
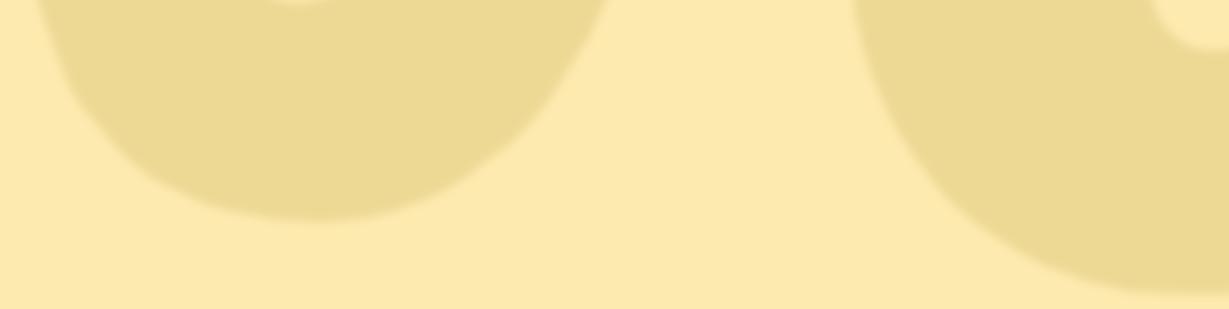
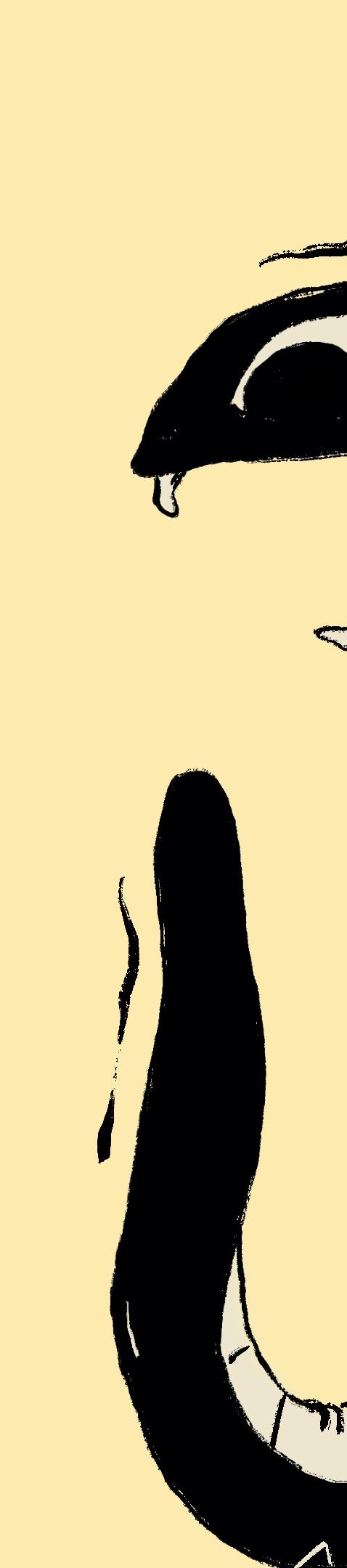
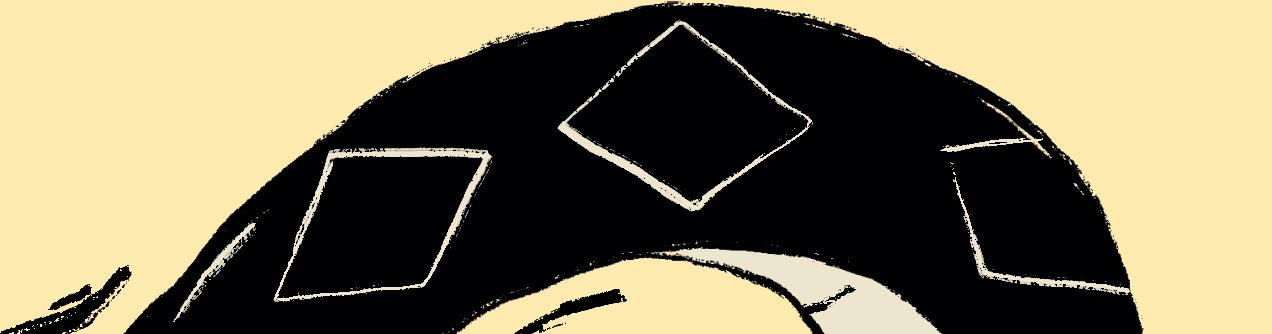
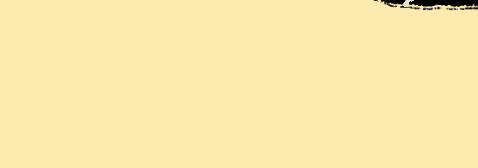
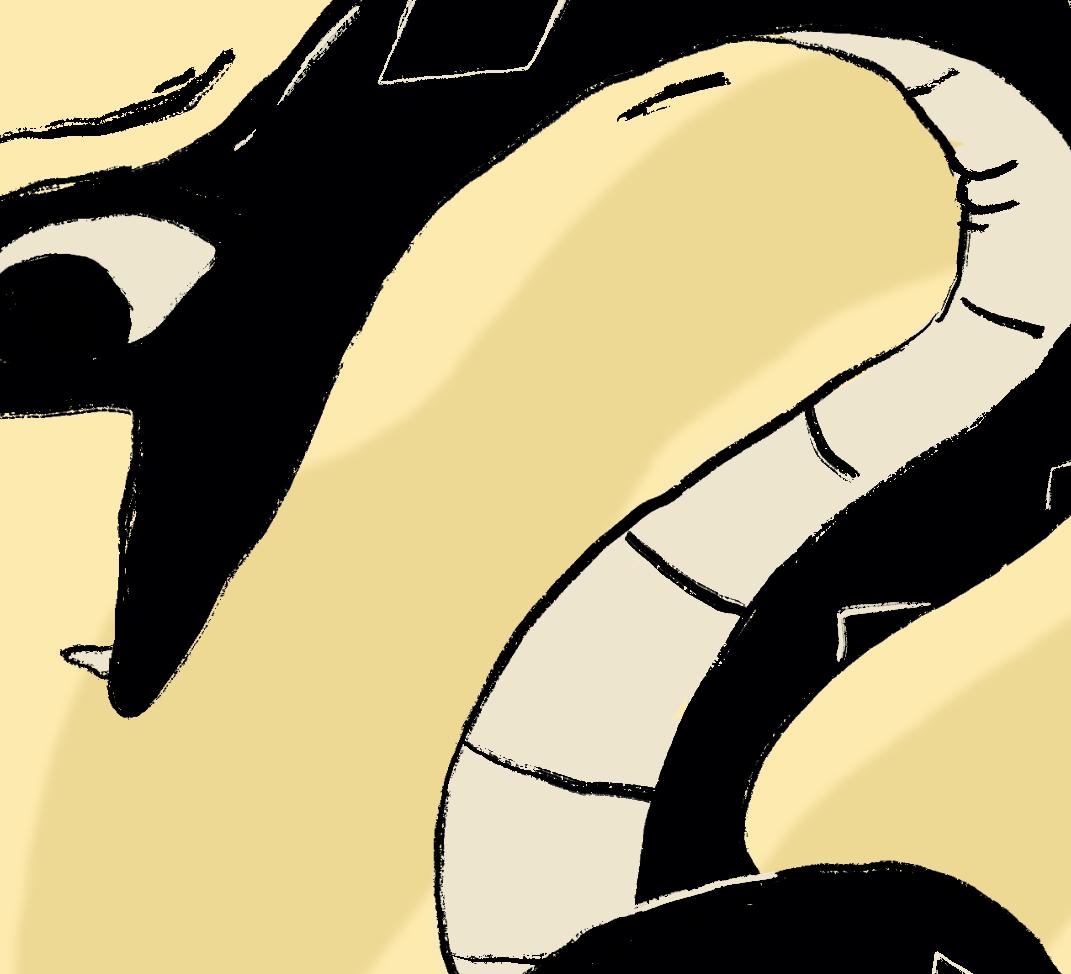

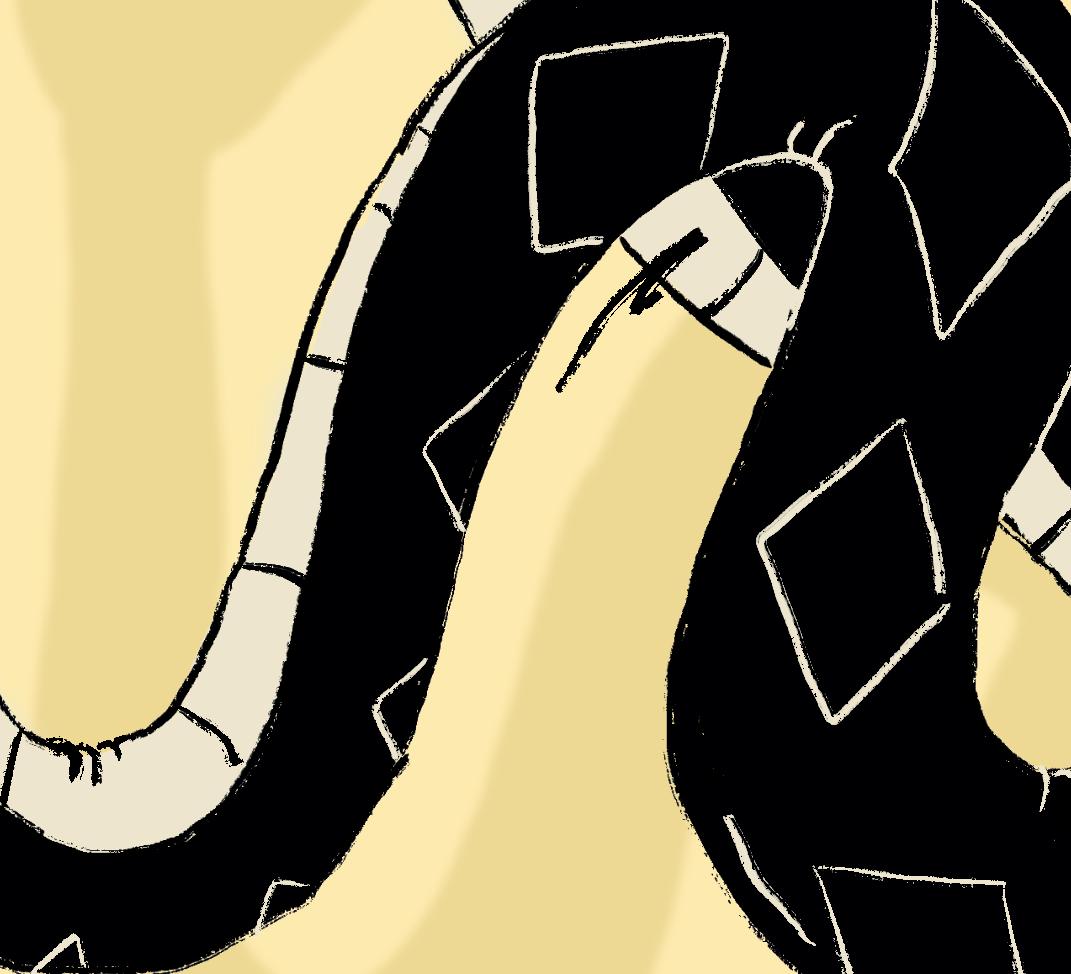

Van selectieve natriumkanaalblokkers naar het repareren van DNA; nieuwe mogelijke behandelingen voor epileptische encefalopathie
Wouter Jan Weuring geboren op 22 november 1989 te Apeldoorn
PhD thesis, Utrecht University - with a summary in Dutch © W.J. Weuring, Utrecht 2022
All rights reserved. No part of this book may be reproduced or transmitted, in any form or by any means, without written permission of the author. ISBN: 978-94-6458-629-9
Lay-out and cover: Lonneke Weuring | www.lonnekeweuring.nl
English language editing: Ian MacMenamin Printing: Ridderprint | www.ridderprint.nl
Dit proefschrift werd mede mogelijk gemaakt met financiële steun van het MING fonds, Vrienden UMC Utrecht & Wilhelmina Kinderziekenhuis: 1616091
Van selectieve natriumkanaalblokkers naar het repareren van DNA; nieuwe mogelijke behandelingen voor epileptische encefalopathie (met een samenvatting in het Nederlands)
ter verkrijging van de graad van doctor aan de Universiteit Utrecht op gezag van de rector magnificus, prof.dr. H.R.B.M. Kummeling, ingevolge het besluit van het college voor promoties in het openbaar te verdedigen op maandag 31 oktober 2022 des middags te 4:15 uur door
Wouter Jan Weuring geboren op 22 november 1989 te Apeldoorn
Prof. dr. K.P.J. Braun
Copromotor: Dr. B.P.C. Koeleman
NaV1.1 and NaV1.6 Selective Compounds Reduce the Behavior Phenotype and Epileptiform Activity in a Novel Zebrafish Model for Dravet Syndrome
CRISPRa-Mediated Upregulation of scn1Laa During Early Development Causes Epileptiform Activity and dCas9Associated Toxicity
Genetic and Functional Differences of Zebrafish Duplicant Genes for Human SCN1A
Part II - Gene Regulation and Editing as a Permanent Cure for Genetic Epilepsy
Influence of Common SCN1A Promoter Variants on the Severity of SCN1A-related Phenotypes
Gene Expression Modulation of DEE Genes Using Short Doublestranded RNAs
Prime Editing for Developmental Epileptic Encephalopathies
9
General Discussion
English Summary
Nederlandse samenvatting
List of Publications
Dankwoord
Curriculum Vitae Design Explanation
Any brain can develop seizures, which are generated when neuronal excitability reaches a certain threshold. While a sporadic seizure can be triggered by sleep deprivation or drug abuse, an underlying brain disorder can lead to a reduction of the intrinsic seizure threshold increasing the propensity for spontaneous recurrent seizures. For this reason, the International League Against Epilepsy (ILEA) established the following definitions to differentiate between seizures and epilepsy:
1. When at least two unprovoked seizures occur more than 24 hours apart
2. One unprovoked or reflex seizure and a probability of further seizures similar to the general recurrence risk of at least 60% after two unprovoked seizures, occurring over the next 10 years
3. Diagnosis of an epilepsy syndrome
Around 50 million people worldwide have epilepsy, making it the most common chronic neurological disease [1]. The five major aetiologies for epilepsy are: structural (e.g. tumor, stroke, malformation of cortical development), infectious (e.g. tuberculosis), immune (e.g. autoimmune encephalitis), metabolic (e.g. inborn errors of metabolism) and genetic (e.g. chromosomal abnormalities or single-gene mutations). Four of these make up approximately 25% of total epilepsy cases, according to the 1975-2014 epilepsy paradigm (Figure 1) [2]
Figure 1 The 1975-2014 Epilepsy Paradigm [2]. Before advanced MRI technologies and DNA sequencing was introduced for clinical diagnostics, the majority of epilepsy cases in 1975 was labeled idiopathic. In 2014, the majority of idiopathic epilepsy was expected to be genetic, although the exact cause often remained unknown. Figure adopted from [2]. In the past decade, GWAS studies helped identify common genetic risk factors.
The fifth, genetic, was previously labeled ‘idiopathic’, referring to a presumed genetic origin that could at the time not be identified in the absence of DNA sequencing technologies. In 2022, the main technology used to discover the genetic cause of epilepsy in a large number of patients is called genome-wide associated studies (GWAS). GWAS requires thousands of DNA samples from epilepsy patients and controls to identify susceptibility loci throughout the genome [3]. These loci, which are usually outside the protein-coding region of a gene, carry single nucleotide variants (SNVs) that can be significantly associated with the patient cohort using stringent statistical analysis. Individual SNVs, which are common in the healthy population and determine the genetic heterogeneity of a species, are usually not disease causing by itself. However, in combination with other SNVs they can generate a ‘polygenic burden’ which, although not yet fully understood, is the primary hypothesis for the cause of most epilepsy cases.
In contrast to ‘common epilepsies’ caused by a polygenic burden, monogenic epilepsy can be considered a simple disease from a genetic perspective, as it is caused by a single mutation in a single gene. Unlike common SNVs, which are present throughout the healthy population, a mutation in an ion channel gene can arise de novo during development and lead to protein dysfunction resulting in disease by itself. The phenotype of monogenic epilepsy is often much more severe
than common epilepsy.
Developmental and epileptic encephalopathy (DEE) is a rare neurological disorder characterized by epileptic seizures that contribute to severe cognitive and behavioural impairments, which can worsen over time. Typically, the age of onset is within the first year of life, after which the disease progresses into a syndrome that includes seizures which are difficult to treat, and particularly cognitive comorbidities and sudden unexpected death during epilepsy (SUDEP) [4]. DEE is defined according to the 2021 nomenclature from the ILAE as follows:
A heterogeneous group of rare neurodevelopmental disorders, characterized by (a) earlyonset seizures that are often intractable, (b) electroencephalographic abnormalities, (c) developmental delay or regression and in some cases, (d) early death (ILAE definition as of 2021).
Over 90 subtypes of DEE have been identified, each caused by mutations in a single gene. For example, DEE6, also called Dravet Syndrome and the prototype DEE, is caused by loss of function mutations in SCN1A. Approximately half of all DEE subtypes are caused by de novo mutations in an autosomal dominant manner, with the other half being recessive or X-linked (Figure 2).
Figure 2 Overview of DEE Genes. Genes adopted from OMIM were categorized in three heritability types; autosomal dominant, autosomal recessive, and X-linked. For each gene, protein location data from UniProt was used to determine its location of expression. It should be noted that UniProt data is usually based on adult human tissue and the category ‘Other/non-CNS specified’ could be expanded to precursor CNS tissue during early development.
DEE-related gene mutations can lead to either gain or loss of function of the corresponding protein. For example, Nav1.6 (SCN8A, DEE13) or Nav1.2 (SCN2A, DEE11) are the main voltage gated sodium channel (VGSC) subtypes on excitatory pyramidal neurons during early development, and gain of function (GOF) mutations in the genes coding for these proteins cause DEE in children as they lead to increased excitatory signaling. In contrast, mutations in SCN1A, the alpha subunit of Nav1.1 which localizes to inhibitory GABAergic neurons, generally cause loss of protein function (LOF) and lead to reduced inhibitory neurotransmission, resulting in increased excitation (the cause of DEE6). This disease mechanism is reflected in anti-seizure drug (ASD) response. The number of seizures can be reduced in patients with DEE13 using ion channel blockers, such as phenytoin, whereas there is no response, or even seizure aggravation, in patients with DEE6, as these drugs further inhibit the function of GABAergic neurons [5]. Chapter 2 of this thesis covers testing several novel channel subtype-selective blockers and activators for DEE6.
While many of the anti-seizure drugs usually work well for common epilepsy patients, seizures in DEE are often drug-resistant, also called ‘refractory’, and comorbidities are not treated, meaning that the developmental part of DEE continues to progress. Although even people suffering from common epilepsy have a three times higher risk of premature death, DEE-affected children can have a SUDEP risk as high as one in six [6]. Patients who survive DEE until adulthood are severely cognitively impaired and require anti-seizure drugs, with many ‘trial-and-error’ approaches throughout life due to drug resistance. Ideally, new medication directly targets the pathophysiological mechanism underlying the cause of the disease, rather than the symptom, which is one of the core aspects of precision medicine [7]. The monogenic cause of DEE and its severity make it an ideal disease for the development of novel targeted treatment approaches at the level of the dysfunctioning protein or even directly aimed at the DNA mutation itself. In the following paragraphs, I will briefly introduce targeted therapies aimed at protein, mRNA, and genomic DNA, which will be covered in more detail in the following chapters.
Typical anti-seizure medication consists of drugs that block the flow of sodium in neurons. However, the earliest VGSC blockers used for epilepsy patients showed little selectivity for VGSC channels in the brain. As there are eleven different VGSC subtypes expressed in humans, non-selective blockers can theoretically bind to any VGSC subtype, as long as the drug is given systemically, which is usually the case. This is reflected in the efficacy and the reported side effects of these drugs. Carbamazepine (CBZ), for example, which is a widely prescribed VGSC blocker throughout the world [8] binds with more affinity to Nav1.4 (skeletal muscle), Nav1.5 (heart tissue) and Nav1.7 (PNS, nociception), before the affinity levels are reached for CNS channels Nav1.1-1.3, and 1.6 [9]. Of the many side effects of CBZ, it is interesting
to note that loss of coordination, ataxia, numbness, pain, and cardiac side effects such as bradycardia and heart failure can be associated with off-target blocking by CBZ of Nav1.4, 1.7, and 1.5, respectively.
In some sporadic DEE cases, non-selective VGSC blockers are effective in reducing the amount of seizures. This is specifically based on the gene and mutation type. In one study, three out of four severely impaired patients with a GOF mutation in SCN8A treated with Phenytoin (PTH), a non-selective VGSC blocker just like CBZ, became seizure-free for several months. Side effects such as ataxia and cognitive dysfunction were considered mild, given the severe seizure phenotype before PHT was administered [5]. This exemplary case report highlights that the seizure-aspect of SCN8A GOF mutations can currently be treated, but DEE being a developmental disorder continues and comorbidities remain.
Within the CNS there are five different VGSC subtypes expressed, each with a different population of cells and regions in the brain. Blocking of VGSC channels that are expressed on GABAergic interneurons is generally undesirable and can perhaps be prevented. By further increasing the selectivity of VGSC blockers, it may be possible to fine-tune medication based on the proteins that are affected in a patient. Medication aimed at this protein will only affect a subset of neurons, while leaving the rest of the brain unharmed. Chapter 2 covers the design and synthesis of several selective VGSC blockers and activators and describes the testing of these novel compounds in a zebrafish model for genetic epilepsy.
The central dogma of molecular biology teaches us that DNA is transcribed into messenger RNA, from where it is translated into a protein. Given that the cause of a genetic disease is present in the DNA, the DNA mutation, it makes sense to also start focusing the treatment on the cause. Currently, many of the novel targeted treatment approaches for DEE, among other genetic diseases, are still aimed at the protein. A well-known example, other than the use of sodium blockers in sodium channelopathies as described above, is quinidine, which blocks potassium channels in GOF KCNT1 mutation syndrome, also called DEE14 [10]. While these treatment approaches can be considered ‘precision medicine’, they still target two levels away from the primary cause of the disorder (Figure 3).
better serve as a tool for fundamental research. The technological aspects and clinical translation of RNA therapies, including CRISPRi/a among other transient gene therapies, are further covered in Chapter 6. Chapter 3 covers a more dedicated insight into CRISPRa specifically applied to rescue the DEE6 (SCN1A) phenotype using our DS zebrafish model. RNA therapeutics based on siRNA and saRNA are briefly covered in Chapter 7, with the identification of novel saRNA hits for DEE26 (KCNB1), DEE94 (CHD2), DEE11 (SCN2A), and DEE62 (SCN3A).
The final level of treatment that truly focuses on the core of genetic disease, according to Figure 3, is gene editing. Since 2019-2021 is marked by the discovery of novel gene editing systems, based on and adapted from the original CRISPR/ Cas9 system developed in 2013, the following paragraph is dedicated to current gene edit systems, as well as their status and application in clinical practice.
In the layman’s press, gene editing is often depicted as in Figure 4A. Despite the forceps and extraction of one DNA base may help the wide public understand the concept of ‘removal of disease-causing mutations’, this is a major oversimplification and does not represent the abilities of current gene edit technologies.
Figure 4 Gene Editing in 2022. A) Popular representation showing removal of a single DNA base (red) which represents a DNA mutation, by using a forceps from the genomic DNA. B) Mechanistic representation of the original CRISPR/Cas9 system from 2013, used to introduce targeted disruptions in the genome. The original Cas9 protein is guided by a single guide RNA to the target location to introduce a double-stranded break. dsDNA breaks in the human genome repaired by the non-homologous end-joining pathway usually lead to random insertions or deletions at the cut-site, resulting in disruption of the genomic region. While this system cannot be used to repair a gene, a mutation can be ‘sliced-out’ if the surrounding sequence allows this.
C) Realistic mechanistic representation of the Prime edit technology (2019-2021), used to repair a mutation. (1) Binding and introduction of a single-stranded break at the mutation site (2) hybridization of the corrected strand and (3) reverse transcription to cover the mutation flanking regions (3). Even if the corrected base pair is inserted following steps 1-3, the edit can be undone via incorrect 5’ flap cleavage (4) or DNA mismatch repair (MMR) (5) pathways, which are currently not well understood in the context of PE. Novel PE systems (2021) carry an MMR inhibitor, that could further influence step 5. [Figure generated with Biorender based on preexisting templates].
Unlike CRISPRi/a, which allows gene enhancement without altering the genome, CRISPR/Cas9 is aimed at creating targeted disruptions with a permanent effect. CRISPR/Cas9 was initially identified as a bacterial adaptive immune response pathway after viral infection. Viral sequences are installed in the bacterial genome in a repeat fashion, which are recognized by CRISPR-associated (Cas) proteins. After a new infection, Cas proteins guided by the viral sequences recognized earlier, bind and catalyze the digestion of viral genetic material, thereby halting infection [14]. By extracting this system from bacteria and using synthetic gene-specific sequences as ‘bait’ for the Cas protein, this immune pathway was remodeled as a tool to enable targeted disruptions in the genome.
CRISPR/Cas9 works well when the aim is to create double-stranded breaks in the genome without having to focus on a correct repair (Figure 4B). This led to the first in-human clinical trials using CRISPR, which started in 2020 [15,16]. Diseases that can benefit from this type of ‘crude’ gene editing have the requirement that the introduced genomic disruption is tolerated by the genome. The diseasecausing mutations aimed for in the above clinical trials fit those requirements. EDIT101, given to patients with a genetic form of blindness called Leber congenital amaurosis-10, is aimed at an intronic mutation in retinal cells, which can be ‘sliced out’ without changing the open reading frame [17]. NTLA-2001 is used for patients with transthyretin amyloidosis and knocks out a gene responsible for protein misfolding [18]. In both examples, the aim is to disrupt a genomic region and not repair the DNA, but in both cases this approach is correctly aimed at the underlying disease mechanism.
Strictly speaking, creating double-stranded breaks to disrupt genomic regions can be called gene editing as long as a gene is targeted, and not introns or regulatory regions. But ‘CRISPR-knockout, or ‘CRISPR-based disruption’ would be a more suitable label to differentiate this approach from true gene editing, a term that is now preferably reserved for techniques that actually repair the DNA. Still, even without precisely repairing a DNA mutation with these early gene-editing drugs, CRISPR/Cas9 can be a life-changing improvement to current medication for these patients. With several treatments, the origin of disease can be removed, circumventing the need for re-dosing throughout life.
Gene editing with the aim of repairing the DNA, which would be applicable to the majority of disease-causing mutations in mankind, has only become possible with recent adaptations of CRISPR/Cas9. These adaptations now make a singlestranded break at the site of mutation and introduce the correctly edited nucleotide. Improved versions of CRISPR/Cas9 are published every other year with the discovery of Base editing (2017) [19], Prime editing (PE) (2019) [20], smaller Cas variants such as Casɸ (2020) [21], and PE coupled to inhibitors of the DNA mismatch-repair pathway just last year (2021) [22]. Comparing these improvements to a forceps plucking
out a mutation as depicted in Figure 4A is unfortunately still in vain. PE, which is currently the most effective gene editor (Figure 4C), is hampered by size and thus by delivery, correct hybridization of the edited strand, and mismatch repair. However, when compared to CRISPR/Cas9 in 2013, PE is at least ten times more effective and, more importantly, the amount of random insertions and deletions is nearly absent.
It took less than eight years from the discovery of CRISPR/Cas9 to the first in-human clinical trials, which is much faster than the average translation from bench-tobedside of novel drugs based on a completely novel technology, highlighting the potential of CRISPR/Cas9. As PE has not been applied to DEE genes to date, Chapter 8 covers this topic: the introduction and removal of DEE6 (SCN1A) and DEE7 (KCNQ2) mutations.
The research described in this thesis is aimed at developing new treatment options of monogenic DEE syndromes, using a broad spectrum of pre-clinical experimental approaches ranging from targeting the protein-level to novel geneediting methodologies. Part I focuses on VGSC-subtype-specific drug screenings and CRISPRa for DEE6, Dravet Syndrome, that act on the protein and mRNA levels in a zebrafish model. Part II covers gene regulation and gene editing efforts for a range of DEE subtypes and genes.
The studies described in Part I were inspired by the inefficacy of current voltagegated sodium channel (VGSC) blocking drugs in patients with DS. To test novel compounds, we generated a genetic DS zebrafish model for high-throughput screening in Chapter 2 and validated its pharmacological profile using known anti-seizure drugs. Based on the mutational background of DS, we tested subtype selective VGSC blockers, which have more affinity for excitatory channels, such as Nav1.6, as an improvement for current VGSC blockers that are hardly selective. In addition, we tested subtype selective VGSC activators that, given that DS is caused by LOF mutations in SCN1A, could prove beneficial. Since zebrafish carry duplicated gene copies for many mammalian genes, we were curious to see if CRISPRa could be used to enhance the expression of scn1Laa, to compensate for loss of scn1Lab in the DS model. In Chapter 3, we show that CRISPRa can be used to upregulate VGSC genes in vivo, but is accompanied by toxicity and development of an epileptiform phenotype in wildtype animals. During these projects, we discovered fundamental differences in duplicated gene functioning and we further dived into the limitations of DS zebrafish via genetic and functional analyses in Chapter 4
The goal of Part II of this thesis was to find out more about the regulatory pathways of DEE genes and whether their expression can be altered by novel RNA therapeutics
based on siRNA and saRNA. In addition, we show data from the first DEE Prime Edit experiments as a means to treat DEE at the origin of disease: the DNA mutation. Chapter 5 lays the foundation for gene-regulation based therapies. By analyzing the promoter region of 95 DS patients, we discovered differences in common SNVs that make up variation in the human genome. SNVs in the SCN1A promoter were shown to alter expression of a reporter gene indicating that disease-severity could be linked to common variations in the genome. To find out if DEE gene expression can be tuned at the promoter or transcript level we used saRNAs and siRNAs on several DEE genes in Chapter 6 . Small RNAs show different effects when guided to distal or proximal promoter regions and can be used to upregulate or downregulate gene expression via promoter-based gene regulation. Chapter 7 reviews current and novel gene therapies, including all those covered in this thesis. While described here for monogenic autism spectrum disorders in particular, these technologies can just as well be applied to DEE. Chapter 8 focuses on DEE gene editing and describes how we used the most recent PE technology to introduce and remove DEE mutations. We further focus on some technical aspects of PE such as Sanger bias, benefits of protospacer adjacent motif (PAM) removal, and delivery of the complete machinery from a single plasmid and viral vector. Chapter 9 provides a general discussion on the technologies used, model system advantages and disadvantages, future prospects, and clinical relevance.
WHO Fact Sheet Epilepsy: https://www.who. int/news-room/fact-sheets/detail/epilepsy
Thomas, R. H., & Berkovic, S. F. (2014). The hidden genetics of epilepsy-a clinically important new paradigm. Nature reviews. Neurology, 10(5), 283–292. https://doi.org/10.1038/nrneurol.2014.62
International League Against Epilepsy Consortium on Complex Epilepsies (2018). Genome-wide mega-analysis identifies 16 loci and highlights diverse biological mechanisms in the common epilepsies. Nature communications, 9(1), 5269. https://doi.org/10.1038/s41467018-07524-z
Abdel-Mannan, O., Taylor, H., Donner, E. J., & Sutcliffe, A. G. (2019). A systematic review of sudden unexpected death in epilepsy (SUDEP) in childhood. Epilepsy & behavior : E&B, 90, 99–106. https://doi.org/10.1016/j.yebeh.2018.11.006
Boerma, R. S., Braun, K. P., van den Broek, M. P., van Berkestijn, F. M., Swinkels, M. E., Hagebeuk, E. O., Lindhout, D., van Kempen, M., Boon, M., Nicolai, J., de Kovel, C. G., Brilstra, E. H., & Koeleman, B. P. (2016). Remarkable Phenytoin Sensitivity in 4 Children with SCN8A-related Epilepsy: A Molecular Neuropharmacological Approach. Neurotherapeutics : the journal of the American Society for Experimental NeuroTherapeutics, 13(1), 192–197. https://doi.org/10.1007/s13311-015-0372-8
Wheless, J. W., Fulton, S. P., & Mudigoudar, B. D. (2020). Dravet Syndrome: A Review of Current Management. Pediatric neurology, 107, 28–40. https://doi.org/10.1016/j. pediatrneurol.2020.01.005
Sisodiya S. M. (2021). Precision medicine and therapies of the future. Epilepsia, 62 Suppl 2(Suppl 2), S90–S105. https://doi.org/10.1111/epi.16539
Tate, S. K., Depondt, C., Sisodiya, S. M., Cavalleri, G. L., Schorge, S., Soranzo, N., Thom, M., Sen, A., Shorvon, S. D., Sander, J. W., Wood, N. W., & Goldstein, D. B. (2005). Genetic predictors of
the maximum doses patients receive during clinical use of the anti-epileptic drugs carbamazepine and phenytoin. Proceedings of the National Academy of Sciences of the United States of America, 102(15), 5507–5512. https://doi.org/10.1073/pnas.0407346102
Hinckley, C. A., Kuryshev, Y., Sers, A., Barre, A., Buisson, B., Naik, H., & Hajos, M. (2021). Characterization of Vixotrigine, a BroadSpectrum Voltage-Gated Sodium Channel Blocker. Molecular pharmacology, 99(1), 49–59.
https://doi.org/10.1124/molpharm.120.000079
Mikati, M. A., Jiang, Y. H., Carboni, M., Shashi, V., Petrovski, S., Spillmann, R., Milligan, C. J., Li, M., Grefe, A., McConkie, A., Berkovic, S., Scheffer, I., Mullen, S., Bonner, M., Petrou, S., & Goldstein, D. (2015). Quinidine in the treatment of KCNT1-positive epilepsies. Annals of neurology, 78(6), 995–999. https://doi.org/10.1002/ana.24520
Damase, T. R., Sukhovershin, R., Boada, C., Taraballi, F., Pettigrew, R. I., & Cooke, J. P. (2021). The Limitless Future of RNA Therapeutics. Frontiers in bioengineering and biotechnology, 9, 628137. https://doi. org/10.3389/fbioe.2021.628137
Morris, G., O’Brien, D., & Henshall, D. C. (2021). Opportunities and challenges for microRNAtargeting therapeutics for epilepsy. Trends in pharmacological sciences, 42(7), 605–616. https://doi.org/10.1016/j.tips.2021.04.007
Kampmann M. (2018). CRISPRi and CRISPRa Screens in Mammalian Cells for Precision Biology and Medicine. ACS chemical biology, 13(2), 406–416. https://doi.org/10.1021/acschembio.7b00657
Ran, F. A., Hsu, P. D., Wright, J., Agarwala, V., Scott, D. A., & Zhang, F. (2013). Genome engineering using the CRISPR-Cas9 system. Nature protocols, 8(11), 2281–2308. https://doi.org/10.1038/nprot.2013.143
Clinicaltrials.gov: NCT04601051
Clinicaltrials.gov: NCT03872479
Maeder, M. L., Stefanidakis, M., Wilson, C. J., Baral, R., Barrera, L. A., Bounoutas, G. S., Bumcrot, D., Chao, H., Ciulla, D. M., DaSilva, J. A., Dass, A., Dhanapal, V., Fennell, T. J., Friedland, A. E., Giannoukos, G., Gloskowski, S. W., Glucksmann, A., Gotta, G. M., Jayaram, H., Haskett, S. J., … Jiang, H. (2019). Development of a gene-editing approach to restore vision loss in Leber congenital amaurosis type 10. Nature medicine, 25(2), 229–233. https://doi.org/10.1038/s41591-018-0327-9
Gillmore, J. D., Gane, E., Taubel, J., Kao, J., Fontana, M., Maitland, M. L., Seitzer, J., O’Connell, D., Walsh, K. R., Wood, K., Phillips, J., Xu, Y., Amaral, A., Boyd, A. P., Cehelsky, J. E., McKee, M. D., Schiermeier, A., Harari, O., Murphy, A., Kyratsous, C. A., Lebwohl, D. (2021). CRISPR-Cas9 In Vivo Gene Editing for Transthyretin Amyloidosis. The New England journal of medicine, 385(6), 493–502. https://doi.org/10.1056/NEJMoa2107454
Gaudelli, N. M., Komor, A. C., Rees, H. A., Packer, M. S., Badran, A. H., Bryson, D. I., & Liu, D. R. (2017). Programmable base editing of A•T to G•C in genomic DNA without DNA cleavage. Nature, 551(7681), 464–471. https://doi.org/10.1038/nature24644
Anzalone, A. V., Randolph, P. B., Davis, J. R., Sousa, A. A., Koblan, L. W., Levy, J. M., Chen, P. J., Wilson, C., Newby, G. A., Raguram, A., & Liu, D. R. (2019). Search-and-replace genome editing without double-strand breaks or donor DNA. Nature, 576(7785), 149–157. https://doi.org/10.1038/s41586-019-1711-4
Pausch, P., Al-Shayeb, B., Bisom-Rapp, E., Tsuchida, C. A., Li, Z., Cress, B. F., Knott, G. J., Jacobsen, S. E., Banfield, J. F., & Doudna, J. A. (2020). CRISPR-CasΦ from huge phages is a hypercompact genome editor. Science (New York, N.Y.), 369(6501), 333–337. https://doi.org/10.1126/science.abb1400
Chen, P. J., Hussmann, J. A., Yan, J., Knipping, F., Ravisankar, P., Chen, P. F., Chen, C., Nelson, J. W., Newby, G. A., Sahin, M., Osborn, M. J., Weissman, J. S., Adamson, B., & Liu, D. R. (2021). Enhanced prime editing systems by manipulating cellular determinants of editing outcomes. Cell, 184(22), 5635–5652. e29. https://doi.org/10.1016/j.cell.2021.09.018
AbstractDravet syndrome is caused by dominant loss-of-function mutations in SCN1A which cause reduced activity of Nav1.1 leading to lack of neuronal inhibition. On the other hand, gain-offunction mutations in SCN8A can lead to a severe epileptic encephalopathy subtype by over activating NaV1.6 channels. These observations suggest that Nav1.1 and Nav1.6 represent two opposing sides of the neuronal balance between inhibition and activation. Here, we hypothesize that Dravet syndrome may be treated by either enhancing Nav1.1 or reducing Nav1.6 activity. To test this hypothesis we generated and characterized a novel DS zebrafish model and tested new compounds that selectively activate or inhibit the human NaV1.1 or NaV1.6 channel respectively.
