under the epicardial layer of the infero-posterior portion of the right atrial medial wall.
The three atrionodal bundles converge to form the proximal atrioventricular bundle (or inferior nodal extension) which is in continuity with the compact node (Fig. 1.3).
Atrioventricular node
The atrioventricular node consists of a proximal portion formed by the atrionodal bundle and the proximal atrioventricular bundle, a central portion also called the compact node, located to the right of the fibrous trigone (or central fibrous body), and a distal portion corresponding to the non-penetrating portion of the distal atrioventricular bundle.
In dogs, the compact atrioventricular node has an elongated shape with its concave surface facing the central fibrous body and the mitral annulus. On average it is 2- to 4- mm long, with a width of 2 mm and a thickness of 0.5 to 1 mm. It is located on the floor of the right atrium approximately 1 mm below the epicardium in the triangle of Koch. The triangle of Koch is bordered by the coronary sinus ostium and its sides are delineated by the tendon of Todaro (an extension of the Eustachian valve) and the attachment of the septal leaflet of the tricuspid valve; its apex corresponds to the penetrating portion of the distal atrioventricular bundle (bundle of His) (Fig. 1.4).
Based on the electrophysiological characteristics of the cells, the atrioventricular node can be divided into three regions: atrionodal (AN), nodal (N) and nodo-Hisian (NH).
The AN region results from the convergence of the atrionodal bundles and the proximal atrioventricular bundle. This region is composed of large cells with a similar morphology to that of Purkinje cells, separated by transitional cells that have an elongated shape. These transitional cells are mixed with P cells, adipocytes, atrial myocytes, collagen and nerve fibers.
The N region is composed of transitional cells closely connected with each other without interposition of connective tissue. For this reason, this region is also called compact node.
The NH region is characterized by P cells and transitional cells connected with the Purkinje cells originating at the atrioventricular distal bundle.
Because of the very different cell populations within the AN, N and NH regions, the atrioventricular node is a site of anisotropic conduction (see p. 99).
In dogs, although the majority of the myofibers direct the electrical impulse along the proximal atrioventricular bundle, preferential pathways also exist in the anterior atrial septum. The presence of these normal pathways represents the anatomical substrate for a property of the atrioventricular node called longitudinal dissociation (Fig. 1.13).
In dogs, the atrioventricular node receives two arterial branches from the circumflex left coronary artery and from the terminal branches of the septal artery. The latter also supplies the His bundle and the proximal part of the bundle branches. Venous drainage occurs via the Thebesian system, which opens into the right atrial chamber through the Thebesian’s ostium, although a small portion of blood also flows into the coronary sinus distal to its opening into the right atrium.
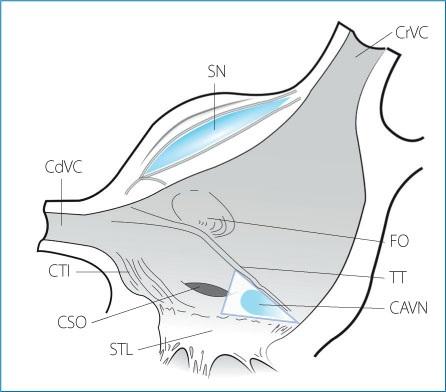
Figure 1.4. Anatomical landmarks of the junctional area and Koch’s triangle. The sides of the triangle, which delimit the location of the atrioventricular node, are formed by the tendon of Todaro and the septal leaflet of the tricuspid valve. The apex of the triangle is formed by the membranous portion of the interventricular septum at the point where the penetrating portion of the distal atrioventricular bundle, or His bundle, enters the atrioventricular junction. The base of the triangle is represented by the coronary sinus ostium. CrVC: cranial vena cava; SN: sinus node; CdVC: caudal vena cava; CTI: cavotricuspid isthmus; CSO: coronary sinus ostium; STL: septal tricuspid leaflet; CAVN: compact atrioventricular node; TT: tendon of Todaro; FO: fossa ovalis.
The atrioventricular node is densely innervated by vagal and adrenergic fibers. The atrioventricular node is predominantly influenced by the left vagal and sympathetic nerves.
Distal atrioventricular bundle
This bundle is the distal prolongation of the compact node and is the only connection between the atrial and ventricular conduction systems. Atria and ventricles are indeed electrically insulated along the entire circumference of the atrioventricular junction and semilunar rings by the fibrous skeleton. The central portion of this structure, known as the central fibrous body, corresponds to a triangle of fibrous tissue situated between the mitral, tricuspid and aortic valve rings. The central fibrous body is crossed posteriorly by the penetrating portion of the distal atrioventricular bundle, also known as the bundle of His.
