
8 minute read
Time for deeper re-assessment of the levelized cost of RFB storage
Redox flow batteries — with their high up-front costs but lower running expenses — may be more financially viable than expected. Kara Rodby, venture capital technical analyst at Volta Energy Technologies, shares details of her research into the subject.
Time for deeper re-assessment of the levelized cost of RFB storage
Lithium ion batteries may be the chemistry of choice for most grid services, but that doesn’t mean that they will be a cost-competitive solution for all applications — particularly emerging longer-duration ones.
Among the various energy storage technologies under development, redox flow batteries are an emerging solution for long duration (that is four hours and longer), stationary applications as their system architecture offers a number of unique advantages.
A RFB generally uses the potential difference between two reduction/ oxidation reactions — known as redox — to drive chemical reactions, thus converting electrical energy to and from chemical energy.
In the RFB architecture in particular, electrolyte (the charge-storing capacity, usually in the liquid phase) is stored in tanks and pumped through the reactor where electrochemical reactions occur.
This open architecture — the physical separation of the electrolyte and the reactor components — decouples energy and power. One can scale the energy capacity by increasing the tank size independently of the power rating (meaning the reactor size can stay fixed). This means RFCs can be designed for virtually any duration. This process of scaling the electrolyte tanks to meet longer durations, while keeping all else fixed, is depicted pictorially in Figure 1 below.
This decoupling is a unique and distinct feature from many conventional battery technologies. This facilitates a number of unique economic benefits, including the decrease of capital costs on a per unit energy basis at increasing durations and long-term cost savings through component-specific maintenance to extend the battery lifetime and remediate decay.
Capital cost is a very important metric for evaluating the economic feasibility of batteries, the most cited being the US Department of Energy’s target for viable grid storage of ≤150 $/kWh: the exact target varies by office within the DOE, and is often even below 100 $/kWh. This is because the capital cost is the primary barrier to adoption: grid-scale storage systems are large capital investments, so high capital costs hinder the ability to finance and install such systems.
The capital cost (Ccapital) of a battery is generally defined as the sum of the energy costs (Cenergy) and the power costs (Cpower), the latter of which is divided by the duration to give consistent units of dollars per unit energy:
For RFBs, the energy costs consist of the electrolyte comprising the active species, solvent, and supporting electrolyte) and tank, while the power costs encompass the stack primarily the reactor, the most important components of which are the membrane and electrodes.
Other balance-of-plant costs, for example, pumps, grid connections and additional costs such as labour, installation, profit margins and the like may or may not be included in these calculations, depending on the study. These are usually represented as power-based costs — they have units of dollars per kW and thus are also divided by the battery duration if summed and included in capital cost calculations.
The equation obviously implies that energy and power costs can be decoupled, which is how the capital cost is reduced as a function of duration. This is true for RFBs, which is why they are particularly attractive for longer duration applications.
This is not the case for many conventional technologies: for example, in lithium batteries the
Figure 1: A representative schematic of capital cost as a function of characteristic discharge time (or duration) for RFBs and lithium batteries. As duration increases, the capital cost of decoupled battery architectures shifts to be dominated less by power costs and more by energy costs
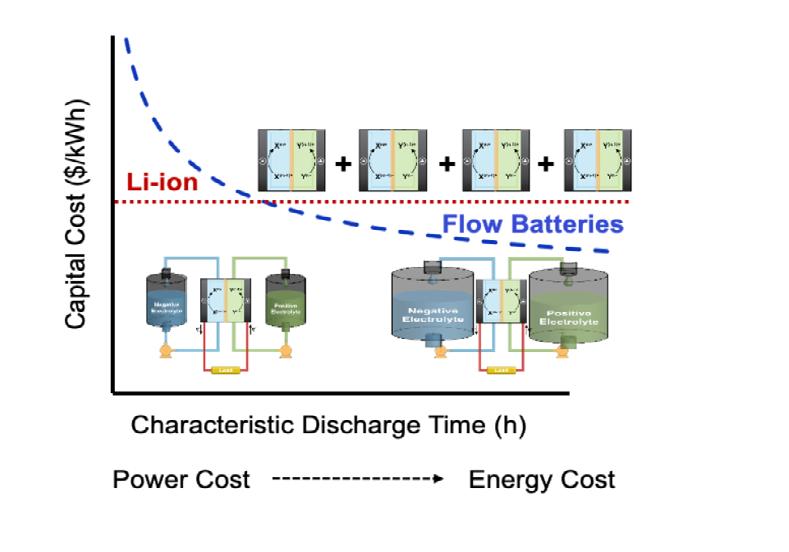
electrode is part of determining both the power output (its electrochemical properties determine the rate of the electrochemical reactions) as well as the energy capacity (its morphology dictates how many ions can intercalate into it for storage).
Thus, there is little room to modulate the ratio of the energy or power ratings, as these are based on physical materials limitations. Rather, increasing duration must be achieved by adding entire modules (as shown pictorially in Figure 1), meaning the total capital cost is approximately fixed (on a per unit energy basis), even with varying duration.
While the capital cost savings at longer durations is a key selling point of RFBs over other battery technologies, there is still minimal deployment of RFBs on the grid.
Despite the large body of literature on RFBs (there are around 4,500 publications, with more than 600 of them a year) RFBs only account for a couple or percentage points of the energy storage systems that have been contracted, announced, or are under construction globally.
The low deployment rate is a result of the nascency of the technology, which creates a chicken-and-egg problem: no one wants to finance a seemingly risky project, but we also cannot de-risk a technology without financing initial demonstrations.
The lithium battery industry avoided this situation via higher revenue beachhead markets: by the time it was ever considered for the grid, Li-ion had already demonstrated improved performance (its roundtrip efficiency is now remarkably high, usually about 90%, compared to RFBs which are generally 80% or lower, depending on the chemistry). There were also lower costs from development and deployment in the relatively large industries of electronics and electric vehicles.
RFBs, however, are only attractive for the medium to long duration grid applications, for which demand is still low. Thus, they are struggling to compete on a cost basis at such low production volumes.
This situation is representative of a number of other nascent storage technologies (for example, metal air batteries). With a need to decarbonize the power sector rapidly to meet targets for 2030, 2050, and beyond, many of these nascent technologies will play a vital role in supporting the array of grid services that exist.
One crucial avenue needed to solve this problem is to develop new technoeconomic models and arguments to motivate the development and, especially, deployment of these more nascent technologies. For example, RFBs offer a lot of long-term operational benefits that save costs, and these are not covered in capital cost calculations.
While the capital cost benefits to RFBs are well-described in the open literature, there are further economic benefits in operation due to ease of maintenance of open systems that are often not captured in techno-economic discussions.
For example, crossover — defined as undesirable active species transport through the semi-permeable membrane that separates the positive and negative electrolytes in the reactor – is usually the most dominant form of capacity fade in RFBs and may halve the accessible capacity within 100-200 cycles.
While this is a relatively fast fade as compared to that typically experienced by LIBs, the open architecture of RFBs allows for targeted, cost-efficient maintenance: in an RFB, one can access the electrolyte (the component affected by crossover) to repair or replace it directly, without altering the still-viable reactor.
This is unlike conventional closed systems such as lithium batteries that require “augmentation” — replacement or addition of entire new modules — and which wastes undecayed reactor components. The costs necessary to maintain performance over time impact the battery’s economic viability, but are not captured in the conventional capital cost estimations that are commonly used to compare and benchmark different technologies. This is especially true for the vanadium RFB (VRFB), which is by far the most researched and deployed RFB chemistry.
VRFB is particularly favoured for its strong performance and resiliency in dealing with electrolyte degradation, which is facilitated by its design as a “symmetric” chemistry, where all active species are based on a single parent compound.
The benefit of this chemical configuration is that the inevitable crossover of active species becomes much less detrimental, as it will not lead to cross-contamination and these losses can be recovered via rebalancing: the transfer and recharging of partial or full volumes of electrolyte between the two reservoirs to balance the concentrations of active species.
Rebalancing allows for indefinite crossover remediation and, thus, lifetime utilization of the same, original electrolyte (assuming other forms electrolyte degradation are managed). Not many chemistries can be configured in a symmetric configuration, and while a handful of chemistries have been demonstrated symmetrically, vanadium remains the most promising and explored of them all.
The long-term cost benefits are primary motivators of RFB development, yet they are not captured in the vast majority of prior technoeconomic modelling literature which has primarily focused on articulating upfront capital costs.
This motivates the development of techno-economic models that consider the variable operating principles of different battery formats and chemistries. To this end, the central thread of this is a levelized cost of storage (LCOS) model that has been developed by this author to model lifetime RFB costs that incorporates capacity fade and recovery.
A combination of the capital cost and the LCOS allows for a better comparison across the range of energy storage technologies with different performance attributes, which can be used to assess and develop economically viable RFB systems.
While the VRFB system benefits from reduced maintenance costs, it suffers from a high upfront cost, due, in part, to the price of the active species.
The magnitude and uncertainty of vanadium prices are considered key impediments to broad deployment, which have motivated research into alternative chemistries based on lowercost and widely-available materials (see separate feature on the price of vanadium as the electrolyte).