We used CRISPR/Cas9 to generate two separate Scn1Lab knockout lines as an alternative to previous zebrafish models generated by random mutagenesis or morpholino oligomers. Using an optimized locomotor assay, spontaneous burst movements were detected that were unique to Scn1Lab knockouts and disappear when introducing human SCN1A mRNA. Besides the behavioral phenotype, Scn1Lab knockouts show sudden, electrical discharges in the brain that indicate epileptic seizures in zebrafish. Scn1Lab knockouts showed increased sensitivity to the GABA antagonist pentylenetetrazole and a reduction in whole organism GABA levels. Drug screenings further validated a Dravet syndrome phenotype. We tested the NaV1.1 activator AA43279 and two novel NaV1.6 inhibitors MV1369 and MV1312 in the Scn1Lab knockouts. Both type of compounds significantly reduced the number of spontaneous burst movements and seizure activity. Our results show that selective inhibition of NaV1.6 could be just as efficient as selective activation of NaV1.1 and these approaches could prove to be novel potential treatment strategies for Dravet syndrome and other (genetic) epilepsies. Compounds tested in zebrafish however, should always be further validated in other model systems for efficacy in mammals and to screen for potential side effects.
Dravet syndrome (DS), previously known as severe myoclonic epilepsy of infancy (SMEI), is a severe form of epilepsy for which current medication strategies remain largely inefficient. Promising new drugs that act on the serotonin pathway such as Fenfluramine (FA), show efficacy in reducing seizures in 50% to 90% of the patients [1]. However, drug side effects may still limit their use, underscoring the need for further drug discovery.
Of all DS patients, 95% carry a de novo heterozygous mutation in SCN1A [2], which encodes the pore forming α-subunit of neuronal voltage gated sodium channel
(VGSC) type 1 (NaV1.1). NaV1.1 ion channels are the primary Na+ channels in GABAergic interneurons [3,4] and induce the fast depolarization of neuronal membranes during action potential initiation. The majority of SCN1A mutations in DS are loss-offunction (LOF) mutations resulting in dysfunctional NaV1.1 channels, or reduced NaV1.1 expression [5,6]. Consequently, excitability and action potential amplitude of interneurons are attenuated leading to reduced GABA release [7]
Another brain VGSC subtype, NaV1.6 is one of the two main sodium channels expressed in pyramidal neurons, which are responsible for excitatory signals via glutamate excretion [7] . SCN8A, which encodes the NaV1.6 α-subunit is also related to epilepsy and approximately 100 mutations have been reported in patients with severe Early Infantile Epileptic Encephalopathy subtype 13 (EIEE13). Unlike the clear LOF mutations in SCN1A, the majority of the functionally tested SCN8A mutations result in gain-of-function (GOF) of NaV1.6 [8]. GOF mutations in NaV1.6 cause channel hyperactivity due to augmented excitability and firing rates of pyramidal cells concurrent with an increase in glutamate release.
This disease mechanism is reflected by the therapeutic response of VGSC blockers. Various clinical reports have shown that SCN8A-related epilepsy patients benefit from VGSC blockers [9,10], contrasting their inefficacy, or even detrimental effects in DS [11]. These observations indicate that NaV1.1 and NaV1.6 represent two opposing sides of the neuronal balance between inhibition and activation. We hypothesize that LOF mutations in SCN1A have a major negative effect on neuronal inhibition via hypoactivity of inhibitory interneurons, shifting the balance to neuronal hyperactivity. In contrast, GOF SCN8A mutations cause increased activity of excitatory pyramidal neurons, also shifting the VGSCrelated balance towards neuronal hyperactivity. This model suggests that either selective activation of NaV1.1 or selective inhibition of NaV1.6 could be a therapeutic approach in the treatment of both DS and SCN8A related epilepsy.
The therapeutic effect of NaV1.1 activation was previously shown in DS mice using spider venom peptide Hm1a that led to a reduction of seizures and mortality [12] In another study using a different mouse strain, Hm1a was found to be lethal at picomolar doses within two hours [13], indicating that safety and administration needs to be further studied. Another NaV1.1 activator is the chemically synthesized small molecule AA43279, which showed anticonvulsant properties in-vivo but has not been tested in an animal model for DS. In comparison with Hm1a, AA43279 is less selective for NaV1.1, indicating it could activate other NaV subtypes at lower concentrations. Nevertheless, AA43279 did not show lethality or sedative and ataxic side-effects at a concentration of 300mg/kg in mice [15]
Inhibition of NaV1.6 in DS was previously mimicked by introducing an SCN8A missense mutation in DS mice, which reduced seizure susceptibility and increased
their lifespan [14]. Compounds that block NaV1.6 selectively have not been published to date, with the only exception a TTX metabolite for which the sensitivity of NaV1.1 is unknown [16]. have not been published to date.
To test if both NaV1.1 agonists and NaV1.6 antagonists could be beneficial in the treatment of DS we generated a novel DS zebrafish model by knocking out the Scn1Lab gene using CRISPR/Cas9. In humans, most of the mutations that cause DS are severe truncating mutations, while mild missense mutations are observed in patients with a milder epileptic phenotype [3541]. To mimic the genetic architecture of DS in human patients as best possible, the animal model should display a 50% haploinsufficiency of SCN1A. Zebrafish likely carry two orthologues for the human SCN1A gene; Scn1Laa and Scn1Lab. While the expression of these genes does not overlap at embryonic- but only at larval stages [18,25], they have a shared functional role in epilepsy [19]. Since 2010, three other Scn1Lab zebrafish models have been introduced, generated by N-ethyl-N-nitrosourea (ENU) mutagenesis [18,20] or morpholino antisense oligomers (MO) [17]. All three display an epileptic phenotype that includes hyperactivity and epileptiform activity recorded from the brain. Nevertheless, CRISPR/Cas9 is currently the most efficient technique to specifically disrupt the gene that is targeted, unlike ENU mutagenesis [21], and acts on the DNA rather than protein as with MO based approaches [23,24] allowing the generation of animal models that better mimic the genetic architecture of disease. As an improvement to previous zebrafish DS models, we generated two separate zebrafish strains with unique truncating mutations in Scn1Lab using CRISPR/Cas9.
The epileptic phenotype and drug response in zebrafish larvae can be measured by quantifying high velocity burst movements which are indicative of epileptic seizures in fish. The effect of anti-epileptic drugs on this unique behavior phenotype was found to be well correlated with reducing the number of spikes recorded from the zebrafish brain using a local field potential (LFP) set-up [33]. LFP spike events, however should not be confused with spikes of single neurons, but instead indicate the synchronized activity of large numbers of cells [3642]. We performed LFP recordings in combination with a behavior essay to establish the initial phenotype in Scn1Lab knockouts, but used the locomotor assay as a single read-out on previously tested drugs as a validation for our model. After molecular validation, we tested NaV1.1 agonist AA43279 and two novel NaV1.6 channel antagonists, MV1369 and MV1312. Our results show that selective targeting of NaV1.1 or NaV1.6 ion channels reduced both the burst movement phenotype and epileptiform events in Scn1Lab knockout zebrafish, indicating a restoration in neuronal signaling.
(>50mm/s) burst movements were separated from regular locomotor data to yield a burst movement assay. Scn1Lab knockouts showed a significantly higher number of spontaneous burst movements (Figure 2A). Using the nisb-WT control zebrafish, burst movements were found to be unique to Scn1Lab knockouts and not caused by the absence of an inflated swim bladder (Figure 2B). No spontaneous burst movements were observed in heterozygous knockout Scn1Lab larvae (Figure 2A). Using a LFP configuration for zebrafish larvae [26], abnormal brain activity was observed in Scn1Lab knockouts including multiple high frequency singleand poly spiking electrical discharges (Figure 2F). Epileptiform activity recorded from Scn1Lab knockouts resembles those observed in previous Scn1Lab knockdown zebrafish models [17,18]
Fenfluramine (FA) has previously been discovered using the Scn1Lab morpholino knockdown model to be effective in reducing DS seizures [17] and is now shown to be equally effective in our Scn1Lab knockout (Figure 3E). Clemizole (CLM), an antihistamine that can also bind to the serotonin receptor in zebrafish, was discovered using the ENU generated Scn1Lab knock-down model to be effective in reducing DS seizures [18]. When exposed for 24hours to equal concentrations of CLM, toxicity was observed including body malformations and death (S7 Figure). When exposed to 50% of this dose, toxicity was present in 20% of the larvae. When testing the larvae that appeared healthy for a burst movement phenotype reduction, we observed no effect (Figure 3F).
Wildtype zebrafish larvae exposed for a short or long incubation time to the general VGSC activator Veratridine (VRT) developed burst movements, confirming the convulsing effects of VRT in healthy control animals (Figure 4A). Interestingly, Scn1Lab knockouts revealed no additional increase, nor decrease of burst movements after being exposed to VRT (Figure 4A). When exposed to NaV1.1 selective activator AA43279, the number of burst movements in Scn1Lab knockouts was significantly decreased (Figure 4B). This effect was only observed in the short, but not in the long exposure group [15] .
Here, we present two CRISPR/Cas9 generated knockout zebrafish models for SCN1A related epilepsies, including Dravet syndrome. The phenotype of Scn1Lab knockouts is characterized by spontaneous burst movements and sudden electrical discharges in the brain, a phenotype comparable to previous Scn1Lab knock-down and ENU-generated zebrafish models. Here, we used an optimized locomotor assay to extract burst movements from regular movement activity and found that the introduction of human SCN1A mRNA rescues this phenotype, indicating that Scn1Lab and SCN1A have a comparable function, at least during early development. Scn1Lab knockouts show a reduction in whole organism GABA levels, and are more sensitive to convulsions induced by GABA antagonist PTZ, compared to wildtype larvae. These results mimic haploinsufficiency of SCN1A in humans that likely affect local GABA levels, leading to the seizure susceptibility in DS patients. Using a local field potential setup for zebrafish larvae, we detect several signals from the brain that indicate seizure activity, including trains of polyspike discharges. By applying standard AEDs and DS specific drugs we observed a comparable pharmacological response as observed in the majority of DS patients, but also highlighted differences in drug response in comparison to previous Scn1Lab zebrafish models. Finally, we show that the NaV1.1 activator AA43279 and the NaV1.6 inhibiting compound MV1312 reduced the burst movement phenotype and the number of epileptiform events in Scn1Lab knockouts, indicating that selective ion channel subtype inhibition or activation could be beneficial in epilepsy.
In comparison to the other Scn1Lab zebrafish models, the Scn1Lab knockout also responds to VPA and STP but not to CBZ and PHT, mimicking the drug response in humans. FA was effective in the Scn1Lab morphant [17] and the Scn1Lab knockout, but not in the ENU generated Scn1Lab missense model (named didys552 [20]. CLM did show effect in the ENU missense model [18,19], but was shown to be ineffective in another study [20], which matches our result using the Scn1Lab knockout. There are several reasons for the differences in drug response. First and above all, different drug and solvent concentrations and drug incubation times have been used by different laboratories to characterize the drug response of Scn1Lab zebrafish models. The didys552 has initially been characterized by exposing drugs in the millimolar range, using 7% DMSO for a 30, or 90 minute exposure time [18], while the MO model was exposed to micromolar concentrations, with 1% DMSO for 24 and 48 hour exposure time [17]. We were interested in concentrations of drugs and solvents [22] that do not cause toxicity regardless of the exposure time and therefore chose relatively low concentrations. Regarding drug exposure time, we propose that drug should be incubated at least 90 minutes to reach a peak metabolism as shown previously [27]. Interestingly, our results mimic the results of studies that use high drug and
solvent concentrations, with the only exception being Clemizole. Second, all four Scn1Lab zebrafish models differ genetically and likely transcriptionally as they are generated by different techniques. ENU generated animal models likely carry more mutations on top of the one in the gene of interest, as ENU being a carcinogen, randomly mutates 1/100.000 basepairs approximately [21]. While the majority of these are intronic, it could be that they affect genes that regulate drug metabolism or perhaps expression of epilepsy-related genes. Third, the drug response could depend on NaV1.1b protein levels, which are not established in any of the Scn1Lab models due to the absence of suitable zebrafish antibodies. Future research using all four Scn1Lab zebrafish models, characterized in standardized experiments with safe solvent and drug concentrations could reveal why differences in drug response occur.
In our study, the non-selective VGSC activator Veratridine induced burst movements in wildtype zebrafish confirming its convulsing properties and suggesting that it likely has stronger effects on Nav1.6 inducing seizures, rather than increasing inhibition through Nav1.1. However, there was no burst movement increase detected in Veratridine exposed Scn1Lab knockouts, underlining that treatment of SCN1A haploinsufficiency could benefit from NaV activators and the need for VGSC subtype selective compounds. AA43279 is a small molecule with reasonable selectivity for NaV1.1 over the other NaV subtypes and was effective in our Scn1Lab knockouts. However, with lesser affinity AA43279 could also activate other NaV subtypes, potentially leading to unwanted side effects. For example, offtarget activation could lead to myotonia (NaV1.4), atrial fibrillation and cardiac arrest (NaV1.5), seizures (NaV1.2) or neuropathies (NaV1.7 and NaV1.8). For this reason, only compounds with high selectivity and efficacy at a very low dose are suitable candidates for translation to human patients. On the other hand, with future improved drug delivery systems such as viral particle-based or nanoparticles targeting the CNS specifically, off-target activation in the peripheral nervous system and other organs could be limited.
Inhibition, rather than activation of VGSC is a treatment method that might be preferential, especially when inhibition can be targeted to one or few channel subtypes only. Inhibitors of VGSC have been used for decades in epilepsy patients and although current VGSC blocking drugs such as carbamazepine and phenytoin are hardly selective, they have been proven safe in humans. Therefore, to assess whether inhibition of Nav1.6 can be a novel treatment strategy for DS, also applicable for epilepsy caused by SCN8A gain-of function mutations and perhaps also epilepsy in general, we tested two compounds that selectively inhibit NaV1.6 channels. MV1312 effectively rescued the burst movement phenotype and reduced epileptiform activity in our Scn1Lab knockouts comparable to AA43279 and Fenfluramine. For this reason, we believe that NaV1.6 selective inhibitors could be just as efficient as NaV1.1 selective activators and are potentially a safer choice in
human patients. Another compound, MV1369 was found to be selective for NaV1.6 over NaV1.2, Na¬V1.5 and NaV1.7 and reduces the burst movement phenotype in Scn1Lab knockouts as well. There are several reasons why this type of compound is equally effective in the Scn1Lab knockout. First, compounds that are selective for human ion channel proteins, could have a different effect when applied to animal models, as the proteins are not identical to those in humans. Only minor differences on nucleotide level could allow, or limit proper binding of compounds in the protein binding site. Second, not all VGSC genes are evolutionary conserved in zebrafish, but only 6 other ion channel genes are known to exist beside Scn1Laa and Scn1Lab: Scn4aa/ab, Scn5Laa/ab and Scn8aa/ab [25]. While the function of these genes is not yet fully understood, it is clear that the absence of the full spectrum of NaV subtypes in zebrafish limits measurable side-effects. As Scn1Lab is knocked out in our model, the supposed remaining inhibitory neurotransmission is regulated by Scn1Laa, highlighted by the efficacy of the NaV1.1 activator AA43279. However, it could very well be that Scn1Laa is only a partial functional duplicant of Scn1Lab, and carries a shared function and protein structure of SCN1A, SCN2A, SCN3A and even SCN9A as proposed earlier [25]. Nevertheless, zebrafish orthologs for SCN8A are well conserved, reaching >85% DNA and protein identity, highlighting that NaV1.6 inhibitors could be properly tested, but results should be interpreted with caution. Therefore, novel compounds tested in zebrafish, should be always be further studied in other model systems, preferably human-derived. To further improve treatment of genetic epilepsy, and reach selective activation or inhibition with more potency and selectivity, drugs should be designed that act on the nucleotide level. As disorders such as DS have a genetic cause, their treatment should also act at the genomeor transcriptome level. For this reason we are looking forward to clinically relevant treatments based on small activating RNAs, CRISPR/a/i and others that act on the human SCN1A or SCN8A gene.
The authors would like to thank Prof. Dr. J. Bakkers and Dr. F. Tessadori (Hubrecht Institute Utrecht, the Netherlands) for help and advice during the project. Compound AA43279 was a kind gift from Dr. T. Benned-Jensen (H.Lundbeck A/S Copenhagen). The authors would like to thank Prof. P. de Witte (K.U. Leuven, Belgium),Dr. A. Zdebik (U.C. Londen, England) and Dr. T.P. de Boer (UMC Utrecht) for their advice on the electrophysiology setup.
All animal experiments were conducted under the guidelines of the animal welfare committee of the Royal Netherlands Academy of Arts and Sciences (KNAW). Adult zebrafish (Danio rerio) were maintained and embryos raised and staged as previously described [3743]. Adult zebrafish were maintained in 4.5-liter polyethylene tanks (Tecniplast) in an Aqua Schwarz holding system (Göttingen) supplied continuously with circulating UV treated filtered tap water, which was exchanged for 10-30% daily. Average water properties were: Nitrit 0,095mg/L, Nitrat 16,7mg/L, Chloride and Ammonium 0mg/L, Hardness 9,8dH, pH 8,2, conductivity 460mS, Oxygen 6,85ppm and temperature 28,5C under cycles providing 14 hrs of light and 10 hrs of dark (14:10 LD; lights on 9 AM; lights off 11 PM). For all experiments described, larvae of 5 dpf were used. For imaging, larvae were embedded in 2% low-melting point agarose prepared with E3 medium.
Gene specific guide RNAs (sgRNAs) were designed targeting Scn1Lab exon 10, using CHOPCHOP [29] with an off-target binding cut-off of 4 or more base pair mismatches. sgRNA oligonucleotides were synthesized according to previously described methods [30]. Oligos are listed in S1 Table. Capped Cas9 mRNA was created by in vitro transcription using Thermo Fisher mMESSAGE mMACHINE™ SP6 Transcription Kit from pCS2-nls-zCas9-nls (Addgene#47929).
Fertilized eggs were injected with 2 nl of a solution containing 500ng Cas9 mRNA, 150ng sgRNA and 0.2uL Phenol Red. sgRNAs targeting efficiency was tested by PCR amplifying the target region of 8 injected eggs at 2dpf. Primer sequences used for genotyping are listed in S1 Table. Injected embryos were raised to adult mosaic fishes. F0 founders were identified from week 10 by genotyping. F0 founders were outcrossed with wildtype fish to generate F1 embryos. F1 embryos were sampled for genotyping to confirm germline transmission of the mutation. The remaining F1 embryos were raised to adulthood and genotyped at week 10 by fin-clipping. Heterozygous knockouts carrying the same mutations were selected and crossed to raise the homozygous knockout F2 generation.
The gene-specific region including the protospacer adjacent motif (PAM) of the sgRNA was submitted to CCtop [31] to detect potential off-target sites. Five potential off-target sites with a maximum of four mismatches were selected for Sanger sequencing. Target sites, locations and the primers used for sequencing are
included in S1 Table.
Scn1Lab knockout or wildtype larvae were pooled (n=20) in eppendorf tubes in triplicates. Samples were centrifuged at 3500 rpm for 12 minutes at 4C° after which they were lysed in 500µL pre-chilled methanol using 0,5 mm zirconium oxide beads in a bullet blender. Samples were diluted 10 times and frozen at -80C° until day of analysis. A detailed Massspectrometry procedure be found in at S1 File..
Locomotor experiments were performed under dark conditions at 28°C using 5dpf larvae placed in a flat bottom 48-well cell culture dish filled with 1mL E3/ drug solution. Larvae were placed in single wells at 4 dpf to prevent stress from pipetting on the day of measurement. Movements were tracked in an automated tracking device (ZebraBoxTM; Viewpoint, Lyon, France) for 90 minutes, stacked in 10 minute bins, of which the first 30 minutes were removed as habituation time for the locomotor chamber. The final recording time for all locomotor experiments yielded one hour total. Threshold parameters of viewpoint software were freezing 1, sensitivity 8 and burst 50 resulting in a burst movement cut-off value of >50mm/s. Locomotor activity was quantified and analyzed by ZebraLabTM software by Viewpoint.
The SCN1A plasmid, which encodes the human neonatal Nav1.1 ion channel, was previously described [28,32]. Capped hSCN1A mRNA was in vitro transcribed using Thermo Fisher mMESSAGE mMACHINE™ T3 Transcription Kit. For mRNA injections, 200ng/ul mRNA was injected in the yolk of one-cell stage zebrafish embryos.
LFP setup and concurrent recording settings were based on previous work [26] with slight modifications. In short, a silver wire carrying glass electrode connected to a high-impedance amplifier, was filled with 1mM NaCl. All larvae that were recorded were exposed to 20 µM D-Tubocurarine pentahydrate as a muscle relaxant for 10 minutes in order to reduce electromechanical artefacts caused by physical twitching. Next, a single larva was embedded in 1.5% low-melting-point agarose (Invitrogen) and the electrode was placed on top of the forebrain. Recordings were performed in current clamp mode using the DAGAN EX-1 amplifier, national instruments 6210 USB digitizer and WinEDR software. The following parameters were used: 3kHz low-pass filter, 0.3Hz high-pass filter, digital gain 10 and 10 μs sampling interval. Recordings were performed for ten minutes after a ten minute habituation time, and epileptiform events were scored manually.
DMSO. For short incubation experiments larvae were incubated for 1 hour, for long incubation experiments, the incubation time was 18 hours. Compounds were ordered at Sigma-Aldrich.
Compounds AA43279, MV1312 and MV1369 were tested for toxicity based on previously established methods [17] with slight modifications. In short; compounds were incubated in the bathing medium of 4 dpf larvae. After 24 hours, the following toxicity parameters were checked: touch response, loss of posture, body deformation and death. When none of these parameters were observed in any of the larvae tested, the concentration was regarded safe.
Data was analyzed and plotted using Graphpad Prism 7.04. Locomotor data did not pass the D’agostino & Pearson normality test, therefore the non-parametric Mann-Whitney U-test was used for data analysis. Mass-spectrometry was normally distributed and was further analyzed using the student t-test. Compound selectivity and seizure event scoring data was normally distributed and therefore analyzed with a multi-comparison ANOVA. A P-value of <0.05 was considered significant.
Conceived project: B.P.C.K. and K.P.J.B.; designed and performed zebrafish experiments: W.W., S.S., M.R., R.S., M.B. and N.V.D.; generated hSCN1A plasmid: L.V.; designed and performed compound synthesis and selectivity assays: M.R., M.I. and I.V.; analyzed the data and wrote the paper: W.W., M.R. and B.P.C.K.
Supplementary data S1-S6 Figure, S1-S2 File, S1 Table and S1 Video can be found in the online version of this publication.
Schoonjans AS, Lagae L, Ceulemans, B. Lowdose fenfluramine in the treatment of neurologic disorders: experience in Dravet syndrome Ther Adv Neurol Disord. 2015;8: 328–338
Parihar R, Ganesh S. The SCN1A gene variants and epileptic encephalopathies J Hum Genet. 2013;58: 573-80
Han S, Yu FH, Schwartz MD et al. NaV1.1 channels are critical for intercellular communication in the suprachiasmatic nucleus and for normal circadian rhythms Proc Natl Acad Sci U S A 2012; 109: E368–E377.
Lorincz A, Nusser Z. Cell-Type-Dependent Molecular Composition of the Axon Initial Segment Journal of Neuroscience 31 December 2008, 28 (53) 14329-14340
Depienne C, Trouillard O, Saint-Martin C, et al. Spectrum of SCN1A gene mutations associated with Dravet syndrome: analysis of 333 patients Journal of Medical Genetics 2009;46:183-191.
Meng H, Xu H, Yu L, et al. The SCN1A Mutation Database: Updating Information and Analysis of the Relationships among Genotype, Functional Alteration, and Phenotype Human mutation 2015; 36: 573580
Ye M, Yang J, Tian C, et al. Differential roles of NaV1.2 and NaV1.6 in regulating neuronal excitability at febrile temperature and distinct contributions to febrile seizures Sci Rep. 2018;8:753.
Møller RS, Johannesen KM, Precision Medicine: SCN8A Encephalopathy Treated with Sodium Channel Blockers Neurotherapeutics 2016;13:190–191
Boerma RS, Braun KP, van den Broek MP, et al. Remarkable Phenytoin Sensitivity in 4 Children with SCN8A-related Epilepsy: A Molecular Neuropharmacological Approach. Neurotherapeutics 2016; 13:192-7
Wang J, Gao H, Bao X, et al. SCN8A mutations in Chinese patients with early onset epileptic
encephalopathy and benign infantile seizures BMC Med Genet. 2017; 18: 104.
Wirrell EC. Treatment of Dravet Syndrome. Can J Neurol Sci. 2016 43 Suppl 3 S13-
Richards KL, Milligan CJ, Richardson RJ, et al. Selective NaV1.1 activation rescues Dravet syndrome mice from seizures and premature death PNAS 2018 115:E8077 E8085
Escoubas P, Diochot S, Célérier M, et al. Novel Tarantula Toxins for Subtypes of VoltageDependent Potassium Channels in the Kv2 and Kv4 Subfamilies Mol Pharmacol 2002; 62:48-57
Martin MS, Tang B, Papale, LA, et al. The voltage-gated sodium channel Scn8a is a genetic modifier of severe myoclonic epilepsy of infancy. Human molecular genetics 2007; 16: 2892-9
Frederiksen K, Lu D, Yang J, et al. A small molecule activator of Nav 1.1 channels increases fast-spiking interneuron excitability and GABAergic transmission in vitro and has anti-convulsive effects in vivo Eur J Neurosci. 2017;46:1887-1896
Rosker C, Lohberger B, Hofer D, et al. The TTX metabolite 4,9-anhydro-TTX is a highly specific blocker of the Na(v1.6) voltagedependent sodium channel. Am J Physiol Cell Physiol. 2007; 293:C783-9.
Zhang Y, Kecskés A, Copmans D, et al. Pharmacological Characterization of an Antisense Knockdown Zebrafish Model of Dravet Syndrome: Inhibition of Epileptic Seizures by the Serotonin Agonist Fenfluramine PLoS One 2015; 10: e0125898.
Baraban SC1, Dinday MT, Hortopan GA. Drug screening in Scn1a zebrafish mutant identifies clemizole as a potential Dravet syndrome treatment. Nat Commun. 2013;4:2410
Griffin A, Hamling KR , Knupp K, et al. Clemizole and modulators of serotonin signalling suppress seizures in Dravet
syndrome Brain 2017 140: 669–683.
Eimon PM, Ghannad-Rezaie M, De Rienzo G, et al. Brain activity patterns in high throughput electrophysiology screen predict both drug efficacies and side effects Nature Communications 2018;9:219
de Bruijn E1, Cuppen E, Feitsma H. Highly Efficient ENU Mutagenesis in Zebrafish Methods Mol Biol. 2009;546:3-12
Chen T, Wang Y, Wu Y. Developmental exposures to ethanol or dimethylsulfoxide at low concentrations alter locomotor activity in larval zebrafish: Implications for behavioral toxicity bioassays Aquatic Toxicology 2011 102: 162-166
El-Brolosy MA, Stainier DYR. Genetic compensation: A phenomenon in search of mechanisms Plos Genetics 2017+ 13: e1006780.
Lawson ND. Reverse Genetics in Zebrafish: Mutants, Morphants, and Moving Forward Trends in Cell Biology 2016 26: 77-79
Novak AE, Taylor AD, Pineda RH, et al. Embryonic and larval expression of zebrafish voltage-gated sodium channel alpha-subunit genes. Dev Dyn. 2006; 235:1962-73
De Witte P, Lagae, L. Serotonergic modulation as a pharmacological modality in the treatment of Dravet syndrome Brain 2017; 140: e35
Kantae V, Krekels EH, Ordas A, et al. Pharmacokinetic Modeling of Paracetamol Uptake and Clearance in Zebrafish Larvae: Expanding the Allometric Scale in Vertebrates with Five Orders of Magnitude Zebrafish 2016 13:504-510
Hawkins NA, Anderson LL, Gertler TS, et al. Screening of conventional anticonvulsants in a genetic mouse model of epilepsy. Ann Clin Transl Neurol. 2017 4:326-339
Labun K, Montague TG, Gagnon JA, et al; CHOPCHOP v2: a web tool for the next generation of CRISPR genome engineering. Nucleic Acids Research 2016; 44: W272-6
Gagnon JA, Valen E, Thyme SB, et al. Efficient Mutagenesis by Cas9 Protein-Mediated Oligonucleotide Insertion and Large-Scale Assessment of Single-Guide RNAs PLoS ONE 2014; 9: e98186
CCtop: Stemmer M., Thumberger T, del Sol Keyer, M., et al. CCTop: an intuitive, flexible and reliable CRISPR/Cas9 target prediction tool. PLOS ONE 2015; 10: e0124633
Volkers L, Kahlig KM, Verbeek NE, Nav1.1 dysfunction in genetic epilepsy with febrile seizures plus or Dravet syndrome European Journal of Neuroscience 2011; 34: 1275.
Afrikanova T, Serruys AK, Buenafe OEM, Validation of the Zebrafish Pentylenetetrazol Seizure Model: Locomotor versus Electrographic Responses to Antiepileptic Drugs PLoS One 2013; 8: e54166
Deuis JR, Dekan Z, Inserra MC, et al. Development of a muO-Conotoxin Analogue with Improved Lipid Membrane Interactions and Potency for the Analgesic Sodium Channel NaV1.8. The Journal of biological chemistry 2016:291; 11829–11842
Tomson T, Battino D, Perucca E. Valproic acid after five decades of use in epilepsy: time to reconsider the indications of a timehonoured drug The Lancet Neurology 2016; 15; 210-218
Ruffolo G, Cifelli P, Roseti C. A novel GABAergic dysfunction in human Dravet syndrome Epilepsia 2018; 59: 2106-2117
Braat S, Kooy F. The GABAA Receptor as a Therapeutic Target for Neurodevelopmental Disorders Neuron 2015;86:1119-1130
Qiao X, Sun G, Clare JJ. Properties of human brain sodium channel α-subunits expressed in HEK293 cells and their modulation by carbamazepine, phenytoin and lamotrigine Br J Pharmacol. 2014:171; 1054–1067.
Cardenas CA1, Cardenas CG, de Armendi AJ, et al. Carbamazepine interacts with a slow inactivation state of NaV1.8-like sodium channels Neurosci Lett. 2006: 408; 129-34.
FH, Mantegazza M, Westenbroek RE, et
41.
al. Reduced sodium current in GABAergic interneurons in a mouse model of severe myoclonic epilepsy in infancy Nature neuroscience 2006;9:1142-1149
Escayg A, and Goldin AL. Sodium channel SCN1A and epilepsy: mutations and mechanisms Epilepsia 2010; 51:1650–1658.
AbstractDravet syndrome (DS) is a monogenic epileptic encephalopathy caused by lossof-function mutations in the voltage-gated sodium channel (VGSC) gene SCN1A. DS has an age of onset within the first year of life and severe disease prognosis. In the past years, it has been shown that upregulation of endogenous SCN1A can be beneficial in animal models for DS, but a complete rescue was not observed. We hypothesized that upregulation during early development that precedes onset of first symptoms might improve disease outcome. To test this hypothesis, we first evaluated the CRISPR activating method for early upregulation of voltage gated sodium channels during early development. We injected CRISPRa components, which target the proximal or distal promoter region of the VGSC gene scn1Laa in the yolk of one-cell stage zebrafish embryos. The effect of both dCas9-VPR and dCas9-VP64 was evaluated. Both CRISPRa fusions showed toxicity in the majority of embryos, with or without guide RNAs. The few embryos that survived developed normally, and dCas9-VPR induces an upregulation of scn1Laa mRNA until 24 hours after fertilization. At 5 days post fertilization, CRISPRa-injected embryos showed an epileptic phenotype, including locomotor burst movements, hyperactivity, and epileptiform activity originating from the brain. In addition to previously published scn1Laa and scn1Lab loss-of-function models, we conclude that gain of scn1Laa function can have an equally severe phenotype. Upregulation of scn1Laa in the current zebrafish model for DS, scn1Lab-KO, aggravated the disease phenotype, highlighting that early-stage upregulation using CRISPRa can lead to both toxicity and a worsening of the disease phenotype.
Haploinsufficiency is a major disease mechanism in severe monogenic disorders like epileptic encephalopathies. Several approaches now exist that aim to correct haploinsufficiency by exploiting the presence of a healthy copy of the gene. One such technique is CRISPR activation (CRISPRa), that acts via the dCas9 protein fused to a transcription activator, guided to the promoter or enhancer region of the gene of interest [1,2]. Here, the level of transcription can to some extend be controlled by the number of single guide RNAs (sgRNAs) that are used, or the type of transcriptional activator [3]. In the past years, CRISPRa has been applied successfully in rodents to treat several haploinsufficiencybased diseases including Duchenne muscular dystrophy, obesity, temporal lobe epilepsy and Dravet syndrome (DS) [4,5,6,7]. DS is caused by mutations in the voltage gated sodium channel (VGSC) gene SCN1A, which lead to loss of channel function in the majority of cases [8]. There are
In vivo Upregulation of SCN1A Orthologue in Dravet Syndrome
few therapeutic options for DS, and while antiseizure drugs or the ketogenic diet may reduce the number of seizures, comorbidities of DS remain and the disease progresses. Recent efforts using CRISPRa showed that by delivering rAAV particles intracerebroventrically, the expression of Scn1a could be increased in the forebrain of 2/6 DS pups, which led to a reduction of febrile seizures [7]. These findings suggest that increasing the levels of endogenous genes can be a therapeutic approach for monogenic loss of function disorders such as DS.
Ideally, treatment of DS and other monogenic epilepsy syndromes should be initiated as early as possible in life, since many are considered to be developmental and epileptic encephalopathies”, with progressive cognitive and developmental sequalae the longer the disorder is left untreated. Delivering CRISPRa components in the developing brain should therefore aim to prevent, halt, or mitigate disease development, including epileptogenesis. To find out if VGSC genes can be upregulated during early development, we applied CRISPRa to developing zebrafish embryos. Scn1laa is a VGSC gene that is expressed both in the peripheral and central nervous system until 48 hours post fertilization (hpf) [9]. From 72hpf onwards, scn1laa expression is restricted to the brain [10]. Functionally, larvae that carry an ENU-generated missense mutation in scn1laa show locomotor hyperactivity and epileptiform activity recorded from the brain [11]. For many zebrafish genes, a genetic duplicant is retained during evolution that in many cases has a comparable function. scn1lab is the duplicant gene for scn1laa which shows expression restricted to the CNS and faint expression in heart tissue, equal to mammalian SCN1A.9 Until now, a total of five different zebrafish models exist carrying either a mutation or translational knock-down of scn1lab, including the CRISPR/Cas9 generated scn1lab KO generated by our group [10,11,12,13,14,15]. A homozygous loss of scn1lab would mimic a heterozygous SCN1A mutation in mammals, as scn1laa is kept intact. Indeed, each of the scn1lab models shows locomotor hyperactivity, epileptiform activity and a drug response that mimics the majority of DS patients. These findings sum up that scn1laa and scn1lab, at least in a loss-of-function state show a comparable phenotype.
To find out if a loss of scn1lab can be compensated by increased levels of scn1laa we applied CRISPRa in one-cell stage zebrafish embryos. As there are no tissue specific enhancers known for this gene, we target two putative promoter regions, preceding a proximal UTR adjacent to the first coding exon and a 20kb distal UTR. Previously, the dCas9-VP160 fusion was used in zebrafish to upregulate developmental genes that play a role in otic vesicle development [16]. Here, we tested dCas9-VP64 and dCas9VPR EGFP which are supposedly a weaker and stronger variant of dCas9-VP160, respectively. At 5 days post fertilization (dpf), a stage during which the epileptic phenotype manifests itself in the scn1lab-KO model we perform locomotor assays
using optimized movement threshold parameters [14]. In addition, we performed local field potential recordings of CRISPRa-injected wildtype embryos.
To test dCas9-VPR and dCas9-VP64 functionality, we used single guide RNAs (sgRNAs) from a previous study that showed successful upregulation of the forkhead box i1 (foxi1) gene .16 At 5hpf, bright GFP expression is detected throughout the blastoderm of dCas9-VPR EGFP injected wildtype embryos, indicating systemic expression of dCas9-VPR proteins (Figure 1A). Using RT-qPCR dCas9-VPR showed an upregulation of 11-fold using 5 sgRNAs (Figure 1B). In comparison to dCas9VP160 in combination with the exact same sgRNAs, the fold change detected with dCas9-VPR is higher, which is in line with the additional transcription-activating units p65 and Rta part of the VPR conjugate. Curiously, dCas9-VP64 showed a 5-fold downregulation at 5hpf.
More importantly, at 24hpf, the majority of dCas9-VPR or dCas9-VP64 injected wildtype embryos showed malformations and developmental arrest when injected with or without sgRNAs (Figure 1C and 1D). To demonstrate that the injection procedure itself is not the cause of this toxicity we also injected embryos with phenol red or Cas9 mRNA leading to >90% healthy embryos at 24hpf. Lowering the concentration of dCas9-VPR mRNA from 500ng/µL to 125ng/µL only minimally decreased toxicity, highlighting that this side-effect is difficult to abolish. Approximately 10% of the embryos that survived the first 24 hours developed normally thereafter (Figure 1E) and these were used for further analyses.
In vivo Upregulation of SCN1A Orthologue in Dravet Syndrome
number of high velocity burst movements, reduces when drugs are applied that are effective in DS patients, and vice versa remain unchanged, or increase when they are not effective or have adverse effects [10,11,12,14]. To find out if increased levels of scn1laa mRNA during early development could affect the locomotor profile, we analyzed wildtype and scn1lab-KO larvae exposed to CRISPRa at 5dpf. There was no difference in toxicity and mRNA fold changes between wildtype and scn1labKO larvae (data not shown).
CRISPRa injected wildtype larvae show a statistical significant increase in overall locomotor activity (Figure 3A). In scn1lab-KO larva, which generally show more movement activity, the activity is further increased after CRISPRa. When analyzing high-velocity burst movements, a phenotype that is rarely seen in healthy embryos, the number is as well increased in both wildtype and scn1lab-KO larvae injected with CRISPRa (Figure 3B). In CRISPRa injected wildtype embryos the number of burst movements is increased to 50% of that in mutants, suggesting the development of an epileptic phenotype, although less severe.