The distal atrioventricular bundle can be divided into three segments (Fig. 1.3): a non-penetrating portion, a penetrating portion or bundle of His, and a branching portion.
The penetrating portion of the distal atrioventricular bundle, or bundle of His, is the continuation of the nonpenetrating bundle, and is believed to start at the point where the cells of the specialized conduction system lose their reticulated distribution to form parallel fascicles, and ends at the point of its first branch, after crossing the right fibrous trigone at the level of the non-coronary aortic cusp. In medium-sized dogs, the His bundle is approximately 5- to 10- mm long.
The branching segment of the distal atrioventricular bundle starts where the postero-inferior fascicle of the left bundle branch branches off the main bundle, and ends at the emergence of the right bundle branch and the left antero-superior fascicle. Its proximal end, which is a direct continuation of the penetrating portion, is located on the posterior side of the non-coronary aortic cusp, while its distal end is located at the junction between the non-coronary and the right coronary cusps of the aortic valve when examined from the left ventricle. The branching segment of the distal atrioventricular bundle is located below the insertion of the septal leaflet of the tricuspid valve when viewed from the right ventricle.
The His bundle is perfused by a small artery arising from the right coronary artery, or less frequently by the circumflex artery, which is a branch of the left coronary artery. The innervation of the His bundle is similar to
that of the atrioventricular node.
Intraventricular conduction system
In dogs, the intraventricular conduction system is trifascicular in nature. The right bundle branch is an extension of the His bundle. The left bundle branch has two divisions that fan out from the branching segment of the distal atrioventricular bundle, separately or as a common trunk (Fig. 1.5).
Right bundle branch
The right bundle branch is in direct continuation with the His bundle. It forms a cord-like bundle that travels subendocardially down the interventricular septum to the anterior papillary muscle. A fibrous sheath insulates it from the surrounding myocardium. It then divides into several intra-cavitary false tendons (anterior, medial, posterior) that terminate in the right ventricular free wall where they ramify into a subendocardial Purkinje network. Electrical activation of the interventricular septum is not initiated by the right bundle branch (Fig. 1.5). The length of the right bundle branch in medium-size dogs is 35 to 40 mm.
Left bundle branch
The left bundle branch fans out of the branching portion of the distal atrioventricular bundle, and then, immediately below the aortic leaflets, forms a postero-inferior fascicle and an antero-superior fascicle, which then travel towards the base of the postero-medial and antero-lateral papillary muscles, respectively. In dogs, the initial truncular portion of the left bundle branch is short (6-8 mm in medium-size dogs) and wide (approximately 5 mm), and its path is subendocardial. It continues to widen until it divides into the two fascicles. Unlike the right bundle branch that has a cord-like aspect, the truncular portion of the left bundle branch is flat and ribbon-shaped.
The number of fascicles that emerge from the common trunk, and their divisions and paths are highly variable. In the human heart, a discrete third branch, positioned between the two other fascicles and called the septal fascicle is commonly present. Nevertheless, for the purpose of electrocardiographic interpretation, two main fascicles are described, one in an antero-superior position and the other in a postero-inferior position (Fig. 1.5).
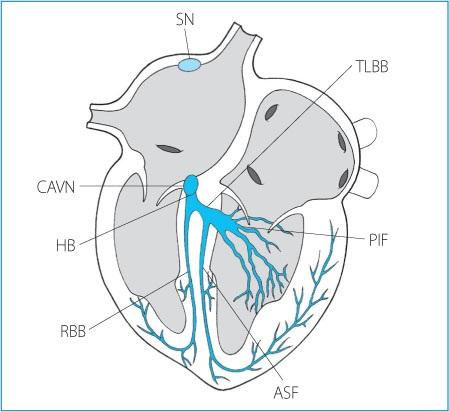
Figure 1.5. Anatomy of the atrioventricular and intraventricular conduction system. SN: sinus node; CAVN: compact portion of the atrioventricular node; HB: His bundle; RBB: right bundle branch; TLBB: truncular portion of the left bundle branch; PIF: postero-inferior fascicle; ASF: antero-superior fascicle.
The antero-superior fascicle appears as a direct continuation of the trunk of the left bundle branch and is
oriented supero-inferiorly and postero-anteriorly. In dogs, the antero-superior fascicle is thin and measures 3.5 to 4 cm in medium-size animals.
The postero-inferior fascicle emerges almost perpendicularly from the truncular portion of the left bundle. In dogs, the postero-inferior fascicle is thick and measures approximately 3.5 cm in medium-size animals.
