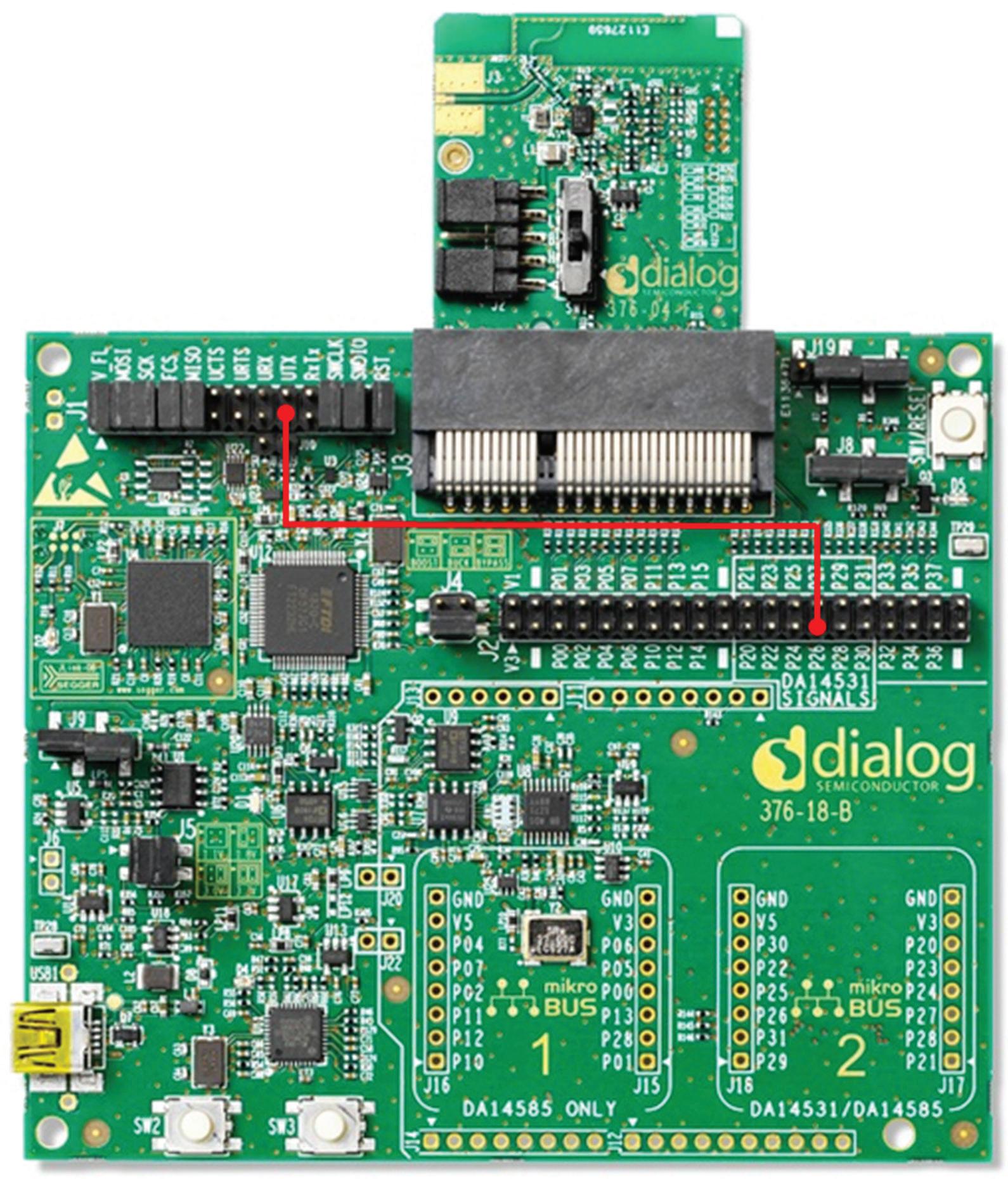
5 minute read
Developing connected medical devices for the IoT
Adrie Van Meijeren, Low Power Connectivity • Dialog Semiconductor
Super-small radio SoCs are being paired with innovative battery technologies to bring inexpensive medical electronics online.
The Internet of Things (IoT) has disrupted many industriesin short order. However, when it comes to adopting the IoT, themedical and pharmaceutical space has largely been held back.
It’s not entirely surprising. The high level of regulation in the medical field and the (literal) life-or- death stakes of introducing new technologies for patient care understandable lengthen development cycles for new medical devices. But engineering roadblocks around power, size and cost have been the biggest factors in making widespread development and adoption prohibitive for disposable connected medical devices.
There is a path forward, though, for developers who want to devise IoT-based medical designs that meet the necessary size, power and cost requirements.
One of the major roadblocks to developing disposable connected medical devices is cost. It can be a prohibitively expensive venture to create designs in a small form factor that integrate a system-on-chip (SoC) and the necessary external components for, say, measuring blood pressure or glucose levels or inhaling medicine. That cost is driven up by the need for components like two crystals rather than a single low-power version; four-layer PCBs rather than cheaper and simpler two-layer boards; and costly batteries. As long as the bill of materials (BOM) remains high and the product isn’t miniaturized, mass market adoption of connected medical devices will slow to a crawl.