To test if the upregulation of scn1laa during early development indeed causes epileptiform activity in zebrafish, we performed local field potential recordings of the zebrafish brain. None of the 7 control larvae showed epileptiform events, in contrast to 6 of 7 larvae injected with CRISPRa components. The epileptiform events include high amplitude and highfrequency spike activity, which originated spontaneously similar to that observed in scn1labKO larvae and other zebrafish models for DS. By counting the number of epileptiform events during a 10 minute recording, a statistically significant increase was detected (Figure 3C&3D)
Our results show that injection of dCas9-VPR or dCas9-VP64 during early embryogenesis in zebrafish causes severe toxicity, visible from 24hpf onwards, with malformations or developmental arrest in almost all embryos even when no sgRNAs are added. Reducing the concentration of dCas9-VPR or dCas9-VP64 did not prevent toxicity. In a previous study using the dCas9-VP160 fusion in one-cell stage zebrafish embryos, no toxicity was observed at 11hpf , but no specific toxicity data were provided for later developmental stages .16 In comparison to dCas9-VP64 used in our study, VP160 carries 6 additional VP16 domains explaining why VP64 likely isn’t the cause. The dCas9 mRNA used in both studies is originally aimed for human gene enhancement and not codon-optimized for zebrafish. While codonoptimization will increase correct translation and therefore function, it is unlikely to remove all observed toxicity. As Cas9 mRNA, both human and zebrafish codonoptimized can be injected in zebrafish embryos without problems, we believe the dCas9 unit, perhaps in combination with the transcription activator is causing the toxicity that should be further investigated.
The small number of embryos that survived the first 24 hours developed normally. In these surviving embryos, the expression of voltage-gated sodium channel gene scn1laa can be enhanced by dCas9-VPR dependent on the number of sgRNAs used and both the distal and proximal promoter can be targeted. Surprisingly dCas9VP64 showed a downregulation for both scn1laa and the control gene foxi1. It is not clear why dCas9-VP64 leads to a down- instead of upregulation but there are several possible reasons. One is that VP64 is a weak transcription activator in comparison to VP160 or VPR and the dCas9 fusion instead acts as a physical transcriptional block for RNA polymerase III.
Few embryos were not affected by toxicity and developed normally with increased scn1laa mRNA levels until 48hpf. At 5dpf, wildtype larvae injected with CRISPRa showed an unexpected increase in locomotor activity, frequency of burst movements and epileptiform activity recorded from the brain. This indicates that an increase of scn1laa mRNA during early development initiates an epileptic phenotype. In addition to previous work characterizing a scn1laa loss of function model, we conclude that gain of function can lead to a comparable phenotype. In mammals the CNS VGSC that clearly shows an epileptic phenotype in both a loss and gain-of function state are SCN2A and SCN8A. While an exact comparison between zebrafish and mammals should not be made, it is possible that scn1laa function resembles that of SCN2A, or a combination of several VGSC genes which was also suggested in the original work that described the discovery of zebrafish VGSC genes.
In scn1lab-KO mutants, there is no functional Scn1lab protein produced due to a truncating mutation in exon 10. By upregulating scn1laa mRNA in these mutants we expected to rescue the epileptic phenotype. On the contrary, our results show an increase of locomotor activity and burst movements, which indicate a worsening of the epileptic phenotype. While in a loss of function state the phenotype of scn1laa and scn1lab is comparable, we show that in a gainof-function state scn1laa is unable to rescue a loss of scn1lab. Therefore their function may not be completely equal.
By applying CRISPRa in an early developmental stage, we aimed at upregulation during development of the CNS. Although the phenotypic consequence is clearly originating from the CNS, it is not clear if there is a relevant upregulation in the desired neuronal cell type. While chromatin state likely restricts expression and enhancement of scn1laa in other tissues, it is possible that a 4-fold change at 24hpf is a summary of larger and smaller fold changes between cells and tissues. In addition, scn1laa mRNA levels normalize at 48hpf, almost 3 days before phenotyping is performed. While CRISPRa-mediated gene upregulation has been shown to increase translation of the desired protein which is present long after mRNA degrades, this should be further investigated.
We hypothesized that by upregulating the expression of the healthy copy of SCN1A before the disease manifests itself, DS disease onset and progression may be halted. In our zebrafish model, we did see a contrasting effect, which is likely caused by the use of a duplicant gene, rather than the healthy gene copy in a heterozygous disease model. We show that the zebrafish scn1laa and scn1lab genes are likely not complete functional copies. Nevertheless, our results show that dCas9 may have negative effects when introduced in early development. Careful evaluation in mammalian models of epilepsy should clarify if negative effects observed here are reserved for zebrafish only. When aiming for compensation of LoF genes via CRISPRa or other gene-enhancement technologies, careful dosing and delivery is vital as we show that early stage upregulation of VGSCs could lead to a more severe phenotype. Even if gene enhancement technologies can be applied in DS patients, their effect reduces over time and repeated dosing is required. Gene correction by gene editing technologies would by far, be superior to gene enhancement technologies as the origin of the disease, the DNA mutation is treated.
The authors would like to thank Prof. Dr. J. Bakkers and Dr. F. Tessadori (Hubrecht Institute Utrecht, the Netherlands) for help and advice during the project.
All animal experiments were performed under the guidelines of the animal welfare committee of the Royal Netherlands Academy of Arts and Sciences (KNAW). Adult zebrafish were maintained in 4.5 liter polyethylene tanks (Tecniplast) in an Aqua Schwarz holding system (Göttingen). The zebrafish were continuously supplied with circulating UV treated filtered tap water, which was exchanged for 10–30% daily. Average water properties were: Nitrite 0,095 mg/L, Nitrate 16,7 mg/L, Chloride and Ammonium 0 mg/L, Hardness 9,8 dH (1 dH stands for 17,8 grams of chalk per cubic meter of water, the pH was 8,2, the conductivity was 460 mS, the Oxygen was 6,85 ppm and the temperature was 28,5 °C under cycles providing 14 hrs of light and 10 hrs of dark.
dCas9-VPR EGFP mRNA is commercially available (Horizon discovery CAS12025). dCas9VP64 mRNA was synthetized from plasmid 47107 (Addgene) after linearization by DraIII followed by the T7 mMessage mMachine protocol (AM1344 ThermoFisher).
Initial injection mixtures were concentrated at 500ng/µL dCas9 mRNA, 100ng/µL sgRNA and 10% phenol red based on previous publications. [14],[16] The droplet size corresponds to approximately 500pL of injection volume, which equals 250pg dCas9 mRNA and 50pg sgRNA.[17] After observation of toxic side-effects, the amount of dCas9 mRNA was reduced to 250ng/µL (125pg per embryo) for all further experiments. Control reactions included 250ng/µL dCas9 mRNA and 10% phenol red. Phenol red control injections were performed with 50% phenol red. All injection mixtures were diluted in milliQ and injected in the yolk of one-cell stage zebrafish embryos.
RNA Extraction and RT-qPCR RNA from embryos or larvae (n=15) was extracted using the trizol-chloroform method. In short, embryos were mechanically homogenized in 200µL trizol using a pipet tip followed by 5 min incubation at RT. 100µL chloroform was added followed by 10 sec vortex and 5 min incubation at RT. Samples were centrifuged and the upper fraction was precipitated by ice cold isopropanol and ethanol centrifugation. Samples were subsequently cleaned up using the RNAeasy mini kit, following the RNA clean-up steps in the protocol (74106 Qiagen). 500µg RNA was used for cDNA synthesis (4368814 ThermoFisher). Duplex RT-qPCR reactions were performed using VIC-eef1ab (assayID) and FAM-scn1a (assayID) taqman assays in 10µL volumes. Normalization and fold changes were measured using the 2^ddCT method. Control reactions showing dCT values of uninjected embryos, and embryos injected with dCas9-fusions without sgRNAs are shown in Supplementary data S1
A silver wire carrying glass electrode connected to a high-impedance amplifier, was filled with 1 mM NaCl. All larvae that were recorded were exposed to 10 μM D-Tubocurarine pentahydrate (DTCP) as a muscle relaxant for 2 minutes in order to reduce electromechanical artefacts caused by physical twitching. Next, a single larva was embedded in 2% low-melting-point agarose (Invitrogen) and the electrode was placed on top of the forebrain (non-invasive). Recordings were performed in current clamp mode using the DAGAN EX-1 amplifier, national instruments 6210 USB digitizer and LabScribeNI software. The following parameters were used: 3kHz low-pass filter, 0.3Hz high-pass filter, digital gain 10 and 10 μs sampling interval. Recordings lasted 12 minutes of which the first 2 minutes were discarded as habituation time.
All zebrafish locomotor assays were performed in a 48-well cell culture disk at 28°C in the dark. Movements were tracked by the ZebraboxTM (Viewpoint, Lyon, France) tracking device. For overall locomotor activity, the actinteg parameter was used which is the sum of all pixel changes during recording. Burst movements were recorded using previously established thresholds for burst counts. [14] Recordings lasted 60 minutes of which the first 30 minutes were removed for chamber habituation.
Statistical analysis were performed using GraphPad prism 8.3. First, a test for normality was performed using the D’Agostino-Pearson omnibus normality test or Shapiro-Wilk Normality test for small sample sizes. For normally distributed data the unpaired T test was used. For data that was not-normally distributed the MannWhitney U-test was used. P-values lower than 0.01 were considered statistically significant and displayed as *<0.01 **<0.001 ***<0.0001.
In vivo Upregulation of SCN1A Orthologue in Dravet Syndrome
Conceptualization, data curation, formal analysis, investigation, methodology, project administration, validation, visualization, writing – original draft, writing – review & editing: W.W.; data curation, writing – review & editing: I.D. and J.H.; data curation: M.K. and R.H.S.; writing – review & editing: J.V.; conceptualization, investigation, resources, supervision, writing – review & editing: K.P.J.B.; conceptualization, investigation, resources, supervision, writing – review & editing: B.P.C.K.
Supplementary data S1 can be found in the online version of this publication.
Perez-Pinera P, Kocak DD, Vockley CM, et al. RNA-guided gene activation by CRISPRCas9–based transcription factors. Nature Methods. 2013;10(10):973-976. doi:10.1038/nmeth.2600
Maeder ML, Linder SJ, Cascio VM, Fu Y, et al. CRISPR RNA–guided activation of endogenous human genes. Nature Methods. 2013;10(10):977-979. doi:10.1038/nmeth.2598
Martella A, Firth M, Taylor BJM, et al. Systematic Evaluation of CRISPRa and CRISPRi Modalities Enables Development of a Multiplexed, Orthogonal Gene Activation and Repression System. ACS Synth Biol. 2019;8(9):1998-2006. doi:10.1021/acssynbio.8b00527
Liao H-K, Hatanaka F, Araoka T, et al. In Vivo Target Gene Activation via CRISPR/Cas9Mediated Trans-epigenetic Modulation. Cell. 2017;171(7):1495-1507.e15. doi:10.1016/j. cell.2017.10.025
Matharu N, Rattanasopha S, Tamura S, et al. CRISPR-mediated activation of a promoter or enhancer rescues obesity caused by haploinsufficiency. Science. 2019;363(6424). doi:10.1126/science.aau0629
Colasante G, Qiu Y, Massimino L, et al. In vivo CRISPRa decreases seizures and rescues cognitive deficits in a rodent model of epilepsy. Brain. 2020;143(3):891-905. doi:10.1093/brain/awaa045
Colasante G, Lignani G, Brusco S, et al. dCas9Based Scn1a Gene Activation Restores
Inhibitory Interneuron Excitability and Attenuates Seizures in Dravet Syndrome Mice. Molecular Therapy. 2020;28(1):235-253. doi:10.1016/j.ymthe.2019.08.018
Ceulemans BPGM, Claes LRF, Lagae LG. Clinical correlations of mutations in the SCN1A gene: from febrile seizures to severe myoclonic epilepsy in infancy. Pediatric Neurology. 2004;30(4):236-243. doi:10.1016/j.pediatrneurol.2003.10.012
Novak AE, Taylor AD, Pineda RH, et al. Embryonic and larval expression of zebrafish voltage-gated sodium channel -subunit genes. Developmental Dynamics. 2006;235(7):1962-1973. doi:https://doi.org/10.1002/dvdy.20811
Baraban SC, Dinday MT, Hortopan GA. Drug screening in Scn1a zebrafish mutant identifies clemizole as a potential Dravet syndrome treatment. Nature Communications. 2013;4(1):2410. doi:10.1038/ncomms3410
Griffin A, Hamling KR, Knupp K, et al. Clemizole and modulators of serotonin signalling suppress seizures in Dravet syndrome. Brain. 2017;140(3):669-683. doi:10.1093/brain/aww342
Zhang Y, Kecskés A, Copmans D, et al. Pharmacological Characterization of an Antisense Knockdown Zebrafish Model of Dravet Syndrome: Inhibition of Epileptic Seizures by the Serotonin Agonist Fenfluramine. PLOS ONE. 2015;10(5):e0125898. doi:10.1371/journal.pone.0125898
Eimon PM, Ghannad-Rezaie M, De Rienzo G, et al. Brain activity patterns in high throughput electrophysiology screen predict both drug efficacies and side effects. Nature Communications. 2018;9(1):219. doi:10.1038/s41467-017-02404-4
Weuring WJ, Singh S, Volkers L, et al. NaV1.1 and NaV1.6 selective compounds reduce the behavior phenotype and epileptiform activity in a novel zebrafish model for Dravet Syndrome. PLOS ONE. 2020;15(3):e0219106. doi:10.1371/journal.pone.0219106
Tiraboschi E, Martina S, Ent W van der, et al. New insights into the early mechanisms of epileptogenesis in a zebrafish model of Dravet syndrome. Epilepsia. 2020;61(3):549 560. doi:https://doi.org/10.1111/epi.16456
Long L, Guo H, Yao D, et al. Regulation of transcriptionally active genes via the catalytically inactive Cas9 in C. elegans and D. rerio. Cell Research. 2015;25(5):638
641. doi:10.1038/cr.2015.35
Rosen JN, Sweeney MF, and Mably JD. Microinjection of Zebrafish Embryos to Analyze Gene Function Journal of visualized experiments. 2009:25:1115 doi: 10.3791/1115
There are currently seven different zebrafish strains that model Dravet Syndrome, a severe childhood form of epilepsy. These models are based on a set of duplicated genes, scn1Laa and scn1Lab, which are the orthologues for human SCN1A. Disrupting one of the genes would mimic a heterozygous disease state in humans, as the gene duplicant is still present. While this ‘disease-state model’ is widely accepted, there is also evidence that the function of these genes might not be completely the same. By analyzing the functional domains we discovered several hotspots in the protein that are not conserved indicating a functional difference. Based on this, we generated scn1Laa knockout zebrafish and compared its phenotype to scn1Lab knockouts. The genetic and functional differences we discovered can have implications for the use of zebrafish as a model for Dravet Syndrome.
Dravet syndrome (DS) is a developmental epileptic encephalopathy caused by heter-ozygous loss of function mutations in the voltage gated sodium channel (VGSC) gene SCN1A. DS has an age of onset within the first year of life and a severe disease prognosis. The past ten years, a total of seven different zebrafish models have been generated to model DS, using morpholino antisense oligomers, random mutagenesis using N-ethyl-N-nitrosourea (ENU), or CRISPR/Cas9, including the heterozygous and homozygous scn1Lab knockout strains by our lab. These have fast-forwarded our understanding of epileptogenesis [1] and behavioural comorbidities [2], enabled rapid drug repurposing for Fenfluramine[3] and Clemizole [4], yielded the efficacy of novel VGSC subtype-selective compounds [5], and were used to test in-vivo functionality of CRISPR activation [6]. Zebrafish carry two orthologs for human SCN1A named scn1Laa and scn1Lab [7], which are duplicated paralogue genes that were initially as-sumed to have similar, or even identical function. Current DS zebrafish models are based on this assumption (Figure 1)
Scn1Laa and Scn1Lab, showing 67% identity in comparison to 88% for the comparison of Scn8aa and Scn8ab.
Paralog genes generally have an equal function, as they are originating from the same ancestor gene, but it is possible that they develop new functions, or discard functions that are not advantageous during evolution. While all voltage gated sodium channels transport Na ions during the generation of action potentials, their location, action potential thresholds and interaction with beta subunits can differentiate between subtype-selective function [13]. To find out if this could be the case for scn1Laa we aligned Scn1Laa, Scn1Lab and SCN1A using ClustalOmega. Based on the general structure of VGSC we analyze in more detail domains such as the voltage sensor, pore region and regions involved with beta subunit binding to predict potential functional differences. We generated a scn1Laa knockout strain using CRISPR/Cas9, performed electrophysiology experiments to measure brain activity patterns and compared the locomotor phenotype with scn1Lab-/-. We summarize with implications and future prospects for the DS zebrafish model and duplicated genes in general.
Previous alignments of Scn1Laa and Scn1Lab with human SCN1A showed only overall similarity without much focus on domains with functional importance. We first per-formed multiple sequence alignment of Scn1Laa or Scn1Lab versus SCN1A using the ClustalW program that show predicted protein-wide identity scores of 67% for Scn1Laa and 77% for Scn1Lab confirming previous findings [ref.novak2006]. This alignment result was used to calculate the percentages of amino acid conservation for the 58 different pro-tein domains of SCN1A as defined in Uniprot. In more detail, out of the 58 protein do-mains 12 show more than 20% difference in conservation between scn1Laa and SCN1A when compared to the conservation between scn1Lab and SCN1A. These low-conserved domains are spread over the ion channel and include TM segments, intracellular linkers, extracellular loops and the N-terminus. Important regions for the primary function of VGSC are the S4 voltage sensor, S5 and S6 porelining segments, the S5-S6 pore-forming loop X and the S2-S3 cytoplasmic linker (inactivation gate). All these regions show a lower conservation score for Scn1Laa, and two regions, the S4 voltage sensor segment in domain II and cytoplasmic linker E in domain IV, exceed more than 20% difference.
Figure 2 Top-down and horizontal structure of VGSC and multiple sequence alignment of Scn1Laa:SCN1A and Scn1Lab:SCN1A. Scores are percentage amino acid identity of Scn1Lab/Scn1Laa versus SCN1A. Roman letters indicate Domain I, II, III and IV, S1-S6=transmembrane segment 1-6, A-D extracellular loops, E-G cytoplasmic linkers.
Low conservation in the voltage sensor may indicate a different voltage threshold needed for channel activation and inactivation which in turn suggests that scn1Laa might be expressed to other cell types, or at a different location within a neuron when compared to scn1Lab. More difficult to explain are the low conservation scores in intracellular linkers and extracellular pore segments. While intracellular linkers could be associated with protein interaction intracellularly, these protein interactions are largely unknown for SCN1A and the zebrafish orthologues. A possible candidate for SCN1A is SCN1B, the beta-subunit of Nav1.1 and required for correct localization of the VGSC to the membrane. However, the exact binding site of SCN1B to SCN1A is not known and since SCN1B also contains both a transmembrane and intracellular domain this remains a topic of debate.
Heterozygous and homozygous scn1Laa knockout zebrafish larvae (scn1Laa+/- and scn1laa-/-) were generated using CRISPR/Cas9 and carry a 7bp deletion in exon 9 leading to a premature stopcodon that predicts a complete loss of function (Figure 3A). All experiments described below were performed with animals at 5 dpf, comparable to previous publications. Scn1laa-/- do not share the hyperpigmentation phenotype, nor the absence of an inflated swim bladder as do models based on scn1Lab. Local Field Potential (LFP) recordings were used to evaluate the presence of abnormal brain activity as described before (4). LFP recordings of scn1laa-/- zebrafish show spontaneous spike activity. This epileptiform activity could clearly be separated from artefacts and confirmed those detected in the scn1Laasa1647 zebrafish model published earlier (Figure 3C). We further tested the
influence of light stimuli to induce epileptiform activity. For this purpose, zebrafish were exposed to intermittent light at 30 second intervals. This was repeated for 5 minutes, during which LFP recordings were continuously made. Under these conditions, scn1laa-/- show repeated trains of biphasic spike activity that follow directly after each light stimulus, suggesting that scn1laa-/- zebrafish display a photosensitive epileptiform phenotype, which was detected in several scn1Lab models earlier.
These findings highlight that scn1Laa and scn1Lab are both involved in epileptogenesis, and affect the sensory pathways of the brain. To find out if there are differences in the locomotor profile of scn1laa-/- a locomotor assay was performed under dark conditions that measured hyperactivity and high velocity burst movements as described before (4). Surprisingly, scn1laa-/- larvae show no alteration in overall movement activity when compared to wildtype zebrafish, unlike scn1Lab-/- which are hyperactive. Burst movements, typical for scn1Lab-/- and used for ASD screenings are also absent in scn1laa-/- highlighting a functional difference (Figure 3B)
Figure 3
Phenotype of scn1laa-/-. A) Sanger trace showing wt (top), heterozygous 7bp deletion (middle) and homozygous 7bp deletion (bottom) strains generated for this study. B) Locomotor data using scn1Laa and scn1Lab knockout strains. C) LFP recording of scn1Laa knockout brain activity 1: spontaneous spike activity 2: light stimuli presented at each asterix 3: LFP artefact 4-7: epileptiform activity.
Summarizing the differences in early expression of scn1Laa and scn1Lab transcripts from previous studies and our structural alignment and phenotype data, we conclude that it is likely that scn1Laa and scn1Lab do not have identical functions.
We first showed that scn1Laa and scn1Lab differ substantially at the protein level. To predict whether these differences have functional consequences, we compared amino acid conservation score for each of the 58 protein domains in VGSC. This analysis showed that sequence conservation was unevenly distributed across the protein, and 12 domains, including the functionally important voltage sensing segment in DII and intracellular linkers, showed more than 20% sequence difference that may indicate a functional difference.
Furthermore, we observed striking differences between the phenotypes of the scn1laa-/- and scn1Lab-/- zebrafish. The two different KO zebrafish do share the presence of spontaneous epileptiform activity and photosensitivity. However, the scn1laa-/- does not have a locomotor phenotype that is characteristic of scn1Lab-/zebrafish. This further suggests that underlying pathophysiology results in a different locomotor phenotype. Scn1Lab-/-, but also scn1Lab-based models with homozygous missense mutations, or a translational knockdown show hyperactivity and high velocity burst movements which are absent in scn1laa-/-. The absence of a locomotor hyperactivity phenotype indicates that scn1Laa might be located to other neurons, other cell compartments such as soma, axon or dendrite and/or have a different action potential threshold. While all VGSC transport sodium, their spatiotemporal location is crucial for each specific subtype and determines its unique function.
It is not clear why another scn1Laa model published previously scn1Laasa1674 does show locomotor hyperactivity and this should be further investigated. It is possible that phenotypic differences come from how accurate the technology is that is used to introduce the mutation to generate the strain. ENU-generated models likely carry additional mutations beside the mutation that is described as caustive, on top of the mutations that are crossed out after ENU treatment [14] whereas CRISPR/Cas9, using stringent sgRNA design is more precise. Differences in chamber habituation time, possible stress from pipetting and recording parameters can potentially contribute to differences in locomotor phenotypes. We generally place larvae in a single well one day prior to the experiment, subtract the first 30m of data due to chamber habituation, and record for a total of one hour.
Overall, given the increasing number of zebrafish models, we believe that
standardization of experiments can rule out the majority of false negative and false positive findings. As we used standardized methods for both scn1laa-/- and scn1Lab-/strains in this study, an effective comparison can be made. With the introduction of the zebrafish epilepsy pro-ject published earlier this year [12], a plethora of different zebrafish models can be within reach for researchers world-wide, hopefully solving the puzzle of inter- and intra-strain variation.
Protein sequences for SCN1A (SCN1A-224, ENST00000674923.1) Scn1Laa (scn1laa-203, ENSDART00000161648.3) and Scn1Lab (scn1lab-202, ENSDART00000151247.3) were initially aligned using both ClustalW and Muscle via Jalview [15]. As alignment identity scores did not differ between these software ClustalW was ultimately used for percentages per protein domain. Protein domains were established using Uniprot (https://www.uniprot.org/) [16] and final figures were generated with Biorender (www. biorender.com).
All animal experiments were conducted under the guidelines of the animal welfare committee of the Royal Netherlands Academy of Arts and Sciences (KNAW).
Adult zebrafish (Danio rerio) were maintained and embryos raised and staged as previously described. Adult zebrafish were maintained in 4.5-liter polyethylene tanks (Tecniplast) in an Aqua Schwarz holding system (Göttingen) supplied continuously with circulating UV treated filtered tap water, which was exchanged for 10–30% daily. Average water properties were: Nitrit 0,095mg/L, Nitrat 16,7mg/L, Chloride and Ammonium 0mg/L, Hardness 9,8dH, pH 8,2, conductivity 460mS, Oxygen 6,85ppm and temperature 28,5C under cycles providing 14 hrs of light and 10 hrs of dark (14:10 LD; lights on 9 AM; lights off 11 PM).
Knockout zebrafish were generated using CRISPR/Cas9 technoloy according to previously published methods [5]. In brief, 500ng Cas9 mRNA and 150ng sgRNA [GATGAGGTTCACCAGGTAGA] + Cas9 scaffold sequence were injected in one-cell stage zebrafish embryos. F0 founder strains were identified by outcrossing leading to F1 heterozygote strains. F2 generation 5 dpf embryos were generated by incrossing the F1 generation, and heterozygote or homozygous knockout embryos were individually validated by sequencing. Primer sequences for PCR amplification and sequencing are FW: TTTGATCCAATCCCTTATCC
Locomotor experiments were performed according to previously published methods [5]. In brief, zebrafish larvae were placed in flat-bottom 48-well plates filled with E3 medium at 4dpf to reduce stress from pipetting on the recording day. At 5dpf movements were tracked in an automated tracking device (ZebraBox™; Viewpoint, Lyon, France) for 90 minutes, stacked in 10 minute bins, of which the first 30 minutes were removed as habituation time for the locomotor chamber. Threshold parameters for the burst movement protocol were freezing 1, sensitivity 8 and burst 50 resulting in a burst movement cut-off value of 50mm/s.
Local field potential recordings of zebrafish brain activity were performed according to previously published methods [5]. In brief, each larvae was exposed to 10 μM DTubocurarine pentahydrate (DTCP) as a muscle relaxant for 2 minutes in order to reduce electro-mechanical artefacts caused by physical twitching and embedded in 1.5% lowmelting point agarose. Next, a silver wire carrying glass electrode connected to a highimpedance amplifier, filled with 1 mM NaCl was directed on top of the forebrain of 5dpf larvae. Recordings were performed in current clamp mode using the DAGAN EX-1 amplifier, national instruments 6210 USB digitizer and winEDR software. The following parameters were used: 3kHz low-pass filter, 0.3Hz high-pass filter, digital gain 10 and 10 μs sampling interval. Each recording lasted 13 minutes, of which the first was used to place the electrode and removed from the final figures.
Writing — original draft preparation: W.W. and J.H.; writing — review and editing: W.W., B.K. and K.B.; data curation visualization: J.H. and W.W.; supervision: B.K. and W.W.; project administration: B.K. and K.B. ; funding acquisition: B.K. and K.B.
Tiraboschi, E., Martina, S., Ent, W., Grzyb, K., Gawel, K., Cordero-Maldonado, M. L., Poovathingal, S. K., Heintz, S., Satheesh, S. V., Brattespe, J., Xu, J., Suster, M., Skupin, A., & Esguerra, C. V. (2020). New insights into the early mechanisms of epileptogenesis in a zebrafish model of Dravet syndrome. Epilepsia, 61(3), 549–560. https://doi. org/10.1111/epi.16456
Grone, B. P., Qu, T., & Baraban, S. C. (2017). Behavioral Comorbidities and Drug Treatments in a Zebrafish scn1lab Model of Dravet Syndrome. Eneuro, 4(4), ENEURO.0066-17.2017. https://doi. org/10.1523/eneuro.0066-17.2017
Zhang, Y., Kecskés, A., Copmans, D., Langlois, M., Crawford, A. D., Ceulemans, B., Lagae, L., de Witte, P. A. M., & Esguerra, C. V. (2015). Pharmacological Characterization of an Antisense Knockdown Zebrafish Model of Dravet Syndrome: Inhibition of Epileptic Seizures by the Serotonin Agonist Fenfluramine. PLOS ONE, 10(5), e0125898. https://doi.org/10.1371/journal.pone.0125898
Baraban, S. C., Dinday, M. T., & Hortopan, G. A. (2013). Drug screening in Scn1a zebrafish mutant identifies clemizole as a potential Dravet syndrome treatment. Nature Communications, 4(1). https://doi. org/10.1038/ncomms3410
Weuring, W. J., Singh, S., Volkers, L., Rook, M. B., van ‘T Slot, R. H., Bosma, M., Inserra, M., Vetter, I., Verhoeven-Duif, N. M., Braun, K. P. J., Rivara, M., & Koeleman, B. P. C. (2020). NaV1.1 and NaV1.6 selective compounds reduce the behavior phenotype and epileptiform activity in a novel zebrafish model for Dravet Syndrome. PLOS ONE, 15(3), e0219106. https:// doi.org/10.1371/journal.pone.0219106
Weuring, W. J., Dilevska, I., Hoekman, J., van de Vondervoort, J., Koetsier, M., van ’t Slot, R. H., Braun, K. P., & Koeleman, B. P. (2021). CRISPRa-Mediated Upregulation of scn1laa During Early Development Causes Epileptiform Activity and dCas9-Associated Toxicity. The CRISPR Journal, 4(4), 575–582. https://doi.org/10.1089/crispr.2021.0013
Novak, A. E., Taylor, A. D., Pineda, R. H., Lasda, E. L., Wright, M. A., & Ribera, A. B. (2006). Embryonic and larval expression of zebrafish voltage-gated sodium channel αsubunit genes. Developmental Dynamics, 235(7), 1962–1973. https://doi.org/10.1002/dvdy.20811
Novak AE, Jost MC, Lu Y, Taylor AD, Zakon HH, Ribera AB. Gene duplications and evolution of vertebrate voltage-gated sodium channels. J Mol Evol. 2006 Aug;63(2):208-21. doi: 10.1007/ s00239-005-0287-9
Griffin, A., Hamling, K. R., Knupp, K., Hong, S., Lee, L. P., & Baraban, S. C. (2017). Clemizole and modulators of serotonin signalling suppress seizures in Dravet syndrome. Brain, aww342. https://doi.org/10.1093/brain/aww342
Kroll, F., Powell, G. T., Ghosh, M., Gestri, G., Antinucci, P., Hearn, T. J., Tunbak, H., Lim, S., Dennis, H. W., Fernandez, J. M., Whitmore, D., Dreosti, E., Wilson, S. W., Hoffman, E. J., & Rihel, J. (2021). A simple and effective F0 knockout method for rapid screening of behaviour and other complex phenotypes. ELife, 10. https://doi.org/10.7554/elife.59683
Eimon, P. M., Ghannad-Rezaie, M., de Rienzo, G., Allalou, A., Wu, Y., Gao, M., Roy, A., Skolnick, J., & Yanik, M. F. (2018). Brain activity patterns in high-throughput electrophysiology screen predict both drug efficacies and side effects. Nature Communications, 9(1). https://doi. org/10.1038/s41467-017-02404-4
Griffin, A., Carpenter, C., Liu, J., Paterno, R., Grone, B., Hamling, K., Moog, M., Dinday, M. T., Figueroa, F., Anvar, M., Ononuju, C., Qu, T., & Baraban, S. C. (2021). Phenotypic analysis of catastrophic childhood epilepsy genes. Communications Biology, 4(1). https://doi. org/10.1038/s42003-021-02221-y
Kiernan, M. C., Mogyoros, I., & Burke, D. (1996). Differences in the recovery of excitability in sensory and motor axons of human median nerve. Brain, 119(4), 1099–1105. https://doi. org/10.1093/brain/119.4.1099
de Bruijn, E., Cuppen, E., & Feitsma, H.
15.
(2009). Highly Efficient ENU Mutagenesis in Zebrafish. Methods in Molecular Biology, 3–12. https://doi.org/10.1007/978-1-60327-977-2_1
Jalview Retrieved November, 2021 from https://www.jalview.org
16.
Uniprot. Retrieved November, 2021 from https://www.uniprot.org
Background: Pathogenic variants in SCN1A cause variable epilepsy disorders with different disease severities. We here investigate whether common variation in the promoter region of the unaffected SCN1A allele could reduce normal expression, leading to a decreased residual function of Nav1.1, and therefore to more severe clinical outcomes in patients affected by pathogenic SCN1A variants.
Methods: Five different SCN1A promoter-haplotypes were functionally assessed in SHSY5Y cells using Firefly and Renilla luciferase assays. The SCN1A promoter region was analyzed in a cohort of 143 participants with SCN1A pathogenic variants. Differences in clinical features and outcomes between participants with and without common variants in the SCN1A promoter-region of their unaffected allele were investigated.
Results: All non-wildtype haplotypes showed a significant reduction of luciferase expression, compared to the wildtype promoter-region (65%-80%, p=0.039 - 0.0023). No statistically significant differences in clinical outcomes were observed between patients with and without common promoter variants. However, patients with a wildtype promoterhaplotype on their unaffected SCN1A allele showed a nonsignificant trend for milder phenotypes.
Conclusion: The non-significant observed trends in our study warrant replication studies in larger cohorts to explore the potential modifying role of these common SCN1A promoterhaplotypes.
Dravet syndrome is one of the most well-known genetic epilepsy syndromes. The main characteristics of the disease are early onset intractable epileptic seizures and a delayed psychomotor development that results in mild to severe intellectual disability (ID). Furthermore, many patients experience walking difficulties and/or behavioural problems (Brunklaus, Ellis, Reavey, Forbes, & Zuberi, 2012; C Dravet, 1978; Charlotte Dravet, 2011; Gitiaux et al., 2016; Rilstone, Coelho, Minassian, & Andrade, 2012).
Mutations in the SCN1A gene are the cause of disease and detected in the majority of Dravet syndrome patients (Parihar & Ganesh, 2013). SCN1A encodes for the α-subunit of a neuronal sodium channel, Nav1.1. The main disease mechanism in
SCN1A-related Dravet syndrome is haploinsufficiency, caused by complete or partial loss of function of the channel, which leads to disturbances in neuronal excitability (Catterall, Kalume, & Oakley, 2010; A Escayg & Goldin, 2010).
Pathogenic variants in SCN1A are also found in patients with much milder phenotypes, such as Genetic Epilepsy Febrile Seizures Plus (GEFS+) syndrome or febrile seizures only (Andrew Escayg et al., 2000). The association of SCN1A with multiple phenotypes may be partly explained by the varying effects of different pathogenic variants: variants that cause a complete loss of function (LoF) of the channel are virtually always associated with severe phenotypes, whereas variants that cause milder disturbances are usually found in milder phenotypes (Meng et al., 2015). However, this does not fully explain the variability that is observed in SCN1A related phenotypes: varying phenotypes have been associated with the exact same variant, even within families, and Dravet syndrome patients with similar LoF variants may show very different clinical outcomes (Akiyama, Kobayashi, Yoshinaga, & Ohtsuka, 2010; Depienne et al., 2010; Guerrini et al., 2010; Harkin et al., 2007; Jansen et al., 2006; Mahoney et al., 2009; Passamonti et al., 2015; PinedaTrujillo et al., 2005; Suls et al., 2010). Several modifying factors have already been proven or suggested to have an influence on these outcomes, such as mosaicism for the pathogenic SCN1A variants, the presence of variants in modifier genes and environmental factors such as anti-epileptic treatment (Ceulemans, 2011; de Lange, Gunning, et al., 2018; de Lange, Koudijs, et al., 2018; Depienne et al., 2010; Gennaro et al., 2006; Guerrini et al., 1998; Marini, Mei, Helen Cross, & Guerrini, 2006).
Another factor that could potentially contribute to phenotypic variability is additional variation in the SCN1A gene itself. Genome-wide association studies (GWAS) have shown a significant association between SCN1A and genetic generalized, focal and unclassified epilepsies in general, and hippocampal sclerosis and febrile seizures (Anney et al., 2014; Kasperavičiute et al., 2013). This observation suggests that common, low risk variation may affect normal function and/or expression of SCN1A SCN1A has at least two major promoters, h1a and h1b that are simultaneously active in various brain regions including the cerebellum, cerebral cortex, putamen, hippocampus and thalamus (Nakayama et al., 2010). Both promoters alone yield transcription activity in a neuronal cell culture assay, though the activity was greatly enhanced when 5’ untranslated exons (UE) were added (Long et al., 2008). A total of five 5’ UEs of SCN1A are currently known, all of them carrying multiple putative transcription factor binding sites (Long et al., 2008; Martin, Tang, Ta, & Escayg, 2007). Adding to the complexity of SCN1A transcription, the 5’ untranslated region including both major promoters are located 75Kb upstream of the first coding exon (Long et al., 2008; Martin et al., 2007) (Figure 1). This region has not been studied extensively in Dravet syndrome patients, but may harbor mutations that could either be the cause of their epilepsy, or include variants that could modify the phenotype caused by another major mutation in the coding region of
the gene. So far, two reports have been published that suggested that pathogenic mutations in the regulatory 5’ region of SCN1A were likely the cause of disease in two Dravet syndrome patients, as no SCN1A coding mutations could be detected. Interestingly, the novel promoter mutations were found to reduce transcription in-vitro, increasing the likelihood of their causality (Gao et al., 2017; Nakayama et al., 2010). These findings stress the importance of the SCN1A promoter-regions for correct functioning of the Nav1.1 channel. It has previously been suggested that part of the 20-30% of Dravet syndrome patients in whom no coding variants in SCN1A could be detected, harbor mutations in its regulatory regions (Djémié et al., 2016). However, in most diagnostic centers the promoter regions are not routinely sequenced when analyzing SCN1A, so its exact role remains unclear.
Figure 1 Overview SCN1A 5’ UTR SCN1A has a complex 5’ UTR. At least three promoters (blue) and five 5’ UE (pink) are currently known. The half-tick up lines indicates both promoter regions including the subsequent 5’ UE with an Initiator element, transcription start site is indicated with an arrow. Dashed lines indicate the distance to the next element. Underlined elements indicate the remaining three 5’ UEs and the first coding exon of SCN1A.
We hypothesize that not only pathogenic mutations, but also common variation on the promoter regions of SCN1A can interfere with normal expression. Although the effects of common variation are likely milder than those of a true pathogenic mutation in the promoter regions, a clinical effect might be detectable when common variation in the promoter regions coexists with a pathogenic mutation in the coding region of SCN1A on the other allele. A small decrease in expression of SCN1A could lead to a decreased residual function of Nav1.1 in patients that are already haploinsufficient, and therefore lead to more severe clinical outcomes. Previously, no significant differences in expression were observed for a group of common variants in the first SCN1A promoter region (Gao et al., 2017). We have cloned a new set of haplotypes and used a slightly altered promoter region that includes the first 5’ UE in the functional expression analysis. In this study, we analyze the first SCN1A promoter-region of 143 participants affected by pathogenic SCN1A variants, to investigate whether common variation in this region can affect phenotypic outcomes.
Functional characterization of common SCN1A promoter variants 5 different SCN1A promoter-haplotypes were defined, based on eight SNPs in the -2271 to 297 region (Figure R1). Haplotype 1 lacks all eight SNPs and was therefore regarded wildtype, from which the relative SCN1A expression levels were estimated for haplotypes 2-5. All non-wildtype haplotypes (2-5) showed a significant reduction in luciferase expression, compared to the wildtype promoter-region (Figure R1). The relative SCN1A expression decreased for 73% by haplotype 2, 64% by haplotype 3, 75% by haplotype 4 or haplotype 5).
