Your Future in Marine Hydrokinetics
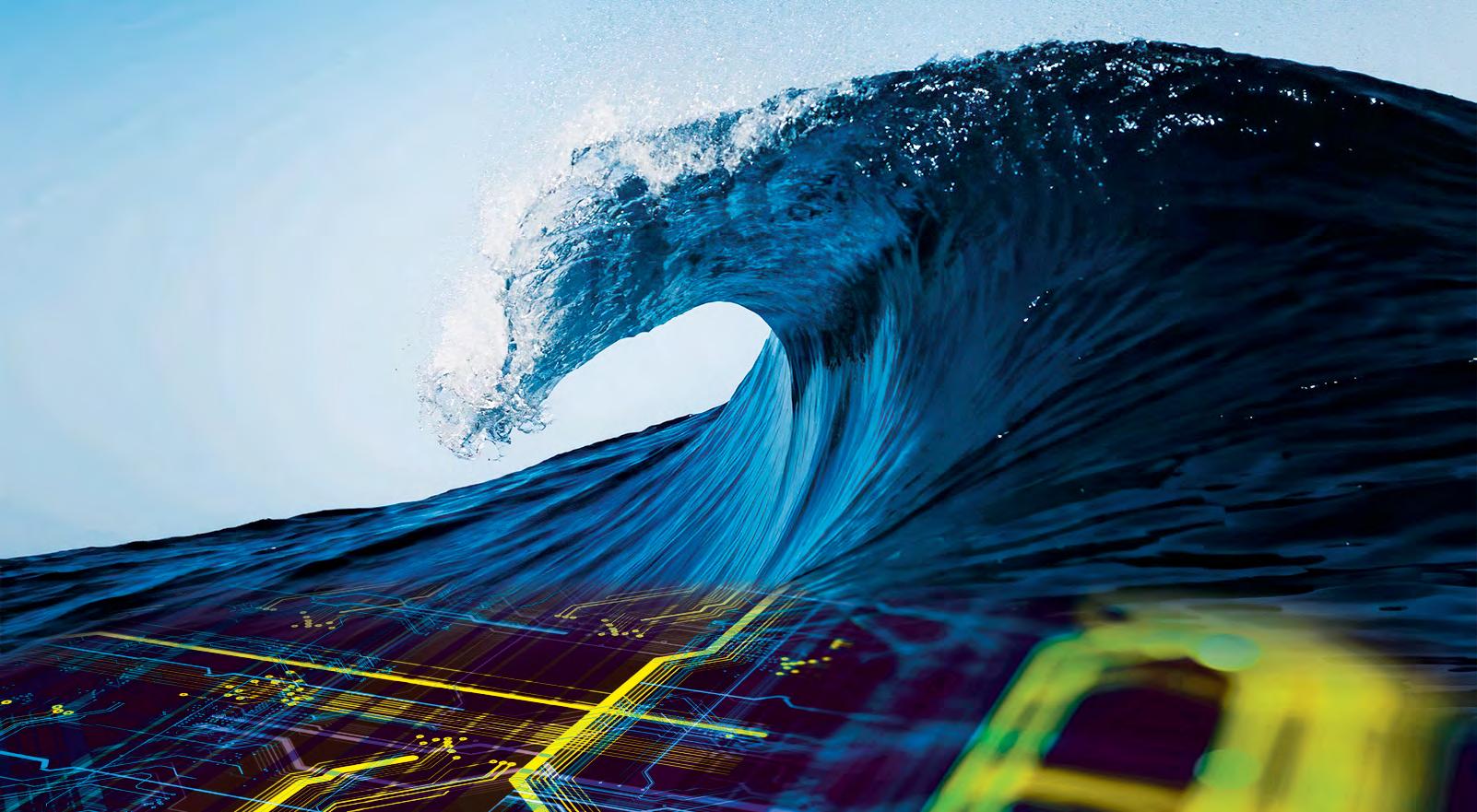
Your Future in Marine Hydrokinetics was originally developed by The NEED Project with funding from the National Renewable Energy Laboratory and the U.S. Department of Energy, Water Power Technology Office. NEED would also like to acknowledge Mr. Walter Schurtenberger of Hydrokinetic Energy Corporation and The College of the Florida Keys for his technical advisement and support in the creation of this unit. NEED is grateful to its Teacher Advisory Board team and staff members for designing this unit:
Nina Corley
Shannon Donovan
Robert Griegoliet
Christine Lauer
Paula Miller
Doug Keaton
Tom Spencer
We all use the word energy daily. We have energy drinks, we pay our energy bills, and politicians discuss energy policy almost daily. However, what energy actually is can be a difficult concept to explain precisely. Our bodies and objects all around us are using energy all the time. Energy allows us to do work, and to affect change. It provides the ability to do what we need to do, whether we can visibly see what is happening or on a microscopic level. Energy exists in two basic forms. Stored energy to use later is called potential energy, while energy in motion is called kinetic energy. Each of these two, broad categories can be broken down further into nine different forms of energy.
One type of potential energy is gravitational potential energy (GPE), which is energy stored by position. A child at the top of a slide or water behind a dam both have gravitational energy. Elastic energy is potential energy that is stored by applying a force. When you wind up a toy car, you are storing energy in the spring inside of it. If you pull back on a rubber band, you are storing elastic energy in it. Nuclear energy is the potential energy within the nucleus of atoms. Very small changes in the nucleus of an atom can release tremendous amounts of energy. The most commonly used form of potential energy is chemical energy. It is the energy in the bonds between atoms of all the substances in the world. The food you eat, the fuel in the car you drive, the wood your campfire burns are all examples of chemical energy.
Kinetic energy – energy in motion - can be broken down into five forms. When large-scale things are moving, they have motion or mechanical energy. A child on a bike, water moving through a stream, and the wind all have motion energy. Thermal energy is the energy that allows atoms and molecules to move around. The more thermal energy in a substance, the faster the molecules move, and the higher its temperature. Sound energy is a form of energy that we often overlook – because a sound is a vibration, it is an energy form. Radiant energy is energy that moves through space in transverse waves. Sunlight and radio waves are examples of radiant energy, as are microwaves and x-rays. Electrical energy is the energy of moving electrons. Electricity is the most common example of electrical energy, but small static electricity jolts and lightning strikes are also examples of electrical energy.
Conservation of energy is not just saving energy. The Law of Conservation of Energy says that energy is neither created nor destroyed. When we use energy, it doesn’t disappear. We simply change it from one form of energy into another. A car engine burns gasoline, converting the chemical energy in gasoline into motion energy. Solar cells change radiant energy into electrical energy. Energy changes form, but the total amount of energy in the universe stays the same.
Energy efficiency is the amount of useful energy you get from a system compared to the energy input. A perfect, energy-efficient machine would change all the energy put in it into useful work— an impossible dream. Converting one form of energy into another form always involves a loss of usable energy, often as waste heat. Most energy transformations are not very efficient. The human body is a good example. Your body is like a machine, and the fuel for your machine is food. Food gives you the energy to move, breathe, and think. Your body is very inefficient at converting food into useful work. Most energy in your body is released as wasted heat.
We use many energy sources to meet our needs. All of them have advantages and disadvantages—limitation or reliability of supply, and economic, environmental, or societal impacts. Energy sources are usually classified into two groups—renewable and nonrenewable.
In the United States, most of our energy comes from nonrenewable energy sources. Coal, petroleum, natural gas, propane, and uranium are nonrenewable energy sources. They are used to generate electricity, heat homes, move vehicles, and manufacture all kinds of products from candy bars to computers. They are called nonrenewable because their supplies are limited, and they cannot be replenished in a short period of time.
Petroleum, for example, was formed hundreds of millions of years ago, before dinosaurs lived, from the remains of ancient sea plants and animals. We could run out of economically recoverable nonrenewable resources someday.
Renewable energy sources include biomass, geothermal, hydropower, solar, and wind. They are called renewable because they are replenished in a short time, almost as quickly as they are used. Day after day the sun shines, the wind blows, and the rivers flow. We use renewable energy sources mainly to make electricity.
*Total does not equal 100% due to independent rounding.
Data: Energy Information Administration
Other: purchased steam, tire-derived fuels, electricity used for storage, etc.
NONRENEWABLE, 87.50%
Petroleum 34.73%
Uses: transportation, manufacturing - Includes Propane
Biomass 4.88%
Uses: electricity, heating, transportation
Natural Gas 33.99%
Uses: electricity, heating, manufacturing - Includes Propane
Wind 3.24%
Uses: electricity
Coal 9.89%
Uses: electricity, manufacturing
Uranium 8.89%
Uses: electricity
*Propane consumption gures are reported as part of petroleum and natural gas totals.
Propane
Uses: heating, manufacturing
Data: Energy Information Administration
Hydropower 2.79%
Uses: electricity
Solar 1.34%
Uses: electricity, heating
Geothermal 0.23%
Uses: electricity, heating
**Total does not equal 100% due to independent rounding.
In the United States, nine of the ten energy sources are regularly used to generate electricity; the only source that is not commonly used for electricity generation is propane. Natural gas is the leading source for electricity, followed by uranium and coal. Wind and hydropower are the leading renewable energy sources for electricity generation.
The most common way to generate electricity is by using thermal energy to boil water into pressurized steam that can turn a turbine Turbines change linear motion into rotational motion; in this case, the linear movement of the steam is changed to a rotation to turn a generator. The generator houses large magnets and coils of copper wire. The turbine blades spin the generator, moving either the coils of wire or the magnets relative to the other.
This produces an electric current. Wind and hydropower use a flowing fluid, air or water respectively, to turn the turbine. Because no thermal energy is lost, wind and water are more efficient sources of energy for generating electricity.
When people hear the word “energy” they often think about electricity. Electricity is not a source of energy – rather it is the energy carrier that we use as we convert energy from one energy source to electrical energy and then to a third form of energy for our intended use. For example, natural gas is burned to generate steam and turn a generator for electricity. That electricity is used by our cell phones and transformed into light and sound energy. Electricity is commonly called a secondary source of energy
Electric energy is carried by electrons, which are tiny particles moving around the nuclei of atoms. Electrons are more likely to stay associated with their respective nuclei unless they’re given additional energy and have the potential to move. That potential is called voltage. Electricity flows when a source of voltage such as a battery causes an electric current to flow through a conductor to an electrical load.
Electrons will move through materials called conductors. These materials are familiar to you; copper and aluminum are excellent conductors of electricity. A common example of a conductor is insulated wire, and the wire may carry the electric current to a light bulb, which is the load. Materials that do not easily allow electrons to move through them are called insulators. The common wiring running through our homes and other buildings consists of a conducting wire surrounded by an insulating coating. The thickness of the insulation on the wire is important. Applications with high voltage require heavy gauge wire with thick insulation because high voltage indicates that the electrons are very highly energized and could, under the right circumstances, move through a thin insulator. Air is an insulator, yet lightning will travel through it given a high enough potential to do so.