In addition to BOM cost, medical designers often must contend with power consumption problems. Medical disposable products must last a long time. Shelf lives of 18 months up to several years are not unusual, followed with a relatively short active life measured in weeks to months. During its time on the shelf, battery capacity can drop from both self-discharge and leakage current to the application itself. Once active life starts, the battery may not have enough capacity available to support it. Clearly, both patients and doctors need IoT medical devices to be dependable – both to treat the patient and also to provide the data necessary to ensure dosages and tests happen correctly.
Finally, there’s the disposability issue. The nature of disposable medical devices is that they will only be used for anywhere between 14 days and two months. Given that short lifetime and their cost, insurance companies are naturally reluctant to support them.
The answer to all these challenges lies in the battery and specifically, in implementing disposable silver-oxide or printed batteries. Recently both high-energy thin film lithium batteries and printed rechargeable zinc batteries have become commercially available. But there are questions about whether or not these technologies are ready for mass deployment.
The fabrication of batteries via 3D printing has several advantages over conventional battery fabrication technologies. For one thing, battery components may be printed directly on the PCB holding the rest of the electronics. Thus there is the possibility of eliminating assembly and packaging steps that discrete batteries require. Additionally, the printing process can also conceivably fabricate complex battery architectures that may be impractical via other means. Printing methods can adjust the shape and thickness of the electrodes and print solid-state electrolyte that is stable and safe.
Printed zinc batteries look promising. One such device from Impact Energy uses a High Conductivity Polymer Electrolyte (HCPE) that is stable, rechargeable, and does not need a sealed container. Because the chemistry is based on zinc rather than lithium, it avoids the safety issues associated with many lithium technologies In additoin, lithium titanate (LTO) and lithium iron phosphate (LFP) are commonly used anode and cathode materials in 3D-printed batteries, but carbon nanomaterials are promising for use as electrodes as well. Carbon nanotubes and carbon nanofibers are widely used in printing inks because of their high mechanical strength, high chemical stability, large specific surface area, and excellent electrical and thermal properties.

One example of a platform used for devising connected medical devices is Dialog Semiconductor’s Smartbond Bluetooth low energy 5.1 system-on-a-chip DA14531, visible on the daughterboard plugged into the dev kit motherboard. The DA14531 is a small, low-power SoC for beacon and tracker devices and is designed to work with any type of (disposable) battery, 3-V coin cell or 1.5-V alkaline button cells, 1.4-V zinc-air cells or even printed batteries. The DA14531 supports dc-dc peak current control, allowing operation with low capacity (e.g. <20 mAh) batteries with high internal resistance. The SoC supports 2.5-dBm output power and a 96.5-dBm link budget. Sleep current can be as low as 700 nA while using a hibernation mode with external wake up trigger. The DA14531finds use in (disposable) smart labels, beacons or trackers.
It also looks as though printed battery electrolytes will help reduce fabrication costs as well. The electrolyte serves as catalyst by promoting the movement of ions from the cathode to the anode on charge and in reverse on discharge. Electrolyte material plays a key role in electrochemical performance, cycle life, and safety of the battery.
There are still numerous challenges before printed batteries can be widely commercialized. One problem is that currently there are only a few printable active materials that can be used as inks. Additionally, much work remains to be done in characterizing how battery inks behave when patterned over top other inks. And though there has been a lot of work done on the materials for the electrodes and electrolytes, current collectors will likely need a similar amount of optimization.
Once the technology is ready, healthcare applications will likely benefit greatly from super-thin 3D-printed batteries. Skin patches using printed batteries are already commercial. Smart skin patches use laminar batteries, often partially printed, combined with printed electrode patterns to deliver drugs, cosmetics, and other chemicals through the skin. Medical diagnostic devices will likely benefit as well.
Wireless sensor/network applications will also benefit. Here, the trend is to combine energy harvesting with thin batteries to keep the package size down. Similarly, new small batteries will be a boon to battery-assisted passive RFID although coin-cells are the main power sources now. Smart card apps are another application wherein several thin-film battery technologies have been optimized for lamination into cards, though the prices are probably too high for disposable uses.
High peak currents can reduce battery capacity and lifespan. High in-rush currents can arise in dc-dc converters which tend to incorporate a high amount of capacitance on the power input to avoid voltage drops on the supply rails. When power is applied initially, the charging of these capacitors can result in an in-rush current that can exceed the nominal load current. If left unaddressed, this high current can cause the voltage rails to fall out of regulation, perhaps making the system unstable or putting it in an unpredictable state. There are various ways of limiting in-rush current. For example, some BLE devices incorporate built-in current limiters .
All in all, the review cycles for medical devices are justifiably long, and it can be several years before they can hit the mainstream. But these devices have game-changing potential for patient care, and it all starts with finally cracking these longstanding design challenges.
References: Dialog Semiconductor, www.dialog-semiconductor.com