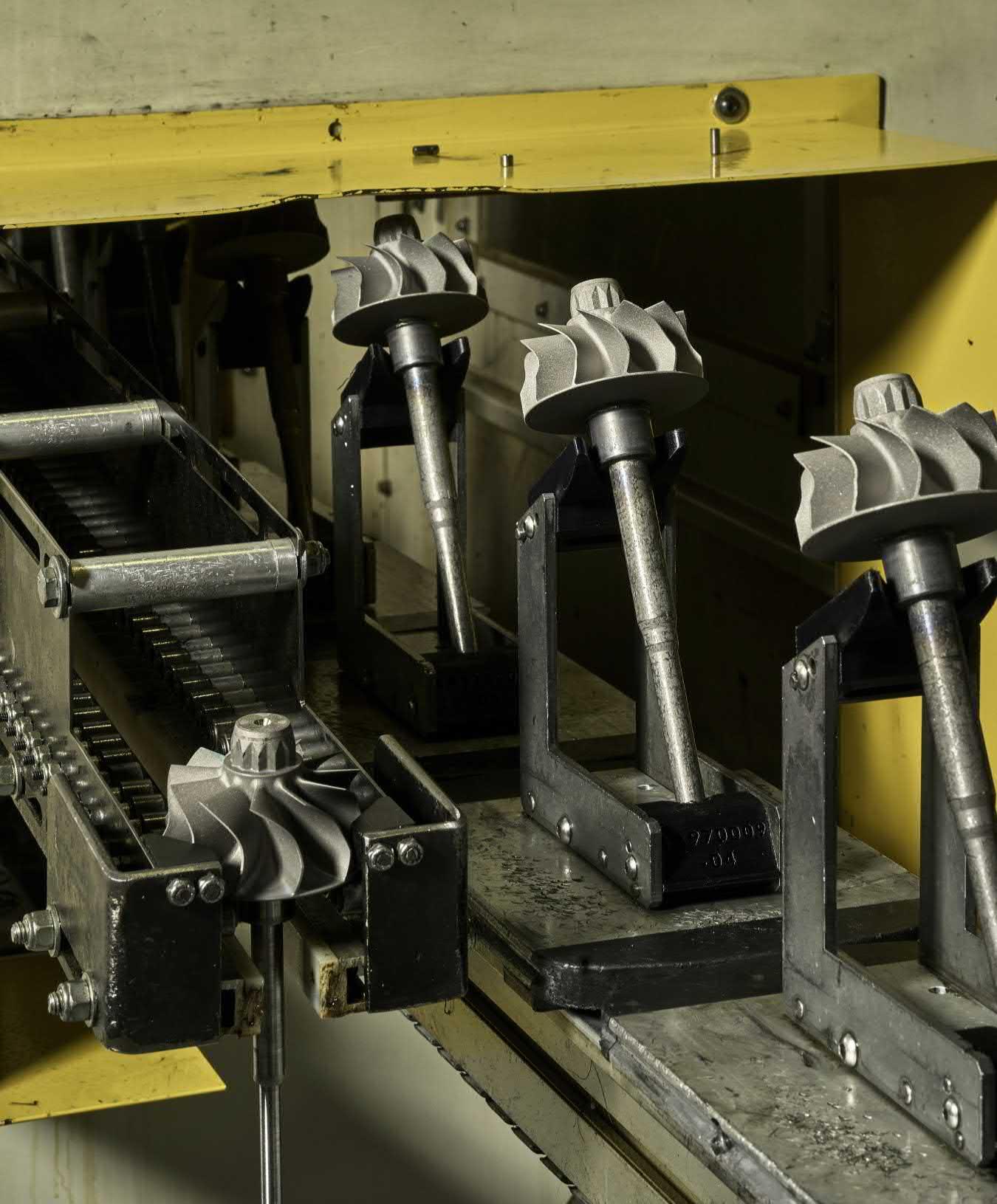
13 minute read
The Taming of the Snail
SEPTEMBER 1918, ATOP PIKES PEAK IN COLORADO, A MONSTROUS
27-liter Liberty V-12 military aircraft engine mounted in the back of a Packard truck came alive on boost. American engineer Sanford Moss had fitted the engine with a turbine-driven supercharger, or “turbocharger, ” in a bid to ram more air and fuel into it, generate more power, and blunt the effect of thin air at high altitude. It worked. The breakthrough arrived too late for use in World War I, but B-17 Flying Fortresses clouding the skies above Europe in World War II had turbochargers fitted to their radial engines, thundering power taking the fight to the enemy.
Advertisement
Early automotive efforts were similarly warlike. The first turbocharged entrant arrived at the Indy 500 in 1952, and by the late Sixties, the turbo Offenhauser engine began its dominance. In Can-Am racing, the Porsche 917/30 was so powerful and dangerous that it killed the entire series.
But in road cars, mass adoption of turbochargers was perpetually just around the corner. While they were ubiquitous on roadgoing diesel engines for decades, it wasn’t so long ago that turbochargers’ widespread deployment on gasoline engines seemed ever out of reach. The snail’s behavior was too violent for civilian life.
Today the corner has been rounded and is receding quickly in the rearview mirror. Among every major automaker, turbocharged gasoline engines have become the norm rather than the exception. Companies renowned for high-revving naturally aspirated gems, such as Honda and Ferrari, are increasingly transitioning their fleets to turbo power. Other automakers like BMW now offer nothing but turbocharged mills.
Surely, industrywide adoption of turbochargers on gasoline engines became inevitable because turbos simply got better, right? While the strides made in turbocharger control and efficiency over the years are undeniable, today’s highly accomplished turbocharged gasoline engines emerged largely from a confluence of hard-won incremental gains in engine development and the pressure of legislation. The breakthrough of turbochargers into the mainstream occurred less because turbos got better at making boost and more because engines got better at handling it.
Historically, turbocharged gasoline engines traded heavily on their ability to provide outstanding performance. Yet, thanks to their ability to kick pumping losses to the curb, they’ve always had the potential to deliver a combination of performance and efficiency their naturally aspirated brethren only dream of.
Instrumental in cultivating this potential was mitigating the effects of knock, an unsavory type of combustion that can destroy an engine’s internal components. Unimaginative engineers describe the deleterious limit imposed by knock as “the knock limit. ” They should have called it “the destroyer of worlds” to articulate its impact more clearly. Knock can dole out harshness to all spark-ignition engines, but for turbocharged ones, the knock limit looms like the Grim Reaper. Compared with naturally aspirated engines, boosted engines develop significantly higher cylinder pressures and temperatures. That’s like blood in the water to knock, as its likelihood— and severity—is linked to precisely those factors.
Normally aspirated engines can generally run at optimum ignition timing without the knock limit getting in the way. Think of the knock limit as the Armco barrier that lines a Formula 1 track. For nonboosted engines, the Armco sits outside the runoff area. Its presence doesn’t influence the racing itself, so you can potentially use the whole track with abandon.
For a turbocharged engine running at high load on pump gas, the knock limit nearly always
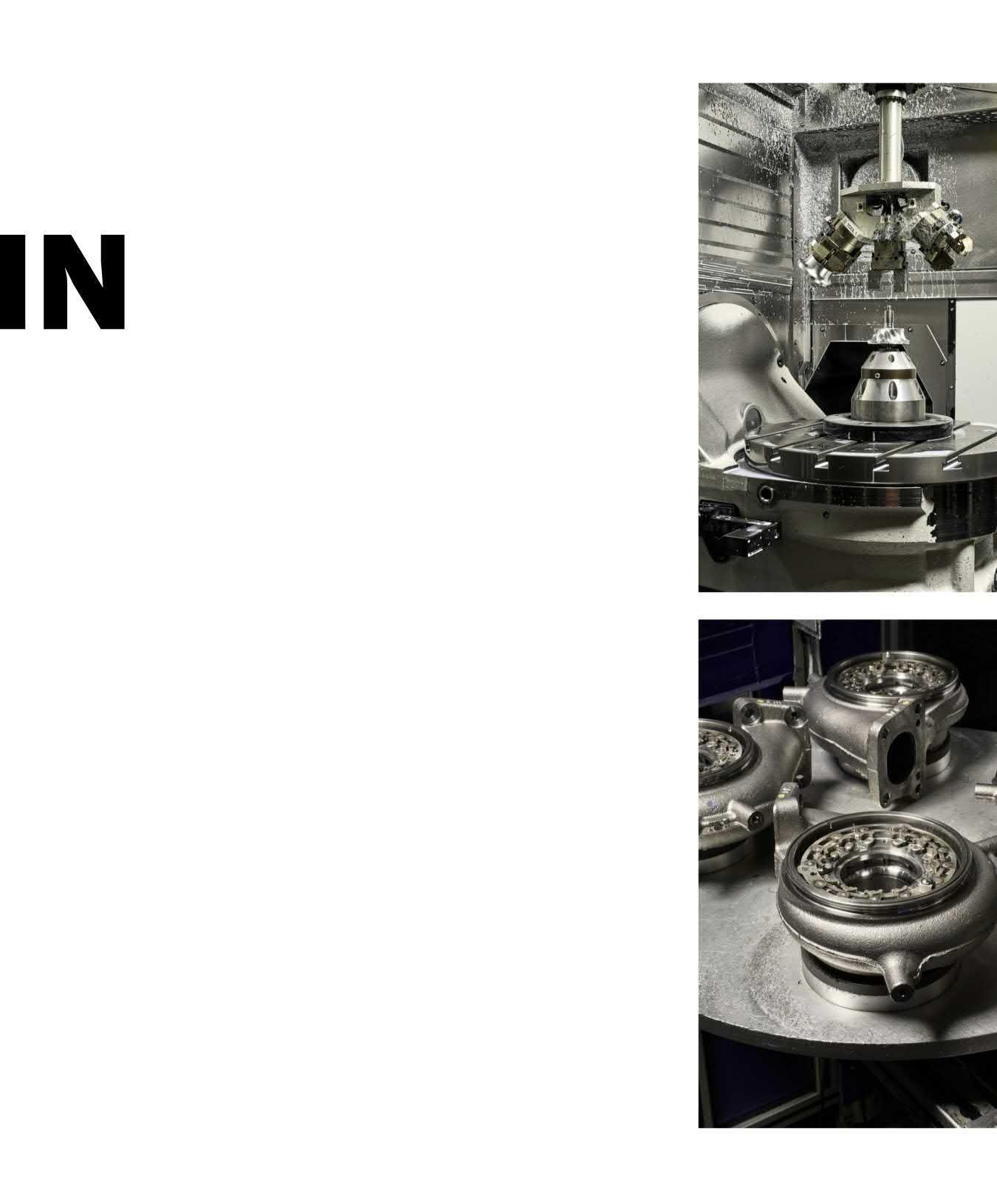
A. The life of a turbo is an extremely hot and frenetic one, so it must be built with absolute precision. B. Lightweight and endowed with a load of turbocharger tech,
Jim’s cart was still not particularly quick. C. Turbine housing assemblies with
Variable Turbine
Geometry (VTG) vane packs installed.


precludes the ignition timing that would produce best results. Accordingly, a turbocharged engine must operate as close to the knock limit as possible without overstepping. Here, our metaphorical Armco barrier sits flush with the track surface. There is zero runoff. To be fast through a chicane, you must drive as close to the barrier as humanly possible. But the penalty for getting even slightly too rowdy with the right pedal at the wrong moment is the Armco wreaking absolute havoc on your day.
It’s a tricky balancing act. Primitive turbo engines had no means to identify or address a knocking condition in real time. In extremis, these engines blithely ran past the limit and smashed themselves into an engine soup of melted pistons, eroded cylinder heads, and pummeled bearings.
In racing applications, you could just throw high-octane fuel at the problem. Early production engineers didn’t have that luxury, leaving avoidance as the only tool in their box. They’d dump the engine’s compression ratio into the basement, run it rich, set the full-load ignition timing a comfortable margin away from the knock limit, and hope for the best. Porsche stuck with this approach on the mighty 911 Turbo for a while, starting with the first one in 1974 up through 1990.
These workarounds kneecapped efficiency and contributed to turbo engines’ characteristic sog-then-surge power delivery. The first mainstream-production turbo gasoline engine, Oldsmobile’s 1962 Turbo-Rocket V-8, kept the compression high and employed a water/methanol (Turbo Rocket Fluid) injection system to enhance octane rating and cool the hot intake air. It was complex and expensive. Worse—at least for owners unfamiliar with turbo technology—was the need to top off fluid reservoirs consistently lest the engine melt down. Despite the shortcomings of early turbocharged efforts, their rush of boost was addictive. Better solutions to addressing knock would change the game.
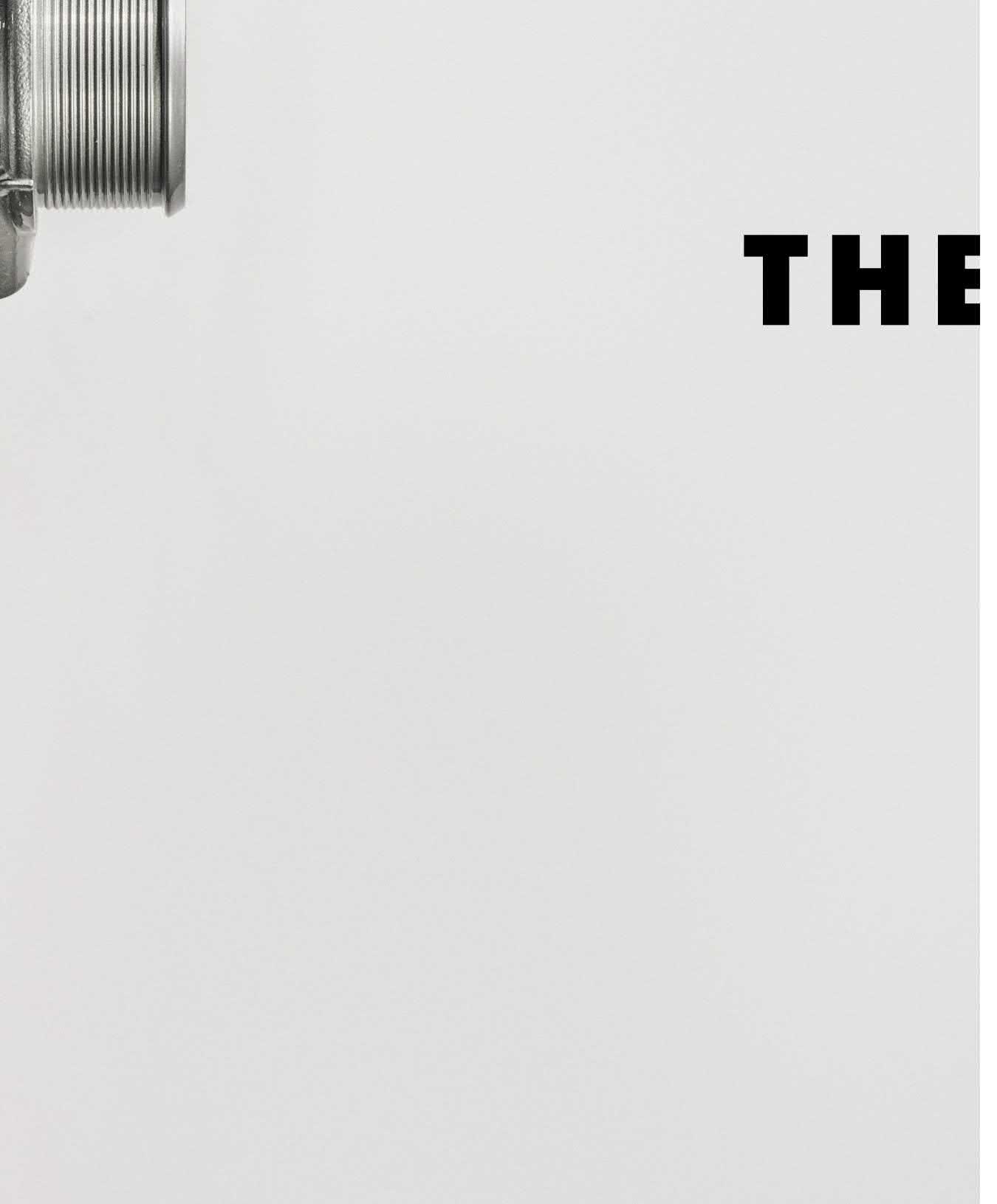
A. As with the internalcombustion engine, the fundamentals of the turbocharger haven’t changed in a century. MIGRATION TO ELECTRONICALLY CONTROLLED IGNITIONS WAS THE
breakthrough that allowed knock-detection systems to flourish. Initially, a block-mounted microphone was tuned to resonate at the frequency a knocking engine produces. When the microphone sang, electronic controls jumped in to retard the ignition timing to quell the knock. These systems prevented prolonged knocking, which saved a lot of engines from a melty death. But they left performance on the table—early controls weren’t smart enough to know which cylinder was knocking, so the timing was dialed out for all cylinders. It was like benching a baseball team’s entire infield because the shortstop took a line drive to the crotch. These systems also couldn’t discern knock noise from unrelated engine-borne noises of similar frequency and intervened on false alarms like valvetrain noise.
Buoyed by cheap gas and adequate engine controls, turbocharged gasoline engines saw a flurry of applications in the Eighties. These were generally found on range-topping models, owing to the extra cost of the turbo and its associated bits and the fact that buyers will pay more for additional performance. It would still be years before turbos were truly common.
Over time, the sophistication of engine-control systems grew. By the Nineties, fueling and ignition timing adjustments could be performed independently for each cylinder, a boon for managing knock without unduly hampering performance. Short- and long-term correction algorithms allowed knock-control systems to learn and adapt to ambient conditions, fuel quality, and engine health. Digital signal processing filtered out stray engine noise, even ignoring the knock sensors during the portions of each piston’s travel where knock never occurred, like during the intake and exhaust strokes. This eliminated those false triggering events.
OF THESE ENGINE-CONTROL SYSTEMS WERE GREAT FOR DETECTING
and managing an already-knocking engine. But why not just make the engine less likely to knock in the first place? As engineers sought to extract higher efficiency from engines to meet rising CAFE standards, they focused on increasing the combustion chambers’ burn rate. It turns out that not only are fast-burn chambers inherently more efficient, but they’re also more knock-resistant.
When a cylinder fires, a globe of fire is initiated by the spark plug. The heat from this fireball pours into the cooler, unburned mixture in its path. Speeding up the fireball’s burn rate reduces the exposure time of the unburned mixture to the heat advancing toward it, reducing its propensity to knock. It’s the difference between resting your palm over an open flame versus quickly passing your hand through it.
One of the most effective ways to create a fastburn chamber is to ensure a cylinder’s contents (its charge) are churning vigorously at the time of ignition—more activity, faster burn. You can probably already guess that it’s not easy to generate sufficiently intense charge motion without incurring a bunch of other compromises.
For example, small intake ports generate high air velocities at low engine speeds, accelerating combustion. The trade-off is that they suck wind as engine speeds climb, choking airflow and limiting performance and efficiency. Or consider elaborately contoured piston crowns with protuberances designed to deflect the incoming intake air deliberately. The additional surface area of all of that piston topology draws energy out of the burning gases that would otherwise provide useful work.
Simulation tools progressively advanced the state of the art in sorting out all of these compromises for engines and turbochargers. Empirical, trial-and-error approaches steadily made way for thermal and fluid modeling software.
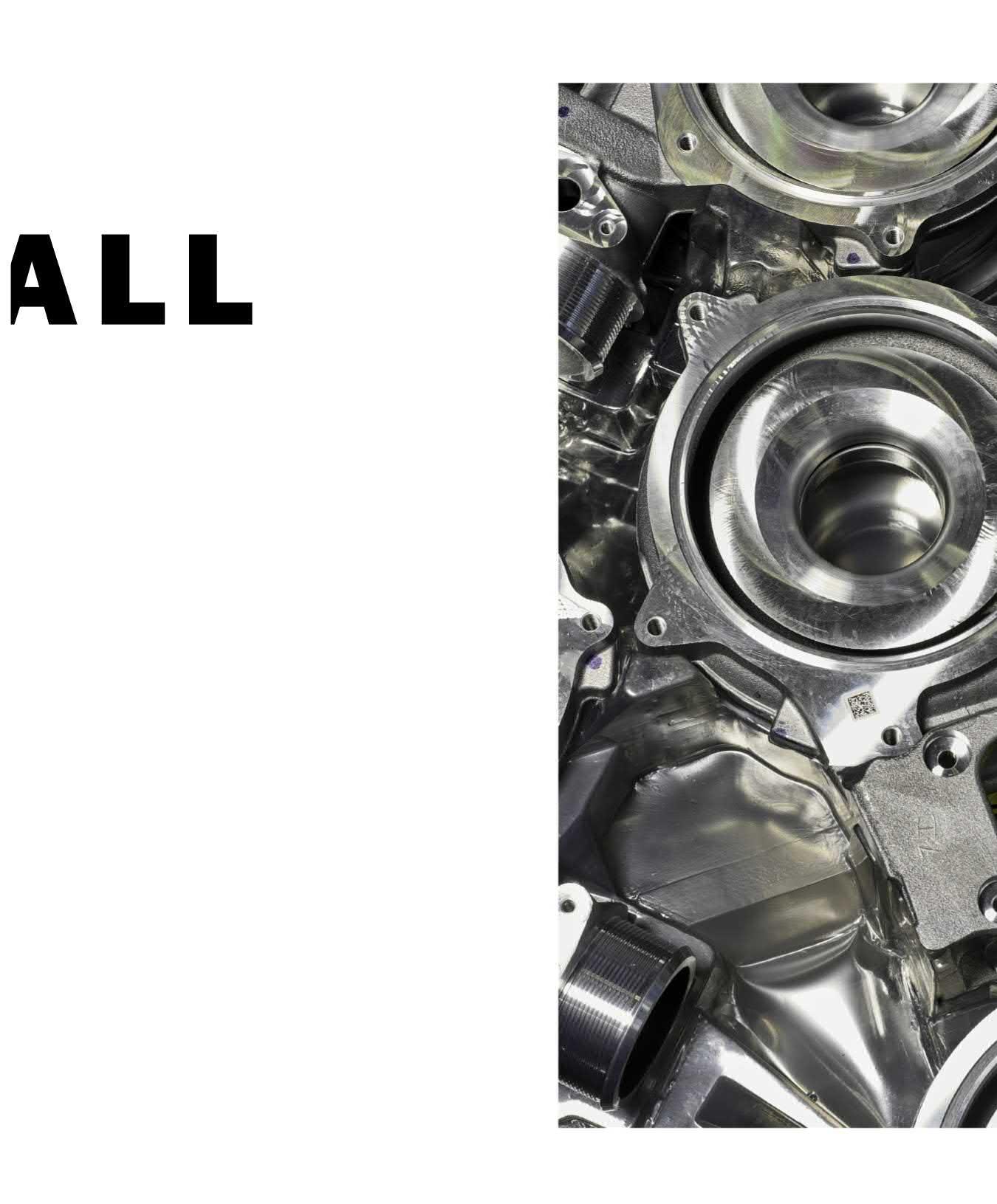
A. A mess of compressor housings staged for final assembly. B. Racks of turbine shaft and wheel assemblies in front of a grinding machine.
And that’s Zema, not
Zima, okay? C. BorgWarner’s facility in Arden, North
Carolina, is home to turbocharger manufacturing as well as
R&D.
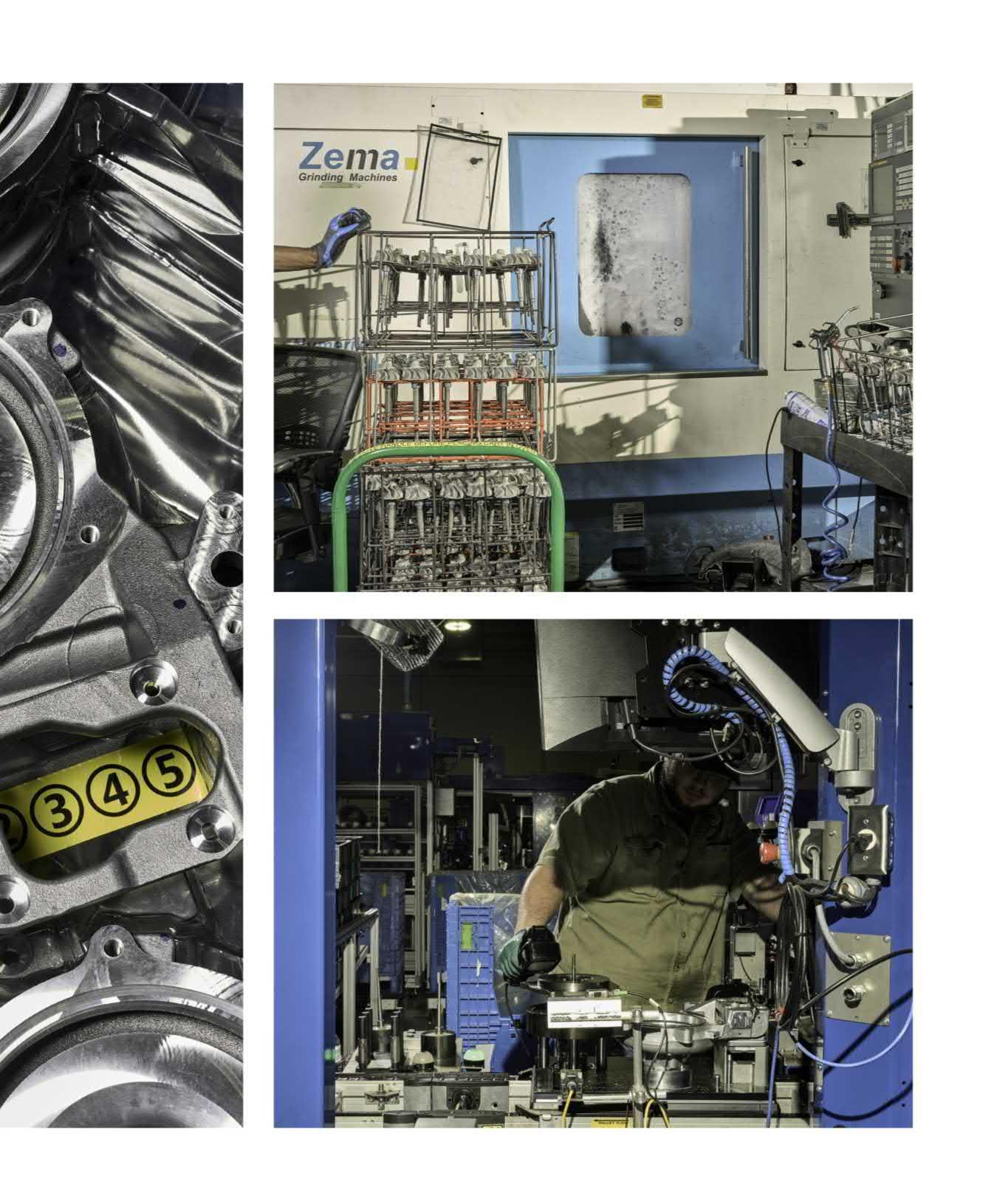
T H E S E A D V A N C E M E N T S I N J E C T I O N T O B E C O M E
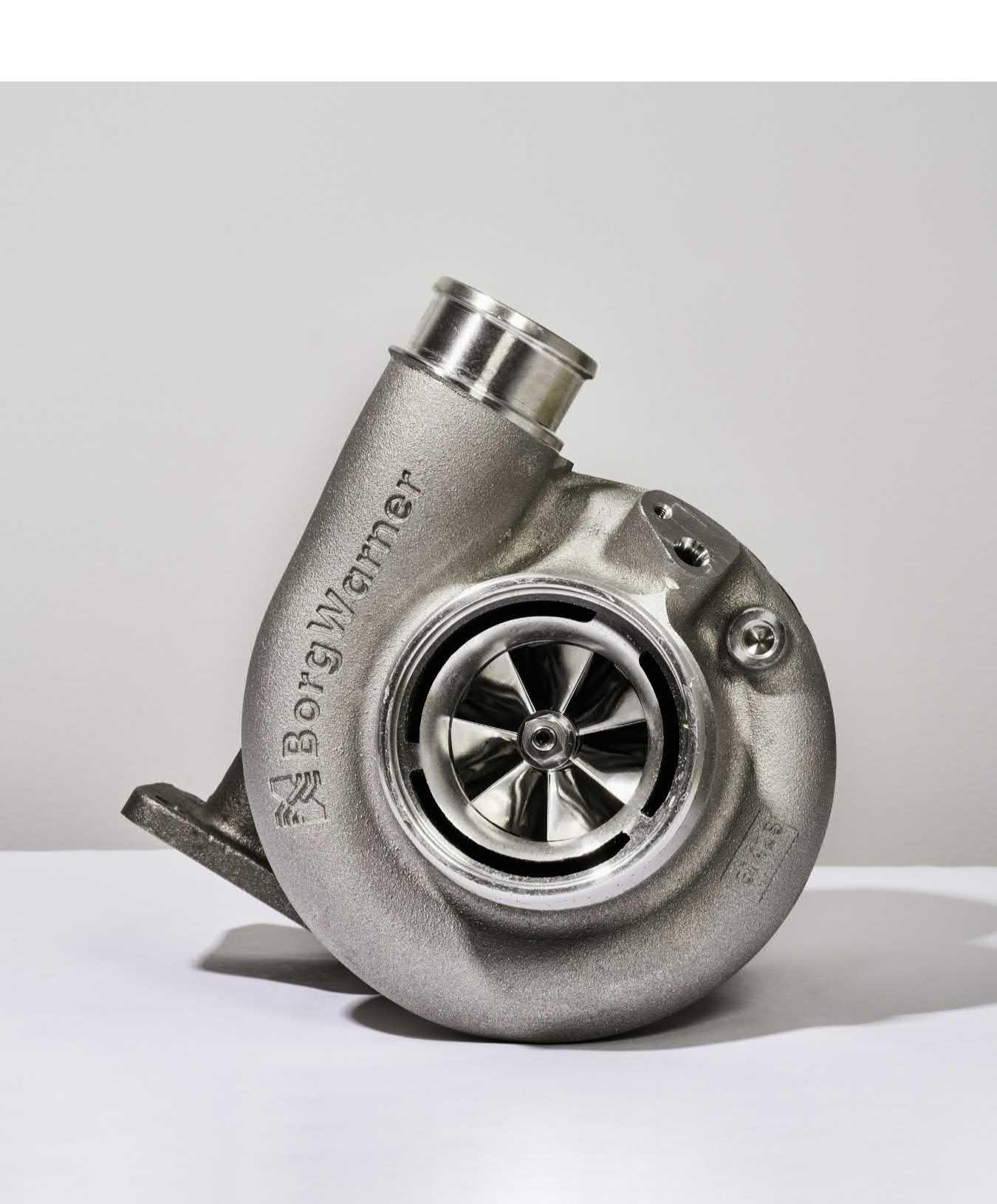
A L L O W E D D I R E C T A R E A L I T Y.

The low computational power available in the early days limited the models’ complexity. Today the ability to numerically assess countless combustion-system configurations—port and chamber geometries, valve events, injectors, piston shapes, and manifolds—with a high degree of fidelity brings high-accuracy modeling to bear on modern powertrain development.
These advancements also allowed direct injection (DI) to become a reality. A long sought-after goal of powertrain engineers, electronically controlled DI arrived stateside in some 2006 Toyota and Audi engines. DI facilitated turbocharging in a big way. The pronounced charge-cooling effect achieved by moving the injector into the combustion chamber reaped benefits in knock resistance, which led to higher compression ratios than were possible with port fuel injection. Off-boost efficiency and drivability also improved with the introduction of DI engines, and their higher volumetric efficiency provided more freedom in turbo sizing.
Yet another front in the war on knock involved managing the bill a turbo hands you for its services. Unlike a supercharger, which saps power directly from the crankshaft in exchange for its air-boosting capability, a turbocharger exacts a backpressure penalty. That is, the pressure in a turbo engine’s exhaust manifold is considerably higher than that of a supercharged or naturally aspirated engine, thanks to the influence of the turbine in the stream of exhaust gases. Among production turbo engines, it’s not uncommon to see exhaust manifold pressure twice that or more of the intake manifold pressure.
No good comes from this high exhaust manifold pressure. Not only does it adversely affect engine breathing for cylinders during their valve overlap period (when both intake and exhaust valves are open simultaneously, albeit briefly), but it also contaminates the cylinder with a higher proportion of hot exhaust gas once the valves close. This extra bit of heat erodes knock resistance.
Variable valvetrains helped counter this effect by allowing the manipulation of valve overlap on the fly or switching to different cam profiles altogether. These systems aided in reducing engine sensitivity to backpressure but did little to reduce the backpressure itself. Simply fitting a larger, laggier turbo would reduce backpressure but alienate car buyers. Instead, turbochargers themselves incrementally became more efficient over the years and packed more airflow-handling potential into ever-smaller packages. These tidier turbos also exhibited less inertia, imbuing them with quicker reflexes when the driver modulates the throttle.
The strides made in knock resistance alleviated but did not eliminate the need for fuel enrichment. This technique of hurling excess fuel into the combustion chambers as a cooling measure is certainly effective in abating knock, but it does no favors for fuel economy. Dialing back the enrichment, though, causes exhaust-gas temperatures to increase during high-load operation, battering exhaust valves, seats, manifolds, and the turbo’s turbine housing and wheel.
Ensuring the long-term durability of these components led to the development of industry-specific high-nickel alloys and austenitic stainless steels tolerant of ever-higher temperatures. These materials allowed boost to coexist with relatively lean operation in a way not previously possible.
Predictably, these metals aren’t cheap. As a result, some manufacturers instead made the calculated choice to continue throwing fuel down the exhaust to keep things cool during mediumand high-load operation because it allowed them to use less costly components. The fuel-economy penalty of this approach sometimes did not show up on the window sticker because the EPA drive cycle consists primarily of lighter loads. In realworld use, these engines often did not live up to the window-sticker promises, and turbocharged gasoline engines suffered reputational damage in the process.
All the while, emissions certification posed yet another hurdle to the viability of turbocharged
A. The BorgWarner
AirWerks S200 SX-E turbocharger is capable of supporting up to 650 hp.
gasoline engines in production cars. Thanks to the astonishing effectiveness of warmed-up exhaust catalysts, cold starts are responsible for a significant part of an engine’s emissions. A catalytic converter below its light-off temperature is little more than an expensive and ineffective muffler. During a cold start, any massive objects in the exhaust stream act like sponges for precious heat that would otherwise help light off the exhaust catalyst sitting downstream. The turbine housings and heavy cast exhaust manifolds made it increasingly difficult to coerce traditional turbo engines into compliance with ever-tightening emissions standards.
Lighter air-gap insulated exhaust manifolds were an outgrowth of the progress in materials research. The hefty turbine housings would have to stay, as they also function as containment devices in the unlikely event the turbo’s spinny bits let go at the mind-bending speeds they routinely reach (200,000-plus rpm). A key tactic to speed light-off was simply opening the turbo’s wastegate during cold starts, diverting exhaust gases around the turbine. However, traditional wastegate actuators worked via boost pressure, which, unless something has gone horribly wrong, is not available at idle. Vacuum-based actuators provided an elegant way to speed lightoff. Later, electronically actuated wastegates provided even greater control.
Other factors played supporting roles. Watercooled turbos eliminated oil-coking concerns. Better management of oil carryover into the intake system reduced the amount of octaneannihilating oil that formerly made its way into the combustion chambers. Piston ring sealing improved. No areas of engine development went unexamined in the pursuit of higher efficiency, better drivability, and increased reliability.
As we look toward the future of internalcombustion engines for production cars, it’s possible we’ve reached the apogee. Research and development budgets are not limitless, and automakers’ investments are being diverted into battery-electric drivetrains. We’ll shortly see a few more advances in turbocharged gasoline engines, electric-assist turbos being the most significant, but it’s clear the sun is setting on the snail. Enjoy the boost while you can.
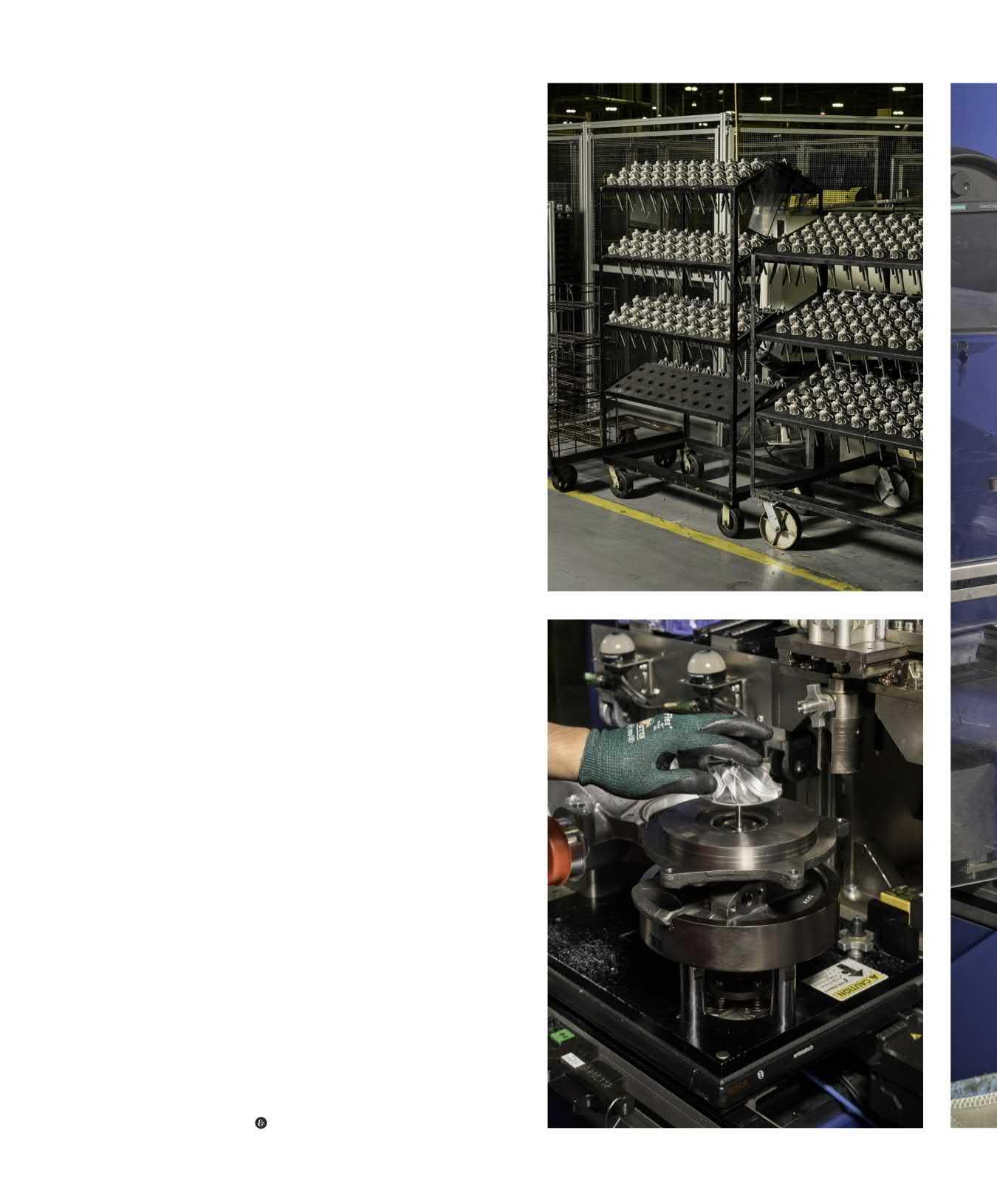
A. That’s a lot of wheels. According to the EPA, the percentage of light-duty vehicles with turbos rose from one percent in 2000 to 34 percent in 2019. B. Even after all of these years, there is something very elegant about producing more power using waste air. C. With increasingly stringent standards for efficiency and emissions, we might be nearing peak internal-combustion engine complexity. B
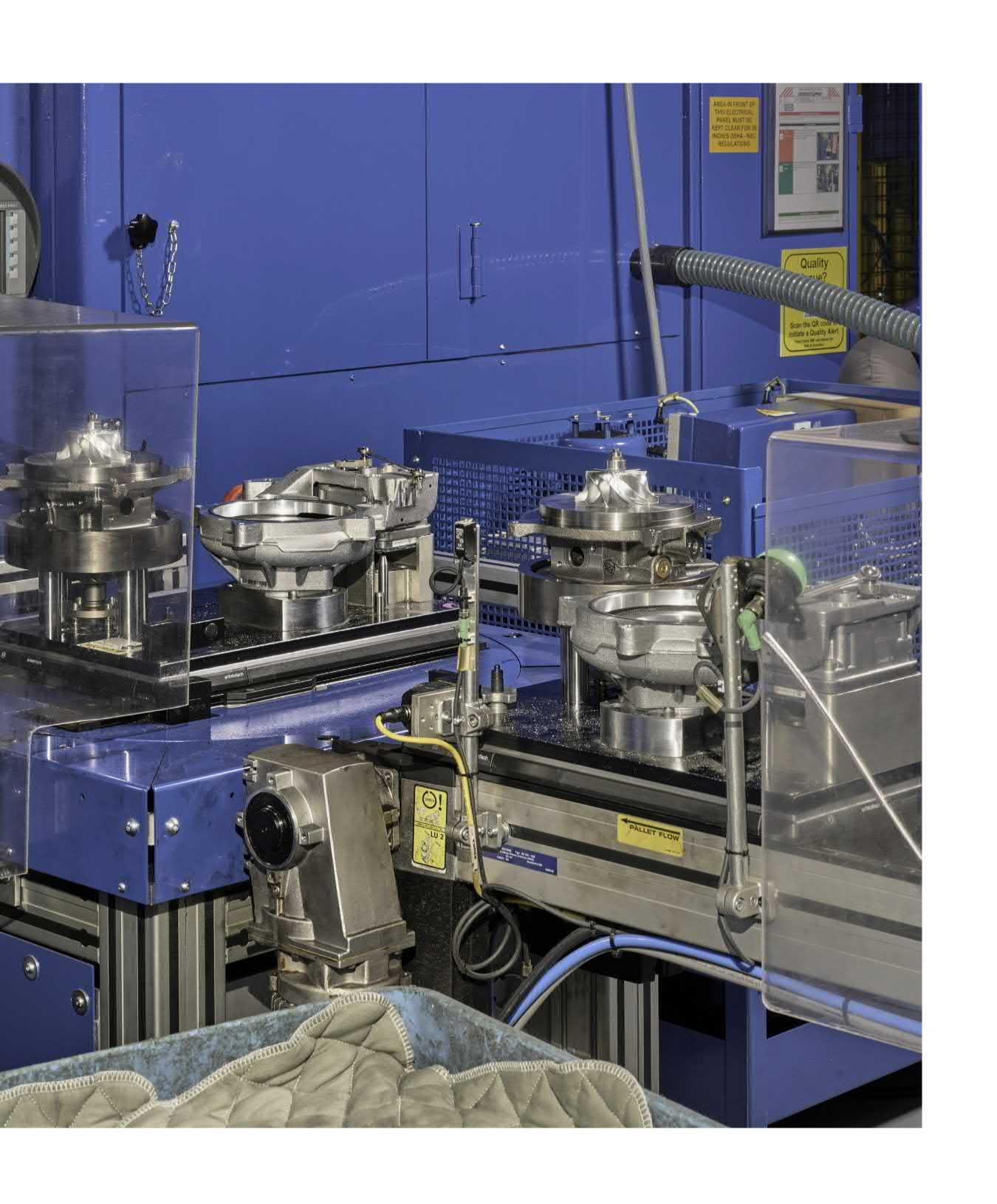