Purkinje network
The Purkinje fibers connect the terminal portion of the conduction system to the endocardial surface of the ventricles. The myocytes that form the Purkinje fibers are connected by a high number of intercalated discs, which facilitate rapid propagation of electrical impulses (approximately 2 m/s).
On the left side, the Purkinje fibers form a subendocardial network that is denser around the papillary muscles and less developed at the base of the ventricles. Some fibers travel directly inside the cavity of the ventricles, and are called “false tendons”. The Purkinje network of the anterior wall of the left ventricle depends on the antero-superior division of the left bundle branch, while the network of the posterior wall is associated with the postero-inferior division. It is more difficult to identify the origin of the septal and apical left Purkinje network, although, in most cases, the interventricular septum and the apical region are activated by fibers in continuity with the postero-inferior division. The distribution of the Purkinje network in the right ventricular wall and on the right surface of the interventricular septum is connected to the major ramifications of the right bundle branch. The concentration of Purkinje fibers is greater in the antero-apical region of the right ventricular wall and in the apical third of the interventricular septum. Purkinje fibers are mostly absent in the ventricular outflow region.
Anatomical substrates for arrhythmias
Several cardiac anatomical structures are involved in the genesis of arrhythmias. The main anatomical substrates that have been identified in dogs are shown in Table 1.1.
Atrial tachycardias originate from foci distributed within the atria, particularly in the region of the crista terminalis, the coronary sinus ostium and the ostia of the pulmonary veins, which have been recognized as a trigger for atrial fibrillation.
Atrial myocardial fiber stretch, fibrosis and electrical remodeling secondary to atrial dilation can promote atrial tachycardia, atrial flutter and atrial fibrillation. Atrioventricular muscular dystrophy or atrial standstill is another rhythm disturbance linked to severe atrial fibrosis.
The cavotricuspid isthmus is a small region of the right atrium that forms the slow conduction area of an atrial flutter circuit. Specifically, it is at the level of the posterior right atrium and delimited by the ostium of the caudal vena cava, the Eustachian ridge and the annulus of the tricuspid valve. The complete circuit of atrial flutter is a ring of myocardium that includes the septal and posterior walls of the right atrium, joined dorsally by the roof of the right atrium. The wavefront of depolarization can travel in a counterclockwise direction, resulting in an arrhythmia called typical flutter, or it can rotate clockwise, resulting in a reverse typical flutter.
Table 1.1. Anatomical structures involved in the genesis of rhythm disturbances in the dog.
Anatomical structure Rhythm disturbance
Atrial myocardium
Crista terminalis
Coronary sinus
Pulmonary veins
Caval veins
Ligament or vein of Marshall
Atrial remodeling
Cavotricuspid isthmus
Accessory pathway (Kent fibers)
Myocardial fibrosis in structural heart disease
Focal atrial tachycardia
Focal atrial tachycardia
Atrial fibrillation
Atrial flutter
Typical and reverse typical atrial flutter
Atrioventricular tachycardia
Focal atrial tachycardia
Atrial fibrillation
Ventricular tachycardia
Ventricular fibrillation
Atypical atrial flutter
Fibrous and/or fatty myocardial replacement
Atrial standstill
Ventricular tachycardia
Occasionally, the electrical insulation of the fibrous skeleton between the atrium and ventricle is interrupted by an accessory atrioventricular pathway. This muscular bundle is also known as a bypass tract because it allows electrical impulses to bypass the atrioventricular node, and travel from atrium to ventricle, or retrogradely from ventricle to atrium. Accessory pathways are responsible for the occurrence of atrioventricular reciprocating tachycardia.
Various forms of ventricular tachycardia circuits are associated with the presence of myocardial fibrosis secondary to cardiomyopathies, including dilated cardiomyopathy, hypertrophic cardiomyopathy and arrhythmogenic right ventricular cardiomyopathy (Fig. 1.6).
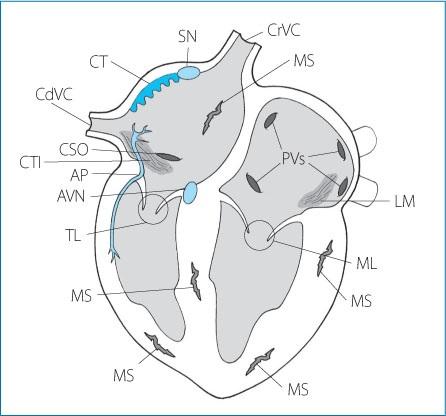
Figure 1.6. Anatomy of the principal sites involved in the genesis of cardiac arrhythmias. SN: sinus node; CT: crista terminalis, CrVC: cranial vena cava; CdVC: caudal vena cava; PVs: pulmonary veins; CTI: cavotricuspid isthmus; CSO: coronary sinus ostium; AVN: atrioventricular node; LM: ligament of Marshall; TL: tricuspid leaflets; ML: mitral leaflets; AP: accessory pathway; MS: myocardial scars.