Voltage is the force or pressure pushing electrons in a circuit, or the likelihood an energized electron will move across an insulating gap. This is also called electrical potential. Think of voltage as the potential to make a jump. The higher the voltage, the more energy the electron has, and the more likely it is to jump the gap. Voltage is measured in Volts (V)
The number of electrons passing a point in one second is called current. Those electrons can be highly energized (high voltage) or not very energized at all (low voltage) but as long as they are moving, they are counted in the current. Current is measured in Amperes (A). Electricity allows us to move energy around between systems for many different purposes.
Voltage and current are related through two properties called resistance and power. Resistance is exactly what it sounds like – a material with a high resistance is not going to allow electricity to flow through it. Given a high enough voltage, current will flow, but the current will be low because the resistance is high. Conductors have lower resistances compared to insulators, but heavy-gauge wire has a higher resistance than fine-gauge wire. Devices called resistors can also be placed in circuits to reduce or control the flow of current. Resistance is measured in ohms.
Power tells us how much work is done in a period of time. The numerical values of voltage and current are multiplied to tell us
the power used (Power = voltage × current). Power is measured in watts. The amount of power itself does not tell us much about what is happening with electric power consumption. For example, 1,000 watts could be 100 volts and 10 Amperes of current, or it could be 1,000 volts and only 1 Ampere of current. However, power is the way an electric utility measures and bills us for the electricity we use.
Common applications of resistance and power exist in everyday life all around us. If you have an electric range or cook top, the large heating element inside is one giant resistor. The higher voltage needed to force electricity through the element is provided by the heavy-duty, higher voltage outlet. If you have a hair dryer or
electric heater, those also have heating elements that work in a similar way, albeit at lower voltage. Microwaves and light bulbs are often described by their power use; however, the most efficient lighting with light emitting diodes (LEDs) inside are usually described by their light output because they use a small amount of power.
Electrical devices use two different types of electric power: DC and AC. Direct current, or DC, means the flow of electrons is in one direction only. Simple electrical devices, such as flashlights, LEDs, and cell phones, run on DC power. Alternating current, or AC, means the polarity of the circuit changes. In the United States, AC power is a 60 Hz system, meaning the direction of current flow changes 60 times in one second.
When you charge a DC device using the AC power in your home, a transformer inside the charging cord changes the AC power to DC, and regulates the voltage being delivered to the battery being charged. Changing the AC electrical energy to DC electrical energy results in some of the energy lost as thermal energy. You can observe this directly by feeling the large plug, or the box in the middle of the cord. When the device is actively charging, the transformer gets warm.
Electric current needs a pathway to flow; this is called a circuit Circuits can be closed, forming a continuous pathway, or open, which has a break in the path. Current will not flow in an open circuit. Switches are used to open or close circuits to turn things off and on.
When connecting loads in a circuit, there are two arrangements. Series circuits have all the components wired so that only one pathway is available for electrons to travel. Parallel circuits allow for more than one pathway for electric current to flow. Simple electrical devices are wired in series. A flashlight, for example, has the power source, switch, and light bulb all wired in line with each other. There is only one path for electricity. When series circuits are not operating correctly, they are relatively easy to troubleshoot; just locate the place in the circuit where the connection has been lost. Parallel circuits are useful when one power source is used to operate several loads. By using parallel wiring, one device can be switched off while another is switched on.
Most complex wiring systems, such as electronics and household wiring, use a combination of series and parallel circuits. Your home has a main power source, where the electric power comes in from the utility. From there, multiple circuits are connected that each radiate to different rooms or appliances in your home. Each of the components on one circuit breaker is wired in series, but all of the circuit breakers are wired parallel to each other from the main power source. Holiday lights with LEDs are also wired in a combined series and parallel configuration. Several sections are wired in series, and each of the sections are wired parallel to each other where they connect to the plug and the outlet.
A closed circuit is a complete path allowing electricity to ow from the energy source to the load.
An open circuit has a break in the path. There is no ow of electricity because the electrons cannot complete the circuit.
One of the many ways that we can produce or manipulate electrical energy is through electromagnetic induction. As a current flows through a wire, a corresponding magnetic field forms around the wire’s cross section, all along the conductor. The strength of the magnetic field is directly related to the amount of current flowing through the wire. It will also change in direct proportion to the current flow. As a result, greater current induces a greater magnetic field, and if the current changes in magnitude or direction, the magnetic field around the wire will also change in magnitude or direction. We represent magnetism as lines of magnetic flux or lines of magnetic force around a conductor that is carrying current.
There are two general types of magnets: Permanent magnets are made from ferrous materials, which contain iron, that have their magnetic poles largely aligned. An object with enough iron can be made into a magnet by running a large electrical current through it. However, the strength of the magnet can be reduced or eliminated by physically striking the material (as in with a hammer). So, if you like your powerful permanent magnet, don’t drop it!
Electromagnets are temporary magnets. An electromagnet is made by using a ferrous material as a core and winding an electrical conductor (such as an insulated wire) around it several times. The coil of wire is not electrically connected to the core. When a current runs through the coil, magnetic flux forms around the coil. This field is intensified by having the ferrous core, which is more capable of accommodating a magnetic field than air. The core is more magnetically permeable than air.
Magnets have north (N) and south (S) opposing magnetic poles very much like the Earth does. When you hold two permanent magnets near each other, you can feel the effect the magnetic fields have on them.
The north poles of the two magnets will repel each other as will both south poles, and the north pole of one magnet will attract the south pole of the other magnet.
The lines of magnetic flux within a magnetic field are easy to see when visualized by iron filings in a clear container with mineral oil. The filings will move within the oil and align themselves with the magnetic field. You can also do this if you have small steel bearings
Like poles of magnets (N-N or S-S) repel each other.
Opposite poles of magnets (N-S) attract each other.
or loose iron filings. Place the magnet beneath a sheet of paper and sprinkle the bearings or filings on the paper. The tiny pieces of metal will align with the magnet’s magnetic field.
Michael Faraday did much of the initial work on electromagnetic induction. A law was named after him as a result of his work. Faraday’s Law states, “the electromotive force around a closed path is equal to the negative of the time rate of change by the magnetic flux enclosed by the path.”
Electromotive force or EMF is another way of saying voltage. The “closed path” referred to above simply means circuit. Michael Faraday is famous for much more than the law that bears his name. He invented inductors, motors, transformers, generators, and linear actuators called solenoids that we still use today. The unit of capacitance, the Farad, is named in his honor.
Generators are devices that take rotating motion and convert it into electrical energy. Generators are made using coils of wire and magnets. Sometimes those magnets are permanent magnets, but other times they are electromagnets using coils of wire wrapped around ferrous cores. Alternators (AC) and Generators (DC) transform rotating motion into electrical energy for us to utilize. There are three ways to increase the power generated by an alternator turned by a tidal or ocean current powered turbine: increase the speed of the water, turning it faster; increase the magnetic field that cuts through the coils of wire by using bigger magnets or electromagnets; or increase the number of turns of wire in the coils that rotate within the magnetic lines of flux, making bigger coils. Each of these options will provide additional power generated by the alternator.
Generating electricity is great, but few of us live next-door to a power plant. Electric power generated in a power plant needs to get to the buildings where we use it. First, the electricity is generated at the power plant. Next, it goes by wire to a transformer that “steps up” the voltage. Power companies step up the voltage in order to combat energy loss.
The electricity is then sent on a nationwide network of transmission lines made of aluminum. Transmission lines are the huge towers and power lines you may see when you are on a highway. The lines are interconnected, so should one line fail, another will take over the load. This network is commonly referred to as the electric grid. Step-down transformers located at substations along the lines reduce the voltage. Substations are small buildings in fenced-in areas that contain the switches, transformers, and other electrical
equipment. Electricity is then carried over local distribution lines that bring electricity to your home. Distribution lines may either be overhead or underground. The overhead distribution lines are the electric lines that you may see along streets.
Before electricity enters your house, the voltage is reduced again at another transformer. This neighborhood transformer reduces the electricity to 240 and 120 volts, the amount needed to run the appliances in your home. Electricity enters your house through a three-wire cable. The “live wires” are then brought from the circuit breaker or fuse box to power outlets and wall switches in your home. An electric meter measures how much electricity you use so the utility company can bill you. The time it takes for electricity to travel through these steps—from power plant to the light bulb in your home—is a tiny fraction of one second.
When you think of the United States, you may picture a map outlining the 50 states and their coastlines. However, the United States actually includes a huge area you can’t see. This area lies beneath the oceans off our coasts. We refer to the land and water off our coasts as being “offshore.”
Economically, the land and water surrounding our coastlines are very important to our nation. Most of the seafood we eat comes from the waters offshore. The submerged lands hold fossil fuels, such as petroleum and natural gas. More recently, renewable sources of energy are generating electricity offshore. All of these resources are important to the United States when considering the world’s increasing demand for energy. Marine renewables have the capacity to greatly increase U.S. renewable generation in the coming years with the continued research and deployment of hydrokinetic technologies.
Source: NREL
The movement of water in the world’s oceans creates a vast store of kinetic energy. Marine Renewable Energy (MRE), also known as Marine Hydrokinetics (MHK), can be harnessed to generate electricity to power homes, transport and industries. MRE encompasses wave energy — power from the movement of surface waves, tidal energy – power from the changes in tides, ocean current energy — power from the motion of ocean currents, ocean thermal energy conversion (OTEC) — power from the heat differential of different thermal layers within a body of water, and salinity and pressure gradient — power from the difference in salt concentration between two fluids.
At the edge of the ocean, where waves lap at the shoreline, is the continental shelf. It is a continuation of the North American continent we live on, and this landmass extends from the shore into the ocean as a gently sloping undersea plain. This undersea world is a fascinating place.
The continental shelf can be as narrow as 20 kilometers (12 miles) along the west coast and as wide as 400 kilometers (249 miles) along the northeast coast of the United States. The water on the continental shelf is shallow, rarely exceeding a depth of 150 to 200 meters (490-650 feet). Often, the continental shelf is a mostly sandy bottom, with great opportunity for offshore energy development. The continental shelf drops off dramatically at the continental slope and continental rise, where the deep ocean truly begins. The deep ocean consists of abyssal plains that are 3 to 5 kilometers (1.8-3.1 miles) below sea level. Many of these plains are flat and featureless, while others are marked with jagged mountain ridges, deep canyons, and valleys. These areas also offer great opportunity for development.
Bathymetry studies must be completed and/or analyzed for any offshore installation to help understand the surface conditions and the geology at and below the seafloor. These studies have often required ships to tow sensing and Sonar equipment called a towfish to collect data. Advances in Autonomous Underwater Vehicles (AUVs) are allowing us to collect this information at a much greater rate. Soon we will have much better maps available to help make these kinds of decisions
In 1982, the United Nations adopted a law giving each country bordering an ocean the rights to an exclusive economic zone off its coast. The EEZ is an area of coastal water and seabed no more than 200 nautical miles (370 km) beyond the country’s shoreline. Each country claims exclusive rights for fishing, drilling, and other economic activities. This includes production of energy from the water, winds, and currents. On March 10, 1983, President Ronald Reagan signed a Presidential Proclamation that set up the U.S. Exclusive Economic Zone (EEZ).