Figure R1 Functional Effect of Common Promoter Variants in SCN1A. Top: Simplified SCN1A 5’ UTR, adapted from Figure 1. Middle: Promoter haplotypes tested in this study. Haplotype 1 depicts a promoter+h1u region without common variants. Haplotype 2,3,4 and 5 carry multiple common variants spread over the promoter region. Bottom: Luciferase expression analysis of SCN1A promoter haplotypes as depicted above. Empty vector (SV40) expression was set to 100%. Haplotype 1, without common variants was used as control haplotype of which the expression reduction of Haplotype 2,3,4 and 5 was measured.
SmMIP-sequencing results were obtained for all participants. In all patients their known SCN1A pathogenic variant could be identified, except for variants undetectable by whole exome sequencing (e.g. deletions of the complete SCN1A gene), meaning no samples swaps had occurred. In 46 patients the promoter variant genotype of their unaffected SCN1A allele could be reconstructed. All other patients had heterozygous genotypes at at least one of the three promoter variant locations, and had no included family members who could be used for haplotype phasing.
The strongest association with common epilepsy has been mapped to the SCN1A region in a recent GWAS of common epilepsy, with the top SNP being rs6432877. The -52 and -1036 promoter variants showed borderline genome wide significant with the “all epilepsy” phenotype, whereas the association of the -1964 variant was much weaker (p=6,20E-8, 1,00E7 and 0.68 respectively). In order to test whether the promoter variants were correlated to the SCN1A GWAS signal, we conditioned on the top GWAS SCN1A-SNP (rs6432877) and observed that the associations were no longer significant indicating that these promoter variants SNPs are in variable Linkage Disequilibrium with the top GWAS SNP (r2=0.21, 0.63 and 0.12 respectively). Conversely, we also tested to see if the signal from the top GWAS SCN1A-SNP could be explained by one of the promoter variants by conditioning on each in turn. The strength of the GWAS signal diminished marginally when conditioning on the -52 and -1036 variants but was not affected by conditioning on the -1964 variant (pcond=3.99E08, 1.16E-05 and 1.12E-13 respectively), indicating that the GWAS signal was not entirely dependent on the promoter variants.
40 of the 46 participants with reconstructed promoter-haplotypes had been diagnosed with Dravet syndrome; the others had either GEFS+ syndrome or febrile seizures, and one participant had never experienced any seizures. Regarding the 40 Dravet syndrome patients: in 9 patients a wildtype promoter-region was detected (haplotype 1); none of the patients carried haplotype 2; haplotype 3 was identified in only one patient; haplotype 4 was present in 12, and haplotype 5 was found in 18 participants. An overview of the clinical outcomes of the 40 Dravet syndrome patients is shown in table 1a. No statistically significant differences were seen between patients with and without the common promoter variants (Table 1, Figure R2-R6). However, patients with a wildtype promoter-haplotype on their unaffected SCN1A allele showed a non-significant trend for milder phenotypes, when compared to patients that carried a variant promoter haplotype: on average, seizure onsets occurred at an older age (6.1 versus 5.1 months, p=0.746), as did developmental delays (median 36-47 months versus median 24-35 months, p=0.265). Furthermore, cognitive capacities declined slower (IQ after 5 years of disease 73 versus 65.9,
p=0.566 ). More favorable cognitive outcome scores were also observed, although this is likely to be at least partly due to the wildtype-patients being younger than the other group. Similar outcomes were seen for Dravet syndrome patients with truncating variants only (Table 1b): although this group consisted of only 19 patients, leading to a lower detection power, a similar non-significant trend for milder phenotypes was observed in patients with wildtype promoters.
Table 1 Clinical outcomes of patients with different promoter haplotypes (all non-mosaic Dravet Syndrome patients)
Among the complete group of 46 participants with reconstructed promoterhaplotypes were eight participants, belonging to four different families that showed a clear intra-familial variability (Figure R7): family 1 consists of a severely affected 10 year old proband with Dravet syndrome, and a father with mild epilepsy and normal cognitive functioning. Family 2 consists of two brothers with Dravet syndrome, one of whom is more severely affected than the other. Family 3 consists of a proband with a phenotype on the border of Dravet syndrome and GEFS+, with regression over the years. His father has never had any seizures. Family 4 consists of two brothers of whom the oldest has severe Dravet syndrome and the youngest has a much milder phenotype. In family 2, 3 and 4, each of the milder participants carried haplotype 5, and each of the more severely affected participants carried haplotype 4. Since only very small, insignificant differences in luciferase expression between haplotype 4 and 5 were observed, the different promoter-haplotypes are unlikely to explain the clinical differences between these patients. However, in family 1, the severely affected patient carried haplotype 5, whereas the milder patient had a wildtype promoter, for which we did observe a large difference in luciferase expression.
distribution of different cognitive outcome scores between patients with and without variants in the promoter-region of their unaffected SCN1A allele
distribution of age at seizure onset between patients with and without variants in the promoterregion of their unaffected SCN1A allele
distribution of onset of developmental delay between patients with and without variants in the promoter-region of their unaffected SCN1A allele
distribution of age at first afebrile seizure between patients with and without variants in the promoter-region of their unaffected SCN1A allele
distribution of IQ/DQ scores after five years of disease between patients with and without variants in the promoter-region of their unaffected SCN1A allele
Figure R8 Family Tree I-IV. Orange box indicates mild epilepsy phenotype with normal cognitive functioning. Light red box indicates a mild DS, or borderline DS/GEFS+ phenotype. Dark red boxes indicate severe DS. Numbers in the boxes correspond to promoter haplotype number or wildtype haplotype from Figure R1.
Our experiments showed that the presence of common variants in the promoterregion of SCN1A cause a significant decrease in luciferase activity, compared to the wildtype promoter. This indicates that SCN1A expression and function may be negatively influenced by such variants, likely due to disturbance of RNA polymerase II and/or transcription factor binding. Although this reduced expression cannot cause epilepsy independently, since a large part of the healthy populations carries these common variants as well, it may modulate the effect of other variants that are present. Our results can only in part be compared to those of Gao et al. (Huang et al., 2014), who found no differences in expression between the most common promoter-haplotypes. These different results may be attributed to three factors.
First, we are measuring a different group of common variants, which results in different expression levels. Second, we have cloned a slightly altered promoter region that is shorter on the 5’ side, but extended on the 3’ side to include the complete h1u. H1u, the first 5’ UE contains transcription factor binding sites such as EBF and the Initiator element that is required to form the transcription complex. Third, we use the Promega dual-assay luciferase plasmid, which has both the renilla and firefly luciferase gene incorporated. In single-assay luciferase assays, using two plasmids, normalization of luciferase data could be less sensitive. In general, the luciferase reporter assay is currently the fastest tool to measure gene expression at the transcriptional level.
Nevertheless, it should be noted that in-vitro assays can never fully mimic an in-vivo state, especially in complex structures such as the brain. While for this study a neuronal cell line was used to perform the expression studies, this can be improved by introducing the luciferase constructs in the brain of an animal model. In this way, the interactions between cell types in the brain are included, approaching the in-vivo state more accurately. Also, SCN1A has two promoters which are consecutively active and five 5’ UE’s currently known, adding up to the difficulty of interpreting SCN1A expression. Nevertheless, we found that a combination of common and rare variants in the SCN1A promoter 1 regionand the first 5’ UE h1u, reduced expression on transcription level. The reduced luciferase expression was in line with our hypothesis that common a set of SCN1A variants may affect expression of the gene and thus lead to more severe phenotypes, when present on the unaffected SCN1A allele of a Dravet syndrome patient. However, the clinical consequences of these different haplotypes were less convincing: no statistically significant differences were seen between patients with and without the common promoter variants, although we did observe a minor trend of more severe outcomes on multiple clinical variables in patients with common promoter variants. There may be several reasons for this. First, it is likely that common variants in the promoter region only have small phenotypic effects, since they otherwise would have been subject to negative selection. This limited effect was also illustrated by Gao et al. (Gao et al., 2017); although a pathogenic point mutation in the SCN1A promoter -region led to an in vitro decrease of expression and mild epilepsy in a proband, the same variant was found in the asymptomatic mother of the patient. This indicates that promotervariants by themselves may only have a limited influence on phenotypes. To detect such small effects, large sample sizes are prerequisite. Our study sample is likely too small to reliably detect any phenotypic consequences. Second, other (stronger) modifiers may simultaneously modulate the effect of promoter-variants. Although we excluded patients with mosaic pathogenic variants, we cannot eliminate the influence of variants in modifier genes and environmental factors on outcomes. If these other factors are strong influencers, they may override any effects the promoter-variants have.
Besides a small sample size, our study has several other limitations. While the luciferase plasmids were fully sequenced, the SCN1A patient promoter haplotypes were reconstructed based on three common SNPs. No sequencing of the complete promoter-region was performed in the participants. The patients’ haplotypes may therefore not fully correspond to the haplotypes tested during the luciferase experiments. Theoretically, patients may harbor additional promoter-variants that could either rescue or aggravate impaired expression. This could have large effects on outcomes in a sample size as small as ours. Furthermore, different primary pathogenic SCN1A variants may influence outcomes; however, a trend for milder phenotypes in patients with wildtype promoters was seen for the group of patients with truncating mutations only as well, which indicates that this effect is limited.
We also analyzed four families of which multiple members were affected by the same pathogenic SCN1A variant, but showed different phenotypes nonetheless; in these cases the effect of the primary mutation on the resulting phenotype is expected to be equal. Since in three of the four families both members had variant-haplotypes, our hypothesis could not explain their phenotypic differences. This is however not surprising, since in two of these families both members were affected by different clinical syndromes; as stated before, the modifying effect of promoter-variants is likely not strong enough to cause this independently. In only one family, consisting of two brothers with Dravet syndrome, the milder brother carried a wildtype promoter on his unaffected allele, whereas the more severe brother carried a variant-promoter. According to our hypothesis, this might explain their phenotypic differences; however, as mentioned previously, we cannot exclude other influencers and definitive conclusions are not possible based on only one family.
In conclusion, we found that common variants in the SCN1A promoter reduce transcription in neuronal cell culture, which may indicate that promoter haplotypes can act as a disease modifier in epilepsy. We however only found a small, nonsignificant effect of the SCN1A promoter on clinical outcomes of Dravet syndrome patients. These results are inconclusive due to a limited detection power; however, the observed trends in our cohort warrant replication studies in larger cohorts to explore the potential modifying role of these common SCN1A promoter-haplotypes. The inclusion of large numbers of Dravet syndrome patients, ideally all with similar primary LoF variants, is essential to detect the likely small effect these haplotypes might have on phenotypes. Sequencing of both complete SCN1A promoterregions, preferably including the 5’-UEs, would be required to obtain conclusive results.
We thank Dr. Vamshidhar R. Vangoor for leading the luciferase read-out experiments. This study was supported by the “Stichting Vrienden WKZ” (project 1614054) on behalf of Stichting Panta Rhei, and the Dutch Epilepsy Foundation (project 2017-01). MMC has
received funding from the European Union’s Horizon 2020 research and innovation programme under the Marie Skłodowska-Curie grant agreement No 751761.
The study was approved by the Ethical Committee of the University Medical Center Utrecht. Informed consent was obtained from participants or their legal caretakers according to the Declaration of Helsinki.
A cohort of 143 participants with SCN1A pathogenic variants was evaluated, of which most have previously been described (de Lange, Gunning, et al., 2018; de Lange, Koudijs, et al., 2018). Only participants with pathogenic variants (class V) or likely pathogenic variants (class IV) in SCN1A were included, according to the American College of Medical Genetics and Genomics criteria (Richards et al., 2015).
All variants had been detected and classified in genetic diagnostic laboratories.
Patients who had previously been shown to be mosaic (n=4) for their pathogenic SCN1A variant were excluded from analyses, as mosaicism may greatly influence outcomes (de Lange, Koudijs, et al., 2018). Our cohort comprised patients with Dravet syndrome, GEFS+, febrile seizures and also four participants who had been seizurefree their entire lives, but did have a child with Dravet syndrome that carried the same pathogenic SCN1A variant. Dravet syndrome was diagnosed based on previously published criteria (Verbeek et al., 2013) and in line with recently published recommendations (Wirrell et al., 2017). Our main statistical analyses of clinical outcomes were performed on patients with Dravet syndrome only. Non-Dravet syndrome patients remained included in the molecular analyses to separately investigate whether different promoter haplotypes could explain the inter-familial phenotypic variability of Dravet syndrome patients and their more mildly affected family members.
Detailed clinical data were collected from medical records for all participants, and a semistructured telephone interview was conducted when possible (n=130). A classification of the developmental outcome was made, rated in a consensus meeting by a child neurologist, neuropsychologist, and clinical geneticist. Developmental outcome was rated on a five-point scale based on available data on IQ and developmental level (1= no ID (IQ or developmental quotient (DQ) >85), 2 = borderline ID (IQ or DQ 70-85), 3 = mild ID (IQ or DQ 50-70), 4= moderate ID (IQ or DQ 30-50), 5 = severe or profound ID (IQ or DQ <30)). When no (recent) IQ or DQ was available, the assessment was made based on school functioning, communication
and adaptive behavior. Furthermore, approximated IQ/DQ scores after five years of disease were calculated, to obtain a cognitive outcome measurement unaffected by the influence of the different ages at assessment of the participants. For this, all IQ- and developmental assessment scores of each patient, conducted at different ages, were interpolated by linear regression as previously described (de Lange, Gunning, et al., 2018). When the first official assessment was made later than five years after seizure onset we used the age at which a developmental delay was first observed (by either parents or clinicians) as the first moment of decline, and IQ/DQ scores up until that age were estimated to be average (=100).
The SCN1A promoter 1 region including h1u (Homo sapiens chromosome 2, GRCh38. p12: 166148836 to 166151403 ) was PCR amplified from human control DNA using primers with a 15bp extension arm used for cloning. Five different haplotypes of 2568bp were selected and ligated in Psicheck-2 plasmids using In-Fusion cloning (clontech). The Psicheck-2 plasmid enables dual-reporter luciferase read-out as it carries both the renilla (Renilla reniformis derived) and firefly (Photinus pyralis derived) luciferase genes. Firefly luciferase is expressed by the HSV-TK promoter and can therefore be used as a control, while Renilla luciferase is controlled by the SCN1A promoter. As both genes are present on the same plasmid, unlike single reporter luciferase systems that require the transfection of two plasmids, the normalization and therefore read-out is more accurate. Plasmids were subsequently sequenced to confirm the haplotypes. SH-SY5Y cells were seeded in 24-well cell culture plates until 80% confluency was reached. Psicheck-2 plasmids carrying the SCN1A promoter haplotypes were transfected using polyethylenimine. After 48 hours, cells were lysed and the lysate transferred to a white opaque 96well plate in which the luciferase recording took place. Firefly and Renilla luciferase activities were detected using the dual luciferase reporter assay system (Promega) in the Varioskan FLASH luminometer (Thermo Fisher Scientific). Read-out was performed twice as a technical replicate, and averaged values were taken as final mean. Luciferase experiments were replicated 8 times. Differences in expression between haplotypes was not normally distributed and therefore analyzed using the Mann-Whitney U-test. For primer sequences see supplementary data
SCN1A was re-analysed in all participants as previously described (de Lange, Koudijs, et al., 2018). In short, all SCN1A exons were captured by single molecule molecular inversion probes (smMIPs) and sequenced on a NextSeq500 (Illumina, San Diego, CA). The resulting data were analyzed using commercial software (SeqNext module of Sequence Pilot; JSI medical systems, Ettenheim, Germany). Reads with the same single-molecule tag were assembled into one consensus read, to correct for PCR and sequencing artefacts. SCN1A pseudogene reads were removed from
alignment and analysis. The used smMIP design included the 5’ promoter region to capture three common promoter-variants (-1964 (rs2212657), -1036 (rs4319946) and-52 (rs16851666)). The promoter-haplotypes of the unaffected SCN1A allele of each patient was reconstructed based on the genotypes on these positions when possible. Direct assignment of genotypes to the unaffected allele was only possible in the case of homozygous genotypes, when the same genotype is present on both alleles. In the case of heterozygous genotypes, assignment of genotypes to the affected and unaffected alleles was only possible if the following condition was met: the participant had an affected family member with a homozygous genotype at the same position, with whom they shared the same inherited pathogenic SCN1A variant. If so, the genotype present on the shared, affected allele is known and the genotype of the unaffected allele can be deducted. When the genotypes of the unaffected allele on all three positions could be reconstructed, one of the five described haplotypes could be matched and assigned.
A recent genome-wide association study (GWAS) of the epilepsies identified a strong association with SNPs in SCN1A (manuscript in press). We analyzed the association of the three common promoter variants with epilepsy using data from the latest epilepsy GWAS. We tested for independent associations of our promoter variants with epilepsy by performing a linear regression on each variant while conditioning on the most significant SCN1A-SNP (rs6432877) from the GWAS. Conversely, we then tested to see if the GWAS association with SCN1A could be explained by our promoter SNPs by conditioning in the opposite direction.
Differences in clinical features and outcomes between Dravet syndrome patients with and without common variants in the SCN1A promoter-region on their unaffected allele were investigated. Ordinal regression, corrected for age, was used to investigate cognitive outcome scores; the Mann-Whitney U test was used to investigate age at seizure onset, age at first notice of developmental delay, age at first afebrile seizure and interpolated IQ/DQ scores after 5 years of disease. A similar analysis was performed for Dravet syndrome patients with non-mosaic truncating pathogenic variants only, to limit the influence of different pathogenic SCN1A variants themselves on the results. All reported tests were performed 2-tailed with an alpha-level for significance of p <0.05. We furthermore separately investigated whether family members, that carry the same pathogenic SCN1A variant but show
varying disease severities, may have different promoter-haplotypes that could explain their different outcomes.
Supplementary data can be found in the online version of this publication.
Akiyama, M., Kobayashi, K., Yoshinaga, H., & Ohtsuka, Y. (2010). A long-term follow-up study of Dravet syndrome up to adulthood. Epilepsia, 51(6), 1043–1052. http://doi.org/10.1111/j.1528-1167.2009.02466.x
Anney, R. J. L., Avbersek, A., Balding, D., Baum, L., Becker, F., Berkovic, S. F., Zimprich, F. (2014). Genetic determinants of common epilepsies: A meta-analysis of genome-wide association studies. The Lancet Neurology, 13(9), 893–903. http://doi.org/10.1016/S14744422(14)70171-1
Brunklaus, A., Ellis, R., Reavey, E., Forbes, G., & Zuberi, S. (2012). Prognostic, clinical and demographic features in SCN1A mutationpositive Dravet syndrome. Brain, 135(8), 2329–36.
Catterall, W. A., Kalume, F., & Oakley, J. C. (2010). Na V 1.1 channels and epilepsy. The Journal of Physiology, 588(11), 1849–1859. http://doi. org/10.1113/jphysiol.2010.187484
Ceulemans, B. (2011). Overall management of patients with Dravet syndrome. Developmental Medicine and Child Neurology, 53(SUPPL. 2), 19–23. http://doi.org/10.1111/j.14698749.2011.03968.x
de Lange, I. M., Gunning, B., Sonsma, A. C. M., van Gemert, L., van Kempen, M., Verbeek, N. E., Brilstra, E. H. (2018). Influence of contraindicated medication use on cognitive outcome in Dravet syndrome and age at first afebrile seizure as a clinical predictor in SCN1A -related seizure phenotypes. Epilepsia, 59(6), 1154–1165. http://doi.org/10.1111/epi.14191
de Lange, I. M., Koudijs, M. J., van ’t Slot, R., Gunning, B., Sonsma, A. C. M., van Gemert, L. J. J. M., … Koeleman, B. P. C. (2018). Mosaicism of de novo pathogenic SCN1A variants in epilepsy is a frequent phenomenon that correlates with variable phenotypes. Epilepsia, (January), 1–14. http://doi.org/10.1111/epi.14021
Depienne, C., Trouillard, O., Gourfinkel-An, I., Saint-Martin, C., Bouteiller, D., Graber, D., … LeGuern, E. (2010). Mechanisms for variable expressivity of inherited SCN1A mutations causing Dravet syndrome. Journal of Medical Genetics, 47(6), 404–410. http://doi.org/10.1136/
jmg.2009.074328
Djémié, T., Weckhuysen, S., von Spiczak, S., Carvill, G. L., Jaehn, J., Anttonen, A.-K., … Suls, A. (2016). Pitfalls in genetic testing: the story of missed SCN1A mutations. Molecular Genetics & Genomic Medicine, 4(4), 457–464. http://doi. org/10.1002/mgg3.217
Dravet, C. (1978). Les epilepsies graves de l’enfant. Vie Med, 8(2), 543–548. Dravet, C. (2011). The core Dravet syndrome phenotype. Epilepsia, 52(SUPPL. 2), 3–9. http:// doi.org/10.1111/j.1528-1167.2011.02994.x
Escayg, A., & Goldin, A. (2010). Sodium channel SCN1A and epilepsy: mutations and mechanisms. Epilepsia, 51(9), 1650–1658. http:// doi.org/10.1111/j.15281167.2010.02640.x.Sodium
Escayg, A., MacDonald, B. T., Meisler, M. H., Baulac, S., Huberfeld, G., An-Gourfinkel, I., Malafosse, A. (2000). Mutations of SCN1A, encoding a neuronal sodium channel, in two families with GEFS+2. Nature Genetics, 24, 343–345. http://doi.org/10.1038/nature4441023a
Gao, Q. W., Hua, L. D., Wang, J., Fan, C. X., Deng, W. Y., Li, B., … Shi, Y. W. (2017). A Point Mutation in SCN1A 5′ Genomic Region Decreases the Promoter Activity and Is Associated with Mild Epilepsy and Seizure Aggravation Induced by Antiepileptic Drug. Molecular Neurobiology, 54(4), 2428–2434. http://doi.org/10.1007/ s12035-016-9800-y
Gennaro, E., Santorelli, F. M., Bertini, E., Buti, D., Gaggero, R., Gobbi, G., Zara, F. (2006). Somatic and germline mosaicisms in Severe Myoclonic Epilepsy of Infancy. Biochemical and Biophysical Research Communications, 341(2), 489–493. http://doi.org/10.1016/j. bbrc.2005.12.209
Gitiaux, C., Chemaly, N., Quijano-Roy, S., Barnerias, C., Desguerre, I., Hully, M., … Nabbout, R. (2016). Motor neuropathy contributes to crouching in patients with Dravet syndrome. Neurology, 87(3), 277–281. http://doi.org/10.1212/ WNL.0000000000002859
Guerrini, R., Cellini, E., Mei, D., Metitieri, T., Petrelli, C., Pucatti, D., … Zamponi, N. (2010). Variable epilepsy phenotypes associated with
of
a familial intragenic deletion of the SCN1A gene. Epilepsia, 51(12), 2474–2477. http://doi. org/10.1111/j.1528-1167.2010.02790.x
Guerrini, R., Dravet, C., Genton, P., Belmonte, A., Kaminska, A., & Dulac, O. (1998). Lamotrigine and seizure aggravation in severe myoclonic epilepsy. Epilepsia, 39(5), 508–512. http://doi. org/10.1111/j.1528-1157.1998.tb01413.x
Harkin, L. A., McMahon, J. M., Iona, X., Dibbens, L., Pelekanos, J. T., Zuberi, S. M., … Wirrell, E. (2007). The spectrum of SCN1A-related infantile epileptic encephalopathies. Brain, 130(3), 843–852. http://doi.org/10.1093/brain/ awm002
R. (2006). Mosaic SCN1A mutation in familial severe myoclonic epilepsy of infancy. Epilepsia, 47(10), 1737–1740. http://doi.org/10.1111/j.1528-1167.2006.00675.x
Martin, M. S., Tang, B., Ta, N., & Escayg, A. (2007). Characterization of 5′ untranslated regions of the voltage-gated sodium channels SCN1A, SCN2A, and SCN3A and identification of cisconserved noncoding sequences. Genomics. http://doi.org/10.1016/j.ygeno.2007.04.006
on the
Huang, A. Y., Xu, X., Ye, A. Y., Wu, Q., Yan, L., Zhao, B., Wei, L. (2014). Postzygotic single-nucleotide mosaicisms in whole-genome sequences of clinically unremarkable individuals. Cell Research, 24(11), 1311–1327. http://doi. org/10.1038/cr.2014.131
Jansen, F., Sadleir, L., Harkin, L., Vadlamudi, L., McMahon, J., Mulley, J., Berkovic, S. (2006). Severe myoclonic epilepsy of infancy (Dravet syndrome): recognition and diagnosis in adults. Neurology, 67(12), 2224–6.
Kasperavičiute, D., Catarino, C. B., Matarin, M., Leu, C., Novy, J., Tostevin, A., Sisodiya, S. M. (2013). Epilepsy, hippocampal sclerosis and febrile seizures linked by common genetic variation around SCN1A. Brain, 136(10), 3140–3150. http://doi.org/10.1093/brain/awt233
Long, Y. S., Zhao, Q. H., Su, T., Cai, Y. L., Zeng, Y., Shi, Y. W., Liao, W. P. (2008). Identification of the promoter region and the 5’-untranslated exons of the human voltagegated sodium channel Nav1.1 gene (SCN1A) and enhancement of gene expression by the 5’untranslated exons. Journal of Neuroscience Research, 86(15), 3375–3381. http://doi.org/10.1002/jnr.21790
Mahoney, K., Moore, S. J., Buckley, D., Alam, M., Parfrey, P., Penney, S., … Young, T. L.
(2009). Variable neurologic phenotype in a GEFS+ family with a novel mutation in SCN1A. Seizure, 18(7), 492–497. http://doi.org/10.1016/j. seizure.2009.04.009
Marini, C., Mei, D., Helen Cross, J., & Guerrini,
Meng, H., Xu, H. Q., Yu, L., Lin, G. W., He, N., Su, T., Liao, W. P. (2015). The SCN1A Mutation Database: Updating Information and Analysis of the Relationships among Genotype, Functional Alteration, and Phenotype. Human Mutation, 36(6), 573–580. http://doi.org/10.1002/ humu.22782
Nakayama, T., Ogiwara, I., Ito, K., Kaneda, M., Mazaki, E., Osaka, H., Yamakawa, K. (2010). Deletions of SCN1A 5′ genomic region with promoter activity in dravet syndrome. Human Mutation, 31(7), 820–829. http://doi. org/10.1002/humu.21275
Parihar, R., & Ganesh, S. (2013). The SCN1A gene variants and epileptic encephalopathies. Journal of Human Genetics, 58(9), 573–580. http://doi.org/10.1038/jhg.2013.77
Passamonti, C., Petrelli, C., Mei, D., Foschi, N., Guerrini, R., Provinciali, L., & Zamponi, N. (2015). A novel inherited SCN1A mutation associated with different neuropsychological phenotypes: Is there a common core deficit? Epilepsy and Behavior, 43, 89–92. http://doi.org/10.1016/j. yebeh.2014.11.009
Pineda-Trujillo, N., Carrizosa, J., Cornejo, W., Arias, W., Franco, C., Cabrera, D., RuízLinares, A. (2005). A novel SCN1A mutation associated with severe GEFS+ in a large South American pedigree. Seizure, 14(2), 123–128. http://doi. org/10.1016/j.seizure.2004.12.007
Richards, S., Azi, N., Bale, S., Bick, D., Das, S., Gastier-Foster, J., Rehm, H. L. (2015).
Standards and Guidelines for the Interpretation of Sequence Variants: A Joint Consensus Recommendation of the American College of Medical Genetics and Genomics and the Association for Molecular Pathology. Genetics
in Medicine, 17(5), 405–424. http://doi. org/10.1038/gim.2015.30.Standards
Rilstone, J. J., Coelho, F. M., Minassian, B. A., & Andrade, D. M. (2012). Dravet syndrome: Seizure control and gait in adults with different SCN1A mutations. Epilepsia, 53(8), 1421–1428.http:// doi.org/10.1111/j.1528-1167.2012.03583.x
Suls, A., Velizarova, R., Yordanova, I., Deprez, L., Van Dyck, T., Wauters, J., … De Jonghe, P. (2010). Four generations of epilepsy caused by an inherited microdeletion of the SCN1A gene. Neurology, 75(1), 72–76. http://doi.org/10.1212/ WNL.0b013e3181e62088
Verbeek, N. E., Maas, N. A. T. Van Der, Jansen, F. E., Kempen, M. J. A. Van, Lindhout, D., &
Brilstra, E. H. (2013). Prevalence of SCN1A-Related Dravet Syndrome among Children
Reported with Seizures following Vaccination : A Population-Based Ten-Year Cohort Study, 8(6). http://doi.org/10.1371/journal.pone.0065758
Wirrell, E. C., Laux, L., Donner, E., Jette, N., Knupp, K., Meskis, M. A., … Berg, A. T. (2017). Optimizing the Diagnosis and Management of Dravet Syndrome: Recommendations From a North American Consensus Panel. Pediatric Neurology, 68, 18–34.e3. http://doi.org/10.1016/j. pediatrneurol.2017.01.025
Novel genome editing and transient gene therapies have been developed the past ten years, resulting in the first in-human clinical trials for monogenic disorders. Syndromic autism spectrum disorders can be caused by mutations in a single gene. Given the monogenic aspect and severity of syndromic ASD, it is an ideal candidate for gene therapies. Here, we selected 11 monogenic ASD syndromes, validated by animal models, and reviewed current gene therapies for each syndrome. Given the wide variety and novelty of some forms of gene therapy, the best possible option must be decided based on the gene and mutation.
At present, gene therapies are on the rise (Figure 1). The first approved gene therapies for human diseases are now being used in the clinic, and we are experiencing an exponential rise in putative gene therapies in development [1,2]
Figure 1 Gene Therapies for Syndromic Autism Spectrum Disorders. Left; transient gene therapies such as antisense ol-igonucleotides (ASO), noncodingRNA (ncRNA), RNA editing and gene delivery.
Right; Permanent gene therapies inlcude those that edit the host cell genome such as gene replacement (aimed at integration in the genome), CRISPR-KO (for targeted genomic disruptions) and Gene editing (for targeted repair of disease-causing mutations). Red and blue nucleotides display synthetic RNA or DNA, respectively. Green nucleotides display host-cell endoge-nous RNA or DNA. Created with BioRender.com SV: Structural variants ASO: Antisense oligonucleotide, GD: Gene delivery, RE: RNA editing, GR: Gene replacement, KO: CRISPR-KO, GE: Gene editing.
A substantial proportion is developed by the pharmaceutical industry, including an equally increasing number by new start-up companies, involving investments of millions of dollars. Therefore, it is expected that gene therapies will become a possibility for a large range of diseases, including autism. Most current gene therapies are focused on monogenic diseases with a strong penetrant single genetic cause that is well understood in terms of disease mechanism. For autism, the development is centered around the monogenic forms. Intensive genetic studies have confirmed that autism spectrum disorder (ASD) has a strong genetic basis that shows a high degree of genetic heterogeneity [3]. The first important distinction is that ASD can be either the sole clinical phenotype or syndromic. In the latter case, ASD is a symptom of a developmental disorder that includes multiple phenotypes, such as epilepsy, intellectual disability and dysmorphic features [4] For non-syndromic ASD, there is genetic evidence for a polygenic or multifactorial genetic architecture, and it is expected that the risk for disease is determined by a combination of multiple environmental and genetic factors [5]
Nevertheless, single gene mutations have also been found that can explain disease in the relatively benign form of sporadic non-syndromic ASD. In contrast, syndromic ASD is typically more severe, and an underlying single genetic cause is known in most cases. It is for this category of ASD that gene therapy is most promising, and for which the first gene therapies are now in an advanced stage of development (Table 1).
All known mutations that cause syndromic ASD lead to loss of protein function, meaning that therapies should aim at upregulation, enhancement or stabilization
of healthy gene products in order to compensate for the mutation effect. There are several technologies that can achieve this, which we separated in two categories: (1) those that do not modify the host cell genome and (2) those that do (Figure 1). Several technologies have been tested to treat the effects of monogenic ASD mutations in a preclinical experimental setting (Table 1), paving the way for gene therapies. In this review, we provide an overview of current gene therapies in development for 11 monogenic autism syndromes, which were chosen based on previous clinical publications and validation by animal models. We describe the different types and mechanisms of gene therapies and discuss how they can be applied to the different types of genetic autism.
Current transient gene therapies that do not edit the genome are based on noncoding RNA (ncRNA), antisense oligonucleotides (ASO), RNA editing and gene delivery (Figure 1). NcRNA therapies involve various technologies that use different types of small RNA, including small interfering RNA (siRNA), microRNA (miRNA), short hairpin RNA (shRNA), circular RNAs (circRNA), small activating RNA (saRNA) and SINEUPs. Many of these RNAs act through the RNA interference (RNAi) pathway and aim to reduce gene expression at the transcriptional level, by binding to their premRNA or mRNA targets and thus labeling them for degradation [23]. Consequently, these ncRNA-based drugs are aimed at gene silencing which it not applicable for LoF mutations in monogenic ASD, although there are a few exceptions. A possibility is to use RNAi techniques to inhibit the inhibitor. For example, regulatory genes or transcription factors that inhibit expression of the target gene can be inhibited using RNAi to increase expression of the target. A putative example exists for SCN1A, involved in developmental epilepsy and associated with ASD. Expression of SCN1A was shown to be negatively regulated by modifier genes RACK1 and MDH2, and silencing of these genes led to SCN1A upregulation [24,25]. Unfortunately, little is known about gene regulation and side-effects of regulatory gene intervention for most monogenic ASD genes, providing an opportunity for further investigation.
SaRNA, circRNA and SINEUP are more novel ncRNA technologies and aimed at increasing gene expression, although at different levels. saRNAs are small, doublestranded RNAs that can be designated to regulatory and promoter regions in the genome where they can enhance transcription by altering the chromatin state, although the exact mecha-nism is still under investigation [26]. CircRNA are circular single-stranded RNA mole-cules that can be designed to bind microRNAs, which would normally negatively regulate target mRNA. By binding to miRNA, its inhibition on mRNA is lost, leading to the upreg-ulation of the target gene. A single circRNA can have more than 50 miRNA binding sites, allowing strong levels of gene regulation [28]. SINEUP are antisense long noncoding RNAs that bind to 5′ untranslated regions of mRNA, stabilizing the molecule and pre-venting it from being degraded. SINEUP
thus act on the post-transcriptional level and lead to protein but not mRNA upregulation of target genes [28]. Each of these expression-enhancing ncRNAs are relatively new and no developments have been achieved for monogenic ASD genes so far.
In contrast to ncRNA, antisense oligonucleotides (ASO) are in a more advanced stage of development. ASO are noncoding single-stranded DNA, RNA or DNA–RNA hybrid molecules, less than 50 bp in size and can be designed for various mechanisms of action [29]. In the simplest approach, comparable to ncRNA, an ASO can reduce the expression of a target gene by binding to the mRNA. The mechanism of action involves the RNAse H1 machinery that catalyzes cleavage of the mRNA upon binding of an ASO ssDNA molecule. Such an approach has been designed and tested to target toxic CGG repeats in the FMR1 gene. Healthy individuals can have several CGG repeats in this region, but when this number exceeds 55, patients develop fragile X associated tremor/ataxia syndrome (FXTAS) or fragile X syndrome (FXS) when the repeat number exceeds 200. By designing an ASO that targets toxic CGG repeats in the FMR1 mRNA, the amount of disease-causing transcripts was reduced in vitro and in vivo [11].
A variation of this approach is used for Angelman syndrome (AG), caused by structural variants in the 15q11-13 locus, often leading to complete loss of the UBE3A gene, other LoF mutations in UBE3A or imprinting errors leading to inactivation of the maternal copy of the gene. In healthy individuals, the paternal copy of the UBE3A gene is imprinted, leading to a maternal-specific expression, which, in the case of AS, is abolished by LoF mutations. The imprinting of the paternal allele of UBE3A is regulated by a paternally ex-pressed UBE3A antisense transcript (UBE3AAS). Therefore, an ASO targeted to this natu-ral antisense transcript was able to unsilence the paternal allele, restoring normal expres-sion of UBE3A in vitro and in vivo [6]. The ASO that targets UBE3A-ATS is under devel-opment by two pharmaceutical companies and currently in phase 1 or 2 clinical trials [29]
ASO can also be designed to affect pre-mRNA splicing. A recent in silico prediction study on 8000 protein coding genes, including those covered in this review, showed that alternative splicing, which usually leads to nonproductive protein, can be prevented by ASO increasing the chance of normal splicing, thereby increasing the levels of functional protein. Several genes were validated in vitro, including SYNGAP1, for which mutations lead to syndromic ASD. The ASO was designed to bind the 3′ splice site between exon 10 and 11, thereby splicing out an alternative exon. Both mRNA and protein levels of SYN-GAP1 were increased in vitro [15]. Finally, ASO can also act on the level of protein transla-tion by blocking translation from alternative translation initiation sites such as upstream open reading frames (uORF). Binding to uORF by ASO blocks its access to the translation machinery, thereby favoring the use of the original ORF to increase productive protein [30]. Several other mechanisms
by which ASO can be used to compensate for LoF muta-tions are explored, but are not yet fully studied for monogenic ASD.
The term gene delivery is used for delivery of healthy genes in the form of complementary DNA (cDNA) as extrachromosomal DNA (ecDNA), or extrachromosomal circularDNA (eccDNA) to the host cell. It is to some extend comparable to gene replacement, but is not aimed at alterations of the host cell genome. Gene delivery can theoretically be applied to all LoF mutations, but is the candidate approach for structural deletions of large size, which are the underlying cause for AG, Phelan–McDermid syndrome (PMD) and NRXN1-related monogenic ASD. By introducing an additional copy of the gene that is lost, the effect of the mutation can to some extend be compensated. Gene delivery has already been tested for FXS [12], AG [7], Rett syndrome (RTT) [8,9] and Tuberous sclerosis complex (TBC) [13], both in vitro and in vivo, highlighting that this type of gene therapy covers the majority of monogenic ASD syndromes. For each of the above-mentioned syndromes, cDNA of the corresponding mutated gene was delivered using recombinant adeno-associated viral vectors (rAAVs). rAAV infects the host cell with the additional gene copy, which localizes to the nucleus where it is transcribed from the cell-native transcrip-tion machinery. While the majority of rAAV-mediated delivered cDNAs reside as ecDNA in the nucleus, rare integration events occur as well, which seem to be concentrated in regions with transcriptionally active genes, CpG-islands and chromosomal breakage sites [31] While the chance of random integration is much lower than the chance that cDNA remains in the nucleus as ecDNA, this could still pose a risk of disrupting otherwise nor-mal functioning genes or regulatory regions. In addition, to maintain correct regulation, promoter type and perhaps even regulatory regions such as 5′ and 3′ UTR should be considered flanking the cDNA, although this is not yet standard practice. EcDNA introduced by rAAV is typically lost after several cell divisions, but can remain present in mature neurons for several years [32], meaning that for monogenic ASD, gene delivery could become a long-lasting therapeutic solution.