The action potential
Resting membrane potential
All cell membranes are polarized. The resting membrane potential of cardiomyocytes corresponds to a difference in electrical charge between the intracellular and extracellular space in the absence of an electrical impulse. At rest, the cell membrane is impermeable to Na+ and partially permeable to K+ and Cl- ions. The Na+ concentration is lower in the intracellular space in part because of the continuous activity of the Na+/K+ pump, which hydrolyses ATP to pump Na+ out of the cell and move K+ into the cell. Potassium concentration therefore remains higher in the intracellular space. Chloride concentration is higher in the extracellular compartment, which promotes the passive diffusion of Cl- into the cell. As a result of the increased permeability to K+, the resting transmembrane potential of most cardiomyocytes (working myocardium and Purkinje cells) varies between −80 mV and −90 mV, which approaches the Nernst potential for K+. A true resting potential does not exist in the P (pacemaker) cells of the sinus node and the atrioventricular node, as these cells continuously depolarize between two action potentials. The lowest transmembrane potential varies from −50 mV to −70 mV in the P cells because of a different expression of ion channels on their surface compared with that of other myocytes (Fig. 1.7).
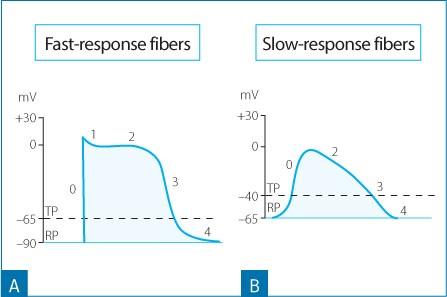
Figure 1.7. Changes in the transmembrane potential value of the fast-response fibers (A) and the slow-response fibers (B). Note how the fastresponse fibers show lower values of the resting transmembrane potential (RP) and the threshold potential (TP), a steeper phase 0 of the action potential and a larger amplitude compared to the action potential characteristics of the slow-response fibers. Phase 1 is absent in the slow-response fibers.
Any stimulus, whether it is from a P cell or an external electrical impulse that is able to alter the transmembrane potential to a critical value, called the threshold potential, can trigger an action potential in the neighboring cells. The threshold potential of the nodal cells is −40 mV, while it is −65 mV to −70 mV in the Purkinje cells and working myocardium.
Phases of the action potential
There are two types of action potentials: “fast response” and “slow response” (Fig. 1.7). The fast response action potential is found in atrial and ventricular working cardiomyocytes and in the cells of the atrioventricular conduction axis, with the exception of the compact node. The slow response action potential is characteristic of the P cells of the sinus node and the compact node (Figs. 1.7 and 1.8).
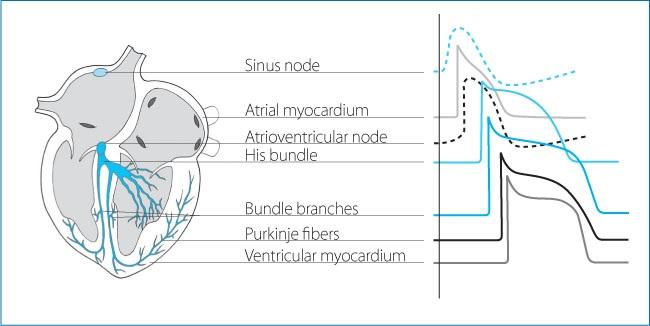
Figure 1.8. Morphology of the action potential in different regions of the conduction tissue, the atrial and ventricular working myocardium. Note the absence of phase 1, the reduction in the slope and amplitude of phase 0, the shorter action potential duration, and spontaneous phase 4 depolarization in the pacemaker cells of the sinus node and the atrioventricular node, compared to the working atrial and ventricular myocardium.
