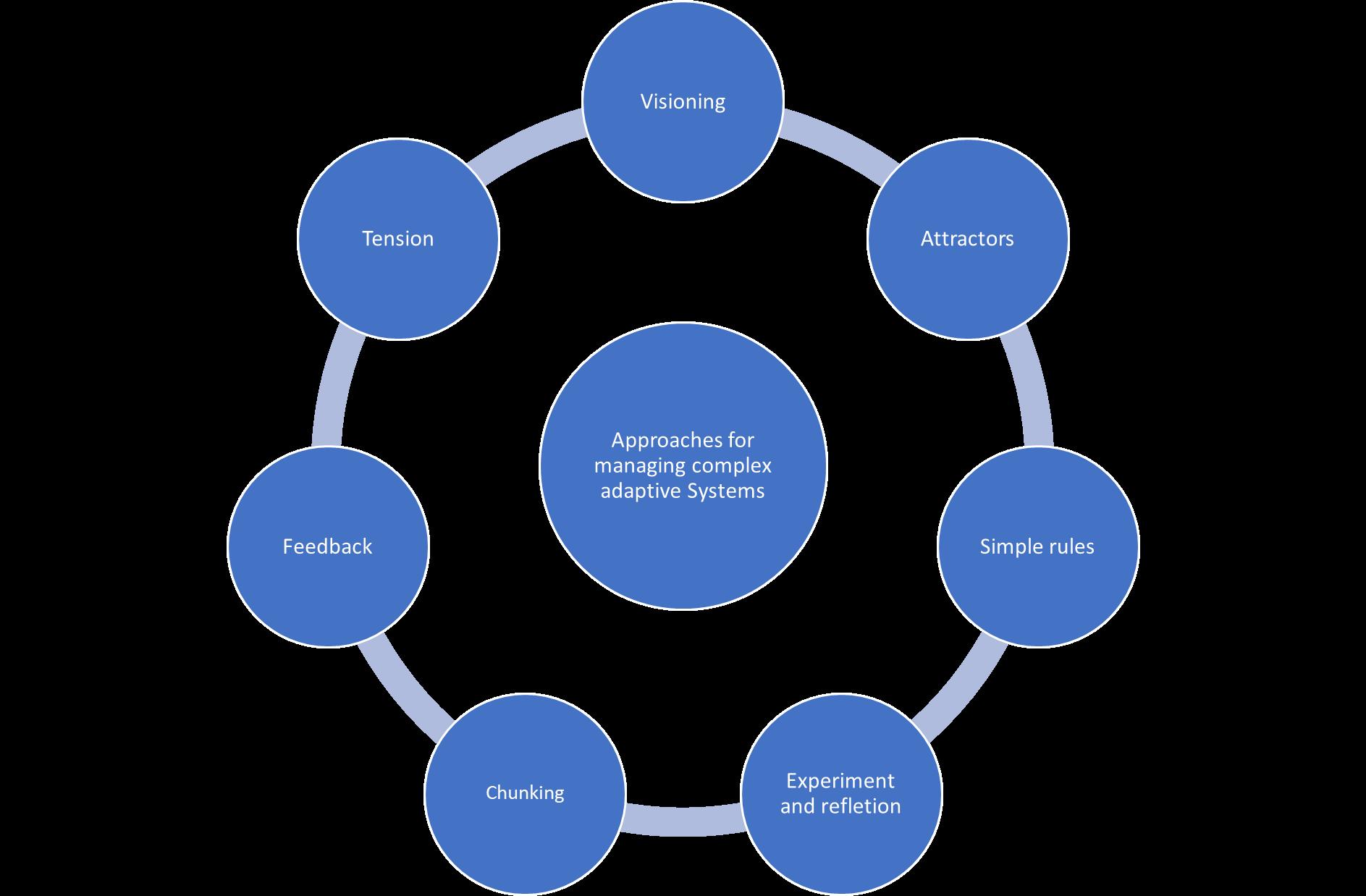
4 minute read
D. Characteristics of liquid fraction of digestate
Table 43 Chemical composition of liquid fraction of anaerobic digestate obtained under different process conditions and feedstocks.
Cattle slurry (50%), pig slurry (35%), cattle slurry, poultry manure (5%), sorghum silage and corn flour (10%)
Cow manure (29%), cow slurry (29.7%), pig slurry (29.7%) and poultry manure (11.6%)
Swine manure (73 8%), corn silage (16.4%), triticale silage (8.2%) and maize flour (1.6%)
Cattle slurry (33%), farmyard manure (24%), maize silage (26%), triticale silage (11%), drying maize residue (3%) and kiwi (3%) et al., 2015) et al., 2016)
2016) Cattle slurry (23%), farmyard manure (30%), energy crops (27%) and agricultural byproducts (20%)
E. System transition background
Complex adaptive systems
The generation, distribution, marketing and consumption of energy in Australia can be conceptualised as a complex adaptive system (CAS) (Figure 25). CAS are made up of a set of connected or interdependent agents or stakeholders (i.e., an organisation, department, team or individual) that respond to a dynamic environment in unpredictable, often non-linear ways (Palmberg, 2009). The control of the system is distributed across stakeholders, who adapt, learn and self-organise through co-evolution in response to drivers of change (Holland, 2006; McCarthy et al., 2007; Zimmerman et al., 1998). The system’s ability to learn and adapt means that its behaviours emerge over time rather than through system design (Smith & Stirling, 2008). For example, Bale et al. (2015) considered stationary energy systems as CAS, and Pearson and Bardsley (2022) analysed the need for adaptation to climate change in stationary energy using a CAS approach. However, almost every biological, economic and social system is a complex adaptive system (CAS) (Ahmed et al., 2005)
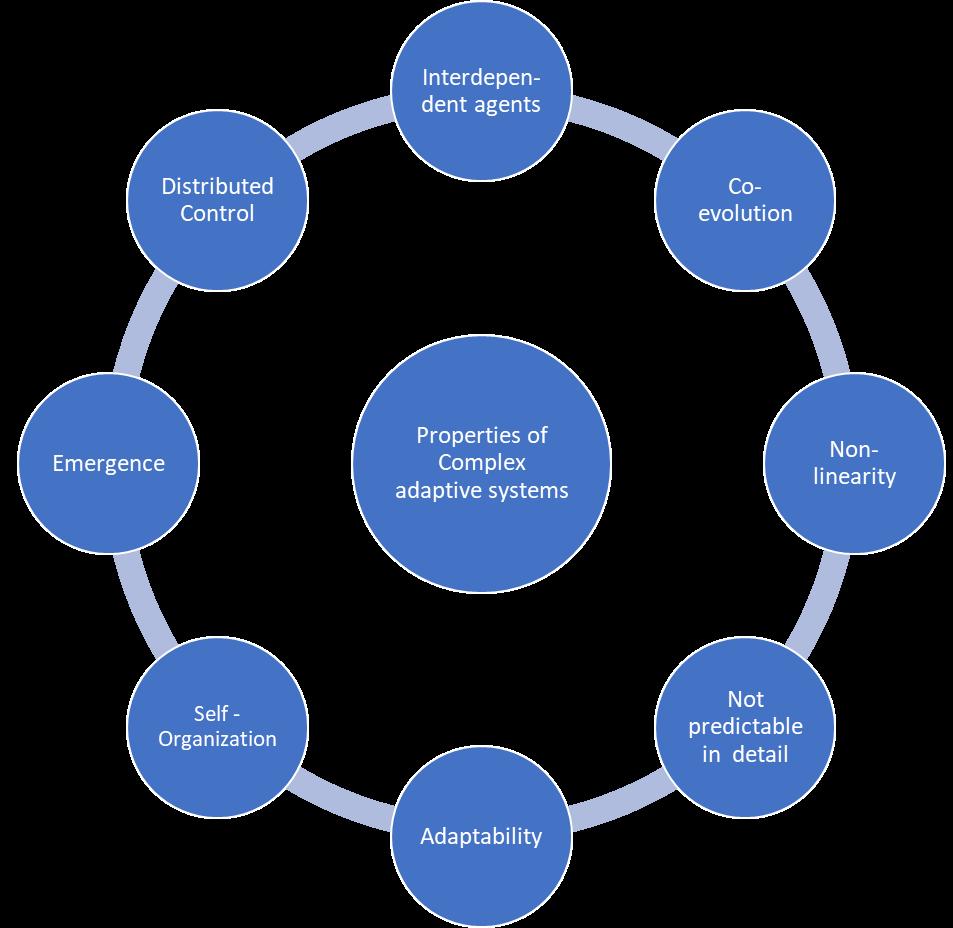
Management of energy systems as CAS is problematical as there are no known mechanisms capable of mapping, in advance, the interdependencies among political (governance, legislative, policy and institutional arrangements), technical (production, distribution, storage and utilisation), environmental (resource, risk and climate), social (livelihoods, energy poverty and social practices) and economic (consumers and the energy market) elements (Di Maio, 2014; Pearson & Bardsley, 2022), which requires unique approaches that influence rather than control behaviour (Figure 25).
Socio-technical systems (STS)