Finally, programmable RNA editing is a developing field that allows the correction of disease-causing mutations at the transcriptome level, leaving the genome intact. Current RNA editors are based on adenosine deaminases acting on RNA (ADAR), which allow adenosine to inosine transversions (‘A-to-I editing’) [33]. Inosine is typically recognized as guanine by the translation machinery, allowing targeted A > G editing in mRNA transcripts. For monogenic ASD, RNA editing has already been tested for RTT, which is caused by mutations in the MECP2 gene. The R106Q mutation, which normally leads to RTT, was removed in the mRNA transcript by reversing the G > A motif at position 317 in an RTT mouse model [10]. After one month, mice treated with RNA editing showed 50% edited MECP2 mRNA in hippocampal neurons, highlighting that this type of gene therapy is both effective in vivo an in vitro
The above technologies have in common that the host cell genome is not edited, which may alleviate concerns about safety, but therefore require repeated dosing throughout life. Repeated dosing provides a degree of control over adverse effects but pos-es a significant burden for the patient. More seriously, adverse effects such as immune re-actions to both viral and non-viral delivery vehicles remain a concern.
The second category of gene therapies includes those with the aim of permanently changing the host genome. Current technologies are gene replacement, gene editing and CRISPR-KO. Gene replacement aims to introduce an additional cDNA copy to compen-sate for LoF mutations by focusing on integrating the cDNA copy into the host cell genome to maintain a lifelong stable expression. Currently, lentiviral vectors (LV) are the method of choice to deliver exogenous cDNA to the host cell. LV infection is followed by insertion of cDNA into the genome by the integrase enzyme, leading to long-term stable expression in both dividing and non-dividing cells [34]. An example of gene replacement for monogenic ASD involves the delivery and replacement of Ube3a by an LV in a mouse model of AS. As discussed above, AS is caused by mutations that cause loss of function of the only active maternal copy of the UBE3A gene. First, human haemopoietic stem cells were transduced with an LV containing the Ube3a gene, and subsequently engrafted in the AS mouse. Eight weeks after delivery, both neonate and adult mice showed normalized Ube3a levels in parallel to a phenotypic rescue [10]. As the animal model was successfully treat-ed in both developmental stages, the therapeutic window for gene replacement for AS might be broader than initially expected. Even if the AS mutation is already present and patients are treated at later stages in life, there might still be a possibility to halt or reverse disease prognosis using LVmediated gene replacement. Equal to the location of sporadic rAAV integration events, LV genomes are integrated at random positions in the genome and seem to be concentrated in active genes, which is undesired. Efforts to improve LV safety are ongoing and include engineered strains that do not longer integrate randomly, but only in so-called ‘safe harbor’ loci [35]
This year, the first in-human gene editing clinical trials started, highlighting that truly curative treatments are closer to becoming a reality. While initially, zincfinger nucleases (ZFN) and transcription activator-like effector nucleases (TALENs) were the method of choice to edit genomic DNA, they are now replaced by those based on CRISPR/Cas9 [36], which offers ease of design, development and increased efficacy. The most important techniques are CRISPR/Cas9-homology directed repair (CRISPR-HDR), base editing (BE) and prime editing (PE) [37]. In brief, CRISPRHDR was discovered in 2013 and is based on the Cas9 nuclease, which is guided by an RNA guide sequence (sgRNA) to the target genomic DNA. Here, a double-strand break (DSBs) is introduced at the mutation site, which in turn is repaired using a repair template DNA oligo with the correct edit via the homology-directed repair
(HDR) pathway. Dividing cells use both HDR and non-homologous end joining (NHEJ) to repair DSBs, but in non-dividing cells, NHEJ is preferred, limiting the application window of CRISPR-HDR [38]. Moreover, due to the DSBs, and the separately introduced HDR template, CRISPR-HDR can introduce random insertions and deletions (indels) at the DSBs site, which are undesired [39]. BE also uses an sgRNA and is based on the original Cas9 enzyme but engineered to create single-stranded ‘nicks’ in the DNA (Cas9n), fused to a deaminase enzyme. Currently, cytosine BE (CBE)- and adenosine BE (ABE) are available and allow C > T or A > G conversions, respectively [38]. ABE and CBE have much higher levels of correct editing and lower indel rates due to the absence of DSBs [37]. However, the 4–7 base pair (bp) editing window can lead to additional C and A bases to change as well, called ‘bystander edits’ [40]. Bystander edits, which are off-target mutations, can be tolerated in some cases if they do not lead to amino acid changes, or they can be ruled out the editing window consisting of a G/T-rich region. PE, the most recent version of CRISPR/Cas9 discovered in 2019, also uses Cas9n, but is fused to a reverse transcriptase (RT) enzyme and allows all four edits in addition to small insertions and deletions [41] . PE uses an sgRNA just like CRISPR-HDR and BE, but is extended at the 3′ site with a RT template and called PE guideRNA (pegRNA), which carries the correct edit [42] . As the pegRNA carries both the guiding sequence and the repair template, editing efficiency is much higher than CRISPRHDR and indel formation is reduced [38]. Given that BE and PE are relatively new, current gene editing therapies for monogenic ASD are based on CRISPR-HDR [39]. Two different mutations in MECP2 that cause RTT were corrected with CRISPR-HDR in induced pluripotent stem cells (iPSCs). Delivery was achieved via plasmid DNA (pDNA) transfection, wherein one plasmid carries the Cas9 enzyme and a second plasmid carries the sgRNA and HDR template. As pDNA transfection never reaches every cell of an in vitro population and edit efficiency of CRISPR-HDR is low, iPSCs were sorted based on fluorescence, leading to editing efficiencies of 20% for R270X [18] and 80% for T158M [19]. CRISPR-HDR was also used to correct the nonsense mutation R841X in SHANK2 [21], which leads to monogenic ASD. Using a comparable strategy, the R841X mutation was removed in iPSCs, showing proof of principle for gene editing for monogenic ASD. While BE and PE have not yet been tested for monogenic ASD mutations, there are reports that show their potential to cure other monogenic disorders [38,42]. In one study, BE was applied on a pathogenic mutation responsible for Hutchinson–Gilford Progeria syndrome (HGP). In an HGP mouse model, BE machinery was delivered using rAAV to correct the C > T mutation.
Additionally, early mice studies already show better editing precision by PE compared to CRISPR-HDR editing [43]. A challenge for CRISPR/Cas9 gene editing technologies is the size of the components, which limits delivery. While pDNA can be transfected to dividing cells to some extent and used for the creation of stable cell lines via FACS sorting, delivery to neurons is near impossible with pDNA. Since the gene editing field is rapidly developing, researchers have found ways to
overcome the size limitations, including the recently introduced downsized Cas9 and BE, which fit in a single rAAV, speeding up future gene editing efforts [44]
During the development of gene editing technologies, a simpler version of CRISPR/ Cas9 was discovered. This version, using the original Cas9 enzyme and an sgRNA (CRISPR-KO) without an HDR template, can be used to introduce targeted DSBs in the genome [45]. These DSBs can be repaired by the NHEJ pathway, which lead to indels resulting in genomic disruptions [36]. In contrast to gene editing, CRISPRKO is not aimed at repairing the disease-causing LoF mutation, but rather ‘slices out’ the mutation carrying region, resulting in indels. Two examples of CRISPR/ KO usage in a therapeutic setting are mutations in regions that tolerated indels, such as intronic mutations, or gain-of-function mutations that are more severe to protein functioning than a premature stopcodon. Another possible therapeutic mechanism via which CRISPR/KO can act is to compensate for the mutation effect by disrupting other regions in the genome such as transcription factors or modifier genes. CRISPR-KO, which is much easier to achieve as there is no need for precise editing, is already applied in human patients. For example, patients with transfusion-dependent β thalassemia (TDT) and sickle-cell disorder (SCD) caused by reduced levels of y-globulin can be treated by using CRISPR-KO targeted to a transcription factor named BCL11A [46]. By knocking out BCL11A, which negatively regulates yglobulin, patients with TDT and SCD show strong allelic editing levels after treatment in combination with myeloablation and increased fetal hemoglobin, improving their clinical picture. In addition to ex vivo studies that use extracted patient cells that are reintroduced after they have been treated with CRISPR-KO, the first in vivo CRISPR-KO clinical trials have started just recently. For the first time in humans, CRISPR-KO was injected directly in the retina of Leber congenital amaurosis 10 (LCA10) patients [47]. LCA10 is caused by mutations in the CEP290 gene, usually in exons. Some patients, however, show mutations in the intron between exon 26 and 27, which negatively affect splicing. By guiding CRISPR-KO with two sgRNAs to sites flanking the mutation, the complete muta-tion-carrying region can be removed, recovering correct splicing and resulting in a functional CEP290. The second in vivo CRISPR-KO clinical trial is aimed at knocking out a complete gene in patients with transthyretin amyloidosis (TTR). TTR is caused by misfolding of the TTR protein due to mutations in the TTR gene. By introducing DSBs in the coding region of TTR, overall TTR levels were reduced up to 28 days after transfusion [48]. For monogenic ASD, CRISPR/KO is also tested as a treatment for AG by knocking out the UBE3A antisense transcript (UBE3A-ATS), which silences transcription as outlined above. This strategy has been tested in mouse models for AG and the treatment was able to res-cue the disease phenotype [17]. Finally, for FXS, CRISPR-KO was used to introduce DSBs in the toxic CGG repeat expansion, recovering FMR1 expression in vitro, exemplifying another situation where CRISPR-KO can be employed with limited genotoxicity [20]. Still, CRISPR-KO can only be used to disrupt genomic regions, not to repair missense or trun-cating mutations, which are the majority of all monogenic
diseases including monogenic ASD. Therefore, both CRISPR-KO and gene editing via CRISPR-HDR, BE or PE should be considered based on the patient mutation.
Monogenic, syndromic ASD is a severe disease that is difficult to treat, warranting novel treatment. Gene therapies that permanently alter the genome can become a game-changing novel therapy for syndromic ASD, with the potential to alleviate the dis-ease symptoms with a few, or even single treatment. Gene therapies that have a transient effect, such as ASOs, ncRNA and RNA-editing leave the genome unedited and will require repeated dosing, but may have the advantage of being controllable and reversible. These differences highlight the important concerns of gene therapies, namely the issues of dos-ing, delivery and safety, which we did not discuss extensively here. Nevertheless, with the rapid pace of technological development comes improved viral and non-viral vectors in-cluding nanoparticles for delivery, more accurate gene editors that show little off-target effects, and controlled transgene expression. It is remarkable how rapidly precise gene editing of a single nucleotide gene mutation is approaching clinical application. For ASD, both transient and permanent gene therapies have already been tested invitro and in-vivo fast-fowarding translation to future medication. The type of gene therapy largely depends on its specific genetic cause, as structural variants require a different approach than missense mutations. Accurate DNA diagnostics and correct prediction of the functional con-sequences of the mutation will likely be equally important for the safe application of gene therapies in the future.
The authors would like to thank Professor K.P.J. Braun for his role in funding acquisition and supervision.
Writing — original draft preparation, W.W., J.G.; writing — review and editing, W.W., J.G., B.P.C.K.; visualization, W.W., J.G.; supervision, B.P.C.K.; project administration, B.P.C.K.; funding acquisition, B.P.C.K.
Benger, M., Kinali, M., & Mazarakis, N. D. (2018). Autism spectrum disorder: prospects for treatment using gene therapy. Molecular autism, 9, 39. https://doi.org/10.1186/s13229-018-0222-8
Lubroth, P., Colasante, G., & Lignani, G. (2021). In vivo Genome Editing Therapeutic Approaches for Neurological Dis-orders: Where Are We in the Translational Pipeline?. Frontiers in neuroscience, 15, 632522. https://doi.org/10.3389/fnins.2021.632522
Havdahl, A., Niarchou, M., Starnawska, A., Uddin, M., van der Merwe, C., & Warrier, V. (2021). Genetic contributions to autism spectrum disorder. Psychological medicine, 51(13), 2260–2273. https://doi.org/10.1017/S0033291721000192
Sztainberg, Y., & Zoghbi, H. Y. (2016). Lessons learned from studying syndromic autism spectrum disorders. Nature neuroscience, 19(11), 1408–1417. https://doi.org/10.1038/ nn.4420
Cheroni, C., Caporale, N., & Testa, G. (2020). Autism spectrum disorder at the crossroad between genes and environment: contributions, convergences, and interactions in ASD developmental pathophysiology. Molecular autism, 11(1), 69. https://doi.org/10.1186/s13229-020-00370-1
Meng, L.; Ward, A.J.; Chun, S.; Bennett, C.F.; Beaudet, A.L.; Rigo, F. Towards a therapy for Angelman syndrome by re-duction of a long non-coding RNA. Nature 2015, 518, 409–412, doi:10.1038/nature1397.
Derbis, M.; Kul, E.; Niewiadomska, D.; Sekrecki, M.; Piasecka, A.; Taylor, K.; Hukema, R.K.; Stork, O.; Sobczak, K. Short antisense oligonucleotides alleviate the pleiotropic toxicity of RNA harboring expanded CGG repeats. Nat. Commun. 2021, 12, 1–17, doi:10.1038/s41467-021-21021-w.
Lim, K.H.; Han, Z.; Jeon, H.Y.; Kach, J.; Jing, E.; Weyn-Vanhentenryck, S.; Downs, M.; Corrionero, A.; Oh, R.; Scharner, J.; et al. Antisense oligonucleotide modulation
of nonproductive alternative splicing upregulates gene expression. Nat. Commun. 2020, 11, doi:10.1038/s41467-020-17093-9.
Daily, J.L.; Nash, K.; Jinwal, U.; Golde, T.; Rogers, J.; Peters, M.M.; Burdine, R.D.; Dickey, C.; Banko, J.L.; Weeber, E.J. Adeno-associated virusmediated rescue of the cognitive defects in a mouse model for Angelman syndrome. PLoS One 2011, 6, doi:10.1371/journal.pone.0027221.
Luoni, M.; Giannelli, S.; Indrigo, M.T.; Niro, A.; Massimino, L.; Iannielli, A.; Passeri, L.; Russo, F.; Morabito, G.; Calamita, P.; et al. Whole brain delivery of an instability-prone Mecp2 transgene improves behavioral and molecular pathological defects in mouse models of Rett syndrome. Elife 2020, 9, 1–30, doi:10.7554/eLife.52629.
Sinnett, S.E.; Hector, R.D.; Gadalla, K.K.E.; Heindel, C.; Chen, D.; Zaric, V.; Bailey, M.E.S.; Cobb, S.R.; Gray, S.J. Improved MECP2 Gene Therapy Extends the Survival of MeCP2Null Mice without Apparent Toxicity after Intracisternal Delivery. Mol. Ther. - Methods Clin. Dev. 2017, 5, 106–115, doi:10.1016/j. omtm.2017.04.006.
Gholizadeh, S.; Arsenault, J.; Xuan, I.C. on. Y.; Pacey, L.K.; Hampson, D.R. Reduced phenotypic severity following adeno-associated virusmediated Fmr1 gene delivery in fragile X mice. Neuropsychopharmacology 2014, 39, 3100–3111, doi:10.1038/npp.2014.167.
Small, J.G.; Milstein, V.; Miller, M.J.; Sharpley, P.H.; Small, I.F. Neurophysiological evidence for actions of ECT. Psycho-pharmacol. Bull. 1988, 24, 391–395.
Cheah, P.S.; Prabhakar, S.; Yellen, D.; Beauchamp, R.L.; Zhang, X.; Kasamatsu, S.; Bronson, R.T.; Thiele, E.A.; Kwiatkow-ski, D.J.; Stemmer-Rachamimov, A.; et al. Gene therapy for tuberous sclerosis complex type 2 in a mouse model by de-livery of AAV9 encoding a condensed form of tuberin. Sci. Adv. 2021, 7, 1–14, doi:10.1126/sciadv.abb1703.
Sinnamon, J.R.; Kim, S.Y.; Fisk, J.R.; Song, Z.;
Nakai, H.; Jeng, S.; McWeeney, S.K.; Mandel, G. In Vivo Repair of a Protein Underlying a Neurological Disorder by Programmable RNA Editing. Cell Rep. 2020, 32, 107878, doi:10.1016/j.celrep.2020.107878
Adhikari, A.; Copping, N.A.; Beegle, J.; Cameron, D.L.; Deng, P.; O’Geen, H.; Segal, D.J.; Fink, K.D.; Silverman, J.L.; An-derson, J.S. Functional rescue in an Angelman syndrome model following treatment with lentivector transduced hema-topoietic stem cells. Hum. Mol. Genet. 2021, 30, 1067–1083, doi:10.1093/ hmg/ddab104.
Wolter, J.M.; Mao, H.; Fragola, G.; Simon, J.M.; Krantz, J.L.; Bazick, H.O.; Oztemiz, B.; Stein, J.L.; Zylka, M.J. Cas9 gene therapy for Angelman syndrome traps Ube3a-ATS long non-coding RNA. Nature 2020, 587, 281–284, doi:10.1038/ s41586-020-2835-2.
Lim, K.H.; Han, Z.; Jeon, H.Y.; Kach, J.; Jing, E.; Weyn-Vanhentenryck, S.; Downs, M.; Corrionero, A.; Oh, R.; Scharner, J.; et al. Antisense oligonucleotide modulation of nonproductive alternative splicing upregulates gene expression. Nature Communications 2020, 11, doi:10.1038/ s41467-020-17093-9
Xie, N.; Gong, H.; Suhl, J.A.; Chopra, P.; Wang, T.; Warren, S.T. Reactivation of FMR1 by CRISPR/ Cas9-mediated dele-tion of the expanded CGG-repeat of the fragile X chromosome. PLoS One 2016, 11, 1–12, doi:10.1371/journal. pone.0165499.
Huong Le, T.T.; Tran, N.T.; Lan Dao, T.M.; Nguyen, D.D.; Do, H.D.; Ha, T.L.; Kühn, R.; Nguyen, T.L.; Rajewsky, K.; Chu, V.T. Efficient and precise CRISPR/Cas9-mediated MECP2 modifications in human-induced pluripotent stem cells. Fron-tiers Genetics 2019, 10, 1–10, doi:10.3389/fgene.2019.00625.
Croci, S.; Carriero, M.L.; Capitani, K.; Daga, S.; Donati, F.; Frullanti, E.; Lamacchia, V.; Tita, R.; Giliberti, A.; Valentino, F.; et al. High rate of HDR in gene editing of p.(Thr158Met) MECP2 mutational hotspot. European Journal of Human Genetics 2020, 28, 1231–1242, doi:10.1038/s41431-020-0624-x
Zaslavsky, K.; Zhang, W.B.; McCready, F.P.;
Rodrigues, D.C.; Deneault, E.; Loo, C.; Zhao, M.; Ross, P.J.; El Hajjar, J.; Romm, A.; et al. SHANK2 mutations associated with Autism Spectrum Disorder cause hyperconnectivity of human neurons. Nature Neuroscience 2019, 22, 556–564, doi:doi:10.1038/s41593-019-0365-8
Zeliadt, N. CRISPR therapy may reverse autism mutation’s effects well past infancy. Spectrum News, October 20, 2019 Available online: https://www.spectrumnews.org/ news/crispr-therapy-may-reverse-autismmutations-effectswell-past-infancy/ (accessed September 2021)
Chen, X. Mangala, S.L. Rodriguez-Aguayo, C. Kong, X. Lopez-Berestein, G. Sood, A.K. RNA interference-based therapy and its delivery systems. Cancer and Metastasis Reviews 2018, 37, 107–124
Dong ZF, Tang LJ, Deng GF, Zeng T, Liu SJ, Wan RP, Liu T, Zhao QH, Yi YH, Liao WP, Long YS. Transcription of the human sodium channel SCN1A gene is repressed by a scaffolding protein RACK1. Molecular Neurobioly, 2014 50(2), 438-48. doi: 10.1007/s12035014-8633-9
Chen YH, Liu SJ, Gao MM, Zeng T, Lin GW, Tan NN, Tang HL, Lu P, Su T, Sun WW, Xie LC, Yi YH, Long YS. MDH2 is an RNA binding protein involved in downregulation of sodium channel Scn1a expression under seizure condition. Bio-chimica et Biophysica Acta - Molecular Basis of Disease. 2017, 1863(6), 1492-1499. doi: 10.1016/j.bbadis.2017.04.018
Kwok A, Raulf N, Habib N. Developing small activating RNA as a therapeutic: current challenges and promises. Thera-peutic Delivery. 2019, 10(3), 151-164. doi: 10.4155/tde-2018-0061
Holdt LM, Kohlmaier A, Teupser D. Circular RNAs as Therapeutic Agents and Targets. Frontiers in Physiology. 2018, 9 (1262) doi: 10.3389/fphys.2018.01262.
Toki N, Takahashi H, Zucchelli S, Gustincich S, Carninci P. Synthetic in vitro transcribed lncRNAs (SINEUPs) with chemical modifications enhance target mRNA translation. FEBS Letters. 2020, 24, 43574369. doi: 10.1002/1873-3468.13928
Markati, T., Duis, J., & Servais, L. (2021). Therapies in preclinical and clinical development for Angelman syn-drome. Expert opinion on investigational drugs, 30(7), 709– 720. https://doi.org/10.1080/1354 3784.2021.1939674
Liang XH, Shen W, Sun H, Migawa MT, Vickers TA, Crooke ST. Translation efficiency of mRNAs is increased by an-tisense oligonucleotides targeting upstream open reading frames. Nature Biotechnology. 2016, 34(8), 875-80. doi: 10.1038/nbt.3589
Stephen SL, Sivanandam VG, Kochanek S. Homologous and heterologous recombination between adenovirus vector DNA and chromosomal DNA. Journal of Gene Medicine. 2008, 10(11), 1176-89. doi: 10.1002/ jgm.1246.
Hampson, D. R., Hooper, A., & Niibori, Y. (2019). The Application of AdenoAssociated Viral Vector Gene Therapy to the Treatment of Fragile X Syndrome. Brain sciences, 9(2), 32. https://doi.org/10.3390/brainsci9020032
Merkle, T., Merz, S., Reautschnig, P., Blaha, A., Li, Q., Vogel, P., Wettengel, J., Li, J. B., & Stafforst, T. (2019). Precise RNA editing by recruiting endogenous ADARs with antisense oligonucleotides. Nature biotechnology, 37(2), 133–138. https://doi.org/10.1038/s41587-0190013-6
Kafri T, Blömer U, Peterson DA, Gage FH, Verma IM. Sustained expression of genes delivered directly into liver and muscle by lentiviral vectors. Nature Genetics. 1997, 17(3), 314-7. doi: 10.1038/ng1197-314.
Schenkwein D, Afzal S, Nousiainen A, Schmidt M, Ylä-Herttuala S. Efficient Nuclease-Directed Integration of Lentivirus Vectors into the Human Ribosomal DNA Locus. Mol Ther. 2020 Aug 5;28(8):1858-1875. doi: 10.1016/j.ymthe.2020.05.019.
Gaj, T.; Gersbach, C.A.; Barbas, C.F. ZFN, TALEN and CRISPR/Cas-based methods for genome engineering. Trends Bio-technol. 2013, 31, 397–405, doi:10.1016/j.tibtech.2013.04.004.
Kantor, A.; McClements, M.E.; MacLaren, R.E. CRISPR-Cas9 DNA Base-Editing and
Prime-Editing. Int. J. Mol. Sci. 2020, 21, 1–21, doi:doi:10.3390/ijms21176240.
Anzalone, A. V.; Koblan, L.W.; Liu, D.R. Genome editing with CRISPR–Cas nucleases, base editors, transposases and prime editors. Nat. Biotechnol. 2020, 38, 824–844, doi:10.1038/ s41587-020-0561-9.
Hirakawa, M.P.; Krishnakumar, R.; Timlin, J.A.; Carney, J.P.; Butler, K.S. Gene editing and CRISPR in the clinic: Current and future perspectives. Biosci. Rep. 2020, 40, doi:10.1042/BSR20200127.
Rees, H.A.; Liu, D.R. Base editing: precision chemistry on the genome and transcriptome of living cells. Nat. Rev. Genet. 2018, 19, 770–788, doi:10.1038/s41576-018-0059-1
Anzalone A V., Randolph PB, Davis JR, Sousa AA, Koblan LW, Levy JM, et al. Search andreplace genome editing with-out doublestrand breaks or donor DNA. Vol. 576, Nature. 2019. 149–157 p.
Scholefield, J.; Harrison, P.T. Prime editing –an update on the field. Gene Ther. 2021, 28, 396–401, doi:10.1038/s41434-021-00263-9.
Gao, P.; Lyu, Q.; Ghanam, A.R.; Lazzarotto, C.R.; Newby, G.A.; Zhang, W.; Choi, M.; Slivano, O.J.; Holden, K.; Walker, J.A.; et al. Prime editing in mice reveals the essentiality of a single base in driving tissue-specific gene expression. Ge-nome Biol. 2021, 22, 1–21, doi:10.1186/ s13059-021-02304-3.
Gupta, D.; Bhattacharjee, O.; Mandal, D.; Sen, M.K.; Dey, D.; Dasgupta, A.; Kazi, T.A.; Gupta, R.; Sinharoy, S.; Acharya, K.; et al. CRISPRCas9 system: A new-fangled dawn in gene editing. Life Sci. 2019, 232, doi: https://doi. org/10.1016/j.lfs.2019.116636
Frangoul, H.; Altshuler, D.; Cappellini, M.D.; Chen, Y.-S.; Domm, J.; Eustace, B.K.; Foell, J.; de la Fuente, J.; Grupp, S.; Handgretinger, R.; et al. CRISPR-Cas9 Gene Editing for Sickle Cell Disease and -Thalassemia. N. Engl. J. Med. 2021, 384, 252–260, doi:10.1056/ nejmoa2031054.
Ledford H. CRISPR treatment inserted directly into the body for first time. Nature. 2020;579(7798):185.
Gillmore, J. D., Gane, E., Taubel, J., Kao, J., Fontana, M., Maitland, M. L., Seitzer, J., O’Connell, D., Walsh, K. R., Wood, K., Phillips, J., Xu, Y., Amaral, A., Boyd, A. P., Cehelsky, J. E., McKee, M. D., Schiermeier, A., Harari, O., Murphy, A., Kyratsous, C. A., Lebwohl, D. (2021). CRISPR-Cas9 In Vivo Gene Editing for Transthyretin Amyloidosis. The New England journal of medicine, 385(6), 493–502. https://doi.org/10.1056/NEJMoa2107454
RNA-therapeutics based on short-interfering RNA (siRNA) and small activating RNA (saRNA) can offer an advantage over protein-based drugs as they act on the genome, or transcriptome level and are relatively easy to deliver due to their small size. In this chapter we set out to validate and improve previously published experiments that showed upregulation of SCN1A transcripts after genetic modifier genes RACK1 or MDH2 were downregulated via siRNA. In addition, we screened over thirty novel small activating RNAs (saRNA) for CHD2, SCN2A, SCN3A and KCNB1 to find out if this technology can be adopted in our lab and successful candidates can be identified for further development. Unlike the other chapters which contain published data, this chapter is set up as a pilot experiment, or “dsRNA screening” with a brief introduction and material & methods subchapter.
Conventional anti-seizure drugs rely on the ability to bind active sites of proteins to inhibit or alter their function. Since only 1.5% of the human genome encodes proteins, and only 1015% of these proteins have active binding sites that can be targeted, the current amount of ‘druggable’ targets in humans is rather limited [1]. In addition, for the production of recombinant proteins proper folding and post-translational modifications are required before they are functionally active, highlighting high resources and time demands. By contrast, nucleic-acid based treatment strategies such as RNA therapeutics avoid many of these limitations as they make use of the translational machinery of the mammalian cell.
The RNA interference (RNAi) pathway, also briefly explained in chapter 6 is a pathway that can be hijacked for therapeutic purposes. In a native state, RNAi is guided by a 20nt single stranded microRNA (miRNA) that can bind endogenous transcripts in order to regulate its expression, or viral RNA as part of the immune response. RNAi can also be activated by short interfering RNA (siRNA), which are 20nt double stranded RNAs that can be designed to virtually any human transcript resulting in targeted downregulation. Binding of siRNA to a target transcript is facilitated by the RNA-induced silencing complex (RISC), a multiprotein complex that consists of Dicer, Argonaute (Ago), TAR RNA-binding protein (TRBP), PACT and GW182 [2]. After
release of the passenger strand, RISC incorporates the single stranded ‘guide RNA’ aimed at complementary targets. After binding of the target transcript, RISC RNAse activity ultimately results in reduced protein expression. As of last year, three siRNA drugs are currently on the market, and seven more are in phase 3 clinical trial [3]
For diseases which are caused by loss of function mutations, applying siRNA would not be a useful technology if the transcript it directly targeted, but it could be applied when targeting modifier genes. Modifier genes act on the genomic, transcriptomic or protein-level and influence the function or expression of target genes. Also covered in previous chapters of this thesis, Dravet Syndrome (DEE#6) which is caused by loss-of-function mutations in SCN1A is known to have at least three of these genetic modifiers; in addition to SCN8A, which is well described in Chapter 2, modifier genes RACK1 [4] and MDH2 [5] have also been found to alter SCN1A expression (Figure 1A). RACK1 (Receptor for Activated C Kinase 1) is a scaffolding protein that shuttles activated protein kinase C to cellular membranes. RACK1 is highly expressed throughout the brain and is involved in various kinds of brain behaviors and neurological disorders. In contrast to its direct role as a scaffolding/ transporter protein, it can also interact with a silencer region in the SCN1A promoter. Cloning of the promoter region carrying the silencer element to control expression of a reporter gene showed that RACK1 can regulate SCN1A expression. Indeed, by knocking down RACK1 using siRNA, SCN1A mRNA expression was increased by 7-fold in differentiated NT2 cells [4] . MDH2 (Malate dehydrogenase 2) is a metabolic enzyme that participates in the citric acid cycle by catalyzing the interconversion of malate to oxaloacetate. MDH2 is also a known response protein during and after epileptic seizures, as MDH2 mRNA levels were found to be increased during and directly after a seizure occurs. MDH2 can bind to the 3’ UTR of SCN1A, and negatively regulate expression at the post-transcriptional level [5]. These findings highlight a possible link between the increased levels of MDH2 after a seizure, which in turn reduce SCN1A mRNA and thereby contribute to epileptogenesis. By knocking down MDH2 using siRNAs, a reporter gene under control of the 3’ UTR of SCN1A was found to be upregulated.
According to ATCC, NT2 cells are hypotriploid with a modal chromosome number of 63 [6], whereas normal human cells have 46, meaning that some chromosomes, possibly those that carry RACK1 and/or SCN1A or regulatory genes are over- or underrepresented. For this reason we were interested if RACK1 knock-down can also lead to upregulation of SCN1A in another neuronal cell line. From a karyotype perspective, differentiated SH-SY5Y cells better reflect a non-cancerous state, with a modal chromosome number of 47 (partial duplication of the q1 arm of chromosome 1) [7]. Like NT2 cells, SH-SY5Y cells can be differentiated to a neuron-like phenotype with cellular markers, neurite outgrowth and slow cell division rates (from here on named Diff.SH). For MDH2 we were interested if endogenous SCN1A mRNA could be increased, as an improvement to expression of a reporter gene in the original study. We design and test the exact same siRNAs from previous studies to target RACK1 and MDH2. Effect on SCN1A is established by qPCR targeting the endogenous transcript.
After 6, 24 and 72 hours post transfection (hpt), modifier gene mRNA levels were measured using qPCR. For both genes at least one siRNA was found to significantly downregulate its expression (Figure 1B & 1C), partly validating previous results [4,5] The other controls, siRNA#2 for RACK1 did not lead to downregulation, and siRNA#2 for MDH2 showed a borderline, but significant upregulation at 6hpt, but downregulation at 72hpt. When the effective siRNAs were transfected, the levels of SCN1A transcript were measured which did not show significant differences (Figure 1D)
Figure 1 Knock-down of SCN1A modifier genes A) Model of RACK1 and MDH2 interactions with the 5’ UTR and 3’ UTR of SCN1A, respectively. B), C) siRNA-medicated knock-down of MDH2 and RACK1. D) SCN1A levels after knock-down of modifier genes. Experiments were performed in Diff.SH cells, in biological duplicates and technical triplicates resulting in 6 datapoints per condition. The student T-test was used to test for significance (*<0.01, **<0.001, ****<0.0001) and a p-value >0.01 was considered not significant (n.s.).
A more recently discovered type of noncoding RNA with therapeutic potential are smallactivating RNA (saRNA). SaRNA are comparable to siRNA in molecular structure, but differ at the mechanism of action and target location although the exact pathways are still part of ongoing research. Where siRNA are targeted to gene transcripts, saRNA should be targeted to promoter or regulatory regions in the genomic DNA. Here, the endogenous transcription machinery is recruited and gene activation is triggered by altering the chromatin state. Interestingly, saRNAs require Ago proteins which are also part of the RISC involved in RNAi. More specifically, while siRNA can use Ago subtypes 1-4, saRNAs only require Ago2, as knocking down Ago1, 3 and 4 did not affect gene activation [8]. Ago2 facilitates separation of the passenger strand and the guide strand is transported to the nucleus. Here, the Ago2-saRNA complex binds the target promoter region in association with helicaseand polymerase-associated proteins RHA, CTR9 and PAF1. This facilitates histone remodeling and ultimately RNA polymerase II (RNAP2) activity leading to activation of transcription [9]. Paradoxally, siRNA and saRNA are exactly similar in design, and as both require Ago proteins it remains interesting to see what mechanism decides whether to form the RISC for RNAi, or transport the guide strand to the nucleus to initiate RNAa. Currently there are two saRNA drugs in phase II clinical trial including 2022’s STK-001 by Stoke therapeutics aimed at upregulation of SCN1A for Dravet Syndrome [10,11]
As saRNAs have not been tested in our lab, we start with validating control saRNAs from previous publications. These include p21-322 [12] targeting the CDKN1A (also called P21) tumor suppressor gene promoter and VEGF-706 [13] which targets the vascular endothelial growth factor gene VEGF promote. After validation, several sets of five saRNAs spanning the promoter region were designed for DEE genes SCN2A, SCN3A, KCNB1 and CHD2 based on previously published design rules [14]. Based on the complex 5’ UTR of these genes, both proximal and distal promoter regions were targeted, and experiments were performed in two cell lines. We used the HeLa cell line as it was used in the original publication to validate functionality of the control saRNAs. To find out what the effect would be in a neuron-like cell model, we used differentiated SH-SY5Y cells, also used for the siRNA transfections.
After 72 hours post transfection, p21-322 yielded a statistically significant upregulation of CDKN1A confirming its effect. VEGF-706 however, did not alter expression of VEGF mRNA (Figure 2A). Next, out of five saRNAs designed to the
distal promoter of CHD2 three showed a statistically significant upregulation of CHD2 transcript in HeLa cells (Figure 2B). Interestingly, for SCN3A four out of five saRNAs show a statistically significant downregulation (Figure 2C)
Figure 2 Screening of saRNA-candidates in HeLa cells A) Fold changes of two control saRNAs copied from previous publications B) saRNA design and fold changes targeting the proximal promoter region of SCN3A C) saRNA design and fold changes targeting the proximal promoter region of KCNB1 D) saRNA design and fold changes targeting the distal promoter region of CHD2. Experiments were performed using biological duplicates and technical triplicates resulting in 6 datapoints per condition. The student T-test was used to test for significance (*<0.01, **<0.001, ****<0.0001) and a p-value >0.01 was considered not significant (n.s.).
Transfection of the same five saRNAs for the CHD2 promoter to Diff.SH cells abolished the upregulation, and one candidate that was not effective in HeLa showed a significant downregulation (Figure 3A). This cell-type specific reversal of effect was also seen for saRNAs that target the SCN3A promoter; saRNA#2 and #3 that led to upregulation in HeLa, now show downregulation in Diff.SH cells. SaRNA#1 also triggered upregulation whereas it was not active in HeLa. SaRNA#4 and #5 which downregulated SCN3A in HeLa were not effective in Diff.SH (Figure 3B)
To find out what the effect of saRNAs on different promoter regions is, five additional saRNAs were designed to target the most proximal promoter region of CHD2. Four from these yielded a significant upregulation, in contrast to the observed effect on the distal promoter region (Figure 3A). In line with this effect, two sets of five saRNAs were designed for the KCNB1 gene, one targeting the proximal and one a distal promoter. Equal to CHD2, saRNAs targeted to the distal promoter-region led to downregulation but upregulation when the proximal promoter was targeted (Figure 3C). Of the five saRNAs that were designed, for the proximal promoter region of SCN2A one shows significant upregulation (Figure 3D).
Figure 3 Validation and Screening of saRNA Candidates in Diff.SH Cells A) saRNA design and fold changes targeting the proximal promoter region of SCN2A B) Fold changes of saRNA#1-5 targeting SCN3A C) Fold changes of saRNA #1-10 targeting KCNB1 D) Fold changes of saRNA#1-10 targeting CHD2. Experiments were performed using biological duplicates and technical triplicates resulting in 6 datapoints per condition. The student T-test was used to test for significance (*<0.01, **<0.001, ****<0.00001) and a p-value >0.01 was considered not significant (n.s.).
In this chapter, covering pilot experiments for RNA-based gene enhancement, we set out to alter the expression of DEE genes in-vitro using two different technologies. The aim of this study was to identify which technology could be used to increase expression and thereby contribute to both fundamental aspects of ncRNA functioning and future transient gene therapies for DS. Our first approach was based on previously discovered SCN1A-modifier genes RACK1 and MDH2. In previous reports, these modifier genes could effectively be knocked-down using siRNAs, which in turn led to SCN1A upregulation. For RACK1 siRNAs the upregulation of SCN1A was detected at the transcript level of the endogenous mRNA, whereas the MDH2 mediated siRNA effect was based on a reporter gene using only the 3’ UTR of SCN1A In Diff.SH cells, we successfully recapitulated the functionality of the siRNAs used in the aforementioned studies. Significant downregulation of modifier genes however, did not lead to upregulation of SCN1A.
One the challenges of this experiment is that the model system (cell type) could influence experimental outcome. Diff.SH (RA-differentiated SH-SY5Y neuroblastoma cells) are known to display only low endogenous levels of SCN1A, which could mean that they are a suitable model for reduced SCN1A expression and therefore a DEE disease state, but low levels of a transcript could also abolish a possible modifiergene pathway. If there are low levels of SCN1A transcript to start with, stabilization of these transcripts via MDH2 knock-down is perhaps not detectable. For RACK1 this argument can be ruled out, as its interaction partner is an SCN1A silencer element in the genome, and therefore does not require baseline expression. However, since there is low baseline expression of SCN1A, it could be that the genomic site is in a closed chromatin state, regulated by another silencer, or silencerindependent pathways. In turn, the silencer that RACK1 should act on, and which interaction could be disturbed by RACK1 knock-down, could also be in a closed chromatin state, further complicating these experiments. In previous studies, the baseline levels of SCN1A were not measured after MDH2 knock-down, but a reporter gene was transcribed under control of the 3’ UTR of SCN1A. This rather ‘synthetic’ system has the benefit that endogenous regulating elements including chromatin state are ignored and a measureable effect can be more easily detected. This, however, is also the downside of reporter gene systems as it could lead to false-positive outcomes, measured in a synthetic ‘non-realistic’ model system.
Knock-down of modifier genes should be carefully approached, and even if tampering with these genes successfully affects the target gene expression, there are likely side effects on different pathways. RACK1 for example does not only interact with the SCN1A silencer element, but its main function is shuttling activated C kinase
to the celmembrane. Reduced levels of RACK1 could range from minor alterations in protein activation to systemic alterations in the translation machinery even associated to tumor growth and metastasis [15] MDH2 is an interesting modifier gene as its levels are increased during and after seizure activity indicating a more direct role in epilepsy, with or without its interaction with SCN1A. Since MDH2 is both a metabolic enzyme and a ‘seizure response’ protein, there is likely an underlying mechanism – not yet discovered – that is responsible for the potential effect of the ketogenic diet on seizure frequency in Dravet Syndrome patients.
In contrast to siRNA, the saRNA screening showed more promising results, although they are complex to interpret. First, for every gene included in this screening at least one saRNA was found that could upregulate expression in Diff.SH cells. These can be interesting leads for further chemical optimization, delivery systems and other model organisms.
There appears to be a cell-type specific element that controls what the effect of the saRNA is, as several of our candidates showed upregulation in one cell line, but downregulation in another. Downregulation via saRNA has not been published before, and this could highlight a previously unknown pathway for saRNAs for which we have two possible explanations. First, since saRNAs are structurally equal to siRNA, they might just as easy act via the RNAi pathway if the binding site is included in the pre-mRNA, or mRNA transcript. While saRNAs are guided to the promoter region, and the promoter is not retained after transcription, this is not the case for genes with complex 5’ UTRs. Voltage-gated sodium channel (VGSC) genes, but also CHD2 typically show a multi-kb (up to 100kb) 5’ UTR with more than one active promoter and several untranslated exons and transcription start sites. In chapter 5 we briefly cover the regulation of SCN1A transcription and display the current layout of the 5’ UTR. SCN1A expression was shown to be regulated by different promoters per brain region, which could indicate that an extensive 5’ UTR is retained in the (pre-) mRNA transcript. If the saRNA is targeted to a downstream promoter that is retained after transcription, there is no reason to rule out that these saRNAs are siRNAs instead. During design we aimed at previously known transcription start sites (TSS), but since the active promoter already differs between brain regions in post-mortem brain tissue [16], it is possible that we did not target the active promoters in the model systems used.
Second, since saRNA are double stranded molecules the passenger strand is complementary to the antisense molecule and transcript. There appears to be no rule for guide and passenger strand selection by Ago2 and they could in theory be equally retained. If the passenger strand is retained and delivered to the nucleus, the natural antisense molecule or transcript (NAT) can be targeted instead. For some genes long non-coding RNA (lncRNA) is transcribed from the antisense strand of target genes which in turn can regulate transcription of the sense strand.
Since lncRNA is not transported to the cytosol, it can be targeted by the Ago2-saRNA instead.
Next, there appears to be a promoter-dependent effect for CHD2 and KCNB1, showing upregulation of gene expression with saRNAs designed to the proximal (ATG-flanking) promoter region, but no effect or even downregulation when a distal promoter region was targeted. In line with this, saRNAs designed for SCN2A and SCN3A also showed upregulation when the proximal promoter was targeted, highlighting that this could be a requirement for future saRNA design-rules.
As our data is limited to two cell lines, we believe improvements with regards to the model system and delivery should be priority before continuing. HeLa cells have a modal chromosome number of 65, which is roughly 30% more than healthy cells in the human genome. While Diff.SH are already much healthier from a karyotypeperspective, and they are a validated neuron-like model system, they are still tumor-derived in their undifferentiated state. Since dsRNA are small in size, they might be delivered with more ease to developed neuronal model systems, such as cortical organoids or MEA-grown co-or multi-cultures carrying both inhibitory and excitatory neurons in addition to astrocytes.
Transfection of dsRNA was performed using Lipofectamine RNAi-max according to manufacturers instructions. Cell line information is added in the ‘approach’ chapters. Sequences of siRNA and saRNA controls and design rules can be found in corresponding publications. Novel saRNA hits are currently tested as part of a separate publication by colleague PhD candidate J. van de Vondervoort and will not be enclosed to this chapter.
Hopkins, A. L., & Groom, C. R. (2002). The druggable genome. Nature reviews. Drug discovery, 1(9), 727–730. https://doi.org/10.1038/nrd892
Zhang, R., Jing, Y., Zhang, H., Niu, Y., Liu, C., Wang, J., Zen, K., Zhang, C. Y., & Li, D. (2018). Comprehensive Evolutionary Analysis of the Major RNA-Induced Silencing Complex Members. Scientific reports, 8(1), 14189. https://doi.org/10.1038/s41598-018-32635-4
Zhang, M. M., Bahal, R., Rasmussen, T. P., Manautou, J. E., & Zhong, X. B. (2021). The growth of siRNA-based therapeutics: Updated clinical studies. Biochemical pharmacology, 189, 114432. https://doi.org/10.1016/j.bcp.2021.114432
Dong, Z. F., Tang, L. J., Deng, G. F., Zeng, T., Liu, S. J., Wan, R. P., Liu, T., Zhao, Q. H., Yi, Y. H., Liao, W. P., & Long, Y. S. (2014). Transcription of the human sodium channel SCN1A gene is repressed by a scaffolding protein RACK1 Molecular neurobiology, 50(2), 438–448. https://doi.org/10.1007/s12035-014-8633-9
Chen, Y. H., Liu, S. J., Gao, M. M., Zeng, T., Lin, G. W., Tan, N. N., Tang, H. L., Lu, P., Su, T., Sun, W. W., Xie, L. C., Yi, Y. H., & Long, Y. S. (2017). MDH2 is an RNA binding protein involved in downregulation of sodium channel Scn1a expression under seizure condition. Biochimica et biophysica acta. Molecular basis of disease, 1863(6), 1492–1499. https://doi.org/10.1016/j.bbadis.2017.04.018
ATCC: CRL-1973 https://www.atcc.org/products/crl-1973
ATCC: CRL-2266 https://www.atcc.org/products/crl-2266
Li, L. C., Okino, S. T., Zhao, H., Pookot, D., Place, R. F., Urakami, S., Enokida, H., & Dahiya, R. (2006). Small dsRNAs induce transcriptional activation in human cells. Proceedings of the National Academy of Sciences of the United States of America, 103(46), 17337–17342. https://doi.org/10.1073/pnas.0607015103
Kwok, A., Raulf, N., & Habib, N. (2019).
Developing small activating RNA as a therapeutic: current challenges and promises. Therapeutic delivery, 10(3), 151–164. https://doi.org/10.4155/tde-2018-0061
Clinicaltrials.gov: NCT04710641
Clinicaltrials.gov: NCT04740476
Morris, K. V., Santoso, S., Turner, A. M., Pastori, C., & Hawkins, P. G. (2008). Bidirectional transcription directs both transcriptional gene activation and suppression in human cells. PLoS genetics, 4(11), e1000258. https://doi.org/10.1371/journal.pgen.1000258
Chen, R., Wang, T., Rao, K., Yang, J., Zhang, S., Wang, S., Liu, J., & Ye, Z. (2011). Upregulation of VEGF by small activator RNA in human corpus cavernosum smooth muscle cells. The journal of sexual medicine, 8(10), 2773–2780. https://doi.org/10.1111/j.17436109.2011.02412.x
Wang, J., Place, R. F., Portnoy, V., Huang, V., Kang, M. R., Kosaka, M., Ho, M., & Li, L. C. (2015). Inducing gene expression by targeting promoter sequences using small activating RNAs. Journal of biological methods, 2(1), e14. https://doi.org/10.14440/jbm.2015.39
Zhang, L., Lv, Y., Rong, Y., Chen, W., Fang, Y., Mao, W., Lou, W., Jin, D., & Xu, X. (2019). Downregulated expression of RACK1 results in pancreatic cancer growth and metastasis. OncoTargets and therapy, 12, 1007–1020. https://doi.org/10.2147/OTT.S176101
Nakayama, T., Ogiwara, I., Ito, K., Kaneda, M., Mazaki, E., Osaka, H., Ohtani, H., Inoue, Y., Fujiwara, T., Uematsu, M., Haginoya, K., Tsuchiya, S., & Yamakawa, K. (2010). Deletions of SCN1A 5’ genomic region with promoter activity in Dravet syndrome. Human mutation, 31(7), 820–829. https://doi.org/10.1002/humu.21275
Background: Prime editing (PE) is currently the most effective and versatile technology to make targeted alterations in the genome. Several improvements to the PE machinery have recently been made, and have been tested in a range of model systems, including immortalized cell lines, stem-cells and animal models. While nicking RNA (ncRNA)-dependent PE systems like PE3 and PE5 are currently considered to be the most effective, they come with undesired indels or SNVs at the edit locus.
Results: Here, we aimed to improve ncRNA-independent systems PE2 and PE4max by generating novel all-in-one (pAIO) plasmids, driven by a tissue-broad EF-1alpha promoter, that is especially suitable for human iPSC models, and linked to a GFP tag for fluorescent based sorting. These novel pAIO systems effectively corrected mutations observed in patients suffering from epileptic encephalopathy, including a truncating SCN1A R612* variant in HEK293T cells and a gain-of-function KCNQ2 R201C variant in patient-derived hiPSC, with edit efficiency up to 50%. We also show that introducing additional silent PAM-removing mutations can negatively influence edit efficiency. Finally, we observed an absence of genome-wide PE offtarget effects at pegRNA homology sites.
Conclusion: Our study shows an improved edit efficacy and accuracy of EF-1alpha driven ncRNA-independent pAIO PE plasmids in hiPSC. Consequently, this enables efficient generation of iPSC-derived disease model.
CRISPR/Cas9 was first used for genome editing in eukaryotic cells in 2013 [1], after which it rapidly evolved into a genome editing tool that has revolutionized the field of biology as a whole. Not only did it lead to an unprecedented increase in our capacity to study gene function and the effects of genetic variation on health and disease. It also brought us closer to the implementation of true precision medicine: the ability to apply treatment strategies specifically targeting the cause of disease; the underlying gene mutation. Prime editing (PE), the most recent gene editing tool in the field, uses a Cas9 nickase (Cas9n) protein fused to a reverse transcriptase (RT), and a PE guide RNA (pegRNA) consisting of a guide, a primer binding site, and RT template containing the desired edit [2]. PE is currently the most efficient and versatile gene edit system and can introduce all four possible base pair (bp) transversions, small insertions and deletions. It also has lower off-target effects
and a higher edit- to-indel ratio compared to previous Cas9n editors. The firstgeneration of PE technologies consist of PE2 (pegRNA + Cas9n-RT) and PE3 (pegRNA + Cas9n-RT + additional nicking RNA (ncRNA)). PE3 generates a second singlestranded break via ncRNA activity and enhances editing efficiencies by 1.5 to 4.2 fold compared to PE2 in immortalized cell lines, it however also increases the number of undesired indels and single nucleotide variants (SNV) [2]. The second generation PE systems have a human codon-optimized RT, a further optimized Cas9n and chemically modified pegRNAs that increase stability. PE2 and PE3 systems that have these enhancements can furthermore be co-expressed with MutL homolog 1 (MLH1dn), which is a DNA mismatch repair (MMR)-inhibiting protein, and are then named PE4max and PE5max, respectively [3]
When PE is applied to more advanced human in-vitro model systems such as human induced pluripotent stem cells (hiPSC) or liver organoid cells, edit efficiencies decrease significantly and are between 0 and 0.83% if no additional selection procedures are applied [4,5]. hiPSC-derived tissues are one of the most frequently used models to study disease mechanism or treatment strategies for genetic disorders nowadays. Where it was initially a common practice to use patient- and healthy control-derived iPSC lines as an experimental setup, it is nowadays preferred to either introduce disease relevant mutations in a control background or to correct mutations in patient-derived iPSCs to generate isogenic lines. This also allows the study of rare pathogenic variants or variants of unknown significance, when patient material is not available. However, introducing or correcting mutations is still highly laborious and costly, creating a bottleneck for more high-throughput projects. Recently, an all-in-one plasmid (pAIO) was published that contains the complete PE3 system (PEA1) thus facilitating transfection of stem cells with all the necessary constructs at once [6]. The pAIO-PE3 plasmid, combined with a puromycin selection showed a significant increase compared to the regular PE3 system in mouse stem embryonic stem cells, with edit efficiencies ranging between 1.7% and 85%. In line with other ncRNA-dependent PE systems, pAIO-PE3 activity however also resulted in a high rate of indel/SNVgeneration for most loci, occurring in up to 90% of the clones [6]. Since PE3 systems are accompanied by higher undesired indels/SNVs, improved versions of ncRNA-independent PE systems would be safer alternatives for gene-editing in iPSC-derived models. Furthermore, for optimal use in human iPSC disease models, elongation factor 1alpha (EF-1alpha) represents a more tissuebroad promoter that can drive stable expression during iPSC differentiation
For these reasons, we generated an pAIO-PE2-GFP and pAIO-PE4max plasmid, driven by the EF-1alpha promoter as an improvement to current cytomegalovirus (CMV)driven prime editors and improved edit efficiencies by adding a cell sorting step.
Pathogenic variants in SCN1A and KCNQ2 are both associated with Developmental Epileptic Encephalopathies (DEE), a group of severe neurological syndromes with an early age of onset and severe prognosis. KCNQ2 R201H is a Gain of Function variant that leads to a severe neonatal-onset encephalopathy without neonatal seizures but prominent startle-like myoclonus, and a burst-suppression EEG pattern [9]. The SCN1A R612* truncating variant was previously described in an individual with a DEE prototype Dravet Syndrome and gave rise to a severe seizure phenotype that resulted in acute encephalopathy leading to death [10]
As a proof of principle, we initially replicated experiments from the original PE publication in HEK293T cells using PE3 to introduce mutations in EMX1 (K263N and G265C)(2). We then showed we could reach comparable edit levels (~20%) when introducing a pathogenic variant in SCN1A (R612*) (Figure 1A). As expected for the ncRNA-depedent PE3 system, all conditions showed unintentend Indels/SNVs.
Figure 1 Introduction of DEE mutations using PE3, PE2max and PE4max. White bars represent editing of the target of interest, grey bars represent presence of undesired SNVs or indels at the edit locus, and black bars represent editing of the silent-PAM removing mutation. A) Validation of PE3 editing in EMX1 (K230N, G232C) and SCN1A (R612*). B) PE3 introduction of KCNQ2 R201H mutation with an addition silent-PAM removing mutation. C) Sanger and WES comparison of R201H double transfected PE3 sample. D) Comparison of ncRNA-dependent and independent editing systems using 3 different pegRNA. E) Efficiency of G to C conversion compared to G to T conversion in SCN1A at position c.1863-1865. The mean ± SD of all individual values of n = 3 to 6 independent replicates are shown.
Next, the KCNQ2 R201H mutation was introduced with an additional silent protospacer adjacent motif (PAM)-removing mutation. After the first round of transfection with PE3, both the edit and the silent PAM-removing mutation were detected. Interestingly, the edit levels of silent PAM-removing mutation exceeded that of R201H (Figure 1B). Another round of PE3 increased the edit levels for both R201H and silent PAM. We performed whole exome sequencing to validate edit efficiencies and to determine whether the exceeded rate of PAM removal was the result of a biased PCR amplification and Sanger sequencing. WES analysis confirmed that approximately 28% of the reads carry both R201H and PAM removal, but another 12% carried only the silent PAM mutation, yielding approximately 40% of the reads with a PAM mutation (Figure 1B & Additional File 1: Supplementary Figure 1 & Figure 2). These findings highlight that Sanger sequencing gives comparable results as NGS for PE3 experiments for R201H and PAM removal in HEK293T cells. Furthermore, introduction of an addition silent PAM-removing mutation can be favored over the intended edit. Cells with only the PAM-removal edit can not be edited anymore during next rounds of PE as Cas9 is unable to bind the DNA, therefore, reducing edit efficiencies.
Next, we compared the edit efficiency of the second generation, PE2max and PE4max multi-plasmid systems to PE3 for 3 pegRNAs in 2 different loci. All three editing systems are approximately equally effective, however, unintentend Indels/ SNVs were only detected for PE3, highlighting the challenge of ncRNA-dependent PE (Figure 1C). Due to the PE4max ability to inhibite MMR, it was previously shown to have higher edit efficiencies compared to PE2max. Interestingly, we did not observe this for our edited loci. Because C to G conversions can be more efficiently introduced as they evade MMR [3], we also validated if changing a NGG motif in SCN1A at position c.1863-1865 (NM_001165963.4) to NCG (p.L623V) would be more efficient compared to NTG (p.L623M). No increased efficiency was observed for L623V compared to L623M using PE2max. Both observations can be explained by the MMR deficiency of HEK293T cell, which limits the MMR-inhibition effect of PE4max and the advantage of specific base changes (Figure 1D)
As an improvement to current CMV-driven PE systems that require several plasmids (Figure 2A), we generated two novel all-in-one EF-1alpha-driven PE systems on a lentiviral backbone using molecular gene cloning. The tissue-broad EF-1alpha promoter is known for its high activity and stability in iPSCs over time, and is also described as one of the most stable promoters during iPSC differentiation [7,8]. Because ncRNA-based PE systems typically lead to additional unintended SNVs and indels, we used the PE2P2A-GFP editor [2] with a pegRNA to yield pAIO-PE2-GFP, and one of the most recent editors PEmax-MHL1dn [3] with a pegRNA to generate pAIO-PE4max (Figure 2B) Because the size of a plasmid influences transfection efficiency, we validated
Figure 2 Generation of all-in-one ncRNA-independent PE systems. WA) Overview of CMV-driven multiplasmid PE systems B) Overview of the two pAIO plasmids generated for this study. Plasmids are abbreviated to pAIO-PE2 and pAIO-PE4max. Grey arrow = CMV, Black arrow = U6, Brown arrow = hSyn1 Orange arrow = EF-1alpha. C) Comparison of multi-plasmid PE3 using two different promotors, versus pAIO-PE2-GFP delivery in HEK293T cells. The fraction of total mCherry, GFP and double positive (mCherry+GFP) cells are plotted. The black bar represents pAIO-PE2-GFP delivery. D) Delivery of PE3 and pAIO-PE2-GFP plasmids to iPSCs measured via flow cytometry and compared to unnucleofected iPSCs.
the delivery efficiency of our AIO plasmids which are 1.5 to 3 times larger than the independent PE plasmids (Figure 2B). We transfected HEK293T cells with our novel pAIO-PE2-GFP and compared it to multiplasmid PE3 transfections using the original pCMV:PE2-P2A-GFP and mCherry-tagged pegRNA and ncRNA plasmids. As expected for the PE3 system, a subset of cells underwent partial delivery shown by only expressing mCherry or GFP (Figure 2C). Delivery with pAIO-PE2-GFP showed similar expression to that of multi-plasmid PE3 in HEK293T, proving efficient delivery of our bigger plasmids (Figure 2C). Furthermore, use of a suboptimal promoter in the multi-plasmid system, shown here as the hSyn1 promoter in HEK293T cells,
significantly decreased the amount of double positive transfected cells compared to the CMV-driven multi-plasmid experiment (Figure 2C right)
Next, we validated the delivery of the pAIO-PE2-GFP to PE3 in iPSCs via flow cytometry. In contrast to our results in HEK293T cells, FACS analysis of the GFP positive population showed a 4- fold higher expression for the pAIO-PE2-GFP system compared to the CMV driven multi-plasmidPE3 system (66% vs 15%, respectively) (Figure 2D). Together, these data show the effective delivery of our AIO-PE system in HEK293T cells and iPSC, and highlight the importance of an optimal promoter and a single source of the PE machinery for transfection of iPSC.
Using PE3, we generated a homozygous R612* HEK293T cell line via single cell sorting and clonal expansion [data not shown]. To validate if pAIO plasmids for the correction of this mutation were functional, HEK293TR612*/R612* cells were transfected with pAIO-PE2-GFP and pAIO-PE4max and compared to PE3. After one transfection, pAIO-PE2-GFP, pAIO-PE4max and PE3 repaired the SCN1A R612* mutation on average for 31%, 33% and 29.5% of the cell population, respectively, validating that pAIO plasmids are functional. No undesired SNVs or indels were detected in pAIO-transfected cells, in contrast to those transfected with PE3 (Figure 3)
Left, Edit efficiencies for HEK293TR612*/R612* cells transfected with pAIO-PE2, pAIO-PE4max or PE3 plasmids. Right, Sanger traces showing a wildtype (WT), HEK293TR612*/R612* and pAIO-PE2 edited cells. Arrow indicates the C>T SCN1A R612 location.
Next, we generated wildtype and integrase-deficient LV particles (IDLV) that could deliver the complete PE machinery. In contrast to control LV and IDLV particles that
carry only the GFP gene, pAIO LV and IDLV show little GFP and marginal editing rates (1.5-1.7%) in HEK293T highlighting that further optimization for transient delivery of large LV cargo is necessary (Additional File 1: Supplementary Figure 3)
To validate the efficiency and accuracy of our pAIO-PE2-GFP and pAIO-PE4max in a more advanced model system, we tested if these systems could correct a pathogenic variant in a patient-derived hiPSC line, and compared them to the standard PE2 or PE3 systems. Nucleofected iPSCs were harvested at 72h and based on Sanger sequencing, we could not detect any edits nor indels for the CMV-PE2 and PE3 systems, which is in line with a previous study using PE3 in iPSC [4,5]. On the other hand, pAIO-PE2-GFP nucleofected cells that had clear GFP expression after 48h (Figure 4A), and pAIO-PE4max showed an average of 8% editing without additional cell sorting, and no undesired SNVs or indels (Figure 4B). Because the timepoint of harvest after transfection of iPSCs differs in the literature (ranging from 48h to 96h), we then performed an experiment using only pAIO-PE4max to validate edit efficiency at 48h vs 72h vs 96h. No significant differences in edit efficiency were observed between the different time points (Additional File 3). To find out if introducing an additional silent PAM-removing mutation could further increase editing, additional pegRNAs were designed. In contrast to our expectation, disturbing the PAM did not increase efficiency of PE at this locus (Figure 4B). Interestingly, while the silent PAM removing mutation affected edit efficiency, it was virtually absent on Sanger (Additional File 1: Supplementary Figure 4)
Figure 4 Figure 4 Patient-derived iPSC editing using the pAIO plasmids
A) Delivery and morphology of nucleofected iPSC. B) Correction of the heterozygous R201C mutation via pAIO plasmids compared to PE2 and PE3 with or without an additional silent PAM removing mutation. C,D) Cell sorting analysis and subsequent sequencing shows increased editing if the high-GFP portion is selected. E,F) Genotyping of single-cell derived clones after pAIO-PE2-GFP nucleofection of hiPSC cells without (E) and with additional silent PAM removing mutation (F).
We next aimed to improve edit efficiency through addition of a FACS sorting step. Since pAIO-PE2-GFP and pAIO-PE4max are both effective in hiPSC with minor differences in edit efficiency, pAIO-PE2-GFP was chosen for FACS sorting due to the benefit of a GFP tag and the absence of the MLH1dn MMR inhibitor which has a risk of introducing unwanted DNA mismatches. Furthermore, we sought to investigate if
the GFP signal intensity is a marker for edit efficiency. hiPSCs were sorted 48h after nucleofection. During sorting we gated the GFP population in two to separate the top half of the GFP signal (high GFP) from the bottom half of the GFP signal (low GFP signal) (Figure 4C). Edit levels showed a remarkably high pAIO-PE2-GFP efficiency in the high GFP population up to 41% and a smaller increase for the low GFP population up to 13% (Figure 4D). These increased efficiencies were replicated when using pAIO-PE2-GFP designed to introduce a silent PAM mutation, reaching 29% and 10% for the high and low GFP population respectively. These data show that FACS sorting on the highest GFP signal can significantly increase the edit efficiency by 5 fold, suggesting that more PE2 exposure increases editing (Figure 4D). Next, using the high GFP populations, we generated clonal lines to validate edit efficiencies. For the pAIO-PE2-GFP without PAM mutation, 16 out of 30 clones (51%) were corrected (Figure 4E), whereas for the pAIO-PE2-GFP with PAM mutation, 11 out of 36 clones (31%) were fully corrected (Figure 4F). Interestingly, although the PAM mutations were not visible on initial sanger traces before sorting, 8 out of 11 (73%) corrected clones carried the PAM mutation. Additionally, because of the lower edit efficiency when using an additional PAM mutation, we anticipated the presence of clones with a PAM mutation but no edit, as these cells cannot be edited anymore due to the disruption of the PAM. Against our expectations no clones were found that carried only the PAM mutation, indicating a Sanger bias, delayed MMR or another unknown reason are causing this observation. Taken together, we show here for the first time that a PE2 plasmid-based system can efficiently introduce mutations in hiPSC without any selection step. We also show that pAIO-PE4max is an equally effective system. Using GFP-based FACS sorting, we could further increased the edit percentage of the pAIO-PE2-GFP nucleofected population by 5-fold. Finally, the introduction of an additional silent-PAM removing mutation was not beneficial for this locus.
We validated 91 clones for one of the most frequently recurring CNV during iPSC culturing; the chr20q11.21 duplication [11,12]. This duplication decreases apoptosis which could be favourable during subcloning iPSC lines. Using Multiplex Amplicon Quantification (MAQ) we verified that none of our clones carried the duplication (Additional File 1: Supplementary Figure 5). Next, we validated in a subset of corrected clones the expression of pluripotency markers Oct4, Nanog and Sox2 (Additional File 1: Supplementary Figure 6). Two corrected clones of this subset were selected to perform WGS to verify the absence of PE mediated off-target effects. WGS analysis of the two corrected hiPSC clones and the parental iPSC line that did not undergo nucleofection shows that no alterations were made within 250 bp of all loci with homology to the pegRNA with a mismatch threshold of 4 bp. We did identify 146 and 139 novel variants respectively in the whole genome of the two corrected
hiPSC clones (data not shown), which is comparable to the number of novel SNVs in PE treated control and PE treated corrected organoid cells [13]. These variants followed an expected distribution of intergenic:intronic:exonic variants (47:51:2) [14] , were not located in a region homologue to the pegRNA, and the majority of variants found (58%) were C:G>A:T substitutions, indicating they are probably the result of accumulation of mutations during cell culture conditions through oxidative stress mechanisms [15] .
In this study, we developed and tested new all-in-one (AIO) plasmids containing the full PE2 and PE4max system and showed efficient introduction or correction of Developmental Epileptic Encephalopathies (DEE) causing variants in SCN1A and KCNQ2 in both HEK293T and hiPSC without off target edits.
Our experiments in HEK293T cells demonstrated efficient introduction of six different mutations in three different genes (SCN1A, KCNQ2 and EMX1) with similar edit efficiencies (~20%) using PE3. However, we observed a high yield of unintended alterations in the editing region for PE3 and other ncRNA-dependent PE systems in line with previous reports [2,3]. This downside was not observed using the secondgeneration ncRNA-independent PE systems, PE2max and PE4max, that showed equal editing efficiency compared to PE3 cells using three pegRNAs for two different loci. Due to the PE4max ability to inhibite MMR, it was previously shown to yield higher edit efficiencies compared to PE2max [3]. Interestingly, we did not observe this for our edited loci in HEK293T cells. Because C to G conversions may be more efficiently introduced as they tend to evade MMR, we also validated if changing a NGG motif in SCN1A at position c.1863-1865 (NM_001165963.4) to NCG (p.L623V) would be more efficient compared to NTG (p.L623M). No increased efficiency was observed for L623V compared to L623M using PE2max. Both observations can be explained by the MMR deficiency of HEK293T cell, which limits the MMR-inhibition effect of PE4max and the advantage of specific base changes.
The few studies that tested multi-plasmid PE3 for editing in hiPSC showed a nearly undetectable edit efficiency [4,5], making it not suitable for the generation of iPSCderived disease models. However, the recently published PEA1 (pAIO-PE3) all-inone plasmid was able to increase the edit efficiency significantly in immortalized cell lines as well as mouse embryonic stem cells (mESC) when combined with a puromycin selection step [6]. It did however induce similarly high unintended alterations as seen in the multi-plasmid approach. We show here the efficiency and accuracy of ncRNA-independent AIO PE plasmids, pAIO-PE2-GFP and pAIO-PE4max. To increase the functional diversity of the plasmid, we changed the CMV promotor
to the elongation factor 1 alfa (Ef-1a) promoter. First, the EF-1a promoter was shown to be a much stronger promotor compared to the CMV promoter in stem cells [7,8] and neurons [16]. Secondly, it is one of the most stable promoters in iPSC and during in vitro iPSC differentiation [17]. We show that our novel AIO PE plasmids, which are larger than those required for multi-plasmid PE systems, show effective delivery in both HEK293T and hiPSC cells as shown by GFP expression in 70% and 65% of cells, respectively. As expected, our Ef-1α driven AIO plasmid showed a 4-fold higher GFP expression compared to the CMV-PE-GFP in iPSC. This highlights the importance of choosing the correct promoter when using plasmid-based systems and may have been a contributing factor to the low success rate of previous studies using CMVPE3 in iPSCs [4,5] .
pAIO-PE2-GFP and pAIO-PE4max are suitable for lentivirus production, given that they are cloned on a pLV transfer plasmid. Together with integrase-deficient packaging plasmids this would yield a transient, single virus gene-edit platform that could be used to edit neurons in-vitro and in-vivo. We showed that while smallcargo lentiviruses and integrase-deficient lentiviruses can be generated, production of the much larger pAIO-PE2-GFP ID-LV is challenging. Curiously, a previous report shows that it is possible to package a large cargo in a single lentivirus, as the full-length dystrophin gene, which is well over 15 kb, was effectively delivered. Since the production titer of pAIO-PE2-GFP viruses was comparable to small cargo lentiviruses, but the GFP expression after transduction hardly visible, we believe that the majority of viruses did not carry the complete cargo. Improved packaging plasmids, upscaled production and alternative tittering methods could perhaps further improve the generation of a single, transient gene edit virus.
We show that our pAIO-PE plasmids are effective for correction of the KCNQ2 R201C mutation in a patient-derived iPSC line. Remarkably, both pAIO-PE2-GFP and pAIOPE4max showed an average correction of 8%. Although, it was expected to see an increased edit percentage in pAIO-PE4max as hiPSC have robust MMR activity [18], we saw an equal edit efficiency, which contrasts with the original report that showed a 2.5-fold increase [3]. Interestingly, we observed an increased amount of cell death in the AIO-PE4max nucleofected cells, which could indicate activation of apoptotic pathways due to increased DNA damage. Furthermore, it has been shown that reduction of the MMR system in ESC due to inhibition of SIRT1 resulted in increased cell death [19]. Prolonged MMR inhibition due to our plasmid approach might activate these apoptotic pathways, which might not happen when using MLH1dn mRNA as described in the original paper. Further research should be performed to clarify these discrepancies.
To further increase edit efficiency, we sorted the pAIO-PE2-GFP nucleofected iPSC population using FACS and showed that selection of the highest GFP expressing cell population has higher edit efficiency as compared to the lower GFP expressing cell
population. This shows that more copies of PE plasmid per cell, and/or a more active cell specific promoter, improves edit efficiency, probably due to the increased rate of proper transcription of the PE machinery. We found that the high GFP population gave rise to a 5-fold increase in edit efficiency (up to 41%), compared to the unsorted cells and subcloning of the iPSC line showed correction of the R201C mutation in 53% of the isolated clones. This efficient generation of subcloned iPSC lines consequently decreased the workload for colony picking and validation significantly.
Next, we tested whether disruption of the protospacer adjacent motif (PAM) could alter edit efficiency, as this could interfere with next rounds of prime editing. Interestingly, by introducing a silent PAM-removing mutation together with the R201C correction, we observed a reduction in hiPSC editing both before and after cell sorting. While the majority of clones carried both PAM removal and the R201C correction, a small subset was PAM-removal absent. This observation was exactly opposite to PAM removal in HEK293T which showed the majority of alleles to carry only the PAM mutation but a small subset to carry the edit as well, which was verified by whole exome sequencing. The apparent preference for either the silent PAM-removing mutation or the desired edit could be cell-type specific, given that HEK293T is MMR-deficient, but this should be further investigated.
Finally, using WGS on the parential iPSC line and two pAIO-PE2-GFP corrected clones we could not find any genome-wide off-target effects at loci with homology to the pegRNA with a threshold of four basepair mismatches, confirming prior evidence of the safety of the PE2 technology. In contrast to a previous study using PE treated cells that did not carry the correction as a negative control [13], we used the parental, unnucleofected line. Using PE treated cells as a negative control could miss potential off-target outcomes as the PE machinery is introduced in both conditions. Comparing the raw number of novel events in control versus edited cells does not exclude unique events potentially caused by the combination of editor and pegRNA, or editor alone. We detected a number of novel SNVs in the two corrected clones compared to the parental line that, while not associated with PE activity, could be the result of subculturing and oxidative stress mechanisms leading to accumulation of random mutations [15]. We therefore highlight the importance of using the correct control condition for WGS experiments but also confirm safety and accuracy of ncRNA-independent prime edit technologies.
We expanded prime editing technologies to central nervous system genes SCN1A and KCNQ2 which are responsible for developmental epileptic encephalopathies and discovered that introduction of a silent PAM-removing mutation is not necessarily
beneficial to prime edit efficacy. The pAIO-PE2-GFP and pAIO-PE4max plasmids are the first to produce detectable editing in iPSCs without a selection procedure facilitating prime editing in patient derived model systems. After sorting GFP positive cells, more than 50% of the clones carried the R201C correction. As an outline for the future, we believe that single PE plasmids can be further optimized with smaller Cas variants and cloning on a smaller plasmid backbone. The single source, Ef-1a driven PE plasmids are efficient tools for the introduction and correction of mutations in immortalized cells lines and patient-derived stem cell models. Finally, improved lentiviral production for larger cargos, ideally combined with integrase-deficient packaging plasmids to yield transient expression would enable an efficient delivery and advance translation of efficient prime editing in vivo.
PegRNA Cloning for PE3, PE2max and PE4max in HEK293T pegRNAs and nicking RNAs were cloned into AAV-U6-sgRNA-hSyn-mCherry (Addgene #87916). The pegRNAs and nicking RNAs were designed using PegFinder [20]. 100µM single-stranded DNA oligos were ordered via IDT in MilliQ. Oligos were annealed by mixing 10 µL of each oligo in 20 µL of 2X annealing buffer (20 mM Tris pH 7.5, 100 mM NaCl and 20 mM EDTA) and ran in the following PCR program; 5 minutes on 95ºC, start on 95ºC and -2ºC/second for 4 seconds, start on 85ºC and -0.1ºC/second for 599 seconds and incubation on 4ºC. The AAV-U6-mCherry plasmid was linearized by SapI following manufacturer’s instructions. Ligation of the oligos was performed by T4 DNA Ligase (NEB M0202L) following manufacturer’s protocol and transformed using TOP10 competent cells. Plasmids were purified using Qiagen mini or midiprep kits and verified by Sanger Sequencing. For oligo sequences see Additional File 2: Supplementary Table.
pLV-EF1a-PE2-P2A-GFP was generated by cloning the original pCMV-PE2-P2A-GFP insert (Addgene #132776) into an EF-1alpha driven lentiviral backbone by Signagen Inc (from here on called pLV-PE2). DNA oligos were designed for introduction of new restriction sites in pLV-PE2. Single-stranded DNA oligos were annealed following previous described protocol. Digestion of the backbone was performed by ClaI (NEB #R0197L) according to manufacturer’s instructions. Ligation of the annealed oligos in the linear backbone was performed by T4 DNA Ligase. pLV-EF1a-PEmax-P2AhMLH1-dn was generated from the pLV-PE backbone. The pCMV-PE4max backbone was linearized by AgeI (NEB #R3552L) and MluI restriction sites were introduced via molecular gene cloning according to above methods with the exception that Stbl3 and not TOP10 competent cells were used. The pCMV-PE4max with MluI restriction site and the pLV-PE plasmid were both digested by MluI (NEB #R0198L) and NotI (NEB
#R0189L). PEmax-P2A-hMLH1-dn insert was ligated in the pLV-EF1alpha backbone and transformed in Stbl3 competent cells. pLV-EF1a-PE2-P2A-GFP (#184445) and pLV-EF1a-PEmax-P2A-hMLH1-dn (#184444) are available on Addgene after publication. Plasmids were verified using Sanger Sequencing. For oligo sequences
see Additional File 2: Supplementary Table
pegRNA Cloning and Generation of pAIO-PE2-GFP and pAIO-PE4max PegRNAs were ordered as 500ng gBlock (double stranded DNA fragments) via IDT and resuspended in 10µL MilliQ prior to cloning in the dual PaqCI (NEB #R0745L) site of pLV-EF1a-PE2-P2A-GFP (from here on called pAIO-PE2-GFP) or pLV-EF1a-PEmaxP2A-hMLH1-dn (from here on called pAIO-PE4max). Digested plasmids and gBlock DNA fragments were purified by Wizard SV Gel and PCR clean-up system (Promega) and transformed in Stbl3 competent cells. pAIO-PE2-GFP:KCNQ2-C201R (#185060) and pAIO-PE2-GFP:KCNQ2-C201R-P (#185061) are available on Addgene after publication. Plasmids were verified using Sanger Sequencing. For oligo sequences see Additional File 2: Supplementary Table
24 hours prior to transfection, HEK293T cells were seeded to yield approximately 50% confluency at day 1 in 6 wells plates. For PE3, three different plasmids were transfected simultaneously in HEK293T cells; 1 µg of pCMV-PE2-P2A-GFP, 0.5 µg of pegRNA plasmid and 0.5 µg of nicking RNA were combined with 50 uL OptiMEM and 4 uL Lipofectamine2000 and added to the cells. For PE2max (Addgene #174820) and PE4max (Addgene #174828), 1.4 µg editor and 0.6 µg of pegRNA was used. Genomic DNA extraction was performed 72 hours after transfection using DNeasy Blood & Tissue Kit from Qiagen. In the case of a second transfection experiment, approximately 20% of the cells remained in culture and transfected when confluency reached 50% again. The remaining cells were used for gDNA extraction. The edit locus was PCR amplified using TaqGold Polymerase and the PCR product was purified by Wizard SV Gel and PCR clean-up kit followed by Sanger Sequencing.