Phases of the fast response action potential
The action potential is divided into five phases: Phase 0 or rapid depolarization. Because the cell membrane is polarized at rest, the first phase of the action potential is depolarization (a change from more negative to less negative and even positive values). It starts with a rapid rise of the transmembrane potential (rate of depolarization of 100-200 V/s in the atrial and ventricular myocytes, and 500-1000 V/s in the fibers of the His-Purkinje system) to a peak voltage of approximately +30 mV that corresponds to a Na+ (INa) current caused by the rapid influx of Na+ through voltage-gated Na+ channels (Nav1.5) and the simultaneous decreased permeability of the membrane to K+. The structure of the Na+ channels includes two “gates”, described by Hodgkin and Huxley; one called the m or activation gate, and the other called the h or inactivation gate. During the resting phase between two action potentials (phase 4, also called diastolic interval), the flux of Na+ across the membrane is blocked because m gates are closed. The voltage-dependent gating of the Na+ channels means that the m gates quickly open when the threshold potential of −65 mV is reached, allowing the rapid influx of Na+ into the cell driven by the concentration gradient. The transmembrane voltage rapidly reaches a value between 0 mV and +40 mV. The transition from a negative transmembrane voltage to a positive one is called the overshoot phase. At this point the flux of Na+ ions stops as a result of the closure of the h gates, which reflects the time-dependent gating property of the channel. The h gates remain closed until the transmembrane potential returns to resting values, preventing any further exchange of Na+ across the membrane until the end of the action potential. Phase 1 or early repolarization phase. This phase is a brief period of repolarization associated with a potassium current (transient outward current or Ito) corresponding to an efflux of K+. The transmembrane potential returns to approximately 0 mV at the end of phase 1. The transient outward current is stronger in the epicardium than the endocardium. When phase 1 is amplified the action potential duration is prolonged, and it is shortened if phase 1 is attenuated. Indeed, the transmembrane voltage at the end of phase 1 determines the magnitude of the Ca2+ current during the subsequent phase of the action potential (phase 2). A calciumactivated chloride current also contributes to phase 1. Of special note in the dog is the ion channel Ito. Ito can be prominent in the epicardium of the dog and is responsible for the J wave that is seen in the terminal portion of the downstroke of the R wave particularly in leads II, III and aVF. The expression of this particular ion channel is not fully mature in the dog until approximately 4 to 5 months of age. Consequently, J waves are most commonly seen in mature dogs. Moreover, this particular deflection varies in breeds. In human beings this may be an indication of an ominous electrophysiologic situation or induced by hypothermia, and although such situations can exaggerate the J wave in the dog, it is a normal finding (Figs. 1.9 and 1.10).
Phase 2 or plateau. This phase is characterized by an influx of Ca2+ through voltage-gated L (long-lasting)type Ca2+ channels. L-type Ca2+ channels open when the transmembrane potential is approximately −10 mV. The depolarizing current ICa-L secondary to the influx of Ca2+ is rapidly balanced by the contribution of two outward currents (a larger current IKs and a smaller current IKr). The small influx of Ca2+ during the plateau phase triggers the release into the cytoplasm of large amounts of Ca2+ stored in the sarcoplasmic reticulum, which subsequently triggers myocardial contraction, a phenomenon known as excitation-contraction coupling. During the final phase of the plateau, the transmembrane potential value decreases gradually in response to a reduction in the conductance for Ca2+, and a simultaneous increase in the conductance for K+, thus initiating phase 3 of the action potential, or repolarization.
Phase 3 or final repolarization. This phase results from at least three K+ currents (IKs, IKr, IK1) associated with an efflux of K+. IKr is the major contributor of phase 3, which ultimately brings the transmembrane potential back to resting values. IKur is a potassium current only measured in the atrial myocytes which contributes to repolarization and is responsible for the shorter action potential duration in the atrial myocytes than in the ventricular myocytes.
Phase 4 or resting phase. During this phase, the membrane potential is back to resting values. The intracellular concentration of ions is restored by ionic pumps (Na+/K+-ATPase, Ca2+-ATPase) and the Na+/Ca2+ exchanger. In the atrial, His-Purkinje and ventricular cells, the value of the resting membrane potential is mainly determined by the high conductance for K+ ions through IK1 channels.
Phases of the slow response action potential
The slow response action potential is a characteristic of not only myocytes in the sinus and atrioventricular nodes but also many other regions of the heart. These myocytes have in common the expression of the transcriptional inhibitor Tbx3. There are several major differences between the slow response and the fast response action potential:
The membrane potential is less negative during phase 4 of the action potential because of the absence of the
potassium channel Kir2 that is associated with the IK1 current and is responsible for maintaining the resting membrane potential at –90 mV.