The interaction of engineered systems with society, such as in energy systems, represents a particular class of CAS known as socio-technical systems (STS). An STS perspective is used to study the development and use of technology as a complex adaptive process where materials and people interact (Smith & Stirling, 2008). STS are open systems that may operate at a range of scales, and larger systems can be composed of interacting sub-systems (nested systems) meaning determining system boundaries is often problematical (Di Maio, 2014) (Figure 26). For example, Australia’s energy system, is composed of multiple socio-technical subsystems (e.g., fossil-fuel, renewable energy and so on). While renewable energy can be conceptualised as an STS, it in turn can be considered as composed of several interacting socio-technical subsystems – solar, wind, geothermal and bioenergy – based on specific (sometimes multiple and competing) technologies. Given the properties of CAS described above, understanding the potential for rapid dynamic reconfiguration in STS (a process of transition) has been conceptualised through a number of methods like the technical-economic perspective (TEP) or the multi-level perspective (MLP) (Wilkinson et al., 2021)
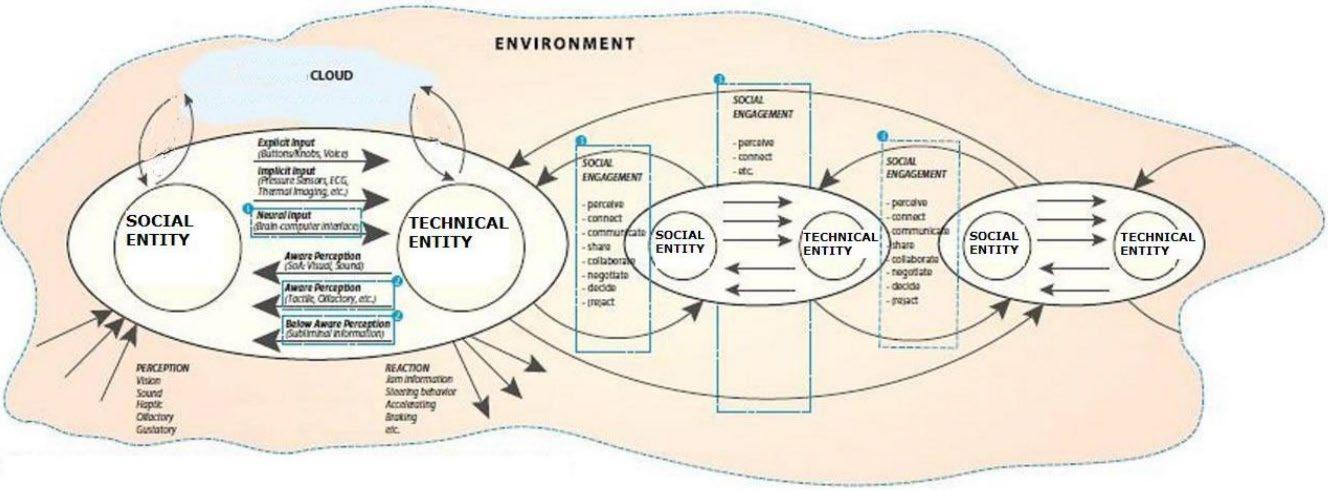
Conceptualising socio-technical transitions
The use of STS perspectives to explore sustainable technologies, such as for decarbonising energy systems, aligns with the recognition that there is no ‘silver bullet’ technological solution, rather, structural changes will be required in social, economic, political and infrastructure systems (Smith & Stirling, 2008)
Because the stakeholders in an STS pursue a range of goals, strategies and plans, designing incentives and rules that influence adaptive behaviour may lead to tipping points or thresholds of adoption (Wruck, 2000) and, ultimately, system transformation. The process by which societal transformation occurs, either incrementally or abruptly, is defined as transition. Transition management, often driven by the desire to improve system sustainability, is a model of system governance that considers and incorporates an understanding of complex systems theory to deliberately intervene in an STS to promote change (Kemp & Loorbach, 2006; Shove & Walker, 2007) Table 44 contrasts the transition management approach with a market-based mechanism to promote sustainable energy transitions in Australia (Rosenbloom et al., 2020)
Carbon pricing strategies
Sustainability transition policy
Conceptual roots
Problem framing and solution orientation
Overriding policy priority
Innovation approach
Contextual considerations
Understanding of politics
Neoclassical economics
Climate change as a market failure problem: price carbon to correct market signal
Efficiency: reduce carbon emission while keeping the economy wide cost at a minimum
Incremental change, indirect simulation of innovation
Universality: carbon pricing for all jurisdictions and sector
Revenue recycling to deal with political realities
Innovation studies, evolutionary economics, institutional theory
Climate change as a system problem: fundamentally transform existing sociotechnical systems
Effectiveness: drive down emissions as quickly as possible
Transformative change, direct stimulation of innovation
Tailoring: policies should be adapted to local and sectoral contexts
Creation of alternatives and formation of supportive coalitions
While transition management appears to provide a mechanism to promote transition, the uncertainty and unpredictability of complex systems suggest that deliberate management to control all of the system elements (e.g. stakeholders, resources stocks and flows, shared vision, thresholds of change) and to ensure that the transformed system has desired attributes is at best extremely difficult (Shove & Walker, 2007)
Although other models of change exist (e.g. the Technological Innovation System approach, Strategic Niche Management and Transition Management) (Köhler et al., 2019), the MLP is a mature, heuristic framework (Geels, 2022) widely applied to empirical studies of change (such as deliberate transition management) in socio-technical systems across diverse knowledge domains (e.g., autonomous vehicles, Fraedrich et al. (2015); phosphorus circularity, Jacobs et al. (2017); and energy systems, Kanger (2021)). The MLP conceptualises transitions as occurring through processes of interaction within and among three analytical levels: niches (the sites of innovation), socio-technical regimes (networks and rules etc. of the incumbent system) and a socio-technical landscape made up of drivers of societal change (Figure 27). Arranz (2017) associates change at the regime level most often with new or visibly changed political manoeuvring (e.g., lobbying, election promises), socio-cultural understanding (e.g., lifestyle choices, fashion), or economic variables (e.g., prices, competition). It appears that the combined weight of multiple factors is more important to regime destabilisation than any single factor alone (Arranz, 2017) Pressure from the landscape level on the regime can open opportunities for niches to form around technological innovations. The potential for disruption or destabilisation by the innovation is generally resisted, actively or passively, by the existing regime (Arranz, 2017). However, over time, through a range of pathways (reproduction, transformation, dealignment-realignment, technological substitution, reconfiguration) change in the regime (Geels & Schot, 2007) may allow the technology to become established.
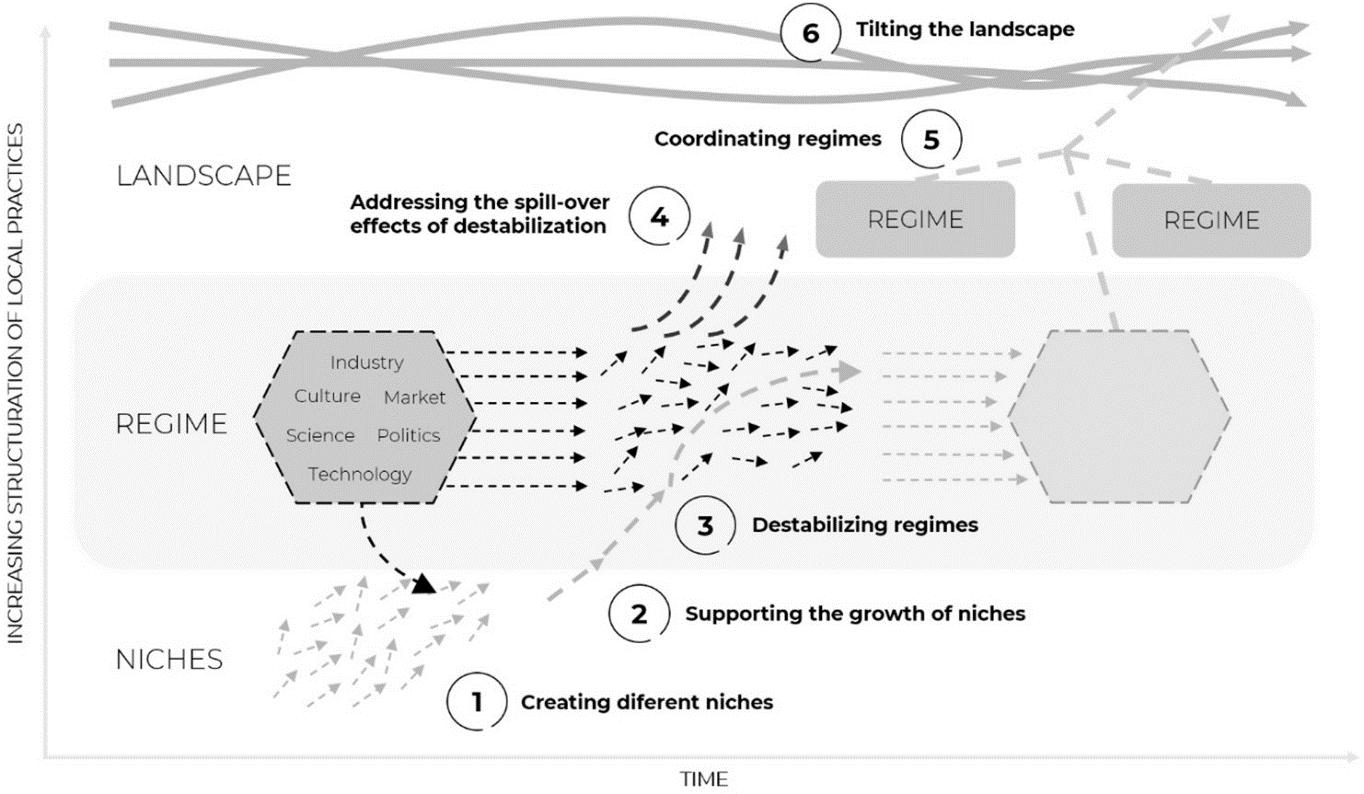