Whole exome sequencing was performed on DNA from HEK293T. Methods and the script used are listed here: https://github.com/UMCUGenetics/DxNextflowWES and the IGV alignment is added as Additional File 1: Supplementary Figure 2
Lentivirus (LV) was produced by transfection of transfer and packaging plasmids in HEK293T cells. The following plasmids were used in a 4:2:1:1 (ug) ratio; transfer plasmid (pAIO): pMDLg/pRRe (or psPAX2-D64 for integrase deficient lentivirus); pRSV-rev; pMD2.g. pDNA was mixed in 200 uL OptiMEM and combined with 24 uL PEI (1 mg/mL). The DNA:PEI complexes were added to the cells after 20 minutes incubation at room temperature and medium was replaced at approximately 18
hours. LV or integrase-deficient LV (IDLV) particles were harvested using the Lenti-Xconcentrator (comp/code) 72 hours after transfection according to manufacturer’s protocol. The titer of harvested lentivirus was established using the Lenti-X RT-qPCR Titration Kit (Takara Bio, Cat. no. 631235). A representative calibration plot and LV tittering of pAIO is added as Additional File 1: Supplementary Figure 3
The hiPSC line harboring the R201C KCNQ2 variant was obtained by reprogramming patient PBMCs. hiPSCs were maintained in StemFlex medium (Thermo Fisher Scientific, A3349401) on Geltrex (Thermo fisher Scientific, A1413302) coated plates at 37°C and 5% CO2. Cells were passaged with ReleSR (Stemcell Technologies, 05872) when they reached 70-80% confluency. The hiPSC line was tested for pluripotency markers (Nanog, Sox2 and Oct4) using qPCR, and showed no abnormalities on CNV analysis.
hiPSC were dissociated using Accutase (Sigma, A6964). Cell pellets of 1E6 cells were resuspended with 20ul supplemented nucleofector solution of the P3 Primary Cell 4D-nucleofector® X Kit S (Lonza, V4XP-3032) and 2ug plasmid (1ug/ul), and nucleofected with the 4D-Nucleofector Core and X Unit (Lonza), with program CB150. 10 min after nucleofection, cells were plated in StemFlex media supplemented with 10uM Y-27632 rho kinase inhibitor (Tocris, 1254). Genomic DNA extraction was performed 72 hours after transfection, unless stated otherwise, using Macherey-Nagel NucleoSpin DNA mini kit (Macherey-Nagel, 740952) according to manufacturer’s protocol. The edit locus was PCR amplified using Titanium Taq DNA Polymerase (Takara, 639208) followed by Sanger Sequencing.
Flow Cytometry Analysis and Fluorescence-Activated Cell Sorting (FACS) of iPSC Nucleofected With pAIO-PE2-GFP 48 hours after nucleofection, hiPSCs were dissociated using Accutase. The pellet was resuspended in 1ml FACS buffer (PBS with 2% Fetal bovin serum), with 1000X Viability Dye eFluor® 780 (Thermo Fisher Sci., 65-0865), and incubated for 20 min on ice. After incubation, 10ml FACS buffer was added, and cells were centrifuged for 3min at 300g to wash the cells. The cell pellet was resuspended in 2 ml FACS buffer and ran through a 30um filter. GFP+ signal was measured using the MACSQuant Analyzer. Cells for sorting were added to the MACSQuant® sorting cartridge (Miltenyi Biotec). Gating hierarchies were constructed using MACSQuant Tyto software before sorting. Cell debris, doublets, and dead cells were gated out. GFP+ signal was divided in two (high and low GFP signal). After sorting cells of the lower GFP signal, the positively sorted cells were collected from the cartridge and seeded in StemFlex media supplemented with 10uM Y-27632 rho kinase inhibitor, the negatively sorted cells were reloaded, and the sorting gate was changed to a higher GFP+ signal.
Subcloning of the edited iPSC lines to generate isogenic clones, was performed by seeding 500 single iPSCs in one well of a 6 well plate coated with Geltrex, in StemFlex media supplemented with CloneR™ (stem cell technologies, 05888). Three hours after seeding, cells were placed in the Incuctye® Live Cell Analysis System and imaged every 3 hours for 7 to 10 days to track if colonies originated from 1 single cell. Cells were kept in CloneR supplement media for 4 days, whereafter the CloneR supplement was removed. Between day 7 and day 10, colonies originating from one single cell were picked and placed in an individual well of a 24 well plated coated with Geltrex and StemFlex media. Clonal lines were split into two 12 wells; one for expansion and one for DNA isolation to check the absence or presence of the R201C mutation.
Sanger files (.ab1) were uploaded in EditR for determination of edit efficiency and unintended SNVs or indels. Sanger files for Figure 1D are added as Additional File 1: Supplementary Figure 1.
DNA was extracted from two sorted clones and the naïve parental hiPSC line. Samples were submitted to BGI Denmark for WGS with standard sequencing coverage of 30X/90Gb data. Reads were aligned to human genome build hg38 using BWA. Single-nucleotide variants and small insertion-deletions were called using both GATK and Strelka variant callers. Novel variants in the predicted offtarget regions of the corrected hiPSC clones were identified as any variant within 250 bp of a predicted off-target site with a mismatch threshold of 4 that was called as reference in the parent clone by both GATK and strelka and as a variant in one of the two corrected clones by both callers (with coverage > 8 and genoqual > 25). Novel variants in the whole genome were further filtered on having no supporting reads in the parent clone, a GATK mapping quality > 50, mapping quality rank score > -2.5 and genotype quality > 70 in both the parent and corrected clone. Variants were annotated using GenomeComb.
Total RNA of five corrected hiPSC clones and the naïve parental iPSC line was isolated using the Macherey-Nagel NucleoSpin RNA mini kit (Macherey-Nagel, 740955) according to manufacturer’s protocol. cDNA was reverse-transcribed from total RNA using the iScript cDNA Synthesis Kit (Bio-Rad Laboratories, 1708890). qPCR reactions were done in triplicate with SYBR Green Real-Time PCR master mixes (Applied Biosystems, 4309155). Expression levels were normalized to GAPDH and the ΔΔCt method was used to determine the relative levels of mRNA expression (Additional File 1: Supplementary Figure 6)
In order to determine whether the recurrent chr20q11.21 duplication(11, 12) appeared in the corrected clonal lines, we screened our corrected clones with the in-house developed Multiplex Amplicon Quantification (MAQ) technique (Agilent) consisting of a multiplex PCR amplification of fluorescently labeled target and control amplicons, followed by fragment analysis. The assay contains six target amplicons located in and around the chr20q11.21 region, and five control amplicons located at randomly selected genomic positions outside the chr20q11.21 region and other known CNVs. These 11 amplicons were PCR-amplified in a single reaction containing 20 ng of genomic DNA. Peak areas of the target amplicons were normalized to those of the control amplicons. Comparison of normalized peak areas between clonal lines and references resulted in a dosage quotient (DQ) for each target amplicon, calculated by the MAQ software (MAQ-S) package (Agilent). DQ values above 1,25 were considered indicative for a duplication (Additional File 1: Supplementary Figure 5).
PegRNAs and ncRNA Oligos for U6-mCherry (5’-3’)
PegRNAs and ncRNA oligos for U6-mCherry (5’-3’)
Design template
ACC-sgRNA-scaffold-3’extension-TTTTTT- (edit in red) -NNNNNNNNNNNNNNNNNNNNNNNNNNNNNNNNN-CAA KCNQ2 pegRNA R201H-P fw
ACCGCAGAATCTGCAGGAAGCGCGTTTTAGAGCTAGAAATAGCAAGTTAAAATAAGGCTAGTCCGTTATCAAC TTGAAAAAGTGGCACCGAGTCGGTGCTCCGGAGTCTGCACTTCCTGCAGATTTTTTT
rv AACAAAAAAATCTGCAGGAAGTGCAGGCTCCGGAGCACCGACTCGGTGCCACTTTTTCAAGTTGATAACGGAC TAGCCTTATTTTAACTTGCTATTTCTAGCTCTAAAACGCGCTTCCTGCAGATTCTGC
ncRNA R201H fw
ACCTCCATTGCGGTGCTGGCCGC rv AACGCGGCCAGCACCGCAATGGA
pegRNA R560W-P fw
ACCCCATCACGTCGTAGGGCCGCGTTTTAGAGCTAGAAATAGCAAGTTAAAATAAGGCTAGTCCGTTATCAAC TTGAAAAAGTGGCACCGAGTCGGTGCAGGAGAGTCTGTGGCCCTACGACTTTTTT
rv ACCAAAAAAGTCGTAGGGCCACAGACTCTCCTGCACCGACTCGGTGCCACTTTTTCAAGTTGATAACGGACTA GCCTTATTTTAACTTGCTATTTCTAGCTCTAAAACGCGGCCCTACGACGTGATGG
ncRNA R560W fw
SCN1A pegRNA R612*
ACCCACCCCCCTGCAGTGTCATG
rv AACCATGACACTGCAGGGGGGTG
fw
ACCCGTCTCTCTCCGTGTCGTCGGTTTTAGAGCTAGAAATAGCAAGTTAAAATAAGGCTAGTCCGTTATCAAC TTGAAAAAGTGGCACCGAGTCGGTGCGTTTGTGCCCTGACGACACGGAGATTTTTT
rv AACAAAAAATCTCCGTGTCGTCAGGGCACAAACGCACCGACTCGGTGCCACTTTTTCAAGTTGATAACGGACTA GCCTTATTTTAACTTGCTATTTCTAGCTCTAAAACCGACGACACGGAGAGAGACG
pegRNA *612R fw ACCGCGTCTCTCTCCGTGTCGTCGTTTTAGAGCTAGAAATAGCAAGTTAAAATAAGGCTAGTCCGTTATCAACT TGAAAAAGTGGCACCGAGTCGGTGCTTTGTGCCCCGACGACACGGAGAGTTTTTT
rv AACAAAAAACTCTCCGTGTCGTCGGGGCACAAAGCACCGACTCGGTGCCACTTTTTCAAGTTGATAACGGACTA GCCTTATTTTAACTTGCTATTTCTAGCTCTAAAACGACGACACGGAGAGAGACGC
fw
pegRNA L623V
ACCATGACCTACTGGTCTGACTCGTTTTAGAGCTAGAAATAGCAAGTTAAAATAAGGCTAGTCCGTTATCAAC TTGAAAAAGTGGCACCGAGTCGGTGCACAGCAACGTGAGTCAGACCAGTAGGTTTTTTT
rv AACAAAAAAACCTACTGGTCTGACTCACGTTGCTGTGCACCGACTCGGTGCCACTTTTTCAAGTTGATAACGGA CTAGCCTTATTTTAACTTGCTATTTCTAGCTCTAAAACGAGTCAGACCAGTAGGTCAT
pegRNA L623M fw ACCATGACCTACTGGTCTGACTCGTTTTAGAGCTAGAAATAGCAAGTTAAAATAAGGCTAGTCCGTTATCAACT TGAAAAAGTGGCACCGAGTCGGTGCACAGCAACATGAGTCAGACCAGTAGGTTTTTTT
rv
AACAAAAAAACCTACTGGTCTGACTCATGTTGCTGTGCACCGACTCGGTGCCACTTTTTCAAGTTGATAACGGA CTAGCCTTATTTTAACTTGCTATTTCTAGCTCTAAAACGAGTCAGACCAGTAGGTCAT ncRNA 612-623 fw
ACCTGATGAGCACAGCACCTTTG
rv AACCAAAGGTGCTGTGCTCATCA
EMX1 pegRNA K230N fw
pegPAM G232C
ACCGAGTCCGAGCAGAAGAAGAAGTTTTAGAGCTAGAAATAGCAAGTTAAAATAAGGCTAGTCCGTTATCAACT TGAAAAAGTGGCACCGAGTCGGTGCGTGATGGGAGCCCTTGTTCTTCTGCTCGGTTTTTT
rv AACAAAAAACCGAGCAGAAGAACAAGGGCTCCCATCACGCACCGACTCGGTGCCACTTTTTCAAGTTGATAACG GACTAGCCTTATTTTAACTTGCTATTTCTAGCTCTAAAACTTCTTCTTCTGCTCGGACTC
fw
ACCGAGTCCGAGCAGAAGAAGAAGTTTTAGAGCTAGAAATAGCAAGTTAAAATAAGGCTAGTCCGTTATCAACT TGAAAAAGTGGCACCGAGTCGGTGCATGGGAGCACTTCTTCTTCTGCTCGGTTTTTTT
rv AACAAAAAAACCGAGCAGAAGAAGAAGTGCTCCCATGCACCGACTCGGTGCCACTTTTTCA AGTTGATAACGGACTAGCCTTATTTTAACTTGCTATTTCTAGCTCTAAAACTTCTTCTTCTGCTCGGACTCGGT
Oligos for pAIO cloning (5’-3’)
Supplementary Figure 1
Supplementary Figure 1
Sanger files for R201H editing with PE2max, PE4max and PE3
Sanger Files for R201H Editing with PE2max, PE4max and PE3
PE2max
PE2max
PE4max
PE4max
Sanger trace of R201H PE2max, PE4max and PE3 editing. Line indicates editing locus, asterix highlights undesired SNVs.Sanger Trace of R201H PE2max, PE4max and PE3 Editing. Line Indicates Editing Locus, Asterix Highlights Undesired SNVs.
respectively. D) Editing rates of pAIO-PE2-GFP LV and IDLV using 250 and 500 MOI in HEK293T cells.
A) GFP lentivirus produced with psPAX and psPAX-D64V packaging plasmids show stable and transient GFP expression respectively, over time with various MOI. Circles: psPAX/LV, squares: psPAX=D64V/IDLV. At D21 GFP expression is absent at 10-100 MOI of IDLV indicating transient expression in contrast to stable expression of LV over time. B) Titering of pAIO-PE2-GFP LV and IDLV plotted on the control LV RNA calibration curve (Lenti-X-titering, Takara). C) Imaging of pAIO-PE2-GFP LV and IDLV at MOI 250 and 500, respectively. D) Editing rates of pAIO-PE2-GFP LV and IDLV using 250 and 500 MOI in HEK293T cells.
Dosage plot of the MAQ analysis. Top: Example of control sample with normal copy number variation at chr20q11.21. Bottom: Example of sample carrying the chr20q11.21 duplication. Dots in the grey region (dosage quotient between 0.8 and 1.2) correspond to two copy numbers. The five dots between dosage quotient 1.2 to 1.5 correspond to three copy numbers. MAQ = multiplex amplicon quantification.
RT-qPCR expression analysis of pluripotency markers Oct4, Nanog and Sox2 in for five R201C corrected clonal lines (C25-C30) and the naïve parental line (R201C-N). Values were calculated using the ΔΔCt method and are normalized to GAPDH and fibroblasts expression data
Cong L, Ran FA, Cox D, et al. Multiplex genome engineering using CRISPR/Cas systems. Science. 2013; doi:10.1126/science.1231143
Anzalone AV, Randolph PB, Davis JR, et al. Search-and-replace genome editing without double-strand breaks or donor DNA. Nature. 2019; doi:10.1038/s41586-019-1711-4
Chen PJ, Hussmann JA, Yan J, et al. Enhanced prime editing systems by manipulating cellular determinants of editing outcomes. Cell. 2021; doi:10.1016/j.cell.2021.09.018
Habib O, Habib G, Hwang GH, Bae S. Comprehensive analysis of prime editing outcomes in human embryonic stem cells. Nucleic Acids Res. 2022; doi:10.1093/nar/gkab1295
Schene IF, Joore IP, Oka R, et al. Prime editing for functional repair in patientderived disease models. Nat Commun. 2020; doi:10.1038/s41467-020-19136-7
Adikusuma F, Lushington C, Arudkumar J, et al. Optimized nickase- and nuclease-based prime editing in human and mouse cells. Nucleic Acids Res. 2021; doi:10.1093/nar/gkab792
Wang R, Liang J, Jiang H, Qin LJ, Yang HT. Promoter-dependent EGFP expression during embryonic stem cell propagation and differentiation. Stem Cells Dev. 2008; doi:10.1089/scd.2007.0084
Kim S, Kim GJ, Miyoshi H, et al. Efficiency of the elongation factor-1alpha promoter in mammalian embryonic stem cells using lentiviral gene delivery systems. Stem Cells Dev. 2007; doi:10.1089/scd.2006.0088
Mulkey SB, Ben-Zeev B, Nicolai J, et al. Neonatal nonepileptic myoclonus is a prominent clinical feature of KCNQ2 gainof-function variants R201C and R201H. Epilepsia. 2017; doi:10.1111/epi.13676
Tian X, Ye J, Zeng Q, et al. The clinical outcome and neuroimaging of acute encephalopathy after status epilepticus in Dravet syndrome.
Dev Med Child Neurol. 2018; doi:10.1111/dmcn.13727
Martins-Taylor K, Nisler BS, Taapken SM, et al. Recurrent copy number variations in human induced pluripotent stem cells. Nat Biotechnol. 2011; doi:10.1038/nbt.1890
Laurent LC, Ulitsky I, Slavin I, et al. Dynamic changes in the copy number of pluripotency and cell proliferation genes in human ESCs and iPSCs during reprogramming and time in culture. Cell Stem Cell. 2011; doi:10.1016/j.stem.2010.12.003
Geurts MH, de Poel E, Pleguezuelos-Manzano C, et al. Evaluating CRISPR-based prime editing for cancer modeling and CFTR repair in organoids. Life Sci Alliance. 2021; doi:10.26508/lsa.202000940
Francis WR, Wörheide G. Similar Ratios of Introns to Intergenic Sequence across Animal Genomes. Genome Biol Evol. 2017; doi:10.1093/gbe/evx103
Kuijk E, Jager M, van der Roest B, et al. The mutational impact of culturing human pluripotent and adult stem cells [published correction appears in Nat Commun. 2020 Aug 4;11(1):3932]. Nat Commun. 2020; doi:10.1038/s41467-020-16323-4
Lee SY, George JH, Nagel DA, Ye H, Kueberuwa G, Seymour LW. Optogenetic control of iPS cell-derived neurons in 2D and 3D culture systems using channelrhodopsin-2 expression driven by the synapsin-1 and calcium-calmodulin kinase II promoters. J Tissue Eng Regen Med. 2019; doi:10.1002/term.2786
Hong S, Hwang DY, Yoon S, et al. Functional analysis of various promoters in lentiviral vectors at different stages of in vitro differentiation of mouse embryonic stem cells. Mol Ther. 2007; doi:10.1038/sj.mt.6300251
Lin B, Gupta D, Heinen CD. Human pluripotent stem cells have a novel mismatch repairdependent damage response. J Biol Chem.
2014; doi:10.1074/jbc.M114.570937
Jang J, Huh YJ, Cho HJ, et al. SIRT1 Enhances the Survival of Human Embryonic Stem Cells by Promoting DNA Repair. Stem Cell Reports. 2017; doi:10.1016/j.stemcr.2017.06.001
Chow RD, Chen JS, Shen J, Chen S. A web tool for the design of prime-editing guide RNAs. Nat Biomed Eng. 2021; doi:10.1038/s41551-020-00622-8
Counsell JR, Asgarian Z, Meng J, et al. Erratum: Lentiviral vectors can be used for full-length dystrophin gene therapy. Sci Rep. 2017; doi:10.1038/srep46880
The aim of this thesis was to investigate novel treatment strategies for mutations that cause developmental epileptic encephalopathies. I tried to couple fundamental research in disease modelling and gene function to applied pre-clinical research covering protein, mRNA, and DNA-based therapies. We generated two novel animal models for disease, designed a custom electrophysiology recording chamber and applied RNA therapeutics, CRISPRa, and Prime editing. The following chapter discusses our data in the context of each of the three levels that therapy for human genetic diseases can act on: protein, mRNA and DNA. Each subchapter ends with a rationale and future perspectives. Finally, the methodologies are critically evaluated including the benefits and drawbacks of zebrafish models systems, cell lines and the delivery of RNA and DNA-based drugs.
Medication for epilepsy patients today consists of ion channel blockers and drugs that act on neurotransmitter levels or receptors, targeting the protein level of disease. Ion channel blockers are drugs that bind the pore region of voltage-gated sodium channels, limiting transport of sodium, resulting in decreased action potential generation. Using VGSC blockers with more affinity for CNS channels, or even within CNS channel subtypes such as those expressed mainly on excitatory neurons, may improve disease outcome.
We set out with exactly this aim in mind: to test novel VGSC blockers with increased selectivity for Nav1.6, which is expressed predominantly on pyramidal excitatory neurons over Nav1.1 expressed on GABAergic interneurons. In collaboration with Dr. Rivara (Parma University, Italy), two compounds, named MV1312 and MV1369, were designed with selectivity of Nav1.6 over other VGSC subtypes. We tested MV1312 and MV1369 in a zebrafish model for Dravet Syndrome (DS) that we generated in our lab. Both compounds effectively decreased the seizure phenotype, highlighting that selective Nav1.6 blocking can be beneficial in DS.
Next, we were interested in the possibility of subtype-selective VGSC activation, since most mutations that cause DS lead to LOF of Nav1.1. Increasing the activity of the remaining Nav1.1 channels could be a more direct approach in comparison to inhibition of Nav1.6. During this project, several Nav1.1 activators were discovered by other groups, including spider toxin Hm1a which proved effective in a DS mouse
model [1]. We collaborated with Dr. Benned-Jensen (Lundbeck Pharmaceuticals, Denmark), who published AA43279, a small molecule Nav1.1 activator [2]. In our study using the DS zebrafish model AA43279 showed similar results to Nav1.6-selective blocker MV1312 summarizing that both activation of Nav1.1 and inhibition of Nav1.6 could be useful for treatment of DS [3]
We generated the first CRISPR/Cas9-based zebrafish model for Dravet Syndrome by specifically knocking out the scn1Lab gene. There are currently ten different zebrafish models for DS, each generated with different technologies, dating back to 2015 [4]. Compared to other DS models, the general pharmacological profile is equal showing inefficacy of non-selective VGSC blockers and effective seizure reduction with valproate, stiripentol and fenfluramine, reflecting the general drug response in DS patients. This surprisingly strong predictive validity of DS zebrafish is sufficient for drug screenings, but face validity and contruct validity are less prominent and further covered in subchapter 9.5. For the first time we show that both selective inhibition of Nav1.6 and selective activation of Nav1.1 can be effective in DS.
In the weeks before we published our manuscript, another selective Nav1.6 blocker called XEN-901 was in development by Xenon Pharmaceuticals who are as of 2022 recruiting patients with a GOF mutation in SCN8A [5]. Given our data, it would be interesting to see how well this drug works in DS patients. As XEN-901 is in further development, MV1312 will not be further tested in rodent models for DS by our group, although it could be a promising alternative. For fundamental research, MV1312 was recently already picked up by another research group due to its selectivity for Nav1.6 channels and used to study visual input in the optic tectum of xenopus [6] .
In theory, compounds that specifically block excitatory channels would be beneficial even for patients with common epilepsy, and could replace conventional, non-selective VGSC blockers. However, Nav1.6 channels are not solely responsible for neuronal excitation and in the case of a GOF Nav1.2 (SCN2A) mutation, a drug like XEN-901 likely needs an equal or higher therapeutic dose than non-selective blockers, highlighting the need for a patient specific approach via genetic diagnosis. Previously, VGSC activators were considered unlikely candidates for clinical purpose. Off-target binding can always occur at prolonged exposure and at a high dose this can cause severe side effects. Still, one of the more recent Nav1.1 activators, Hm1a, was reproduced just two years ago via recombinant protein technology to yield rHm1b. rHm1b was found to be a safe and promising candidate in mice models of DS highlighting that this isn’t the end for Nav1.1 activators in drug discovery [7]
Even with VGSC subtype selective drugs, side effects will remain as drugs have to
be redosed just like current ASDs. As long as the DNA mutation remains and faulty mRNA continues to be produced, there will be incomplete rescue of the phenotype. By testing these compounds in zebrafish we opened the avenue of both Nav1.6 inhibition and Nav1.1 activation, but this model lacks the capacity of testing for side effects. Also, since the nucleotide conservation of some VGSC genes in zebrafish is low, and duplicate genes exist, there can be doubts about the construct validity. We believe that the zebrafish can be used to test promising compounds in a highthroughput fashion, but validation should always be done in mammalian models.
Based on the genetic background of DS, which is caused by heterozygous loss-offunction mutations in SCN1A, the remaining gene copies can be increased at the transcription level. In the case of the zebrafish, which has duplicated genes for SCN1A: scn1Laa and scn1Lab, a homozygous truncating mutation in scn1Lab leaves the two scn1Laa alleles intact for targeted upregulation, which we covered in Chapter 3. After the discovery of CRISPR/Cas9, a catalytically inactive Cas9 was developed named ‘dead Cas9’ (dCas9) which binds, but does not cut the DNA. By fusing transcriptional activators or inhibitors to dCas9, genes can be down or upregulated by guiding the machinery to promoter regions of target genes.
First of all, we found that zebrafish injected with CRISPRa showed severe toxicity with or without a sgRNA in more than 50% of the animals including developmental arrest and malformations. Toxicity was not present in embryos injected with wildtype Cas9, indicating that the transcriptional activator combined with dCas9 was responsible. The remaining animals continued to develop normally until 5 days post fertilization and were selected for further analysis.
As the promoter regions of scn1Laa were not functionally tested yet, we targeted both a proximal and distal promoter region, lying adjacent and 10kb upstream of the translation start site, respectively. Both promoter regions could be targeted by CRISPRa, showing upregulation of scn1Laa and by targeting both promoters simultaneously, an increased fold change was detected highlighting a dosing effect also found in other CRISPRa studies. Interestingly, when comparing the mild transcription activator dCas9-VP64 to dCas9-VPR, which consists of Rta and P65 proteins in addition to VP64, transcription was reduced rather than upregulated, indicating that VP64 alone is less effective in zebrafish.
In scn1Lab knockouts the locomotor profile and burst movements were further increased after scn1Laa upregulation, contrasting our expectation that increased scn1Laa could compensate for loss of scn1Lab. After scn1Laa upregulation using CRISPRa in wildtype zebrafish, a seizure-phenotype was developed showing epileptiform activity, increased locomotor activity and burst movements
implicating that scn1Laa GOF is just as impactful as scn1Lab LOF. The fact that the phenotype of scn1Lab knockouts was worsened and toxicity was present in more than half of the embryos led us to conclude that CRISPRa is not effective in treating DS in zebrafish at an early developmental stage. The development of a seizure phenotype in wildtype zebrafish with increased scn1Laa highlights that the scn1Laa protein is likely involved in excitatory signaling, which raises the question how much duplicated genes share in function.
We implemented the CRISPRa technology for zebrafish embryos in our lab and successfully enhanced the expression of a VGSC gene. In wildtype embryos this led to the development of a seizure phenotype, and in DS zebrafish the phenotype worsened, which was unexpected. We targeted two different promoter regions and used different dCas9 fusions that show different outcomes. Two interesting and somewhat contradictionary findings are the dCas9-fusion associated toxicity and the downregulation of gene expression with dCas9-VP64.
At the time of this study, CRISPRa was a relatively new technology, that had only been applied in zebrafish embryos once before [8]. We first tested if we could replicate an experiment from that study by enhancing the expression of a control gene. The observed fold changes were comparable, meaning we successfully implemented CRISPRa. In contrast to the other study, we observed toxicity at early developmental stages, which was not reported, although a time-window difference of the phenotype can in part explain this.
During this project and slightly after publication of our manuscript, two other in vivo CRISPRa studies were published, using mouse models of DS [9,10]. Injections were done at later developmental stages than in our study and were well tolerated. Unfortunately, the phenotypic rescue was mild, showing a reduction of temperatureinduced seizures in some animals whereas spontaneous seizures and SUDEP, the most severe characteristics of DS mice, were not reduced by CRISPRa. The absence of a full rescue of the phenotype was attributed to low delivery to target cells, confirming the main challenge of current CRISPR studies, even with recombinant adeno-associated viral vectors which are currently the number one delivery vehicle for genetic drugs. Even if delivery can be pushed to reach more neurons in the brain, CRISPRa requires repeated dosing as the effect is transient.
In addition to differences in gene layout, such as duplicated gene sets in zebrafish, the need for allele-specific upregulation in case of a heterozygous SCN1A mutation, the size of dCas9 fusion proteins but also the fact that re-dosing should happen once expression levels return to baseline, makes us critical for therapeutic interventions based on CRISPRa.
An alternative to CRISPRa to enhance or decrease gene expression is the use of small RNAs that can be guided to promoter regions to activate expression (saRNA), or targeted to mRNA to decrease translation (siRNA). Both saRNA and siRNA-based drugs are in development for use in patients with genetic diseases due to their efficacy and small size which facilitates delivery. We set out to alter the expression of DEE genes in vitro using both technologies. Our first approach was based on previously discovered SCN1A-modifier genes RACK1 and MDH2. In previous reports, these modifier genes could effectively be knocked-down using siRNAs, which in turn led to SCN1A upregulation. For RACK1 siRNAs, the upregulation of SCN1A was detected at the transcript level of the endogenous mRNA [11], whereas the MDH2 mediated siRNA effect was based on a reporter gene using only the 3’ UTR of SCN1A [12]. To validate and improve these experiments, we replicated them in neuronal cells and measured the endogenous transcript levels. While we successfully recapitulated the functionality of the siRNAs, we did not observe the modifier effect on SCN1A. SaRNAs would have a more direct effect on gene expression, bypassing modifier genes. To increase the number of potential saRNA hits, we tested thirty novel saRNAs for four different DEE genes. For each gene saRNAs were found that increase gene expression, but the effect was determined by cell-type and promoter region. Interestingly, some saRNAs were found to decrease gene expression, opening another avenue of the saRNA landscape and expanding its application toolbox.
Downregulation of modifier genes MDH2 and RACK1 was successfully replicated by us in improved in vitro models systems, but no upregulation of SCN1A was observed. It is likely that cell-type specific effects were detected in previous studies, highlighting the use of a sensible model system to test a hypothesis. Thirty novel saRNAs were tested for four DEE genes, and for each gene a hit was identified that enhanced gene expression at the transcription level. There was a cell-type, and promoter-type specific effect that even displayed gene downregulation for some saRNAs which is a unique finding for these type of small RNAs.
Genetic modifiers date back to the late 90s and highlight the complexity of human genetics. While it is interesting to label them as therapeutic targets, it should be clear that these are usually genes that are unaffected by the disease-causing mutation, which is present on genes that the modifiers act on. By altering the expression of modifier genes, native pathways of these genes are altered as well. For MDH2 this could mean changes in the Krebs cycle and, after prolonged exposure, the development of cancer [13] as also shown by LOF mutations in this gene. What complicates this route and further abolishes potential modifier-gene-based
treatments using MDH2 is that LOF mutations can even lead to the development of DEE, DEE51 to be precise [14]. LOF MDH2 mutations can be passed down through generations and lead to DEE via autosomal recessive transfer. At least three DEE51 patients are described with compound heterozygous mutations in MDH2. This is in line with RACK1, which expression levels are associated with the development and metastasis of cancer [15,16] highlighting that altering its function with the aim of changing SCN1A is an inadvisable avenue to follow. As siRNAs were, and still are, strong and versatile tools for the downregulation of genes, they might be better used to compensate for GOF mutations in DEE genes as a more direct approach.
For LOF mutations, which make up the majority of DEE cases and many other genetic diseases in mankind, small activating RNA (saRNA) can be a more promising tool. It is very interesting to see that gene expression can be altered just by guiding a 20 nucleotide RNA to the regulatory region of a gene, without coupling it to transcription activators like with CRISPRa. However, since the discovery of saRNA dating back to 2008 [17], only two candidates have made it to clinical trials in 2022, perhaps marking difficulties confirming pre-clinical in vitro findings or little interest in the technology. In addition to potential clinical candidates, saRNA can also help identify transcriptionally active regions also shown by our screening for DEE genes.
One of our key findings is that targeting the most proximal promoter yielded upregulation of all four genes. This could become a design rule for saRNA in general. The reason why targeting distal promoter units generally yielded no effect or even caused downregulation is interesting, such a clear difference has not been shown before. In summary, our saRNA findings mark a potential undiscovered mechanism of saRNAs and our novel hits can contribute to potential novel treatment options for DEE. Further improvements can include chemical modification of saRNAs to increase stability, labeling of passenger and guide strands to find out which is used and the use of patientderived model systems, such as hiPSC differentiated neurons that better mimic the genetic and transcriptomic environment.
All of the above described drugs and potential future genetic therapies have in common that re-dosing is still required, which is the starting point of why we want to improve current epilepsy drugs. While altered mRNA or protein levels can remain stable over several weeks or months, the DNA mutation remains, meaning incorrect protein is continuously being produced. 2021 marked the first inhuman gene editing clinical trials using CRISPR/Cas9 [18,19]. Given that these still use the original CRISPR/ Cas9 system from 2013, which creates a double-stranded break in the genome, improved versions such as Base editing and Prime editing (PE) can follow shortly. We introduced several mutations in HEK293T cells as proof of principle, including
the clinically relevant truncating R612* mutation in SCN1A. After this, we removed the mutations as well, showing the pegRNA functionality. We made improvements to the PE system by cloning all the components on a single plasmid instead of multi-plasmid systems, improving from partial to full delivery from a single source. Our all-in-one PE, although larger in size, showed high delivery rates to HEK293T, and efficient removal of SCN1A R612*. From a patient with KCNQ2-encaphalopathy (DEE7), we generated a stem cell culture (hiPSC) which carried the heterozygous R201C mutation. With our all-in-one PE, this mutation was also removed, confirming functionaility of PE both in immortalized cell lines and patient-derived stem cells. We applied NGS to screen for off-target effects and detected no off-target editing at sites with homology to the pegRNA with a threshold of four nucleotide mismatches confirming the safety of PE.
We applied PE for DEE mutations and improved the current PE system by enabling expression from an eukaryotic (Ef-1a) promoter in contrast to the cytomegalovirus (CMV) promoter used before. We edited both HEK293T cells and hiPSC and show relatively high edit efficiencies with our all-in-one PE2 system. In contrast to PE3 and even PE5max, which are currently the most efficient PE systems [20], PE2 does not require an additional nickingRNA. For us, this trade-off between efficiency and safety meant that a less efficient but safer gene-editing system is beneficial, as redosing can be applied without concerns for off-target effects.
The field of gene editing is moving rapidly, with new editing enzymes, smaller Cas9 variants and improved delivery systems every other year since 2017, highlighting that current clinical trials are just the start of gene editing drugs. Efforts in RNA therapeutics and gene therapy that are already ongoing will likely encounter more delivery, safety and administration challenges that will help pave the road for future gene editing drugs. Since CRISPR/Cas9 is relatively cheap to incorporate in research (plasmid DNA for PE can be ordered from Addgene for as little as $65) [21] and results of editing can be observed within 3 days after transfection, the technology is likely to be adopted all over the world in the coming years.
Given that gene-editing permanently removes the disease-causing mutation in the target tissue, there would be no need for re-dosing. A common error against geneediting would be that all the cells that carry the mutation should be repaired. In the case of SCN1A mutations that is not necessarily vital as changing a severe DS phenotype to a milder form such as generalized epilepsy (GE) or GE with febrile seizures (GEFS+) is already a life-changing solution for many patients. It is therefore an interesting idea to consider re-dosing gene edit drugs when the proportion of targeted cells edited is limited. After a single dose 5% of the target tissue may be edited, but this level can increase after a second, third or fourth dose. Edited cells
are no longer recognized by the gene editing machinery due to a mismatch between the pegRNA and the genomic DNA that carries the corrected basepair, meaning that unedited cells are preferred when dosing is repeated. In Chapter 8 we briefly touch upon this topic, repeated transfections (doses) of PE can increase the edit efficiency in a stepwise manner, without the need for additional cell sorting.
By showing that we can introduce and remove DEE mutations, we increased the number of functional pegRNAs. The 20 nucleotide sgRNA region that binds the mutation site will likely still be required for future versions based on CRISPR/Cas9. Therefore our work could contribute to future gene edit drugs when a patient is identified with a SCN1A R612* or KCNQ2 R201C mutation. In 2022, PE is still far from perfect and while the number of offtarget mutations and undesired indels is nearly absent, delivery is still the main challenge, especially to the brain. It made sense that one of the earliest genetic drugs for nervous system diseases was aimed at delivery to motor neurons in the PNS [22], as these neurons are relatively easy to reach with rAAV. In contrast to the PNS which can be targeted via spinal cord injections, the CNS is much more complex to deliver drug to and even if a local rAAV injection can be performed, for example during epilepsy surgery, current technology is still lacking in efficient spread further from the injection site. Promising avenues for in vivo gene editing would be the generation of smaller PE or Cas9 variants, preferably under 3kb, which allows packaging with the sgRNA/pegRNA in a single recombinant adeno-associated viral vector.
The zebrafish, used since the 1980s to model human diseases, is a versatile model organism useful for rapid drug screenings in vivo, and to study regenerating characteristics of cardiac, brain and skin tissue. The true power of zebrafish is its small size and rapid development, which allows a high number of animals to be tested in a relatively short time. This was shown by the repurposing of Fenfluramine, a drug previously prescribed for patients with obesity, as it lowers their serotonin levels resulting in reduced food-intake [23]. Within three years from testing Fenfluramine in a DS zebrafish, it was prescribed to DS patients, which displays an unnaturally fast pace of ‘tank-to-bedside’ as a parallel of the commonly used term ‘bench-to-bedside’ [24]
Our work using the zebrafish as a model system yielded a novel CRISPR/Cas9 generated strain for DS, one of the earliest in vivo CRISPRa studies, and the discovery of subtypeselective VGSC compounds. These findings, while requiring laborious
experiments, would not have been possible with mammalian model systems using the same amount of time and cost investment. There is a surprisingly wellconserved predictive validity for DS zebrafish models, which is captured in ASD response. DS zebrafish treated with CBZ and PHT, which are not effective in DS patients, also show no changes in the seizure phenotype. Vice versa, Valproate, Stiripentol, and Fenfluramine, which can reduce seizures in DS patients, were also effective in DS zebrafish.
Face validity for zebrafish models for epilepsy is shown by locomotor hyperactivity, burst movements, photosensitivity and epileptiform activity detected from the brain. While the complexity of the zebrafish brain is a separate topic, its largest limitation for epilepsy research is that there is no clearly defined prefrontal cortex [25], which is the region from which seizures are recorded in mammalian model systems and human patients. We therefore used the term ‘epileptiform activity’ which can be recorded using a single needle electrode in a glass capillary placed on top of the forebrain. Given that the optic tectum (midbrain) and cerebellum (hindbrain) in zebrafish larvae are relatively large structures that lie adjacent to the forebrain, and the whole brain is just several millimeters in size, epileptiform activity could be originating from these regions just as well. It is tempting to claim that what we detected in zebrafish embryos reflects generalized or, hindbraininitiated, focal seizures but there is little anatomical overlap with the mammalian, let alone the human brain. Interestingly, in adult zebrafish focal and generalized events can be detected using a multi-channel EEG [26], highlighting that more developped zebrafish might display improved face validity.