A stable resting membrane potential does not exist during phase 4, as slow depolarization starts immediately after the end of the preceding action potential (diastolic depolarization). Two mechanisms are responsible for the spontaneous depolarization that characterizes the slow response action potential: the “membrane or voltage clock” and the “calcium clock”. The “voltage clock” corresponds to the progressive reduction in repolarizing currents via the closure of potassium channels at the end of an action potential and several depolarizing currents: If current from the activation of HCN channels leading to an influx of Na+; and ICa,T and ICa, L currents from the activation of calcium channels leading to an influx of Ca2+. The “calcium clock” is initiated by the spontaneous release of Ca2+ from the sarcoplasmic reticulum through the ryanodine receptor which triggers an influx of Na+ and an efflux of Ca2+ in a 3:1 ratio (3 Na+ for 1 Ca2+) through the transmembrane Na+/Ca2+ exchanger. The “calcium clock” mainly contributes to the final portion of phase 4. Phase 0 is dependent on a calcium current (ICa,L), and due to the absence of voltage-gated Na+ channels (Nav1.5) the upstroke of phase 4 is not as steep as it is in regular myocytes. The threshold potential of phase 0 is −40 mV and its slow upstroke results in a low conduction velocity. There is no phase 1.
Finally, pathologic states, especially ischemia, can lead any cardiomyocyte to develop the characteristics of slow response cells and depolarize spontaneously (abnormal enhanced automaticity).
The pacemaker current If is a major contributor of spontaneous automaticity in the sinus node and the atrioventricular junction. It also appears to participate in the diastolic depolarization of Purkinje cells, although this complex process likely involves other factors, including intracellular Ca2+ cycling and a K+ current called IKdd. It is possible, however, that IKdd and If represent the same current.
Transmural dispersion of action potential Epicardial myocytes exhibit an action potential with a prominent phase 1 and a doming shape. Myocytes in the mid-myocardium, referred to as M cells, have a prominent and clearly visible phase 1 and a longer action potential compared to the myocytes of the epicardium and the endocardium. Finally, endocardial myocytes have an action potential with a small phase 1 and a duration intermediate between epicardial and midmyocardial cells.
These differences in duration and morphology of the action potentials reflect different expressions of Ito and IKs potassium channels: Ito channels are present in large numbers in epicardial myocytes, to a lesser extent in the M cells and are almost absent in the endocardial myocytes. The reduced number of IKs channels in M cells explains their prolonged phase 2.
The differences in amplitude and duration of the action potentials generate a transmural electrical gradient during repolarization of the heart. The resulting electrical heterogeneity between layers of myocardium can serve as the substrate for arrhythmias, via a mechanism called reentry (see p. 99).
Correlation between the phases of the action potential and electrocardiographic waves
The QRS complex of the surface electrocardiogram represents the manifestation on the body surface of the algebraic sum of two action potentials with different morphology, one deriving from the activation of the subendocardial myocytes and one resulting from the activation of subepicardial myocytes. The action potential of the subendocardial cells starts and ends earlier than the action potential of the subepicardial cells since depolarization follows an endocardium to epicardium direction, while repolarization progresses from the epicardium to the endocardium. The correlation between the monophasic action potential and electrocardiographic waves is as follows (Fig. 1.9): Phase 0 - beginning of the QRS complex; Phase 1-J point; Phase 2 - S-T segment;
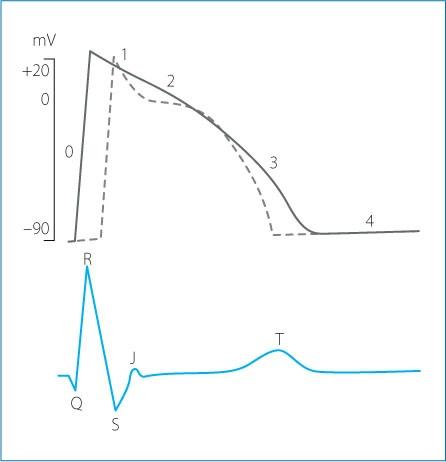
Figure 1.9. Correlation between the phases of the transmembrane action potential and electrocardiographic waves. The solid line represents an action potential in the subendocardial cells, while the dotted line corresponds to an action potential recorded from the subepicardial cells.
Phase 3 - T wave; Phase 4 - electrical diastole.
On the electrocardiogram, the duration of the action potential of ventricular epicardial cells is approximately from the onset of the QRS complex to the peak of the T wave and for the mid-myocardial cells, it is from the onset of the QRS complex to the end of the T wave. The transmural dispersion of repolarization can be approximated by the interval from the peak to the end of the T wave. As described previously, the heterogeneity of repolarization within the ventricles can become the substrate for arrhythmias. The risk of arrhythmias is maximum during a brief portion of ventricular repolarization, called the vulnerable period, which corresponds to the peak of the T wave in lead II of the surface electrocardiogram in dogs. A stimulus of adequate intensity delivered during the vulnerable period can trigger ventricular fibrillation. There is also a vulnerable period for the atria which corresponds to the descending branch of the R wave or the S wave.