The first three nautical miles offshore belong to the state that it borders. According to the Outer Continental Shelf Lands Act, the Federal Government controls the area beyond that, known as the Outer Continental Shelf, or OCS.
The Outer Continental Shelf consists of 1.7 billion acres of submerged lands, subsoil, and seabed in a specified zone up to 200 nautical miles from the U.S. coastline to the edge of the EEZ, or even farther if the continental shelf extends beyond 200 nautical miles. The OCS is divided into four regions: the Atlantic Region, the Gulf of Mexico Region, the Pacific Region, and the Alaska Region.
A nautical mile is based on the circumference of the Earth. Visualize this: cut the Earth in half at the Equator, pick up one of the halves, and turn it on its side. The Equator is the edge of the circle.
A circle is divided into 360 degrees. Each degree divides into 60 minutes. One nautical mile is one minute of arc on the planet Earth. Every country in the world uses this unit of measurement for travel in the air and on the oceans.
We are used to measuring distances in meters and feet. A nautical mile is equal to 1,852 meters, or 1.852 kilometers. In the English measurement system, a nautical mile is equal to 1.1508 miles, or 6,076 feet.
At the Equator, the Earth measures 40,075.16 km (or 24,901.55 miles). How many nautical miles is it around the Equator?
There is tremendous energy in ocean waves. But harnessing wave energy, or extracting the power from a wave, is a big challenge. Wave energy devices extract energy directly from the surface motion of ocean waves and convert the energy into electricity. A variety of technologies and promising designs are undergoing demonstration testing around the world.
Waves are caused by the wind blowing over the surface of the ocean, although they can also be affected by tides, weather conditions, and underwater events. The size of waves depends on the speed of the wind, the duration the wind blows, and the distance of water over which the wind blows, known as the fetch. Usually, the greater the fetch, the higher the waves. A strong breeze of 48 kilometers per hour (30 mph) can produce waves three meters (10 feet) high. Violent storm winds of 105 kilometers per hour (65 mph) can produce waves nine meters (30 feet) high.
As the wind blows over the water, there is friction between the wind and the surface of the water. The wind pulls the surface water in the direction it is going. The water is much denser than the air and cannot move as fast, so it rises and is then pulled back down by the force of gravity. The descending water’s momentum is carried below the surface, and water pressure from below pushes this swell back up again. This tug of war between gravity and water pressure creates wave motion.
Ocean waves are, therefore, the up and down motion of surface water. The highest point of a wave is the crest; the lowest point is the trough. The height of a wave is the distance from the trough to the crest. The wavelength is the distance between two crests. Small waves may have lengths of a few inches while the crests of large storm waves may be several football fields apart. Waves usually follow one another, forming a train. The time it takes two crests in a train to pass a stationary point is known as the period of a wave. Wave periods tell us how fast the waves are moving.
The U.S. has over 95,000 miles of coastline for harnessing wave energy, however, not every mile is created equal. The best wave resources in the U.S. for producing power are found in and around deeper waters of the Pacific Ocean. Wave energy potential is often described by power per meter of wave crest. The Pacific Coast of the U.S. has many areas of the coast that have potential for 35 kW/m averaged throughout the year. For contrast, the Gulf of Mexico and the Atlantic Coast resources are more consistently near 10-20 kW/m. Wave energy technology is being tested in several sites, both in the Atlantic and Pacific, and will help maximize the resources to bring power to the people, wherever they may be.
Wave Formation Circular orbit in open water
Friction distorts the circular orbit
Top of wave moves faster. Increasingly elliptical orbit Wave begins to break
Image courtesy of IKM Testing UK
Wave and other oceanographic data can be gathered from wave-powered buoys like the one depicted above. The buoy is equipped with instruments and sensors that record wind speed, wave height, water temperature, air temperature, and other conditions. The sensors send data back to shore, however, the sensors require power to send such signals. This buoy is moored or anchored to the ocean floor with cables. The cables are wound around gears or winches that are connected to a generator. As the cables move up and down with wave action, the kinetic energy is transformed into electricity through the winding and unwinding of the cable. Wires connected to the generator send electricity to a small battery for storage, and into the computing and sensing devices on the buoy to power lights and send signals.
Research and development of wave energy is really just beginning. Worldwide, over a hundred conceptual designs of wave energy conversion devices have been developed, but only a few are built as full-scale prototypes or tested under real world conditions. So far, no particular technology is considered an ultimate solution.
Wave energy devices turn motion or mechanical energy into electric current. How motion energy is obtained from ocean waves is what makes each device unique. Wave energy technology can be grouped into a few major categories, each with a different method for harnessing energy from waves: attenuators, point absorbers, overtopping devices, pressure differential, oscillating water columns, and osculating wave surge converters.
There is one thing each kind of device has in common - in order for it to be economically successful, it must overcome some major technical challenges. It has to survive the harsh ocean environment, and it must efficiently extract energy from waves. Other major technological challenges include generating electricity in a water environment, and getting that power through the ocean to the existing electric grid. There are additional challenges with the mechanical systems, mooring and anchoring the device, reliability, and predictability or wave forecasting.
In addition to technological challenges, developers must consider how a device may impact the surrounding marine environment. Some environmental concerns include migratory species, and changing the flow of sediments near the shore. In some places, citizens and business owners have concerns about losing commercial and recreational fishing grounds and the impacts these devices have on recreational activities like surfing and kayaking.
An attenuator is generally a multi-segmented device that floats on the surface of the ocean. It is anchored in place parallel with the incoming waves. The device harnesses energy as the motion of the waves causes flexing between each segment. The mechanical motion of the flexing is converted to electrical energy using hydraulic pumps and generators.
An example of this technology is in use by the Scottish company, Mocean. They invented wave attenuators known the Blue Star, the larger Blue Horizon and BlueX. The device is a raft with hinged joints. Passing waves cause each joint to rise and fall. The motion of the hinges drive the generators to produce electricity. The wave energy converters are anchored to the sea floor by moorings and then connected to the grid with subsea power cables.
A point absorber often looks much like an ocean buoy. It is a floating structure that captures energy from the vertical motion of waves. A point absorber is not oriented any particular way in relation to the waves because it “absorbs” the energy from waves from every direction. The up and down bobbing motion drives a linear (rotary) generator or hydraulic energy converter to generate an electric current. The moving body of the absorber can be submerged or on the surface, and may be fixed to the sea bed or another structure. Several companies are successfully demonstrating this type of technology around the world. Ocean Power Technologies has deployed the PowerBuoy® off the coast of New Jersey for a U.S. Navy project. Other point absorber designs of many different shapes and approaches are being deployed, tested, and improved. Sites like the Wave Energy Test Site (WETS) in Hawaii, Scripps Oceanography Institution, and PacWave testing site in Oregon help companies to prove and improve their designs. CalWave has been testing and improving a design called the xWave which is a fully submerged, square-shaped, point absorber design that is protected from storms and swells by staying below the surface. Another example is the Fred.Olsen Lifesaver device, a device shaped like a round life saver that uses winches and cables to generate electricity as it bobs up and down.
One way to harness wave energy is to focus the waves into a narrow channel, which can concentrate their power or size. Overtopping devices direct or channel the waves and allow them to spill over into a reservoir. The higher water in the reservoir creates a pressure difference between the water at the surface and in the reservoir, forcing fluid to travel through a turbine generator, much like a hydroelectric dam. Overtopping devices have been demonstrated both onshore and offshore. The Wave Dragon is one such example of a floating offshore overtopping device. It focuses or reflects the waves towards a ramp. Behind the ramp is a large reservoir where water that runs up the ramp can be stored and used to generate electricity. The Wave Dragon was first developed for use off the coast of Denmark in the early 2000s, and another is in use off the coast of Wales in the U.K.
Pressure differential devices are constructed below a wave and are built to capitalize on the pressure difference that occurs between crests and troughs of waves. If you’ve ever sat down quickly on an air mattress and rolled or bounced someone off the other side, you might be able to imagine how this device works. On one side of the system, the crest of the wave has a higher pressure, and it causes a device in the chamber to compress downward. On the other side, the trough has a lower pressure causing a device on this end of the chamber to expand upwards. There is air between the two expanding and contracting chambers, and power can be generated by the air as it expands and contracts from side-to-side. These devices are often located close to shore and are attached to the seabed.
Oscillating water column devices are typically partially submerged, and can be free floating or fixed onshore. The device is constructed so that they contain a shallow chamber of air on top of a column of water. Wave action causes the water column to rise and fall, causing the air in the chamber above to be compressed and decompressed with the movement. Trapped, or forced air can then spin a turbine generator to generate electricity.
Oscillating wave surge converter technology harnesses energy directly from the surging and swelling motion of waves. It uses the swaying motion between a float, flap, or membrane that is free to move and a fixed point that is attached to a structure or the seafloor. Energy can be collected from the motion of the waves relative to the body of the device. Depending on the device design, there could be a generator at the hinge/fixed point that generates the electricity. Alternatively, the moving body could be used to create pressure in a fluid that will drive a turbine generator.
In many areas of the world, the wind blows with enough consistency and force to provide continuous waves. The total power of waves breaking on the world’s coastlines is estimated at 2-3 million megawatts. Along with the west coast of the United States, the west coast of Europe and the coasts of Japan and New Zealand are good sites for harnessing wave energy. There aren’t yet any major commercial wave energy plants, but there are a few small ones deployed across the globe. Onshore and offshore devices will likely need to be deployed in arrays of multiple devices - much like a wind farm – in order to make the best use of wave potential for electricity generation.
Tides are caused by the interaction of gravitational forces between the Earth, moon, sun, and the Earth’s oceans. The force from the moon is much more powerful since it is closer to the Earth. The moon pulls on the ocean water that is closest to it. This creates a bulge in the surface of the water, called a tidal bulge. The water on the opposite side of the Earth also forms a tidal bulge, because the gravitational pull is smaller due to the greater distance from the moon on the far side of the Earth. These bulges produce high tides. The influence of the sun is apparent when it is aligned with the moon and the tides become higher than at other times.
Between the tidal bulges are lower levels of water that produce low tides. The tidal bulges move slowly around the Earth as the moon does. Halfway between each high tide is low tide. Most shorelines have two high and two low tides each day. However, the Gulf of Mexico only experiences one high and one low tide each day because of its geographic location.
On most of the U.S. coast, the tidal range, or the difference between high and low tides, is only about one to two meters (3-6 feet). When a high tide flows into a narrow bay where the water cannot spread out, the tidal range can be very large. These areas are potential locations for harnessing tidal power. The Bay of Fundy in Canada has the highest tides in the world, sometimes rising over 15 meters (50 feet).
A tidal power plant, or tidal barrage, is built across an estuary, the area where a river runs into the ocean. The water here rises and falls with the tides. A tidal barrage has gates and turbines at its base. As the tide rises, the water flows through the barrage, spinning the turbines, then collects in the estuary. When the tide drops, the water in the estuary flows back to the ocean. The water again turns the turbines, which are built to generate electricity when the water is flowing into or out of the estuary.