Construct validity, especially for DS zebrafish models, is much lower than for mammalian model systems due to the low conservation score and gene duplication event in zebrafish, described in more detail in Chapter 4. In addition to low construct validity, we discovered concerns for predictive validity as well, such as the inefficacy of Clemizole (CLM) in scn1Lab knockouts versus their seizure-reducing effect in another scn1Lab model published by another group [27]. CLM, which belongs to the anti-histamine class of drugs is not related to CNS diseases, but was discovered using zebrafish as a repurposed drug for DS in another study as it can act on the Serotonin pathway as well, like Fenfluramine [23]. The reason why Fenfluramine was, but CLM was not effective in our strain is a topic of debate that was not picked up by other research groups. In Chapter 2 and 4 we briefly touch upon technologies used to generate animal models, and highlight the differences between random mutagenesis with ENU and CRISPR/Cas9, which could explain differences in drug response. Interestingly, DS patients are currently recruited to enroll in a clinical trial for EPX-100 (Clemizole Hydrochloride) [28], as an add-on therapy with the first results expected by the end of September 2022. Based on our results alone, Clemizole would not have been selected as a candidate for use in DS patients.
In general, we learned that the zebrafish model system has benefits but also important limitations and can only be used to some extent, based on a specific research question. For model translation to human patients, zebrafish genes should be selected with high conservation scores and ideally not be a part of a duplicated gene set. Given that future medication for genetic diseases will be based on RNA or DNA, zebrafish is no longer fit as a model organism for testing these therapies. The low conservation scores of zebrafish genes, promoters, transcription regulators, Argonaute protein pathways that are essential for RNA therapeutics and the presence of duplicated gene sets make mammalian model systems much more suitable for this purpose. However, when solely aiming to test conventional drugs for repurposing purposes, using zebrafish can significantly speed up data acquisition via high-throughput drug screenings before a smaller sub set of positive hits can be further validated in mammalian model systems.
The most widely used model system in molecular biology consists of cell lines, of which some have been in use since the 60s, such as HeLa, derived from Henrietta Lacks [29]. Cell lines have the advantage that they continue to grow steadily, often rapidly allowing a fast experimental trajectory. However, the user friendliness of these cell lines is derived from their cancerous origin, which also means that they are not healthy cells. For example the karyotype of frequently used HeLa or HEK293T cell lines show a modal chromosome number of 82 and 64 respectively, which is 36 and 18 more than normal cells, respectively [30,31]. It is not just an addition of chromosomes; these cell lines carry massive chromosomal rearrangements leading to genes that are over or underrepresented, translocated to other chromosomes or even inverted. This unequivocally results in alterations in expression levels, changes in regulatory regions, but also epigenetic regulation resulting in changes in gDNA accessibility. In this thesis we tried to find a middle way between using a sensible model system and being able to perform the experiments with the time and resources that were available. In the experiments that involved the use of HeLa cells in Chapter 5, we replicated the experiments in Diff.SH-SY5Y cells, which are from a karyotype perspective nearly equal to healthy cells showing a modal chromosome number of 47 [32]. Interestingly and validating the variance between cancerous cell lines, we indeed found differences when the same saRNA was transfected. Packaging of Lentiviral particles in chapter 8 involves the use of HEK293T cells, but these cells are actually generated for exactly this purpose: being a viral particle bioreactor due to the SV40 large T-antigen that enhances replication of plasmid DNA [33] .
In Chapter 8 we introduced a novel gene editing technology and the initial aim was to test this technique rather than use a model system that represents the human brain. HEK293T cells have been used in the original Prime Edit (PE) publications of 2019 and 2021, and since they are easy to transfect, they were used for an initial
proof-of-principle experiment. PE using our novel all-in-one delivery systems were later tested in patient-derived induced pluripotent stem cells (hiPSC). HiPSC are reprogrammed cells, usually originating from fibroblast or bone-marrow tissue and have become one of the most popular disease modeling tools for drug screens and to study disease mechanisms. The reason for this success is the iPSC’s capability to differentiate into theoretically any cell type [34], and the genetic architecture resembles that of a patient, rather than that of a tumor from decades ago, as is the case for immortalized cell lines.
A downside of hiPSC is that they are driven to the stem cell state with transcription factors (TF). To maintain this state, TF have to be supplied continuously, which is usually done by transducing the patient fibroblasts with lentiviral (LV) vectors [35]. LV vectors which belong to the class of retroviruses, also covered in Chapter 6 integrate their cargo more or less randomly in the genome, and as the number of LV vectors that reach a single cell is currently impossible to establish, there could be thousands of LV genomes in a typical hiPSC clone, ultimately leading to both genetic and transcriptomic differences. These are however, in no way comparable to those in immortalized cell lines, but should be given thought during experiment design. After establishing the hiPSC, they require a second round of differentiation [36] to the tissue type of interest. This requires the addition or removal of certain growth factors and TF (again via stable, random integration of LV vectors), and equal to organoid technology can result in small but significant deviations from ‘true’ patient cells and tissue that made up the original biopsy material. Culturing of stem cells will inevidently introduce additional mutations as well, which is briefly covered in Chapter 8 showing an abundancy of novel mutations that arise during culturing of an isogenic control line. This highlights that a "true" isogenic control cell line exist perhaps only for a single passage.
One major challenge for all novel RNA or DNA-based drugs, especially for the nervous system, is delivery. In a lab-based setting, plasmid DNA or ‘naked’ RNA can effectively be delivered to fast-growing cell lines to test a technology. Delivery to neurons in vitro is already more difficult, but possible using electroporation, viral vectors such as rAAV or LV and non-viral lipid, polymer or metal nanoparticles [37]. In in vivo models for neurological diseases, viral carriers are currently still the method of choice for delivery in the case of rodents and non-human primates, but safety aspects with regards to unintentional cargo integration and immune responses can occur, especially after multiple or increased doses.
There have been many improvements to the initial rAAV capsids allowing targeted delivery to GABAergic neurons for example [38], but the cargo size limits everything above 4 kilobases, given that a promoter should be included as well. AAV is already
used as a delivery tool for the first genetic drugs to human patients shown by the recent Zolgensma and Luxturna gene therapies [39] and RNA therapeutic (ASO) Nusinersen [40]. Both Zolgensma and Nusinersen are designed to infect motorneurons in the spinal cord, and in contrast to Nusinersen, which is administered intrathecally, Zolgensma can be injected intravenous [41]. Preliminary findings for both drugs are promising, but long-term effects are currently being investigated [40,41]. From the patient perspective it is clear that intravenous administration is preferred, but if, and how well rAAV can also reach the CNS remains to be seen.
LV have a larger packaging limit, but integrate their cargo in the host-cell genome, which could be an outcome for permanent gene delivery, or RNA-based drugs that require repeated dosing, but is not useful for gene editing. For gene editing, the best of both worlds would be a non-toxic virus that allows transient expression. We tried to package all the PE components in a single integration-deficient LV in Chapter 8, which pushes the boundaries of LV vectors. We found marginal GFP expression and edit rates, meaning that a transient PE virus can be generated, although this current one is far from perfect.
The aim of the research presented in this thesis was to investigate novel therapies for DEE. These consist of novel VGSC blockers and activators that act at the protein level, gene enhancement and gene regulation-based technologies that act at the expression/mRNA level, and gene editing that is aimed at the cause of DEE: the genomic DNA. Each of these three levels at which medication can act and the model systems used per study have advantages and disadvantages. Any medication that is given systemically may lead to side-effects, and the more often it is administered, the more severe and chronic its adverse effects will be. Current and future protein and mRNA-based therapies still rely on repeated dosing throughout life, posing perhaps a milder, but similar drawback. In addition, a single dose of RNA therapeutic, or even gene delivery likely leads to uneven distribution to target cells. Some cells can receive many more copies than the other, and while aiming for gene upregulation or compensation of a LOF mutation for example, the result might be overexpression and even GOF with unknown consequences. For gene delivery (or gene therapy, or gene replacement) in particular, mimicking the regulation of the endogenous gene is difficult or perhaps even impossible using current methods. Gene therapy is typically achieved via promoter-driven plasmids, which integrate in the genome, or reside in the nucleus as extrachromosomal DNA. The promoter that drives the gene, for example SCN1A can be made neuron-specific by using hSyn1, but this by far does not compare to the tightly regulated, multi-TSS, 70kb-stretching 5’ UTR of endogenous SCN1A
If we truly want to revolutionize the therapeutic landscape for monogenic diseases,
I believe we should be bold and start focusing on the origin of disease and by doing so, most, if not all of the above arguments can be ruled out. The road from genetic disease to corrected gene may be long, and it is likely that early gene-editing drugs will not be perfect precursors, but at least effort is put into the correct challenge: repairing the DNA. Once gene editing becomes more of a routine procedure in the lab, and the technology is further optimized, more complex issues such as the age of treatment vs age of onset can be tested. DEE, which typically has an age of onset within in the first years of life, can be a typically challenging disease as the brain is rapidly developing during this time. Neurons with a mutated VGSC gene might have already differentiated, and little is known about how fast the turnover of VGSC proteins in vivo is. It may be possible that the DNA is repaired, but the corrected proteins are translated at later stages, meaning that a short-term effect is not a guarantee. The rapid development during the first years of life may also pose an advantage as neuronal progenitor cells and stem cells are still dividing, likely facilitating drug delivery.
One of the more practical challenges of genetic drugs is their cost. Zolgensma, one of the first effective gene therapies in the world, is currently the most expensive drug in the world, even though its price dropped from 2 to 1 million euros per dose in 2021 [42]. Given that synthetic RNA and DNA can be synthesized for researchers for as little as €0.07 per basepair, the complete SMN2 gene that makes up the majority of Zolgensma can theoretically be ‘purchased’ for approximately €100. It is clear that research-grade RNA or DNA cannot be compared to fully safety-tested and optimized drugs, but shown by the rapid development and low cost of mRNA vaccines during the CoViD19 pandemic (well under 10$ per dose [43] ), it must be possible to reduce pricing in the future years. Since RNA and DNA is much easier to synthesize than recombinant proteins, and can be made patient-specific by changing a single SNV, they are very likely candidates for future medication.
In parallel, increased next generation sequencing efforts of patients once they arrive at the hospital (the ‘Genome-first’ approach), or perhaps the whole population of a country such as the genome of Iceland project [44], could be promoted. This can identify the carriers of recessive DEE mutations, which make up approximately 50% of the DEE landscape (see also Chapter I Figure 2). Moving to the near future, I vouch for immediate DNA diagnosis of children after an initial convulsion to determine if a DEE mutation is present. Based on already existing functional data a LOF or GOF effect can be predicted to which the type of ASD can be tailored to.
Selective Nav1.6 blockers and Nav1.1 activators can compensate for loss of zNav1.1b in scn1Lab knockout zebrafish models for Dravet Syndrome.
CRISPRa can enhance VGSC gene expression during early development, but is accompanied with toxicity due to the dCas9-VPR/VP64 fusion.
Zebrafish duplicated orthologue genes for SCN1A are not identical in function, questioning the validity of zebrafish models for Dravet Syndrome.
Common variants in the promoter region of SCN1A can affect endogenous gene expression levels, altering the severity of disease.
Small activating RNAs were discovered for KCNB1, CHD2, SCN2A and SCN3A and show cell-type and promoter-type specific up-or downregulation.
Prime Editing can be used to remove clinically relevant KCNQ2 mutations in patientderived model systems using a novel all-in-one editing system.
Richards, K. L., Milligan, C. J., Richardson, R. J., Jancovski, N., Grunnet, M., Jacobson, L. H., Undheim, E., Mobli, M., Chow, C. Y., Herzig, V., Csoti, A., Panyi, G., Reid, C. A., King, G. F., & Petrou, S. (2018). Selective NaV1.1 activation rescues Dravet syndrome mice from seizures and premature death. Proceedings of the National Academy of Sciences of the United States of America, 115(34), E8077–E8085. https://doi.org/10.1073/pnas.1804764115
Frederiksen, K., Lu, D., Yang, J., Jensen, H. S., Bastlund, J. F., Larsen, P. H., Liu, H., Crestey, F., Dekermendjian, K., Badolo, L., Laursen, M., Hougaard, C., Yang, C., Svenstrup, N., & Grunnet, M. (2017). A small molecule activator of Nav 1.1 channels increases fastspiking interneuron excitability and GABAergic transmission in vitro and has anticonvulsive effects in vivo. The European journal of neuroscience, 46(3), 1887–1896. https://doi.org/10.1111/ejn.13626
Weuring WJ, Singh S, Volkers L, Rook MB, van ‘t Slot RH, et al. (2020) NaV1.1 and NaV1.6 selective compounds reduce the behavior phenotype and epileptiform activity in a novel zebrafish model for Dravet Syndrome. PLOS ONE 15(3): e0219106. https://doi.org/10.1371/journal.pone.0219106
Weuring WJ, Hoekman JW, Braun KPJ, Koeleman BPC. Genetic and Functional Differences between Duplicated Zebrafish Genes for Human SCN1A. Cells. 2022; 11(3):454. https://doi.org/10.3390/cells11030454
Clinicaltrials.gov: NCT03467100
Adrian C. Thompson, Carlos D. Aizenman. Regulation of Nav1.6-mediated sodium currents underlie the homeostatic control of neuronal intrinsic excitability in the optic tectum of the developing Xenopus laevis tadpole. bioRxiv 2021.10.07.463558; doi: https://doi.org/10.1101/2021.10.07.463558
Chow, C. Y., Chin, Y., Ma, L., Undheim, E., Herzig, V., & King, G. F. (2020). A selective NaV1.1 activator with potential for treatment of Dravet syndrome epilepsy. Biochemical pharmacology, 181, 113991.
https://doi.org/10.1016/j.bcp.2020.113991
Long, L., Guo, H., Yao, D., Xiong, K., Li, Y., Liu, P., Zhu, Z., & Liu, D. (2015). Regulation of transcriptionally active genes via the catalytically inactive Cas9 in C. elegans and D. rerio. Cell research, 25(5), 638–641. https:// doi.org/10.1038/cr.2015.35
Colasante, G., Lignani, G., Brusco, S., Di Berardino, C., Carpenter, J., Giannelli, S., Valassina, N., Bido, S., Ricci, R., Castoldi, V., Marenna, S., Church, T., Massimino, L., Morabito, G., Benfenati, F., Schorge, S., Leocani, L., Kullmann, D. M., & Broccoli, V. (2020). dCas9-Based Scn1a Gene Activation
Restores Inhibitory Interneuron Excitability and Attenuates Seizures in Dravet Syndrome
Mice. Molecular therapy : the journal of the American Society of Gene Therapy, 28(1), 235–253.
https://doi.org/10.1016/j.ymthe.2019.08.018
Yamagata, T., Raveau, M., Kobayashi, K., Miyamoto, H., Tatsukawa, T., Ogiwara, I., Itohara, S., Hensch, T. K., & Yamakawa, K. (2020). CRISPR/dCas9-based Scn1a gene activation in inhibitory neurons ameliorates epileptic and behavioral phenotypes of Dravet syndrome model mice. Neurobiology of disease, 141, 104954. https://doi.org/10.1016/j.nbd.2020.104954
Dong, Z. F., Tang, L. J., Deng, G. F., Zeng, T., Liu, S. J., Wan, R. P., Liu, T., Zhao, Q. H., Yi, Y. H., Liao, W. P., & Long, Y. S. (2014). Transcription of the human sodium channel SCN1A gene is repressed by a scaffolding protein RACK1. Molecular neurobiology, 50(2), 438– 448.
https://doi.org/10.1007/s12035-014-8633-9
Chen, Y. H., Liu, S. J., Gao, M. M., Zeng, T., Lin, G. W., Tan, N. N., Tang, H. L., Lu, P., Su, T., Sun, W. W., Xie, L. C., Yi, Y. H., & Long, Y. S. (2017). MDH2 is an RNA binding protein involved in downregulation of sodium channel Scn1a expression under seizure condition. Biochimica et biophysica acta. Molecular basis of disease, 1863(6), 1492–1499.
https://doi.org/10.1016/j.bbadis.2017.04.018
Zhang, B., Tornmalm, J., Widengren, J., Vakifahmetoglu-Norberg, H., & Norberg, E. (2017). Characterization of the Role of the Malate Dehydrogenases to Lung Tumor Cell Survival. Journal of Cancer, 8(11), 2088–2096. https://doi.org/10.7150/jca.19373
Ait-El-Mkadem, S., Dayem-Quere, M., Gusic, M., Chaussenot, A., Bannwarth, S., François, B., Genin, E. C., Fragaki, K., Volker-Touw, C., Vasnier, C., Serre, V., van Gassen, K., Lespinasse, F., Richter, S., Eisenhofer, G., Rouzier, C., Mochel, F., De Saint-Martin, A., Abi Warde, M. T., de Sain-van der Velde, M., PaquisFlucklinger, V. (2017). Mutations in MDH2, Encoding a Krebs Cycle Enzyme, Cause EarlyOnset Severe Encephalopathy. American journal of human genetics, 100(1), 151–159. https://doi.org/10.1016/j.ajhg.2016.11.014
Chen, L., Min, L., Wang, X., Zhao, J., Chen, H., Qin, J., Chen, W., Shen, Z., Tang, Z., Gan, Q., Ruan, Y., Sun, Y., Qin, X., & Gu, J. (2015). Loss of RACK1 Promotes Metastasis of Gastric Cancer by Inducing a miR-302c/IL8 Signaling Loop. Cancer research, 75(18), 3832–3841. https:// doi.org/10.1158/0008-5472.CAN-14-3690
Xiao, T., Zhu, W., Huang, W., Lu, S. S., Li, X. H., Xiao, Z. Q., & Yi, H. (2018). RACK1 promotes tumorigenicity of colon cancer by inducing cell autophagy. Cell death & disease, 9(12), 1148.
https://doi.org/10.1038/s41419-018-1113-9
Mao, Q., Li, Y., Zheng, X., Yang, K., Shen, H., Qin, J., Bai, Y., Kong, D., Jia, X., & Xie, L. (2008). Up-regulation of E-cadherin by small activating RNA inhibits cell invasion and migration in 5637 human bladder cancer cells. Biochemical and biophysical research communications, 375(4), 566–570. https:// doi.org/10.1016/j.bbrc.2008.08.059
Clinicaltrials.gov: NCT04601051
Clinicaltrials.gov: NCT03872479
Chen, P. J., Hussmann, J. A., Yan, J., Knipping, F., Ravisankar, P., Chen, P. F., Chen, C., Nelson,
J. W., Newby, G. A., Sahin, M., Osborn, M. J., Weissman, J. S., Adamson, B., & Liu, D. R. (2021). Enhanced prime editing systems by manipulating cellular determinants of editing outcomes. Cell, 184(22), 5635–5652. e29. https://doi.org/10.1016/j.cell.2021.09.018
Prime Edit plasmid pCMV-PE2 / https://www.addgene.org/132775/
Brakemeier, S., Stolte, B., Kleinschnitz, C., & Hagenacker, T. (2022). Treatment of Adult Spinal Muscular Atrophy: Overview and Recent Developments. Current pharmaceutical design, 10.2174/1381612 828666220329115433. Advance online publication. https://doi.org/10.2174/13816128 28666220329115433
Zhang, Y., Kecskés, A., Copmans, D., Langlois, M., Crawford, A. D., Ceulemans, B., Lagae, L., de Witte, P. A., & Esguerra, C. V. (2015). Pharmacological characterization of an antisense knockdown zebrafish model of Dravet syndrome: inhibition of epileptic seizures by the serotonin agonist fenfluramine. PloS one, 10(5), e0125898. https://doi.org/10.1371/journal.pone.0125898
Lagae, L., Sullivan, J., Knupp, K., Laux, L., Polster, T., Nikanorova, M., Devinsky, O., Cross, J. H., Guerrini, R., Talwar, D., Miller, I., Farfel, G., Galer, B. S., Gammaitoni, A., Mistry, A., Morrison, G., Lock, M., Agarwal, A., Lai, W. W., Ceulemans, B., FAiRE DS Study Group (2019). Fenfluramine hydrochloride for the treatment of seizures in Dravet syndrome: a randomised, double-blind, placebo-controlled trial. Lancet (London, England), 394(10216), 2243–2254. https://doi. org/10.1016/S0140-6736(19)32500-0
Langova, V., Vales, K., Horka, P., & Horacek, J. (2020). The Role of Zebrafish and Laboratory Rodents in Schizophrenia Research. Frontiers in psychiatry, 11, 703. https://doi.org/10.3389/fpsyt.2020.00703
Cho, S. J., Byun, D., Nam, T. S., Choi, S. Y., Lee, B. G., Kim, M. K., & Kim, S. (2017). Zebrafish as an animal model in epilepsy studies with multichannel EEG recordings. Scientific reports, 7(1), 3099. https://doi.org/10.1038/
s41598-017-03482-6
Griffin, A., Hamling, K. R., Knupp, K., Hong, S., Lee, L. P., & Baraban, S. C. (2017). Clemizole and modulators of serotonin signalling suppress seizures in Dravet syndrome. Brain, aww342. https://doi.org/10.1093/brain/aww342
Clinicaltrials.gov: NCT04462770
Njoku D. B. (2013). The immortal life of Henrietta Lacks. Anesthesia and analgesia, 117(1), 286. https://doi.org/10.1213/ ANE.0b013e31828bfecc
ATCC: CCL-2
ATCC: CRL-1573
ATCC: CRL-2266
Merten, O. W., Hebben, M., & Bovolenta, C. (2016). Production of lentiviral vectors. Molecular therapy. Methods & clinical development, 3, 16017. https://doi.org/10.1038/mtm.2016.17
Hildebrandt, M. R., Reuter, M. S., Wei, W., Tayebi, N., Liu, J., Sharmin, S., Mulder, J., Lesperance, L. S., Brauer, P. M., Mok, R., Kinnear, C., Piekna, A., Romm, A., Howe, J., Pasceri, P., Meng, G., Rozycki, M., Rodrigues, D. C., Martinez, E. C., Szego, M. J., … Ellis, J. (2019). Precision Health Resource of Control iPSC Lines for Versatile Multilineage Differentiation. Stem cell reports, 13(6), 1126–1141. https://doi.org/10.1016/j.stemcr.2019.11.003
Nethercott, H. E., Brick, D. J., & Schwartz, P. H. (2011). Derivation of induced pluripotent stem cells by lentiviral transduction. Methods in molecular biology (Clifton, N.J.), 767, 67–85. https://doi.org/10.1007/978-1-61779-201-4_6
Sepehrimanesh, M., & Ding, B. (2020). Generation and optimization of highly pure motor neurons from human induced pluripotent stem cells via lentiviral delivery of transcription factors. American journal of physiology. Cell physiology, 319(4), C771–C780. https://doi.org/10.1152/ajpcell.00279.2020
Ngowi, E. E., Wang, Y. Z., Qian, L., Helmy, Y., Anyomi, B., Li, T., Zheng, M., Jiang, E. S., Duan, S. F., Wei, J. S., Wu, D. D., & Ji, X. Y. (2021).
The Application of Nanotechnology for the Diagnosis and Treatment of Brain Diseases and Disorders. Frontiers in bioengineering and biotechnology, 9, 629832. https://doi.org/10.3389/fbioe.2021.629832
Dimidschstein, J., Chen, Q., Tremblay, R., Rogers, S. L., Saldi, G. A., Guo, L., Xu, Q., Liu, R., Lu, C., Chu, J., Grimley, J. S., Krostag, A. R., Kaykas, A., Avery, M. C., Rashid, M. S., Baek, M., Jacob, A. L., Smith, G. B., Wilson, D. E., Kosche, G., Fishell, G. (2016). A viral strategy for targeting and manipulating interneurons across vertebrate species. Nature neuroscience, 19(12), 1743–1749.
https://doi.org/10.1038/nn.4430
Keeler, A. M., & Flotte, T. R. (2019). Recombinant Adeno-Associated Virus Gene Therapy in Light of Luxturna (and Zolgensma and Glybera): Where Are We, and How Did We Get Here?. Annual review of virology, 6(1), 601–621. https://doi.org/10.1146/annurevvirology092818-015530
Mendell, J. R., Al-Zaidy, S. A., Lehman, K. J., McColly, M., Lowes, L. P., Alfano, L. N., Reash, N. F., Iammarino, M. A., Church, K. R., Kleyn, A., Meriggioli, M. N., & Shell, R. (2021). Five-Year Extension Results of the Phase 1 START Trial of Onasemnogene Abeparvovec in Spinal Muscular Atrophy. JAMA neurology, 78(7), 834–841. https://doi.org/10.1001/jamaneurol.2021.1272
Acsadi, G., Crawford, T. O., Müller-Felber, W., Shieh, P. B., Richardson, R., Natarajan, N. Castro, D., Ramirez-Schrempp, D., Gambino, G., Sun, P., & Farwell, W. (2021). Safety and efficacy of nusinersen in spinal muscular atrophy: The EMBRACE study. Muscle & nerve, 63(5), 668–677. https://doi. org/10.1002/mus.27187
Nuijten M. (2021). Pricing Zolgensmathe world’s most expensive drug. Journal of market access & health policy, 10(1), 2022353. https://doi.org/10.1080/20016689. 2021.2022353
Light DW, Lexchin J. The costs of coronavirus vaccines and their pricing. J R Soc Med. 2021;114(11):502-504. doi:10.1177/01410768211053006
44.
Gudbjartsson, D., Helgason, H., Gudjonsson, S. et al. Large-scale whole-genome sequencing of the Icelandic population. Nat Genet 47, 435–444 (2015).
https://doi.org/10.1038/ng.3247
Since the rise of DNA sequencing technologies, it became apparent that the majority of diseases in humankind have – at least partly – a genetic origin.Many types of Epilepsy are also caused by DNA mutations. A DNA mutation in turn, is transcribed in messenger RNA (mRNA), which is translated to a malfunctioning protein. In the case of epilepsy, the malfunctioning protein can be a channel in the cell membrane of neurons, which would normally regulate how neurons communicate with each other. When malfunctioning, the neuronal excitability is changed with epileptic seizures as a result. Current treatment of epilepsy – antiseizure medication - is aimed at the consequence of a DNA mutation; the malfunctioning protein. In this thesis we set out to improve epilepsy treatment by targeting three levels of treatment; the protein, the mRNA, and the DNA.
As an improvement to current channel-blocking protein-based drugs, we set out to improve selectivity by testing novel compounds in a zebrafish model of genetic epilepsy. Two types of compounds successfully improved the seizure phenotype; one that reduces activity of an ‘overactive’ channel-protein, and one that increases the activity of a channel-protein that is working less efficiently. Next, we tested three different technologies to enhance the expression of epilepsy genes, with the aim of altering mRNA levels to compensate for the malfunctioning protein. We found that, while some of them work, there was toxicity that should be further studied before next steps towards possible implementation can be considered. Finally, we tested if two known DNA mutations that lead to epilepsy can be repaired using geneediting technologies. In patient-derived stem cells, we successfully removed the DNA mutation, highlighting that in theory this would be a potential and powerful treatment option of genetic epilepsies in the future.
Sinds de opkomst van DNA sequencing technologieën is bekend geworden dat een groot deel van de ziektes in de mens veroorzaakt wordt door mutaties in ons DNA. Een bekend voorbeeld is kanker, maar epilepsie valt hier ook onder. Epilepsie wordt gekenmerkt door aanvallen, of insulten, die door verstoringen in het brein veroorzaakt worden. Deze verstoringen vinden plaats tussen de hersencellen. Op deze cellen bevinden zich openingen met een doorvoer die ervoor zorgen dat signaal-en voedingsstoffen, zoals elektrolyten van de ene cel naar de andere cel overgebracht kunnen worden. Deze doorvoer “kanaaltjes” zijn eiwitten, en worden op hun beurt, zoals ieder eiwit in het menselijk lichaam, door DNA gemaakt. Een enkele fout in het DNA kan ervoor zorgen dat het eiwit niet meer goed functioneert, zoals het teveel doorlaten van elektrolyten, een volledige stop van doorvoer of een haperende doorvoer waardoor hersencellen overprikkeld raken met een epileptische aanval als gevolg. Terwijl de oorzaak van deze soort epilepsie al jaren bekend is (de DNA mutatie) bestaat behandeling voornamelijk uit het bestrijden van de consequentie (het verkeerde functioneren eiwit). In dit proefschrift heb ik geprobeerd een opstap te maken voor nieuwe behandelingen van epilepsie, specifiek gericht op het eiwit dat gemuteerd is, op een signaal molecuul dat de instructies bevat voor het eiwit (het RNA) en als laatste een mogelijke behandeling specifiek gericht op de oorzaak van epilepsie; de DNA mutatie.
Om te testen of dit zou kunnen werken heb ik met mijn team voor elke van deze drie soorten mogelijke behandeling (gericht op eiwit, RNA en DNA) experimenten uitgevoerd, en het volgende ontdekt. 1) Het verhogen van de specificiteit van eiwitblokkerende moleculen kan behulpzaam zijn bij de behandeling van epilepsie, 2) synthetische, korte RNA moleculen kunnen de expressie van epilepsie-genen beïnvloeden en daarmee mogelijk het effect van de DNA mutatie milder maken en 3) met de nieuwste ‘Gene-Edit’ technologie gebaseerd op CRISPR/Cas9 bekend van o.a. de volkskrant en NRC, kunnen we mutaties die epilepsie veroorzaken repareren. Belangrijk om te weten is dat deze experimenten in het laboratorium zijn uitgevoerd in een petrischaal en nog niet volledig getest zijn voor de veiligheid in patienten.
A. B. C. D. E. F. G.
29/05/2019 Influence of common SCN1A promoter variants on the severity of SCN1A-related Phenotypes Iris M. de Lange, Wout J. Weuring, Ruben van ‘t Slot, Boudewijn Gunning, Anja C. M. Sonsma, Mark McCormack, Carolien de Kovel, Lisette J. J. M. van Gemert, Flip Mulder, Marjan J. A. van Kempen, Nine V. A. M. Knoers, Eva H. Brilstra, and Bobby P. C. Koeleman - Molecular Genetics and Genomic Medicine
05/03/2020 NaV1.1 and NaV1.6 selective compounds reduce the behavior phenotype and epileptiform activity in a novel zebrafish model for Dravet Syndrome Wout J. Weuring, Sakshi Singh,Linda Volkers,Martin B. Rook,Ruben H. van ‘t Slot,Marjolein Bosma,Marco Inserra,Irina Vetter,Nanda M. Verhoeven Duif,Kees P. J. Braun,Mirko Rivara,Bobby P. C. Koeleman. - Plos One
09/12/2021 Istaroxime treatment ameliorates calcium dysregulation in a zebrafish model for Phospholamban R14del cardiomyopathy Sarah Kamel , Dr Chantal van Opbergen , Lotte Koopman , Arie Verkerk , Bas Boukens , Mr Berend de Jonge , Ms Yeszamin Onderwater , Eva van Alebeek , Sonja Chocron , Clarissa Polidoro , Wout J. Weuring ,Marc Vos , Dr. Teun de Boer , Dr. Toon van VeenNature communications
10/08/2021 CRISPRa-mediated upregulation of scn1laa during early development causes epileptiform activity and dCas9-associated toxicity Wout J. Weuring Ivana Dilevska, Jos Hoekman, Joep van de Vondervoort, Martijn Koetsier, Ruben H. van ’t Slot, Kees P.J.Braun, Bobby P. C. Koeleman - CRISPR Journal
22/10/2021 Gene Therapies for Monogenic Autism Spectrum Disorders Weuring WJ, Geerligs J,Koeleman BPC. - Genes
30/12/2021 Genetic and Functional Differences between Duplicated Zebrafish Genes for Human SCN1A Wout J. Weuring, Jos W. Hoekman ,Kees P. J. Braun and Bobby P. C. Koeleman - Cells
Submitted 14/04/2022 Efficient, easy and safe prime editing strategy to correct or induce gene edits in hiPSC using a single Ef-1α driven all-in-one plasmid Weuring Wout J.†, Dirkx N † (shared first author), De Vriendt E, Smal N, van de Vondervoort J , van ’t Slot Ruben , Koetsier M , Braun Kees JP, Weckhuysen S, Koeleman Bobby PC – Submitted Nucleic Acids Research
Dit proefschrift zou niet mogelijk kunnen zijn zonder de hulp van een grote groep mensen:
Als eerst wil ik graag de leden van de beoordelingcommissie Dr. S.A. Fuchs, Prof. dr. W.L. van der Pol, Prof. dr. N.N. Kasri, Prof. dr. J. Verhaagen en Prof. dr. R.J. Pasterkamp bedanken voor het lezen en beoordelen van dit proefschrift. Prof. dr. D. Bär, bedankt voor het leiden van de ceremonie.
Ik heb het geluk gehad om met twee fantastische supervisoren te werken; mijn promotor Prof. K.P.J. Braun en copromotor Dr. B.P.C. Koeleman die mij het vertrouwen gunden en wegwijs hebben gemaakt in de medisch-academische wereld. Kees, bedankt voor alle tijd en energie die je hebt geïnvesteerd in de begeleiding van mijn onderzoek. Je positieve houding bij onze vergaderingen, kritische vragen en zorgvuldige, snelle commentaar op manuscripten waren voor mij een onmisbare steun en aanmoediging om verder te gaan en af te maken wat we begonnen zijn. Bobby, jij hebt de start van mijn academische carrière mogelijk gemaakt, dank daarvoor. Je enthousiasme voor de wetenschap en onuitputtelijke voorraad ideeën voor onderzoek zijn ontzettend inspirerend. Ik kijk ook met veel plezier terug op de zeiltocht, duiksessie, Muay Thai wedstrijd en diners in binnen- en buitenland.
Aan alle coauteurs; dankjewel voor jullie bijdragen aan de artikelen die tot stand zijn gekomen, onderzoek doen gaat makkelijker en sneller als je het samen doet en jullie waren onmisbaar.
Martijn, Ruben, Joep, Flip & Remi, ik heb genoten van de tijd die we gedeeld hebben in de Koeleman groep, bedankt! Martijn, jij stond altijd
klaar om in het lab te helpen en hebt een grote bijdrage aan het experimentele werk geleverd. Daarnaast voelt het alsof we op elkaar kunnen bouwen, dat is prettig samenwerken! Vinicius, Glen, Daniel, Jessica and Mircea thank you for making a warm welcome in the flex-room! Edith, Karen, Helen, Laura, Iris, Carlo, Marc, Roy, Luca, Stephan en Martin bedankt voor de leuke jaren in het lab en daarbuiten. Luan, I am really happy we met and that we share our passion for climbing. The 3-weekly sessions at Neoliet, but also rock climbing in Slovenia were really good deviations from the rush hours of PhD and helped me stay healthy and focused. I hope we can continue improving together and go for that 7B, Thanks buddy!
Prof. J.P.W.M. Bakkers, Federico Tessadori, Mark Reijnen en alle dierverzorgers bedankt voor het helpen opzetten, testen en uitvoeren van in vivo experimenten in het Hubrecht Instituut.
Mijn vrienden en familie zijn onmisbaar in het dankwoord, bedankt voor jullie steun en afleiding de afgelopen jaren. Ian, maatje. Wat tof dat jij je tijd en vaardigheden hebt willen gebruiken voor de taalbewerking van mijn proefschrift. Slàinte a charaid! Lonneke wat een tijd en energie heb je gestoken in het ontwerpen van de stijl, leesbaarheid en cover van dit proefschrift. Je hebt heel snel een lastig technisch onderwerp weten vorm te geven in een grafische analogie. Dankjewel! Yuri en Koen, jullie zijn prachtige paranimfen. De rest van de Gnoes; bedankt! Papa, Mama, Judith en Lonneke; het is mooi om samen Weuring-Kroesen te zijn. Papa en Mama wat een fijne mensen zijn jullie, door jullie vertrouwen, nature (genen) en nurture (opvoeding) heb ik zover kunnen komen. Danaë dankjewel voor dat we samen zijn. Alle mooie momenten die we gedeeld hebben maken dat ik een punt kan zetten achter dit proefschrift. Ik zie dat we een mooie tijd tegemoet gaan met de kleine.
Wouter Jan Weuring is geboren op 22 November 1989 te Apeldoorn, Nederland. Hij rondde zijn opleiding hoger laboratorium onderwijs (Life Sciences) af aan de Hogeschool Utrecht. Via een minor aan de Vrije Universiteit Amsterdam werd hij ingeloot voor de Master Biomedische Wetenschappen aan de Universiteit Utrecht en haalde zijn diploma in 2015. Door dit pad te kiezen is er van zes jaar studietijd bijna twee en een half jaar besteed aan stages in binnen- en buitenland, resulterend in een brede interesse voor moleculair biologisch onderzoek. De twee meest uiteenlopende projecten betreffen de ontwikkeling van een moleculaire biosensor voor het meten van short-chain fatty acids bij TNO onder leiding van Dr. G.Roeselers en het analyseren van microbiota in het spijsverteringkanaal van G.portentosa in het Cavanilles Instituut, Valencia, onder leiding van Dr. E. Maiques.
In 2015 is hij aangenomen als wetenschappelijk medewerker onderzoek door Dr. B. Koeleman in het UMC Utrecht. Tijdens dit jaar heeft hij verschillende technieken getest en geïmplementeerd waaronder het maken, onderhouden en uitvoeren van experimenten met de zebravis als proefdier. In 2016 kreeg hij de mogelijkheid om in te stromen in het tweede jaar van de Flamenco Gitaar opleiding aan de Fundacion Christina Heeren in Sevilla, Spanje.
Bij terugkomst in Nederland werd hij uitgenodigd om promotieonderzoek te doen naar monogenetische epilepsie in het laboratorium van Dr. B. Koeleman. Naast analytische- en onderzoeksvaardigheden heeft hij een breed scala aan technieken getest en geïmplementeerd om moleculair biologische vraagstukken te beantwoorden, waaronder de nieuwste CRISPR gene-editing technologie Prime Editing. Hij heeft negen stagiaires begeleid tijdens hun BSc., Msc. of SUMMA onderzoeks stages en de basiskwalificatie onderwijs behaald tijdens het zes maanden durende full-time PhD Teaching Talent programma.
Door zijn promotieonderzoek bij een UMC te doen is de nadruk op de patiënt steeds belangrijker geworden. Dit gaf de doorslag om als Scientist de nieuwe generatie gentherapieën te ontwikkelen bij uniQure, zijn huidige werkgever. Hij woont in Utrecht met zijn vriendin Danaë en twee katten.