Electrical properties of the myocardium and their relationship with the action potential
Several properties characterize the myocardium: excitability, automaticity, refractoriness and conduction velocity.
Excitability is the ability of a cell to generate an action potential as a result of a stimulus equal to or above the membrane threshold potential. Excitability depends on the availability of Na+ channels to open in response to a stimulus.
Automaticity is the ability of a cell to spontaneously generate an action potential. Automaticity is a characteristic of the sinus node (the leading pacemaker) and subsidiary pacemakers, including various areas in the atria, the ostia of pulmonary veins, the coronary sinus, atrioventricular valves, portions of the atrioventricular conduction axis and the His-Purkinje system. These subsidiary pacemakers are usually latent because they are inhibited by the faster rate of the sinus node (overdrive suppression). Working atrial and ventricular myocytes do not have the property of automaticity, and only generate an action potential if triggered
by an adequate stimulus.
Refractoriness or refractory period is a period of time when myocytes are non-excitable, and it extends from phase 0 to the end of phase 3 of the action potential. The refractory period is due to the inactivation of the Na+ channels soon after the onset of the action potential (Fig. 1.10). The total refractory period can be divided into effective and relative refractory periods:
The effective refractory period is defined as the period beginning with phase 0 of the action potential and ending during the repolarization phase (approximately halfway during phase 3), when an appropriate electrical stimulus cannot evoke another action potential. Indeed, when the membrane is depolarized to a value of −50 mV or less, all Na+ channels are inactivated, and therefore an action potential cannot be initiated. Electrocardiographically the effective refractory period of the ventricles begins with the QRS complex and ends at the beginning of the T wave.
The relative refractory period is the time between the end of the effective refractory period and the end of the action potential. It, therefore, extends from the mid-portion to the last portion of phase 3. This period corresponds to the progressive re-activation of the Na+ channels that occurs when the membrane potential returns below −50 mV. Na+ channels have fully recovered when the membrane potential reaches −90 mV. During the relative refractory period, myocytes can respond to very intense stimuli that initiate action potentials with a decreased upstroke velocity (phase 0) due to the low number of Na+ channels available. As a result, impulse conduction is slower within the myocardium. On the electrocardiogram, the relative refractory period of the ventricles corresponds to the initial portion of the T wave.
The phase of supernormal excitability is a short period after the relative refractory period when a subthreshold stimulus can elicit an action potential. The action potential generated is not “better” than normal, but instead unexpected, because the same stimulus would fail to initiate an action potential if delivered just before or just after the supernormal phase. The phase of supernormal excitability corresponds to the period when the membrane potential is close to the membrane threshold potential, as it returns to diastolic values.
The sum of the effective refractory period and the relative refractory period represents the total refractory period. The difference in refractory period between cardiac cells within the conduction system and the different layers of the myocardium limits the risk of retrograde conduction.
Changes of the cardiac cycle length (heart rate) alter the duration of the action potential, and therefore the refractory period: an increase of the cycle length (slower heart rate) results in an increase of the action potential duration; conversely, a shortening of the cycle length (faster heart rate) is associated with a decrease of the action potential duration. The potassium currents responsible for this mechanism are IKS and Ito
Conduction is the property of cells to propagate an impulse from one cell to another. The conduction characteristics vary between fast response fibers and slow response fibers. In the fast response fibers, conduction velocity is proportional to the intensity of the Na+ current during phase 0 of the action potential (slope of phase 0) and the maximum diastolic potential value (or resting potential). The slope of phase 0 corresponds to the rate of change of the membrane voltage over time (dVm/dt). An increase in the slope of phase 0 causes an increase in conduction velocity along the cell. The value of the resting membrane potential determines the number of Na+ channels available at the onset of phase 0 (100 % for a resting membrane potential of −90 mV, 50 % for a resting membrane potential of −75 mV and 0 % for a resting membrane potential of −40 mV). A more negative resting potential results in a larger amplitude phase 0 and higher conduction velocity along the myofiber. In addition to the slope and amplitude of phase 0 of the action potential, other factors that contribute to conduction velocity include the diameter of the cells, the number of intercalated discs between cells, and the type of connexin that forms the gap junctions. Conduction velocity of the fast fibers varies from 0.8 to 1 m/s for the atrial and ventricular myocytes, and reaches 1 to 5 m/s in specialized conduction tissue.