Building large-scale tidal barrages is less likely than alternative methods of harnessing tidal power. One option is a tidal fence. Tidal fences are similar to tidal barrages in that they stretch across narrow areas of moving water. Unlike tidal barrages, tidal fences do not dam water, but allow it to flow freely. This makes them cheaper to install as well as having less impact on the environment. However, tidal fences may inhibit the movement of large marine mammals and ships.
Tidal power is very reliable and predictable, which can make it a valuable resource. One main challenge to harnessing tidal power is geography. The highest tidal ranges are generated when the sun, moon, and Earth are in line. Water will flow in greater volume when this combined pull occurs. These are called spring tides. Lower tidal ranges, or neap tides, occur when the sun, moon, and Earth are less aligned, causing less volume of water. Tidal stream resources are largest where there are good tidal ranges and where there is good current speed to move the water. Geographical features like straits, inlets, channels, and islands can create higher velocities of currents and amplify the rise and fall of tides. This movement can be captured by turbines. Like wave power, tidal power is still in development in many areas, but the technologies are more advanced. A few commercial and pre-commercial scale systems have been installed in the U.K. and U.S. There are a few types of tidal technology designs including tidal barrages, tidal fences, and tidal turbines.
Tidal water is captured at high tide behind a dam. When the tide turns, the water is released to the sea, passing through a set of turbines.
Underwater turbines work like submerged windmills, but are driven by flowing water rather than air. They can be installed in the ocean in places with high tidal current velocities or in places with fast, continuous ocean currents. There are a few general designs for tidal turbines, but the design and deployment can vary greatly based on the area selected. Tidal turbines are similar to wind turbines, using spinning blades that face the direction of the flow of water. These turbines can be ducted (enclosed), or open, and can be fixed on the ocean floor or floating at any point in the water column. The SeaGen and Open Hydro turbines are two examples of tested axial flow designs. Cross flow turbines capture the kinetic energy of moving water with spinning blades that are oriented perpendicular to the direction of water flow. Cross flow turbines are mounted vertically or horizontally, and can be open or ducted in their construction. Like axial turbines, these can also be placed anywhere in the water column, but bottom mounting is most common. The TideGen Turbine Generator Unit is an example of cross flow design in action. Tidal kite devices are designed very much like they sound. A buoyant wing or kite with a turbine attached to it is tethered to a cable. The cable is fixed at a location that enables it to “fly” in the tidal stream, often swooping in a figure-eight like motion. Archimedes screws are also constructed very similar to how they might sound. These corkscrew-shaped devices allow water from the tides to move up the spiral shaped-turbine, rotating the device to generate energy. Finally, oscillating hydrofoils utilize a wing attached to an arm that can oscillate with the flow of water. As the water flows, it lifts and lowers the wing, and a hydraulic system can be used to generate the electricity.
The city and state of New York have partnered with Verdant Power to harness the energy in the ebb and flow of the tides in Manhattan’s East River. The Roosevelt Island Tidal Energy (RITE) Project is the first gridconnected (non-commercial) array of tidal turbines in the world. After completing a successful demonstration phase with six turbines that generated 70 megawatt-hours of electricity, the project received the first U.S. commercial license for tidal power. The project installed three 35-kilowatt turbines in 2020 with hopes to expand with more funding.
Ocean waters are constantly moving and flowing in complex patterns around the Earth called currents. Waters in the upper 400 meters (1,300 feet) are called ocean surface currents. They are formed by wind, water salinity, temperature, topography of the ocean floor, and the Earth’s rotation. Moving water below 400 meters is considered deep water current and its movement is mostly driven by density differences and gravity. Ocean currents are relatively constant and flow in one direction.
Ocean currents contain an enormous amount of energy because water is very dense. Ocean water is about 800 times more dense than air. This means slow flowing water exerts more force than a very strong wind over comparable surface areas. Capturing the energy potential remains a challenging technology problem.
Some of the ocean surface currents on America’s OCS are the Gulf Stream, Florida Straits Current, and California Current. The Gulf Stream is a powerful current in the North Atlantic Ocean. It originates in the Gulf of Mexico, exits through the Strait of Florida, and follows the eastern coastline of the United States and Newfoundland. It travels 40 to 120 kilometers (25 - 75 miles) a day at speeds ranging from 1.75 km/hour to 5 km/hour (or about one to three knots). The Gulf Stream strongly influences the climate of the East Coast of the United States and many Western European countries. It keeps temperatures warmer in the winter and cooler in the summer compared to surrounding areas.
Most ocean currents are driven by wind and solar heating of surface waters near the Equator, while some currents result from differences in density and salinity between surface and deeper waters. As global winds drag on the ocean’s surface, they pull the water in the direction that the wind is blowing. Because of the Coriolis Effect, major surface ocean currents curve to the right in the Northern Hemisphere (in a clockwise spiral) and to the left in the Southern Hemisphere (in a counter-clockwise spiral). These major spirals of ocean-circling currents are called gyres and occur north and south of the Equator.
It is not just surface water that is affected by global winds and the Coriolis Effect — deep water currents are formed this way, too. As surface water molecules are pushed by the force of the wind, they, in turn, drag deeper layers of water below them. Each deeper layer moves more slowly than the layer above it, until about 100 meters deep. Below 100 meters, ocean currents are formed by differences in the density and salinity of the water. These differences are used by Ocean Thermal Energy Conversion systems to generate electricity (see page 19 for more details). As each successively deeper layer of water moves more slowly to the right or left, it creates a spiral effect known as the Ekman Spiral. Because the deeper layers move more slowly than the shallower layers, they tend to twist around and actually end up flowing in the opposite direction of the surface current. In this way, ocean water travels a continuous path all around the Earth.
Ocean currents are one of the largest untapped renewable energy resources on Earth. However, they are also the least understood ocean energy resource. Engineers are researching and developing technology designs to harness ocean current energy, but it’s still at an early stage of development.
To harness energy from ocean currents economically, and on a commercial scale, there are some major technical challenges to overcome. For example, the logistics of maintenance are likely to be complex and the costs potentially high, so system reliability will be extremely important. The system components will need to be built from materials resistant to corrosion in salt water. Another challenge involves keeping naturally occurring marine growth from building up on the system components.
At this time, there are no commercial, grid-connected systems operating in the open ocean. Only a few small-scale prototypes and demonstration units have been built and tested. Tidal turbines and ocean current technologies are similar, and many of the turbines used for tides could be used for harnessing energy form currents.
A project in Japan was able to showcase the viability of ocean current power with a very large, deep water turbine called Kairyu. Japan is one location where you can find some of the fastest ocean currents in the world. Two Japanese companies, NEDO and IHI, constructed a turbine to capitalize on the swift, steady deep water currents. The turbine looks almost like an airplane and consists of
three cylindrical floats or pods, each about 20 meters in length. Two turbines are mounted on the outer two pods, with each rotating in an opposite direction. This opposite rotary motion cancels out any torque and keeps the system stable in the steady currents. The system is moored in about 100 meters of water, and installed so it maintains its locations about 30-50 meters below the surface. The model was successful in its tests for launch and generation from 2018-2021. Developers will continue to research and explore scaling the system for additional deployment and launches.
Oceans cover about 70 percent of the Earth’s surface. Radiant energy from the sun is absorbed by the ocean’s surface and some of it is transformed into heat. There is a large difference or layering affect between the warm surface waters and deeper waters in tropical and subtropical oceans. Deep ocean water does not receive any sunlight, and stays at a cool, constant temperature year round. This difference in temperature between the ocean layers can be used to generate electricity in an Ocean Thermal Energy Conversion (OTEC) plant. An Ocean Thermal Energy Conversion power plant can be designed as either open-cycle, closed-cycle or as a hybrid system. Each system uses a heat engine or heat exchanger to capture energy as heat flows naturally from hot to cold.
In an open-cycle system, warm surface water enters a low pressure vacuum chamber, where it boils and turns into steam. The steam spins a turbine connected to an electrical generator. Salt and other contaminants are left behind in this process. In a heat exchanger, the steam is exposed to cold ocean water and condenses back to a liquid. It is now desalinated fresh water that may be used for drinking or agriculture.
In a closed-cycle system, warm surface water passes through a heat exchanger to boil a fluid with a low boiling point. The steam created spins a turbine connected to a generator producing electricity. Cold ocean water condenses the steam back into the original fluid and
flows back through the system to repeat the cycle. The working fluid and ocean water never mix. Used ocean water is piped back into the ocean fairly near shore.
A hybrid OTEC system produces electricity with a closed-cycle system and fresh water with an open-cycle system all at the same time. Warm ocean water enters a low pressure vacuum chamber, where it boils and turns into steam. The heat of the steam boils ammonia in a separate container. As the ammonia boils and turns into steam, it spins a turbine to generate electricity. Boiling the ocean water removes its salt and other impurities. When this steam condenses in the heat exchanger, it emerges as fresh, pure water for drinking or agriculture.
In November 2009, the large Norwegian energy company, Statkraft, opened the world’s first osmotic power plant. The prototype power plant is based on the natural process of osmosis or changes in salinity. In the osmotic power plant, seawater and freshwater are separated by a special membrane in a large tank. Freshwater can move through the membrane but saltwater cannot. The salt content of the seawater draws freshwater through the membrane to dilute the salinity, increasing pressure on the seawater side of the membrane. The increased pressure is used to spin a turbine to generate electricity.
Hydrokinetic devices must be able to thrive in what can often be a harsh environment. Salt, water, and motion put together can easily wear down many different materials. When constructing a device or technology for testing and use in the marine renewable energy world, materials matter.
When it comes to selecting materials for a project, application is key. It is important to consider exactly how the materials will be used, and which properties are most important. When considering the materials for a device, several factors come into play, including availability, cost, strength, and transportability of the materials. Materials that are lightweight and strong, but extremely expensive, may not be practical in the quantities needed to construct a hydrokinetic equipment. MHK devices need to be rigid enough to withstand the motion of the water, cables, ropes, and anything else that might rub against them. However, depending on the technology and placement, the materials may also need to be somewhat flexible. The constant motion also requires that the materials be durable enough to limit fatigue over time. Corrosion must also be considered, and seals or coatings may also need to be added to the design to protect sensors, components, or joints. Materials used in MHK can vary widely depending on the technology and application and include everything from concrete, composites, and fiberglass, to resins, ceramics, polymers, and adhesives.
When designing prototypes and testing hydrokinetic technologies, engineers in marine renewable energy will often consider the process of additive manufacturing. Additive manufacturing uses computer-aided-design (CAD) programs or 3D printing and scanning to create very precise shapes. Additive manufacturing allows for the engineer and design teams to create designs quickly, often called rapid prototyping, without great expense and time involved with fabricating, machining, and conventional manufacturing. Additive manufacturing often uses polymers and composite materials that allow engineers and designers to construct designs and test them in very short time frames, (months or less), and repeat their designs easily for continued refining and testing. Utility-scale or large-scale devices are more often made with steel. Perhaps future developments will showcase a role for additive manufacturing in large-scale devices.
The process of choosing a location for MHK equipment, known as siting, requires consideration of many factors. As with any human endeavor, there are trade-offs, which means that compromises are necessary. With any common resource, we seek to find compromises that are the “highest and best use” of our resources. In the world of real estate, appraisers use four criteria to determine the highest and best use of an area: legal permissibility, physical possibility, financial feasibility, and maximum productivity. When it comes to offshore
energy, the questions related to highest and best use can be very complicated because of so many services that this area provides. In addition to wave power density, current flow velocity, tide tables, and water depth, developers must also consider seafloor geology, wildlife in the area, ship or recreational traffic, and local electricity infrastructure when selecting a site to place their equipment. Often, the location for deployment will dictate the type of equipment technology. For example, an area that experiences very little variation in tidal flow volume between high and low tide would not be a great candidate for tidal equipment. Tide charts, flow volume charts, and topography maps must be consulted. When selecting an area for current technology, flow volume and current speed and direction data should be gathered. Sometimes, this requires consulting data already compiled by local buoys already in place for use by the Navy or NOAA, however data may need to be gathered locally and may require deploying a data collection buoy.
Environmental and social considerations are also important once a site and technology are being investigated. Design and development teams will need to look at who uses the water in and around the area they might like to deploy. They must gather information on fishery, recreational, and even naval traffic in the area. Teams must also take a survey of sea life in the area, looking for protected species or migratory animals that live in the waters where the equipment and infrastructure will be placed. They will also need to make sure the area in question is able to be connected to those who will use the power. There will need to be infrastructure (electric substations or power lines) to connect to, or else it must be built. And it must be feasible to deploy the equipment and the necessary cables given the geography and geology of the local seafloor. Finally, teams must also even consider the possibility of extreme weather. Coastal areas can be subjected to stronger storms that bring intense wind, wave, and current conditions, and equipment must be able to withstand these conditions or be removed and redeployed easily.
Deciding where to bring power cables on shore is another siting consideration. It wouldn’t make much sense to have an electricitygenerating installation located in an area where there was no onshore infrastructure with which to connect. Developers and planners will consider the existing electrical grid in the area, coastal geology, and other land uses for the area where they will bring the electricity onshore.
Cables from the generators are buried under the seafloor and brought onshore near an existing substation to further distribute the electricity. Powerful storms can cause increased movement of sediment on the shore and seafloor in coastal areas. If cables get uncovered, the abrasion from moving sand and rocks can damage cables, the seafloor, and create a dangerous situation. Engineers must study the seafloor and conditions and create a plan at the start of the project to ensure that lines stay safely buried.
Construction of any utility-scale generation facility is a complicated matter in and of itself. Different trades need to be coordinated to do their respective jobs so that the work is not delayed or workers are not getting in each other’s way. Typically, materials arrive onsite as they are needed and are installed by specialists who also arrive on site as needed.
Offshore construction requires much more planning at the early stages. A single vessel, specially designed for the specific anchor or generator type, is loaded with everything needed to build a foundation if needed, install the generating equipment, and connect to the cables that tie into the grid. All of the materials and equipment must be loaded such that they are easily accessible in the order in which they are needed without upsetting the balance of the ship or knocking materials into the water. Some vessels are large enough to house workers, and some require workers to be ferried out to the worksite.
Most of the commercially-available construction vessels have been built specifically for installing offshore wind turbines. Because MHK technology is primarily in the experimental phase, commercial shipbuilders are not making vessels that are designed for installing or maintaining tidal, current, or wave technology. As those
technologies improve and move into utility-scale implementation, companies will design and manufacture ships that are specially suited for that task.
Just as special training and licenses are needed to operate large trucks and heavy construction equipment on land, pilots of commercial vessels are required to be licensed. As the size of the ship increases, the licensing and training requirements change.
The United States Coast Guard issues the licenses needed for most small watercraft. The license most people acquire is the OUPV, or “6-pack”, which is for vessels under 100 gross tons and with 6 or fewer passengers. These vessels are usually used for small tours, charter fishing, whale watching, etc. OUPV stands for Operator of Uninspected Passenger Vessel.
A passenger vessel with more than six passengers requires a Master 25-200 license. There are two Master license categories: Under 100 ton; and Under 200 ton. These licenses require several background and medical checks, endorsements, training hours, and passing an examination. A Master 25-200 license can be issued for operation in coastal waterways, or on inland waterways such as lakes and rivers.
Very large vessels are staffed by many different people with varying areas of expertise. Most people who work on these vessels have achieved some level of post-secondary education. The United States Merchant Marine Academy prepares civilians for work on ocean-going vessels including cargo ships, tankers, tugs, and cruise ships. Operated directly under the United States Department of Transportation Maritime Administration (MARAD), the Merchant Marine Academy is MARAD’s flagship educational institution.
There are also six state maritime academies that operate under a memorandum of agreement (MOA) with MARAD and provide bachelor degrees in many different areas pertaining to the design, operation, and maintenance of large ships. Graduation from one of these six academies also provides entry into the Merchant Marine. The six state academies are California State University Maritime Academy in Vallejo, CA; Great Lakes Maritime Academy in Traverse City, MI: Maine Maritime Academy in Castine, ME; Massachusetts Maritime Academy in Buzzards Bay, MA; SUNY Maritime College in Schuyler, NY, and Texas A&M Maritime Academy in Galveston, TX.
Image: Carron Brown on Flickr
The ship shown has been built for installing offshore wind turbines in relatively shallow water. The steal beams you see extending upward from the hull are extended downward to the seafloor once the ship reaches the construction site and the vessel is jacked up on them until the ship hovers over the water. Then construction of the wind turbine can be completed.
Like with any big project, developers and regulators involved in MHK projects must assess the potential impacts on all stakeholders and the environment. In the case of MHK generating sites, the stakeholders include the marine organisms that live or migrate through the affected areas, the habitats that support them, and those tasked with protecting the creatures.
Organisms that live on or near the seafloor are referred to as benthic organisms. Organisms that live in any environment are determined by a combination of abiotic (nonliving) and biotic (living) factors. Common benthic organisms that live at the depths where anchors for MHK devices may be built include corals, sponges, echinoderms, mollusks, cephalopods, and crustaceans. While the initial construction phase will disrupt some benthic organisms and fish, the system and its components may provide additional surface area that these organisms can colonize, thus creating an artificial reef structure. Artificial reefs have been used in other parts of the globe to enhance marine ecosystems.
Organisms that live in the water column are referred to as pelagic organisms. Some, like most phytoplankton, are free floating, and many are active swimmers including, zooplankton, fish, and marine mammals. The greatest concerns related to offshore development is around potential impacts on marine mammals, fish, sea birds, and turtles.
One significant wildlife concern related to marine hydrokinetic project siting is the impact on whales or other migratory creatures. Knowledge of migration patterns is important to ensure that the timing of construction does not coincide with when species are in the area. To assess potential risk, scientists are studying behaviors and feeding patterns through direct observation from ships and tagging studies.
Siting any technology so that they do not interfere with sealife is important. But, how does the underwater ecosystem respond after the devices are in place? While this has not yet been studied widely for MHK generating sites, diver and AUV images from offshore wind turbines and abandoned oil and natural gas equipment across the globe demonstrate that underwater components serve to provide a safe structure for reef growth, fish populations, and other marine life. It is likely that any anchoring or foundational structures for MHK generation will yield similar results.
The development and operation of MHK projects could create many jobs in the future. Each of the phases of operation will have permanent or temporary jobs created.
Research and Development – Analysts, scientists, and engineers will be needed to develop plans to look at the feasibility, environmental impact, and optimization technology.
Installation and Testing – Workers will be needed where the turbine components are built and deployed. Mariners and technicians will be called upon to complete phases of marine construction. Port Authority personnel may also help with the construction and provide lay-down areas for the connectivity infrastructure. Engineers, technicians, electricians, construction specialists, and testing specialists will work to ensure that the turbines are ready to begin producing power and put it on the grid.
Operations and Management – Engineers will be needed to monitor and deliver the resulting power from the facilities farm to those who draw power from the grid every day. There will be ongoing jobs for managers, analysts, engineers, technicians, and support personnel of all kinds throughout the life cycle of the project.
Research Scientist - studies and defines the basic science behind our natural systems and provides data to the scientific and technical community
Environmental Scientist - studies environmental systems and their interactions often characterizing effects of the built environment on natural systems and animal species
Marine Geologist / Geophysicist - studies the geology of our Earth including the makeup of the ocean floor and its component layers
Naval Architect - designs marine vessels to particular purposes and to accomplish specific missions
Design Engineer - designs major systems and subsystems within any technological machine - ensuring that the component parts work together to handle all loads they encounter and accomplish the design’s overall purpose as efficiently as possible.
Marine Engineer - sub-specialty of mechanical engineering that operates in the marine environment, and designs everything from docks to buoys to systems for ships and other vessels
Coastal Engineer - sub-branch of civil engineering that focuses on coastal systems and structures that interact with shorelines and water bodies
Mechanical Engineer - engineering field that focuses on mechanical and thermal systems used in technical applications
Electrical Engineer – engineering field that focuses on both electrical (power) systems and electronic (control systems)
Civil Engineer - engineering field that focuses on the built environment - in buildings, roads, bridges, water, and sewer systems
Environmental Engineer - engineering specialty that focuses on the natural environment’s integration with the built environment - often finding innovative ways to accomplish project objectives while addressing problems such as runoff or contamination
Cable Technician - specialized technician who is responsible for installing and terminating cable systems connecting the devices to each other and to shore
GIS / Mapping Technician - specialized technician who is responsible for providing geographic characterization of the natural environment and precise location of technical systems installed within it
Inspection Technician / Drone Pilot/ AUV operator - specialized technician trained to inspect technical systems by operating robotic drone systems with digital video cameras on-board
Vessel Crew/Mariner - captains, deck hands, and vessel engineers (on larger vessels) execute shipping, construction, and research missions in oceans and rivers
Welders - specialized technicians that are trained to join metals through a variety of technologies and sometimes below the surface of bodies of water, can be land-based or a certified, specialized welding diver
Diver - professionals who work under water to inspect, document and repair systems
Outside Machinist - professional mechanic capable of installing mechanical systems on vessels
Fabricator / Shipfitter - specialized technician that prepares and machines large metal parts to assemble
Painter / Coatings Specialist - specialized technician that deals with the application of primers, paints and other coating systems to protect system components
Rigger / Payload Specialist - specialized technician that deals with safely and efficiently moving and securing loads that are in transport or that are being made into larger assemblies during construction
Project Manager - professional manager that oversees the entire project, from beginning to end, including the integration of the component systems
Project Analyst - professional analyst who reports to the Project Manager and prepares detailed analysis and intelligence on specifically assigned parts of the project
Administrative Support Staff - office professionals that support and enable the work of the team to proceed.
? Question
How will electric current passing through a wire affect a compass?
Hypothesis
In your science notebook, write a hypothesis stating how you think electric current passing through a wire will affect a compass.
9-volt Battery
Compass
Alligator clip
Nail
1. Take the nail and hold it next to the compass. Move the nail around over the compass and observe the interaction. Record your observations in your science notebook.
2. Connect one end of the alligator clip to the negative terminal of the 9-volt battery.
3. Wrap the wire of the alligator clip around the nail, starting at the flat end or head of the nail, and moving towards the pointed end. Leave enough wire to reconnect the battery.
4. Connect the other alligator clip to the positive terminal of the 9-volt battery.
5. Hold the pointed end of the nail near the compass. Observe the interaction. Record your observations in your science notebook.
6. Hold the flat end or head of the nail near the compass. Observe and record the interaction.
1. What did the electric current create when it passed through the wire around the nail?
2. What are the two poles of a magnet called?
3. Which pole was the flat end of the nail? Which pole was the pointed end of the nail? Support your answer with your observations.
4. How do the parts of an electromagnet work together to create magnetism?
5. Will you accept or reject your hypothesis? Upon what experimental evidence are you basing your conclusion?
How are electricity, magnetism, and motion related?
Hypothesis
Write a hypothesis stating what you think about the relationship between electricity, magnetism, and motion.
Two motors
Two alligator clips
9-volt Battery
Disassembled motor
Hand generated flashlight
Masking tape
1. Fold a piece of tape like a flag onto the shaft of the assembled motor and put an X on one side.
2. Connect the terminals of one of the assembled motors to the 9-volt battery with the alligator clips. What happens? Make observations in your science notebook.
3. Connect the alligator clips to opposite terminals of the battery. Observe and illustrate your observations. (Hint: pay attention to the direction of the spinning motor shaft.)
4. Look at the disassembled motor. Remove the rotor (coil of wire and magnets). Record and illustrate the disassembled motor and label the parts in your science notebook.
5. Connect the terminals of the two assembled motors to each other using alligator clips (no battery is involved here). Grasp the shaft of the motor without tape and give it a hard, swift spin, observing the tape on the shaft of the other motor. Record your observations.
6. Using the assembly from step #5, turn the shaft in the opposite direction and observe the tape. Record your observations. Make a diagram in your science notebook, indicating the direction the taped shaft spins according to the direction of the motor without tape.
7. Observe the hand generated flashlight but do not shake it or attempt to generate light yet. Pay attention to the coil of wire and the magnets.
8. Draw the hand generated flashlight in your science notebook and label the parts. Try to explain how the flashlight works.
9. Shake the hand generated flashlight several times and observe what happens. Record your observations in your science notebook.
1. What energy transformation occurs in this activity?
2. What happened to the motion of the motor when the clips on the battery were swapped? Explain what is occurring in the battery to cause this.
3. Think back to when the two motors were hooked together. What happened to the direction of the shaft on the second motor when you reversed the turn on the first? Explain why this occurred.
4. Will you accept or reject your hypothesis? Upon what experimental evidence are you basing your conclusion?
How is electricity generated?
! Caution
The magnets used in this model are very strong. Refer to page 28 for rmore safety information.
Use caution with nails and scissors when puncturing the bottle.
1 Small bottle
1 Rubber stopper with ¼” hole
1 Wooden dowel (12” x ¼”)
4 Strong rectangle magnets
1 Foam tube
1 Small nail
1 Large nail
Spool of magnet wire
Permanent marker
1 Pair sharp scissors
Masking tape
Fine sandpaper
1 Push pin
1 Multimeter with alligator clips
Hand operated pencil sharpener
Ruler
Utility knife (optional)
1. If needed, cut the top off of the bottle so you have a smooth edge and your hand can fit inside. This step may not be necessary. If necessary, a utility knife may be of assistance.
2. Pick a spot at the base of the bottle. (HINT: If the bottle you are using has visible seams, measure along these lines so your holes will be on the opposite sides of the bottle.) Measure 10 centimeters (cm) up from the base and mark this location with a permanent marker.
3. On the exact opposite side of the bottle, measure 10 cm up and mark this location with a permanent marker.
4. Over each mark, poke a hole with a push pin. Do not distort the shape of the bottle as you do this. CAUTION: Hold a rubber stopper inside the bottle behind where the hole will be so the push pin, and later the nails, will hit the rubber stopper and not your hand, once it pokes through the bottle.
5. Widen each hole by pushing a nail through it. Continue making the hole bigger by circling the edge of the hole with the side of the nail. (A 9/32 drill bit twisted slowly also works, using a rubber stopper on the end of the bit as a handle.)
6. Sharpen one end of the dowel using a hand operated pencil sharpener (the dowel does not have to sharpen into a fine point). Push the sharpened end of the dowel rod through the first hole. Circle the edge of the hole with the dowel so that the hole is a little bigger than the dowel.
7. Remove the dowel and insert it into the opposite hole. Circle the edge of the hole with the dowel so that the hole is a little bigger than the dowel. An ink pen will also work to enlarge the hole. Be careful not to make the hole too large, however.
8. Insert the dowel through both holes. Hold each end of the dowel and swing the bottle around the dowel. You should have a smooth rotation. Make adjustments as needed. Take the dowel out of the bottle and set aside.
9. With a permanent marker, label one hole “A” and the other hole “B.”
1. Tear 6 pieces of tape approximately 6 cm long each and set aside.
2. Take the bottle and the magnet wire. Leave a 10 cm tail, and tape the wire to the bottle about 2 cm below hole A. Wrap the wire clockwise 200 times, stacking each wire wrap on top of each other. Keep the wire wrap below the holes, but be careful not to cover the holes, or get too far away from the holes.
3. DO NOT cut the wire. Use two pieces of tape to hold the coil of wire in place; do not cover the holes in the bottle with tape (see diagram).
4. Without cutting the wire, move the wire about 2 cm above the hole to begin the second coil of wraps in a clockwise direction. Tape the wire to secure it in place.
5. Wrap the wire 200 times clockwise, again stacking each wrap on top of each other. Hold the coil in place with tape.
6. Unwind 10 cm of wire (for a tail) from the spool and cut the wire.
7. Check your coil wraps. Using your fingers, pinch the individual wire wraps to make sure the wire is close together and close to the holes. Re-tape the coils in place as needed.
1. Measure 4 cm from the end of the foam tube. Using scissors, carefully score a circle around the tube. Snap the piece from the tube. This piece is now your rotor.
2. On the flat ends of the rotor, measure to find the center point. Mark this location with a permanent marker.
3. Insert the small nail directly through the rotor’s center using your mark as a guide.
4. Remove the small nail and insert the bigger nail.
5. Remove the nail and push the dowel through, then remove the dowel and set aside. Do NOT enlarge this hole.
6. Stack the four magnets together. While stacked, mark one end (it does not matter which end) of each of the stacked magnets with a permanent marker as shown in Diagram 1.
7. Place the magnets around the foam piece as shown in Diagram 2. Make sure you place the magnets at a distance so they do not snap back together.
8. Wrap a piece of masking tape around the curved surface of the rotor, sticky side out. Tape it down at one spot, if helpful.
9. Lift the marked end of Magnet 1 to a vertical position and attach it to the rotor. Repeat for Magnets 2, 3, and 4.
10. Secure the magnets in place by wrapping another piece of masking tape over the magnets, sticky side in (Diagram 3).
WARNING: These magnets are very strong. Use caution when handling.
1. Slide the sharp end of the dowel through Hole A of the bottle.
2. Inside the bottle, put on a stopper, the rotor, and another stopper. The stoppers should hold the foam rotor in place. If the rotor spins freely on the axis, push the two stoppers closer against the rotor. This is a pressure fit and no glue is needed.
3. Slide the sharp end of the dowel through Hole B until it sticks out about 4 cm from the bottle.
4. Make sure your dowel can spin freely. Adjust the rotor so it is in the middle of the bottle.
5. Using fine sandpaper, remove the enamel coating from 4 cm of the end of each wire tail, leaving bare copper wires. (This step may need to be repeated again later if re-testing the model).
The stoppers can be cut in half so that one stopper is made into two, to allow for more materials. These often slide more easily on the dowel. This must be done using sharp scissors or a utility knife, and can often be dangerous. As this step is not required (the kit supplies you with two stoppers to use), exercise extreme caution.
If the foam rotor fits snugly on the dowel, put the stoppers on the outside of the bottle to help center the rotor in the bottle. Leave enough space to allow free rotation of the rotor.
The dowel may be lubricated with lip balm or oil for ease of sliding the stoppers, if necessary.
If a glue gun is available, magnets can be attached to the rotor on edge or on end to get them closer to the coils of wire. Use the magnet to make an indentation into the foam. Lay down a bead of glue, and attach the magnets. If placing the magnets on end, however, make sure they clear the sides of the bottle for rotation.
1. Connect the leads to the multimeter to obtain a DC Voltage reading.
2. Connect one alligator clip to each end of the magnet wire. Connect the other end of the alligator clips to the multimeter probes.
3. Set your multimeter to DC Voltage 200 mV (millivolts). Voltage measures the pressure that pushes electrons through a circuit. You will be measuring millivolts, or thousandths of a volt.
4. Demonstrate to the class, or allow students to test, how spinning the dowel rod with the rotor will generate electricity as evidenced by a voltage reading. As appropriate for your class, you may switch the dial between 200 mV and 20 volts. Discuss the difference in readings and the decimal placement.*
5. Optional: Redesign the generator to test different variables including the number of wire wraps, different magnet strengths, and number of magnets.
*Speed of rotation will impact meter readings.
Note: Your multimeter may look different than the one shown. Read the instruction manual included in the multimeter box for safety information and complete operating instructions.
If you are unable to get a voltage or current reading, double check the following:
Did you remove the enamel coating from the ends of the magnet wire?
Are the magnets oriented correctly?
The magnet wire should not have been cut as you wrapped 200 wraps below the bottle holes and 200 wraps above the bottle holes. It should be one continuous wire.
Are you able to spin the dowel freely? Is there too much friction between the dowel and the bottle?
Is the rotor spinning freely on the dowel? Adjust the rubber stoppers so there is a tight fit, and the rotor does not spin independently.
The magnets in the Science of Electricity Model are very strong. In order to separate them, students should slide/twist them apart. Please also take the following precautions:
When you set the magnets down, place them far enough away from each other that the magnets won’t snap back together.
The tape should hold the magnets on. If you want something stronger and more permanent you can use hot glue.
When you are finished with the magnets and ready to store them, put a small piece of cardboard between them.
Keep magnets away from your computer screen, cell phone, debit/credit cards, and ID badges.
Your teacher will assign your group a specific topic in MHK generation to study. Your assignment is to construct a model of the technology and present the technology to your class in written or digital form. Your teacher may want you to make the model an actual working, generating model, or it may simply be for illustrative purposes. Use the following sections to plan and work on your project with your groupmates.
Technology Assigned:
What design(s) are most prominent in this area of marine hydrokinetic generation? There may be one or two, or there may be several. List them and put a star next to the one or two that are most promising.
Make a sketch of the generator you will model:
What materials will you use? Identify which part(s) of the model will be made from which materials.
As you study your MHK topic, make sure you identify the MHK source type (current, OTEC, tide, wave, etc.) and make a list of the pros and cons of the technology. Identify whether the technology is in planning, research and development, early testing, or later testing stage. Identify the major researcher(s) working on the technology you are showcasing. The resources listed below may be helpful as you work on your project. You may also use other sources as approved by your teacher.
Advantages and disadvantages of technology:
MHK Resources
U.S. Energy Information Administration – www.eia.gov
idal Power Information -https://www.eia.gov/energyexplained/hydropower/tidal-power.php
Georgia Coastal Research Council - https://www.gcrc.uga.edu/tidal-energy/
Ocean Renewable Power Company - https://orpc.co/
U.S Department of Energy, Water Power Technologies Office
https://www.energy.gov/eere/water/marine-energy-program
https://www.energy.gov/eere/water/marine-and-hydrokinetic-technology-development-and-testing
https://www.energy.gov/eere/water/downloads/marine-energy-united-states-overview-opportunities
National Renewable Energy Laboratory (NREL)
https://maps.nrel.gov/marine-energy-atlas
Overview of opportunities (Feb 2021) https://www.nrel.gov/docs/fy21osti/78773.pdf
US Fish and Wildlife Service – Hydrokinetic Energy - https://www.fws.gov/node/265253
National Hydropower Association – Marine and Hydrokinetic - https://www.hydro.org/policy/technology/marine-hydrokinetic/
NC State University, Mechanical and Aerospace Engineering - https://www.mae.ncsu.edu/corelab/59-2/
PRIMRE - https://openei.org/wiki/PRIMRE
https://openei.org/wiki/PRIMRE/MRE_Basics
https://openei.org/wiki/PRIMRE/Databases/Projects_Database
How can wave energy be captured to generate electricity?
Have you ever wondered why some objects float, and others do not? The answer lies in the density of an object. Density is defined as the mass per unit volume and is measured in grams per cubic centimeter (g/cm3). Let’s assume we have a painted block, and we don’t know what it’s made of. Will it float in water? If the block’s density is lower than the density of water, it will float. If the block has a density higher than water’s density, it will sink. If the densities are equal, the object will hover in a suspended state, neither floating nor sinking. The science behind floating extends deeper than just density. The force that keeps an object floating on a fluid, like water, is called buoyant force, or the force exerted on an object that is partly or completely immersed in a fluid. Buoyancy is caused by the differences in pressure acting on opposite sides of the immersed object. The unit of the buoyant force is the Newton (N).
Imagine we have one kilogram (1 kg) of steel. If that steel was shaped into a cube, it would be about 0.5 meters (50 cm) on a side, and have a volume of about 0.125 m3 (0.5m x 0.5m x 0.5m). The weight of the cube would be about 9.81 N, because W=mg, where W is the weight (N), m is the mass of the object (kg), and g is the acceleration due to gravity (9.81 m/s2), and 1.00 × 9.81 = 9.81. In order to float, the water must be able to balance the weight of the cube with a supporting force pushing up on the cube. So, how forcefully is the water pushing up on the cube?
The buoyant force of the cube is calculated using the equation Fb= ρ × V × g where Fb is the buoyant force, ρ is the density of the water (1.00 kg/m3), V is the volume of the water displaced by the object (m3), and g is the acceleration due to gravity (9.81 m/s2). Because our cube will displace a maximum of 0.125 m3 of water, and the density of water is 1.00 kg/m3, the maximum buoyant force water can provide the cube is 1.00 × 0.125 × 9.81, or 1.23 Newtons. Since the weight is greater than the maximum buoyant force, the cube sinks. And, given that our cube is made of steel, this isn’t at all surprising to anyone who understands that dense objects like steel cubes sink. Suppose we flatten that 1.00 kg of steel out and make a boat out of it, so the volume is 100 times bigger? The volume of the boat is now 12.5 m3, but the weight is the same 9.81 N. Will it float? The weight is the same, so only the buoyant force will change. Now our buoyant force is calculated Fb = 1.00 × 12.5 × 9.81, and comes out to 123 Newtons. Indeed, the boat floats (as we would expect). Comparing the steel cube to the steel boat illustrates Archimedes Law, that an immersed object is buoyed by a force equal to the weight of the fluid displaced. When we calculated the maximum buoyant force, Fb, we calculated the weight of the water displaced if the entire steel cube or boat was completely submerged, (the top was exactly even with the surface of the water). We don’t want a boat to fill with water. If the weight of the object is less than the weight of the water it displaces, it will float. If the weight of the object exceeds the weight of the water it displaces, kerplunk! Down it goes.
2 Shake-light flashlights
1 Heavy-gauge, zip-close, plastic bag (large enough to hold one flashlight)
Alligator clips
Digital multimeter or galvanometer
Empty 1-gallon water bottle, with cap
Utility knife
Balance
1. Measure the mass of the flashlight and record it in the data table.
2. Disassemble the flashlight according to your teacher’s directions.
3. Disconnect the switch, measure its mass, and set it aside. Disconnect the wires from the circuitry of the flashlight. Remove the circuitry, LED, and lens, leaving the plastic housing, wire coil, and magnet. Record the mass of each component in the data table.
4. Use sandpaper to remove any enamel coating off the wire ends. Be careful, the wire is very fine and can break easily.
5. Measure the mass of the plastic bag. Record the mass in the data table.
6. Place the plastic housing, coil, and magnet assembly inside the plastic bag. Connect the two alligator clips to the sanded ends of wire. It may be helpful to loop the wire around one jaw of the clip to keep it in place.
7. Extend the alligator clips out of the plastic bag. Seal the bag around the extended wires.
8. Cut a slit that runs from 3 cm from the bottom to 3 cm from the top in one side of the water bottle. The slit should be perpendicular to the table if you stand the water bottle up. Cover the two sides of the slit with tape.
9. Put the cap on the bottle and lay it on its side with the slit at the top. Pour water through the slit until the bottle is about half full.
10. Push the plastic bag and flashlight assembly through the slit so the flashlight floats on the surface of the water.
11. Connect the alligator clips to the multimeter or galvanometer. If you are using a digital multimeter, turn it on to the appropriate setting as instructed by your teacher.
12. Rock the bottle back and forth, creating waves inside the bottle. The flashlight should rock end-to-end on the surface of the water, forcing the magnet back-and-forth through the wire coil.
13. Observe the meter or galvanometer output and record your data.
14. Remove the plastic bag and flashlight.
15. Open the flashlight assembly again, and add the second magnet.
16. Repeat steps 8-11, recording your data and observations.
17. Disassemble everything according to your teacher’s instructions.
Copy the following table and statement into your science notebook.
Record your multimeter or galvanometer data below:
1. What was the total mass of the flashlight? _____ g What was the mass of the parts you put in the bag? _____ g What was the mass of the entire floating flashlight assembly? _____ g
2. Calculate the gravitational force, in Newtons, of the floating flashlight assembly.
3. According to Archimedes Principle, what was the weight of water displaced by the floating flashlight? Explain how you know.
4. If you decided to scale this model up so it generated enough electricity for a home, or a group of homes, or even a whole neighborhood, what design constraints do you think you would encounter? Think about materials, size, manufacturing, and everything involved in scaling this model up.
Clear plastic tubing
Two rubber stoppers
Magnet wire
1 cylindrical magnet
Parafilm or other waterproofing
Alligator clips
Digital multimeter or galvanometer
Waterproof duct tape or hot glue gun
1. Leave at least 30 cm of a wire tail. Keeping the wire tightly packed, wrap magnet wire around the center of the plastic tubing. Your teacher may tell you how many wire turns you should use. However, if you are permitted to determine the number of wire turns, make sure you record this number for later discussion. NOTE: the wire must remain in one long, continuous piece. When you have finished winding wire around the tubing, leave another 30 cm tail before cutting the wire.
2. Use waterproof tape or a hot glue gun to hold the wire coil in place.
3. Insert a rubber stopper firmly into one end of the tubing.
4. Insert the magnet into the tubing.
5. Insert the other rubber stopper into the other end of the tubing.
6. Wrap the stopper-tubing junctions well with parafilm or other waterproofing substance.
7. Use sandpaper to remove the enamel from at least 3 cm from both wire “tails”.
8. Connect the alligator clips to the wire tails. Wrap both junctions well with water proofing.
9. Connect the other ends of the alligator clips to the leads on the multimeter or connections on the galvanometer.
10. Keeping the galvanometer out of the water, place the tubing assembly in the water and allow it to stabilize.
11. Gently rock the tub of water to create waves, and observe the reading on the multimeter or galvanometer.
In your science notebook, make a diagram of your model. Include the number of wire turns you have wrapped around the tubing. Record the observations you make of your model, including the galvanometer reading corresponding to the position of the generator model.
1. How does the output of this model compare to the flashlight model in Part 1? Use data collected from the activity to support your claim.
2. What design improvements might you make to your model to make it perform better? Be specific. If your teacher allows, test your improvement ideas.
Clear plastic tubing
Cylindrical magnets
Fishing line
Hot glue gun
Magnet wire
Pool noodle, Styrofoam, or other buoyant materials
Sandpaper
Fishing bobber or floats
Galvanometer
Waterproof duct tape
Repurposed plastic containers
Heavy objects for anchoring
Alligator clips
1. Think about what you know about waves. What action(s) do they produce? How can you take advantage of that action with a vertical, rather than horizontal, wave generator model?
2. Sketch a design idea or two in your science notebook that uses the materials your teacher provides or approves. Remember the critical parts every generator must have: A coil of wire; A moving magnetic field; One moving part; One stationary part. How can you accomplish while keeping your generator in one location and in a vertical position?
3. Show your sketches to your teacher and discuss which would likely work best.
4. Build your model according to your design. Make a diagram and record measurements, wire turn numbers, etc. so it could be replicated by someone who has not seen it.
5. Put your model in the tub of water to test its stability and functionality. Don’t worry about connecting it to a galvanometer yet.
6. Record your test results. Are there any items you need to change? Record those changes in your notebook.
7. Make the changes you feel are necessary and re-test.
8. Repeat steps 5-7 until you have a good working model that remains vertical, in the same location, and will probably generate some electricity.
9. Remove your model from the water and dry the ends of the wire.
10. Connect the wire to alligator clips that are also connected to the galvanometer. Wrap any connections that might get wet with parafilm.
11. Place your model back in the water. Gently rock the tub to create waves while observing the galvanometer. Record your results.
1. In your science notebook, record all of your design sketches, diagrams, and changes. Make sure someone who has not seen your final, working model would be able to duplicate it.
2. As you rock the tub of water and create waves, record the galvanometer reading and relate it to the position of the magnet with respect to your wire coil.
1. What design challenges did you encounter that were unexpected? Be specific. Cite evidence from the activity.
2. How does the output of a model generator in the vertical position compare to the output of a similar model generator in the horizontal position? Be specific, citing evidence from parts 2 and 3.
3. Imagine you are an engineer working on a design team to come up with a working, utility-scale wave generator. Which of these two models, horizontal rocking or vertical bobbing, would you use? What scale-up challenges do you think you would encounter building something that size?
alternating current an electric current that reverses its direction at regular intevals or cycles; in the US, the standard is 120 reversals or 60 cycles per second; typically abbreviated as AC ampere (amp) a measurement of electric current
Archimedes Law the buoyant force on an object is equal to the weight of the fluid displaced by the object atom the most basic unit of matter
attenuator long, multi-segmented device that floats on the surface of the ocean; it harnesses energy as the motion of the waves causes flexing between each segment autonomous underwater vehicle (AUV)
robot that travels underwater without input from an operator
benthic bottom-dwelling; organisms that are benthic reside at the bottom of lakes, rivers, streams, and the ocean buoyant force upward force of a fluid on a body placed in it, such as water on a boat
chemical energy energy stored in the bonds between atoms; this energy is released when one or more substances change into new substances
circuit pathway for electric current to flow
conductor material that conduct electricity with little or no resistance
Coriolis effect result of earth’s rotation on the atmosphere and ocean waters; the Coriolis effect makes storms swirl counterclockwise in the Northern Hemisphere and clockwise in the Southern Hemisphere
crest topmost point of a wave
current energy energy in the moving water of ocean currents
density amount of mass per unit volume
desalinated ocean water with the salt removed direct current electric current that flows in one direction only
elastic energy energy stored in an object that has been stretched or pulled out of its natural state
electric current electrical energy moving through a conductor
electric grid system of power plants, distribution lines, and transmission lines that provides electricity
electrical energy energy transferred by moving electrons
electromagnet magnet produced by electric current
electromagnetic induction production of voltage across an electrical conductor in a changing magnetic field
electron the particle in an atom that carries a negative electrical charge energy the ability to do work or make a change
energy source substances or places from which we obtain energy
estuary tidal mouth of a large river, where the tide meets the stream
ferrous metal containing iron
fetch distance of water over which wind blows generator device that converts motion energy into electrical energy
gravitational potential energy energy stored in an object held up against gravity, such as water behind a dam or a child at the top of a slide
gyre spiral or vortex
heat engine device that changes heat into work by pulling thermal energy from a reservoir and using it to do work
hydrokinetic energy system generating electricity from waves or directly from the flow of water in ocean currents, tides, or inland waterways
insulator material that resists electrical current flow
kinetic energy energy of motion
load the part of an electrical circuit that uses electricity to do work, such as a light bulb
marine hydrokinetics renewable energy source from the movement of ocean water in currents, tides, and waves
mechanical motion energy motion energy, the movement of objects and substances from one place to another
mooring tying and making stationary a device on water; a site where vessels, barges, or other floating objects can be tied
nonrenewable energy source
an energy source with a long term replenish rate and reserves that are limited, including petroleum, coal, natural gas, uranium, and propane
nuclear energy energy contained in the nucleus of an atom
ohm
a measurement of resistance in an electrical circuit
osmosis movement of water through a membrane from low concentration of salt to high concentration of salt
osmotic power using osmosis to generate electricity
OTEC ocean thermal energy conversion
outer continental shelf areas in the oceans and Gulf of Mexico under the jurisdiction of the government of the United States and not the individual states themselves
parallel circuit conductor or system of conductors providing more than one pathway through which electrical current can flow
pelagic organism living in the water column between the surface and bottom of a lake or ocean period time it takes for the crests of two concurrent waves to pass a stationary point point absorber device that captures energy from the up-and-down motion of waves
potential energy stored energy
power the rate at which energy is produced or consumed
power plant site where electricity is generated, such as a nuclear power plant or hydropower generating facility pressure force per unit of area; pressure is measured in Newtons / m2 or pounds per square inch
prototype the first object produced from a new design
pumped storage
hydropower water pumped to a high reservoir during times of electricity abundance, then released to flow downhill and generate electricity when demand is high
radiant energy energy that travels in electromagnetic waves
renewable energy source
resistance
an energy source with a short term replenish rate, including biomass, geothermal, hydropower, solar, and wind
measure of the opposition to current flow in an electrical circuit, measured in ohms
resistor circuit component designed to control the current through the circuit
secondary source of energy often called an energy carrier; requires another source, like coal, to be converted for generation; electricity and hydrogen are examples
series circuit conductor or system of conductors providing only one pathway through which electrical current can flow siting process of determining the location for an installation, such as a power plant or wind farm sluice a sliding gate for controlling the flower of water
sound energy movement of energy through substances in longitudinal waves
substation
location where step-down transformers reduce the voltage of electricity from large transmission lines before distributing it to neighborhoods and businesses
thermal energy internal energy of substances that causes the atoms or molecules to move tidal barrage a facility built like a dam that allows the tides to power turbines and generate electricity
tidal bulge the area of the Earth where the moon’s gravitational force creates high tides
tidal energy energy stored in the movement of tides
tidal fence an open structure with vertical-axis turbines mounted across a channel
tidal power hydropower derived from the rise and fall of the tides
tidal turbine underwater turbine driven by the movement of the tides
tide ebb and flow of water due to the gravitational pull of the moon on the oceans
transformer a device that changes the voltage of electricity
trough lowest point of a wave
turbidity cloudiness or opacity of water with suspended matter
turbine machine of curved blades or buckets that transforms the linear motion of a moving fluid into rotational motion to turn a generator
volt measure of electric potential or force
voltage measure of the pressure or potential difference under which electricity flows through a circuit watt measure of power
wave energy energy stored in waves in the ocean
wavelength distance from one point on a wave to the same point on the next wave
This portal was sponsored by the U.S. Department of Energy, Office of Energy Efficiency and Renewable Energy, Water Power Technologies office, and is part of a larger open energy information site supported and maintained by the National Renewable Energy Laboratory. openei.org/wiki/PRIMRE/STEM
Explore a variety of STEM resources for MRE and MHK, including education and training programs, educator resources, career pathways, prizes & competitions and site tours.
https://openei.org/wiki/PRIMRE/MRE_Basics
The movement of water in the world’s oceans creates a vast store of kinetic energy. Marine Renewable Energy (MRE), also known as Marine Hydrokinetics (MHK), can be harnessed to generate electricity to power homes, transport and industries. MRE encompasses wave energy — power from the movement of surface waves, ocean current energy — power from the kinetic energy of ocean current, ocean thermal energy conversion (OTEC) — power from the heat differential of different thermal layers within a body of water, and salinity gradient — power from the difference in salt concentration between two fluids.
The NEED Youth Energy Conference and Awards gives students more opportunities to learn about energy and to explore energy in STEM (science, technology, engineering, and math). The annual June conference has students from across the country working in groups on an Energy Challenge designed to stretch their minds and energy knowledge. The conference culminates with the Youth Awards Ceremony recognizing student work throughout the year and during the conference.
For More Info: www.youthenergyconference.org
All NEED schools have outstanding classroom-based programs in which students learn about energy. Does your school have student leaders who extend these activities into their communities? To recognize outstanding achievement and reward student leadership, The NEED Project conducts the National Youth Awards Program for Energy Achievement.
Share Your Energy Outreach with The NEED Network! This program combines academic competition with recognition to acknowledge everyone involved in NEED during the year—and to recognize those who achieve excellence in energy education in their schools and communities.
What’s involved?
Students and teachers set goals and objectives and keep a record of their activities. Students create a digital project to submit for judging. In April, digital projects are uploaded to the online submission site.
Check out:
www.NEED.org/need-students/youth-awards/ for more application and program information, previous winners, and photos of past events.
AES
AES Clean Energy Development
American Electric Power Foundation
Appalachian Voices
Arizona Sustainability Alliance
Baltimore Gas & Electric
Berkshire Gas - Avangrid
BP America Inc.
Bob Moran Charitable Giving Fund
Cape Light Compact–Massachusetts
Celanese Foundation
Central Alabama Electric Cooperative
The City of Cuyahoga Falls
Clean Virginia
CLEAResult
ComEd
Con uence
ConocoPhillips
Constellation
Delmarva Power and Light
Department of Education and Early Childhood
Development - Government of New
Brunswick, Canada
Dominion Energy, Inc.
Dominion Energy Charitable Foundation
DonorsChoose
East Baton Rouge Parish Schools
East Kentucky Power Cooperative
EcoCentricNow
EDP Renewables
EduCon Educational Consulting
Enel Green Power North America
ENGIE
Entergy
Eversource
Exelon
Exelon Foundation
Foundation for Environmental Education
FPL
Generac
Georgia Power
Gerald Harrington, Geologist
Government of Thailand–Energy Ministry
Greater New Orleans STEM
GREEN Charter Schools
Green Power EMC
Guilford County Schools–North Carolina
Honeywell
Iowa Governor’s STEM Advisory CouncilScale Up
Iowa Lakes Community College
Iowa State University
Illinois Clean Energy Community Foundation
Illinois International Brotherhood of Electrical Workers Renewable Energy Fund
Independent Petroleum Association of New Mexico
Kansas Corporation Energy Commission
Kansas Energy Program – K-State Engineering Extension
Katy Independent School District
Kentucky Environmental Education Council
Kentucky O ce of Energy Policy
Kentucky Power–An AEP Company
Liberty Utilities
Llano Land and Exploration
Louisiana State Energy O ce
Louisiana State University – Agricultural Center
LUMA
Marshall University
Mercedes Benz USA
Minneapolis Public Schools
Mississippi Development Authority–Energy Division
Motus Experiential
National Fuel
National Grid
National Hydropower Association
National Ocean Industries Association
National Renewable Energy Laboratory
NC Green Power
Nebraskans for Solar
NextEra Energy Resources
Nicor Gas
NCi – Northeast Construction
North Shore Gas
O shore Technology Conference
Ohio Energy Project
Oklahoma Gas and Electric Energy Corporation
Omaha Public Power District
Paci c Gas and Electric Company
PECO
Peoples Gas
Pepco
Performance Services, Inc.
Permian Basin Petroleum Museum
Phillips 66
PowerSouth Energy Cooperative
Prince George’s County O ce of Human Resource Management (MD)
Prince George’s County O ce of Sustainable Energy (MD)
Providence Public Schools
Quarto Publishing Group
The Rapha Foundation
Renewable Energy Alaska Project
Rhoades Energy
Rhode Island O ce of Energy Resources
Salal Foundation/Salal Credit Union
Salt River Project
Salt River Rural Electric Cooperative
Schneider Electric
C.T. Seaver Trust
Secure Futures, LLC
Shell
Shell Carson
SMUD
Society of Petroleum Engineers
South Carolina Energy O ce
Southern Company Gas
Snohomish County PUD
SunTribe Solar
United Way of Greater Philadelphia and Southern New Jersey
Unitil
University of Iowa
University of Louisville
University of North Carolina
University of Northern Iowa
University of Rhode Island
U.S. Department of Energy
U.S. Department of Energy–O ce of Energy
E ciency and Renewable Energy
U.S. Department of Energy - Solar Decathlon
U.S. Department of Energy - Water Power Technologies O ce
U.S. Department of Energy–Wind for Schools
U.S. Energy Information Administration
United States Virgin Islands Energy O ce
Virginia Cooperative Extension
We Care Solar
West Virginia O ce of Energy
West Warwick Public Schools