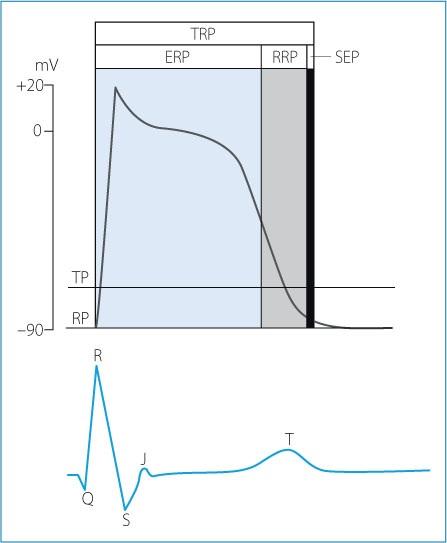
Figure 1.10. Ventricular action potential, refractory periods and relationship with the surface electrocardiogram. ERP: effective refractory period; RRP: relative refractory period; SEP: supernormal excitability period; TP: threshold potential; RP: resting potential; TRP: total refractory period.
Conduction in the nodal tissue (slow-response action potential) is much slower, between 0.05 and 0.1 m/s. It results from the slow depolarization phase associated with an influx of calcium, the large amount of connective tissue with nodal tissue, the small diameter of the intranodal myocytes, the low number of intercalated discs between cells and the composition of the gap junctions (mostly connexin 45).
Spontaneous automaticity of pacemaker cells
Pacemaker cells present in the sinus node, AN and NH regions of the atrioventricular node, the bundle of His and the Purkinje fibers have the ability to spontaneously depolarize and generate a regenerative action potential in the absence of an external stimulus. The membrane potential progressively changes to less negative values during phase 4 until it reaches the threshold potential for the initiation of an action potential.
The rate of spontaneous depolarization is modulated by three main factors: the slope of phase 4 depolarization, the threshold potential and the membrane potential at the initiation of phase 4 (Fig. 1.11A):
An increase in the slope of phase 4 allows the membrane potential to reach the threshold potential sooner, which leads to an increase in the discharge rate of the pacemaker (increased heart rate); conversely, a decrease in the slope of phase 4 prolongs the time needed to reach threshold, causing a reduction in the discharge rate of the pacemaker (Fig. 1.11B).
A shift of the threshold potential towards less negative values delays the onset of phase 0 of the action potential, causing a reduction in the pacemaker discharge rate, while a shift of the threshold potential towards more negative values results in an earlier onset of phase 0, causing an increase in the rate of the pacemaker (Fig. 1.11C).
A less negative membrane potential at the initiation of phase 4 makes it easier to reach the threshold value, and results in an increase of the discharge rate of the pacemaker. Alternatively, if the membrane potential is more negative at the beginning of phase 4 (hyperpolarized), the discharge rate of the pacemaker decreases (Fig. 1.11D).
Under normal conditions, the rate of discharge of the sinus P cells is higher than the intrinsic rate of the P cells in other parts of the heart (i.e. atrioventricular node, bundle of His and Purkinje network). In dogs, the rate of spontaneous depolarization of the P cells is 70 to 160 bpm in the sinus node, 40 to 60 bpm in the AN and NH regions of the atrioventricular node, and 15 to 40 bpm in the Purkinje fibers (Fig. 1.12).
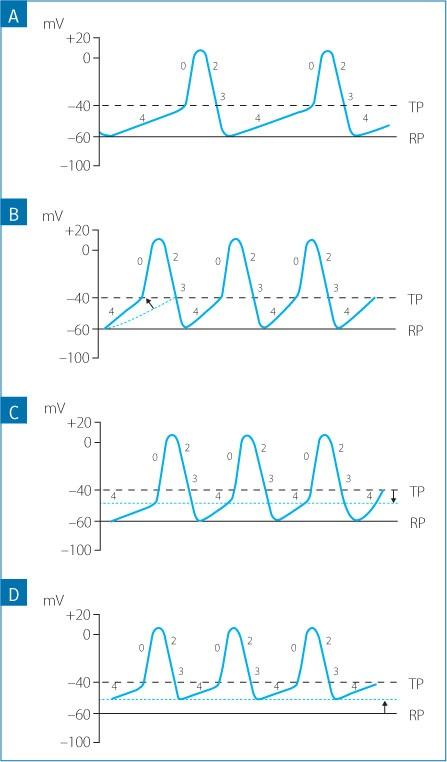
Figure 1.11. Mechanisms involved in the control of the firing rate of the pacemaker cells of the sinus node. A) Normal firing rate of the sinus node. B) An increase in the slope of phase 4. C) A lower (more negative value) threshold potential. D) A less negative membrane voltage at the onset of phase 4 depolarization. The increase of the depolarization rate of the pacemaker cells is usually caused by a combination of these
Another random document with no related content on Scribd: