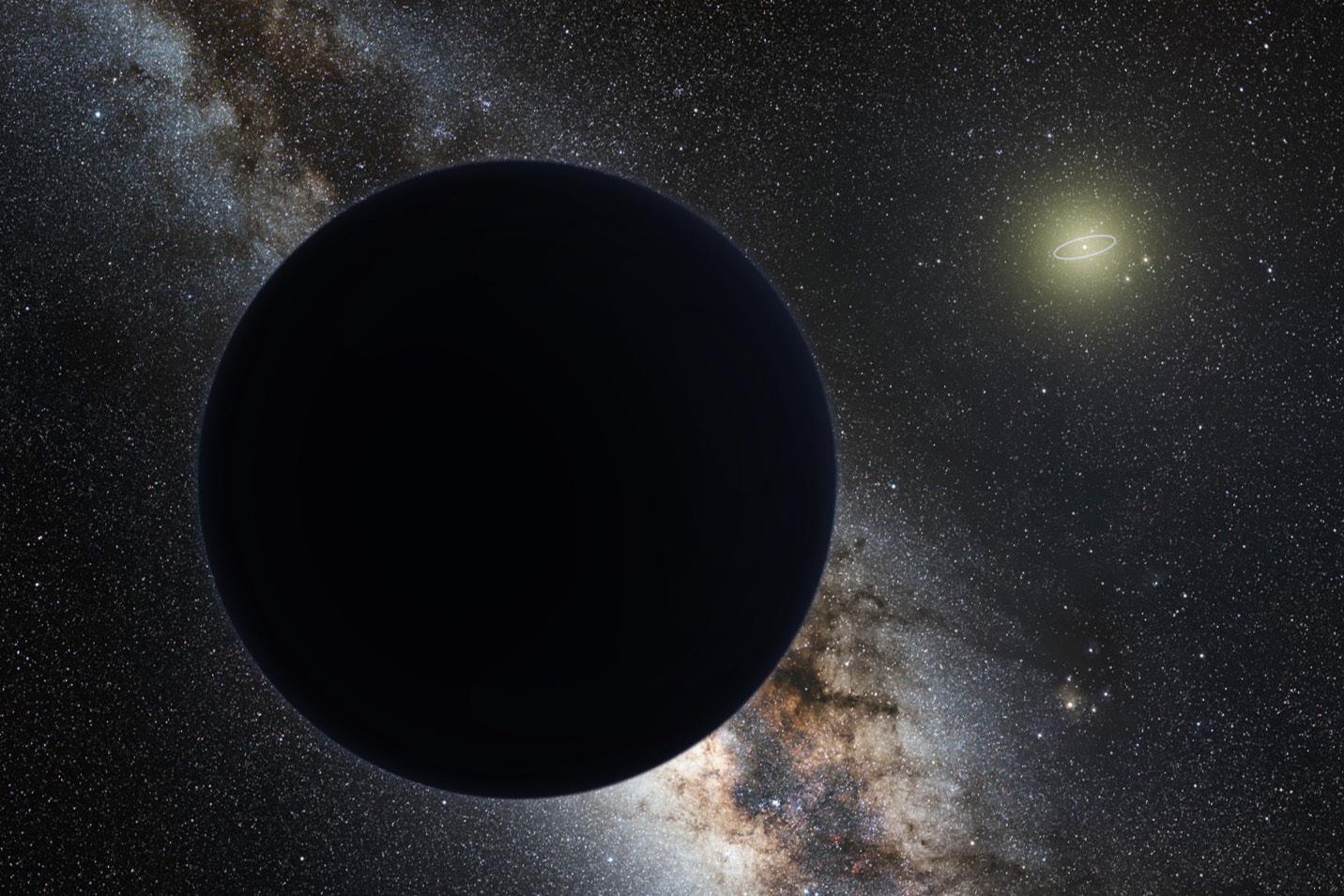
39 minute read
Individual Articles
Hunting for Planet Nine by its Influence on Neptune
BY ALEX GAVITT '23
Advertisement
Cover image: Artist’s impression of Planet Nine. The yellow ring around the Sun is Neptune’s orbit.
Source: Wikimedia Commons/ nagualdesign and Tomruen, CC-BY-SA 4.0 Abstract As more and more Kuiper belt objects have been discovered, astronomers have begun to notice that many of their orbits are aligned contrary to the expected random distribution around the Sun. Furthermore, some have extremely long or highly inclined orbits that cannot be explained by the gravitational influence of known objects. As a result, some astronomers have suggested that there may be a ninth planet, orbiting far beyond Neptune, that is influencing the orbits of these Kuiper belt objects. This paper provides scientific background for the Planet Nine hypothesis and describes a calculation of the transit timing variation (TTV) it would introduce in Neptune’s orbit. Ultimately, it finds that the TTV would be too small and occur over too long a timescale to be useful in finding Planet Nine. What is a Planet? Wanderers
The word “planet” comes from the ancient Greek word for “wanderer.” This definition offers a profound insight into humanity’s original understanding of the planets: they clearly move through the sky independent of the slow and fixed movement of the other stars. Under the geocentric model of the solar system, astronomers counted seven planets that fit this definition: Mercury, Venus, Mars, Jupiter, and Saturn, as well as the Sun and the Moon. The advent of the heliocentric solar system, then, was the first nail in the coffin of this definition, for it stripped the wandering Sun and Moon of the title “planet,” while also adding the Earth to the planetary ranks, despite its apparent lack of motion (Brown, 2010, pp. 18–21).
What sealed the fate of the “wanderer” definition was the discovery of Uranus in 1781
by the astronomer William Herschel. While he initially assumed this new object was a comet, its near-circular orbit and lack of a tail quickly prompted the realization that it was, in reality, a new planet. As Uranus is not generally visible with the naked eye, it was not one of the “wanderers” known to the ancient Greeks. Soon after the discovery of Uranus, astronomers realized that all was not well with their models of the solar system. Predictions of Uranus’ orbit from Newton’s Law of Gravitation differed noticeably from its observed orbit, suggesting that there was another object nearby, massive enough to influence Uranus’ orbit. Calculations narrowed down this object’s likely position, and astronomers soon found the planet Neptune, whose gravitational influence resolved the errors in Uranus’ orbit (Krajnović, 2016; Brown, 2010, pp. 21–24).
The Rise and Fall of Planets
Once Uranus had established the precedent for new planets, astronomers began finding many more. The first of these was Ceres, located between Mars and Jupiter; the discovery of Ceres was soon followed by the discovery of the nearby objects Pallas, Juno, and Vesta. Further discoveries of objects in this region eventually resulted in those four losing their status as planets and being reclassified as something new: asteroids, part of a vast “asteroid belt” between Mars and Jupiter. Undaunted by this reclassification, some astronomers continued to search for new planets. In 1930, the astronomer Clyde Tombaugh found the object Pluto, orbiting out past Neptune, which became the solar system’s ninth and final planet. Though Pluto is a bit irregular compared to the other planets—its orbit is more elliptical (so much so that it crosses Neptune’s orbit) and is inclined from the plane of the other planets’ orbits, and it is smaller and much less massive than even Mercury—but it was generally accepted as a planet. By the 1990s, however, astronomers began discovering other objects orbiting in the same region as Pluto, a region that became known as the Kuiper belt. The discovery of these objects raised the specter that Pluto might fall from planethood, just as Ceres and its companions had (Brown, 2010, pp. 21–27).
Pluto held on to its planetary status for about a decade and a half after the first Kuiper belt objects were discovered. In 2005, however, a team of astronomers led by Caltech professor Michael Brown discovered an object that forced the issue: a Kuiper belt object, now known as Eris, estimated at slightly larger than Pluto. Astronomers were left with a choice: either accept Eris—and possibly many more— as a planet or reclassify Pluto as something else. Eventually, in 2006, the International Astronomical Union (IAU) adopted a formal definition of a planet, making the first time that explicit requirements for planethood had been laid down. Under the IAU definition, a planet in the solar system must (IAU, 2006):
1. Be in orbit around the Sun; 2. Have sufficient mass for its self-gravity to overcome rigid body forces so that it assumes a hydrostatic equilibrium (nearly round) shape; and 3. Have cleared the neighborhood around its orbit.
Pluto, by virtue of the surrounding Kuiper belt objects, has not cleared its neighborhood, and so failed the new definition. A footnote in the IAU definition stated clearly that “the eight planets are: Mercury, Venus, Earth, Mars, Jupiter, Saturn, Uranus, and Neptune,” and the matter was considered settled.
Past the eight planets are the trans-Neptunian objects, or TNOs. Originally, these objects were split into two main groups: the Kuiper belt and the Oort cloud. Kuiper belt objects (KBOs) orbit within about 20 astronomical units (AU) of Neptune, while the Oort cloud lies upwards of a thousand AU away. Kuiper belt objects are difficult to observe since they are so far away, which is why most were not discovered until the 1990s. The Oort cloud, on the other hand, while thought to be a repository of comets, is too far away to observe and remains theoretical.
Planet Nine Recent observations have suggested that the matter of planets may not be as settled as astronomers believed in 2006. In 2016, astronomers Michael Brown—the same one who discovered Eris—and Konstantin Batygin published a paper laying out the evidence for a ninth planet orbiting far beyond Neptune. Unlike Pluto and the other Kuiper belt objects, this would be a true planet; its mass is currently estimated at five to ten times that of the Earth’s (Batygin et al., 2019, p. 36). Just as the existence of Neptune was originally inferred from its gravitational influence on Uranus, the evidence for Planet Nine comes from anomalous observations, this time of Kuiper belt objects, that would be explained by Planet Nine’s gravitational presence. In their initial paper, Batygin and Brown put forth three main arguments for Planet Nine: the presence “Pluto, by virtue of the surrounding Kuiper belt objects, has not cleared its neighborhood, and so failed the new definition."
Figure 1: Diagram showing the components of specifying an orbit, including the argument of perihelion (labeled as argument of periapsis, as perihelion refers specifically to objects that orbit the Sun) and the longitude of the ascending node. Source: Wikimedia Commons/ Lasunncty, CC-BY-SA 3.0
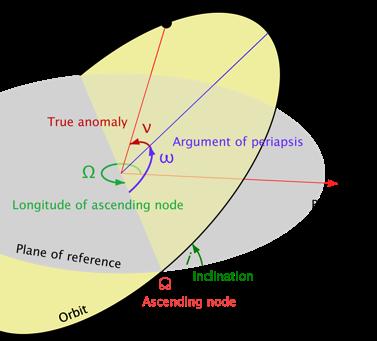
of remote objects like Sedna, the clustering observed in the perihelia of many TNOs, and the presence of TNOs that orbit at a high inclination compared to the plane of the eight known planets (Batygin & Brown, 2016, p. 1).
Sedna and 2012 VP113
One of those remote objects that does not fit in the Kuiper belt is the TNO Sedna, which has a highly eccentric orbit with a perihelion, or minimum distance from the Sun, of 76 AU. While some other TNOs do travel that far from the Sun, at the time, no others were known that always stayed at least that far away—most objects that travel that far were launched outward as a result of a close encounter with Neptune’s gravitational field, but Sedna never even gets close to Neptune. When Sedna was first observed in 2004, its discoverers—Brown and fellow astronomers Chadwick Trujillo and David Rabinowitz—suggested a few possibilities for this strange orbit. They found that a star passing by the solar system perpendicular to the plane of the Earth’s orbit around the Sun, known as the ecliptic, at a speed of ~30 km/s and a distance of ~500 AU could lift Sedna from a more normal orbit to its observed one. However, they suggested it would be more likely that the Sun formed as part of a stellar cluster and the other stars in the cluster pushed Sedna into its orbit before the solar system moved away from the cluster. Ironically, they also suggested that an unseen planet could be responsible for Sedna’s orbit, though they considered that very unlikely and the planet they suggested was much smaller and closer than Planet Nine (Brown et al., 2004, p. 648).
Then, in 2014, Trujillo and Scott Shepherd found another object like Sedna: 2012 VP113, whose perihelion is 80 AU. Interestingly, they did not find any Kuiper belt objects between 55 and 75 AU, despite the fact that such objects would be closer and therefore easier to detect. Based on that absence, Trujillo and Sheppard suggested that Sedna and 2012 VP113 may be better categorized as inner Oort Cloud objects (Trujillo & Shepherd, 2014, p. 471).
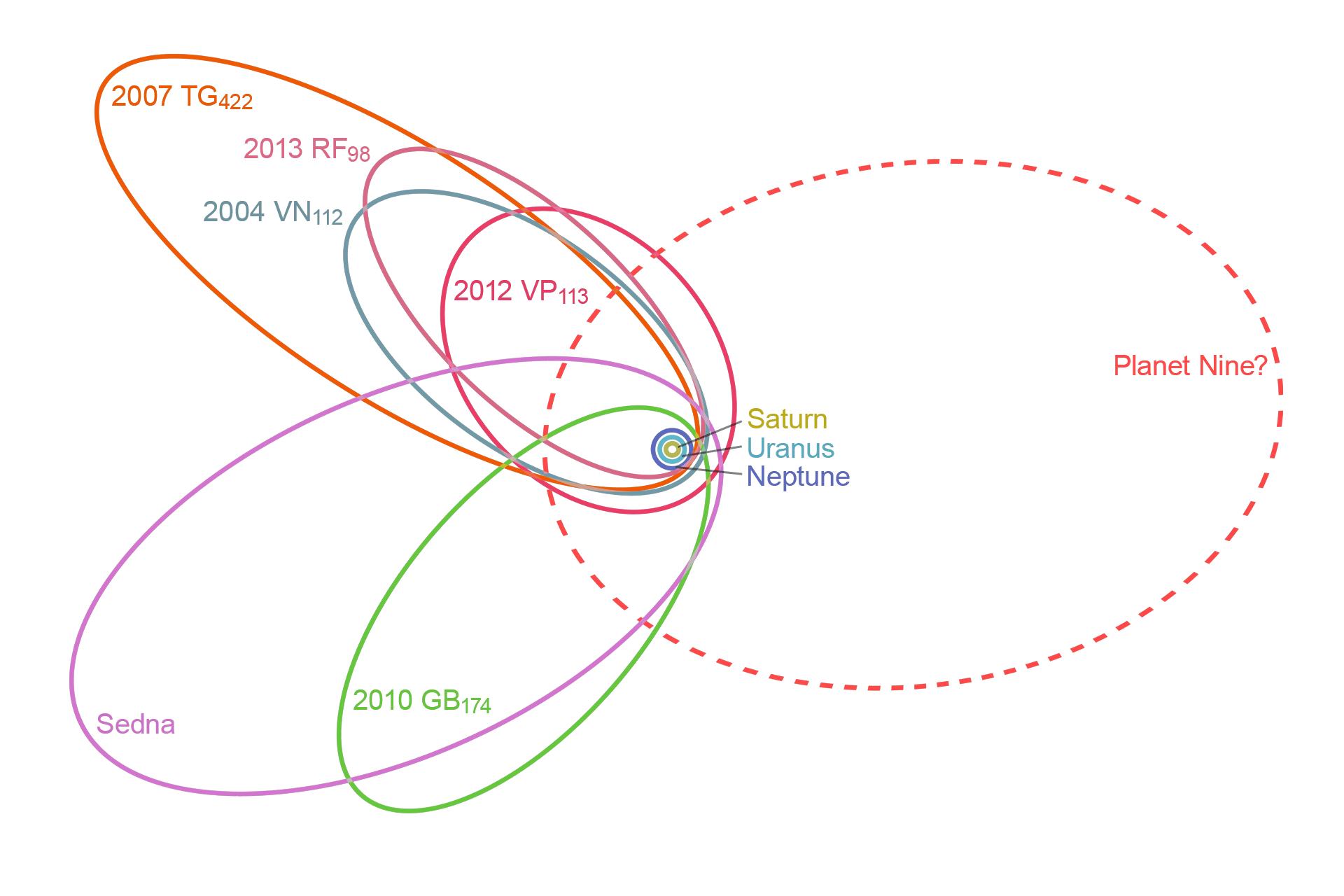
Figure 2: Diagram showing some of the anomalous TNO orbits (left) and a theoretical orbit for Planet Nine (right), with the orbits of Saturn, Uranus, and Neptune in the center for reference.
Source: Wikimedia Commons/ nagualdesign, CC-0
Perihelion Clustering
In their paper, Trujillo and Sheppard also noticed that Sedna and 2012 VP113 have something else in common: their arguments of perihelion are very similar. The argument of perihelion, represented by the variable ω, is the angle formed between a line from the object’s perihelion to the Sun and the ecliptic. The gravitational influence of the giant planets (Jupiter, Saturn, Uranus, and Neptune) is supposed to randomize these values over the lifespan of the solar system (Batygin & Brown, 2016, p. 1). Instead, Trujillo and Sheppard found that not only did Sedna and 2012 VP113 have similar arguments of perihelion but that every known Kuiper belt object with a semi-major axis greater than 150 AU and a perihelion distance greater than Neptune’s orbital distance (30 AU) has an argument of perihelion within 55° of about 340° (Trujillo & Shepherd, 2014, p. 472).
In their paper, Trujillo and Sheppard suggested a planet of about 5ME orbiting the Sun with a semi-major axis of about 250 AU could be responsible for this alignment (Trujillo & Shepherd, 2014, pp. 472–473). Batygin and Brown were intrigued by this possibility and set out to explore it in more detail. In their paper, they noted that Neptune’s gravity can affect objects with perihelion distances greater than its own, which would disturb any effects caused by Planet Nine’s gravity. After simulating the orbits of the Kuiper belt objects identified by Trujillo and Sheppard, they found that objects with perihelia less than 36 AU generally fall under Neptune’s influence. For the remaining objects, they found that the arguments of perihelion clustered around 318° ± 8°. Intriguingly, they also found that the longitudes of ascending nodes, the angle, Ω, in the ecliptic between a defined reference point and the intersection of the ecliptic and the plane of the object’s orbit, for these objects clustered around 113° ± 13°. Taken together, the clustering of these measurements indicates that the orbits of these objects are actually aligned in physical space and therefore are likely being influenced in the same way (Batygin & Brown, 2016, p. 2).
Highly-inclined TNOs
To explain this alignment, Batygin and Brown began running simulations of a ninth planet whose gravity would pull the orbits of the Kuiper belt objects into the proper shape. After some trial and error, they found a scenario that predicted both remote objects like Sedna and the perihelion clustering: a planet of about 10ME and a semi-major axis of about 700 AU, orbiting 180° away from the clustered perihelia (Batygin & Brown, 2016, p. 11). This “antialignment,” with the planet orbiting opposite the Kuiper belt objects, was initially puzzling, as such an orbit would generally be unstable and lead to collisions. Batygin and Brown, however, realized that such an orbit could be stable if Planet Nine had sufficient gravity to trap the Kuiper belt objects in mean-motion resonance. This resonance develops over time “After some trial and error, they found a scenario that predicted both remote objects like Sedna and the perihelion clustering: a planet of about 10ME and a semimajor axis of about 700 AU, orbiting 180 degrees away from teh clustered perihelia."
as objects orbit and exchange energy. If one object is sufficiently more massive than the other, this effect ultimately results in the smaller body making an integer number of orbits for every orbit the massive body completes. This effect is seen elsewhere in the solar system, for example, between Neptune and Pluto, where Pluto makes three orbits for every two that Neptune completes, which prevents them from colliding despite the fact that Pluto crosses Neptune’s orbit. Likewise, under this model, the aligned Kuiper belt objects would be in meanmotion resonance with Planet Nine, allowing the planet to orbit opposite the Kuiper belt objects and still be stable (Batygin & Brown, 2016, p. 6; Batygin & Morbidelli, 2017). However, because of Planet Nine’s high eccentricity, many of these resonances are more complex ratios (e.g. 2:7), and therefore are not helpful for narrowing down Planet Nine’s location in the sky (Bailey et al., 2018).
Interestingly, this model made another prediction: that Planet Nine’s gravity would push some trans-Neptunian objects farther away and to a higher inclination, to the point where they would disappear from view, only to then bring them back into view in a highly inclined orbit, possibly even one perpendicular to the celestial plane. While Batygin and Brown were initially puzzled by that prediction, they soon realized there is such a population of trans-Neptunian objects: at the time, astronomers had already found several TNOs with highly inclined orbits, some of which are in fact roughly perpendicular to the celestial plane. The presence of these objects has never been explained, but the fact that the Planet Nine model offers an explanation for a phenomenon it was not trying to explain lends a measure of additional credence to its other predictions (Batygin & Brown, 2016, p. 10).
Further Developments
In their initial paper, Batygin and Brown noted that discovering additional Kuiper belt objects would be crucial to eliminating any sampling bias and refining the orbital parameters of Planet Nine. Since then, additional objects with orbits that fit the predictions of the Planet Nine hypothesis, such as 2015 TG387 and 2015 BP519, have been found (Sheppard et al., 2019, p. 10; Becker et al., 2018, pp. 10–11). One study found that Planet Nine’s gravity could explain the solar obliquity, an unexplained misalignment between the Sun's axis of rotation and the celestial plane (Bailey et al., 2016), though later revisions to estimates of Planet Nine’s mass and orbit concluded that it cannot account for all of the solar obliquity (Batygin et al., 2019, p. 38). Some have suggested that the observed clustering of Kuiper belt objects may just be the result of observational biases (Shankman et al., 2017), but both subsequent observations and a detailed statistical analysis suggest that is not the case (Brown & Batygin, 2019). Since the clustering appears real, most alternate theories suggest that it is caused by a celestial body or bodies other than a planet, such as a ring of icy objects with an orbit and total mass similar to Planet Nine or a black hole with a mass similar to Planet Nine that was captured by the Sun’s gravity (Sefilian & Touma, 2019; Scholtz & Unwin, 2019). Nevertheless, Planet Nine remains the simplest and most likely explanation for the anomalous orbits observed in TNOs, though attempts to locate it with a telescope have yet to succeed. Depending on how far away Planet Nine is and where it is in its orbit, it may be just at the limit of the observational abilities of powerful telescopes like the Subaru Telescope. If it is too far away for current telescopes to see, the Vera Rubin Observatory in Chile, which is projected to begin observations in late 2022 (LSST, n.d.), should be able to find it.
In 2019, Batygin and Brown published a thorough analysis of the evidence for Planet Nine thus far. Whereas the original paper suggested Planet Nine would be upwards of 10ME and have a semi-major axis of roughly 700 AU, the most recent data indicates it should be both smaller (mass between five and ten ME) and closer (semi-major axis between 500 and 800 AU); a higher mass indicates a greater distance. Despite the decrease in mass, a 5ME Planet Nine would actually be easier to detect than the original 10ME proposal because it would be closer to Earth (Batygin et al., 2019, p. 39). However, while the decrease in mass refines the Planet Nine hypothesis to better fit its core prediction—the alignment of objects in the Kuiper belt—it also means that Planet Nine cannot explain the entirety of the solar obliquity (Batygin et al., 2019, p. 38).
To prove or disprove the existence of Planet Nine, scientists need predictions that can be tested and observed; one possible method for generating these predictions can be borrowed from the techniques of exoplanet hunters. One of the primary ways astronomers search
Figure 3: Plot of Neptune's TTV over time in the 5ME (top) and 10ME (bottom) scenarios.
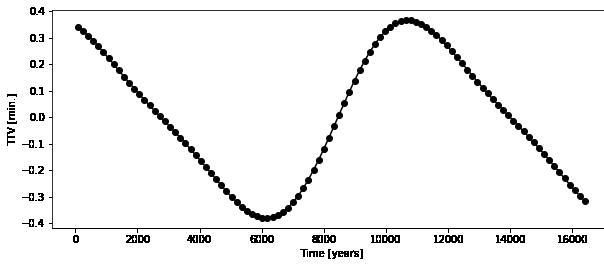
Source: Created by the writer using the TTV2Fast2Furious code, CC-BY-SA 4.0
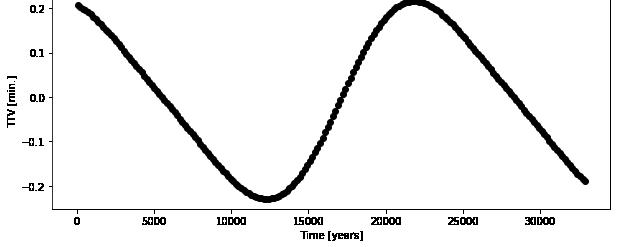
for exoplanets is by looking for stars that periodically dim slightly, which can indicate that a planet is moving between the star and the Earth. This method is very good at finding large planets that orbit close to their star (since such planets will block more light) and is dependent on the planet’s period—if the planet completes an orbit every 10 days, for example, astronomers should see the star dim every 10 days, like clockwork. If the timing of these transits instead rises and falls over time, that suggests that there may be another planet that is gravitationally tugging on the first.
While stars are gravitationally dominant in their planetary system and generally keep their planets orbiting at a constant rate, they are not the only gravitationally noteworthy bodies. The planets themselves tug on each other, tweaking their orbits slightly. As a result, each time a planet goes around its host star, it takes a slightly different amount of time. The difference in these times is known as transit-timing variation (TTV). These variations increase and decrease over time, like a wave. As a result, astronomers generally talk about the amplitude of a TTV (the maximum amount, positive or negative, by which one planet changes the period of another planet) and the period of the TTV cycle (how long it takes a planet’s TTV to come back to the same value and slope, not to be confused with the period of the planet’s orbit).
TTV observations don’t always establish an exact orbit for the second planet, but they can help prove its existence and narrow down the range of possible orbits. Since TTV effects are usually small (on the order of minutes), they are easier to notice in planets with short orbital periods—which, as planets orbit more quickly the closer they are to their star, are exactly the kind of planets that are easy to detect by the dimming of a star. Nevertheless, the general principle holds and can also be applied to more remote planets like Neptune and Planet Nine. Calculating these orbital effects is complex and, in this paper, was accomplished with the “While stars are gravitationally dominant in their planetary system and generally keep their planets orbiting at a constant rate, they are not the only gravitationally noteworthy bodies.”
TTV2Fast2Furious code (available at https:// github.com/shadden/TTV2Fast2Furious). This code takes in information about the orbital characteristics of at least two planets and runs that information through a matrix equation, before outputting a graph of both planets’ TTV over time. The code was originally developed for calculations with exoplanets—specifically the ones that orbit close to their host star that are easier to detect. While the program is capable of integrating the data over a sufficient number of transits to show a complete TTV cycle for Neptune, even on its innermost orbit, Planet Nine completes only about one orbit over that timespan (giving it, at most, two transits). Increasing the timespan to include enough Planet Nine transits returned an error in the code as the integral exceeded its maximum number of subdivisions. Since the purpose of this calculation is to explore the feasibility of detecting a TTV in Neptune’s orbit assuming that Planet Nine exists, the TTV of Planet Nine is irrelevant anyways and it was removed from the final graphs to avoid any confusion. Because of the extremely long orbital periods compared to those of the planets the code was designed for, the code was also adjusted to display time in years instead of days on the graphs.
Calculation Details
In order to calculate a planet’s TTV, the TTV2Fast2Furious code needs to know the mass, period, eccentricity, and initial transit time of both the “target” planet and the planet perturbing that target planet’s orbit. This section details the steps taken to supply those parameters and calculate Neptune’s TTV as a result of Planet Nine’s gravitational influence for the minimum and maximum predicted mass of Planet Nine (5ME and 10ME respectively). While the mass of Planet Nine could also lie somewhere between these values, by considering both extremes, these calculations find the maximum and minimum TTV values, essentially establishing the best and worst case scenarios for detecting Planet Nine’s influence on Neptune. Data for Neptune was taken from Williams (September 2018) and the full orbital parameters for both the 5ME and 10ME scenarios for Planet Nine were taken from Batygin et al. (2019). A complete list of adopted values is in Appendix A.
The given mass values for Neptune and Planet Nine were converted to solar masses (the units expected by the code) using the values of MS and ME from NASA’s Sun and Earth data (Williams, February 2018, 2020). Given the period of Neptune’s orbit in sidereal days, the period of Planet Nine, P9, can then be defined in terms of its semi-major axis (a9) and Neptune’s semimajor axis (aN) and period (PN), with Kepler’s Third Law, which yields:
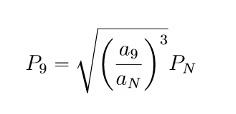
The eccentricities of both Neptune and Planet Nine are given in the sources and required no further conversion. Since the initial transit time for the planets would depend on the location of a hypothetical alien observer, the code was tested with different values to see how much they would impact the results. This testing found that the value assigned to the initial transit time had only a small effect on Neptune’s initial TTV value and no noticeable effect on its TTV amplitude or period. In the final calculations, Neptune was assigned an initial transit time of half its period (indicating that it transits at aphelion, where the transit probability is higher) and Planet Nine an initial transit time of a quarter of its period. For the 10ME scenario, the code was also adjusted to calculate results out to 200 transits of Neptune, which, because Planet Nine is farther away and therefore has less of a gravitational impact on Neptune, was necessary to produce a full TTV cycle.
Results
Running the code resulted in a TTV amplitude of 0.4 minutes and period of 12,300 years in the 5ME scenario and a TTV amplitude of 0.2 minutes and a period of 22,000 years in the 10ME scenario. For comparison, the first significant planet discovered by TTV, Kepler-19c, gives the transiting planet Kepler-19b a TTV amplitude of 5 minutes with a period of 316 days (Ballard et al., 2011, p. 15). Since its discovery, Neptune has completed just a little bit more than one orbit. As such, even in the best-case scenario of a 5ME Planet Nine, finding Planet Nine by looking at variations in Neptune’s orbit does not seem feasible.
Conclusion Indirect evidence for the existence of Planet Nine has grown over time, but astronomers have yet to actually observe it. The principal challenge is that astronomers have only had a few decades to observe the TNOs it influences, and they have periods of hundreds of years. Astronomically speaking, they have barely
moved since they were discovered. In contrast, when the only other planet to be discovered by calculation—Neptune—was found, astronomers had already known about Uranus for 65 years and had been able to observe most of its orbit. Indeed, seeing the perturbations over time in Uranus’ orbit is what enabled Le Verrier to predict Neptune’s orbit. While the goal that motivated this paper was to help narrow down the range of possible orbits for Planet Nine by calculate the TTVs expected for Neptune as a result of Planet Nine’s gravitational influence, the calculation ultimately found that they are likely too small and occur over too long a timescale to be of much use in finding Planet Nine. Nevertheless, the search for Planet Nine continues. If it is not found within the next few years, the Vera Rubin Observatory, which is scheduled to begin observations in 2022, should have the observational capacity to detect Planet Nine and will be conducting observations as part of a survey of the whole sky every night that it can, which should finally resolve the search for Planet Nine.
Acknowledgments
Appendix A: Adopted Values
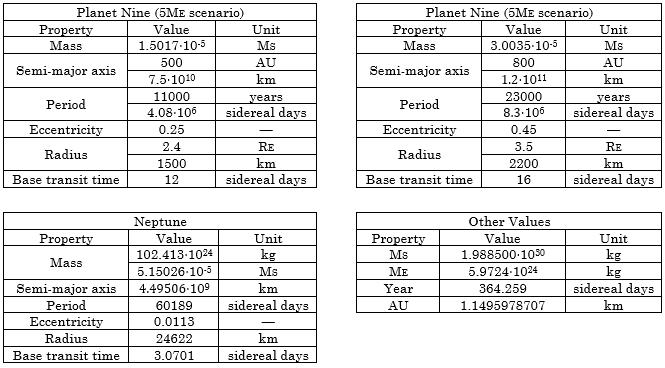
This paper would not exist without Dartmouth College Professor Elisabeth Newton, who taught me quite a bit about astronomy, guided me from a vague notion of writing something about Planet Nine to the idea of doing a TTV analysis, helped me understand the TTV2Fast2Furious code, and provided feedback on the paper. This paper also wouldn’t read anywhere near as well as it does without the efforts of the editors who worked on it; thank you to Nathalie Korhonen Cuestas in my Astronomy 15 class and the DUJS editors Sam Neff, Madeline Brown, and Eric Youth for all of their suggestions and comments. And finally, I am enormously grateful to a few people whose influence helped me personally write this paper: Professor Mike Brown of the California Institute of Technology, whose work inspired my interest in Planet Nine, and the friends who kept me sane through the writing process— they know who they are. “If it is not found within the next few years, the Vera Rubin Observatory, which is scheduled to begin observations in 2022, should have the observational capacity to detect Planet Nine...”
Bailey, E., Batygin, K., & Brown, M. E. (2016). Solar obliquity induced by Planet Nine. The Astronomical Journal, 152(5). https://doi.org/10.3847/0004-6256/152/5/126 Bailey, E., Brown, M. E., Batygin, K. (2018). Feasibility of a resonance-based Planet Nine search. The Astronomical Journal, 156(2). https://doi.org/10.3847/1538-3881/aaccf4
Ballard, S., Fabrycky, D., Fressin, F., Charbonneau, D., Desert, J., Torres, G., Marcy, G., Burke, C. J., Isaacson, H., Henze, C., Steffen, J. H., Ciardi, D. R., Howell, S. B., Cochran, W. D., Endl, M., Bryson, S. T., Rowe, J. F., Holman, M. J., Liassauer, J. J., … Borucki, W. J. (2011). The Kepler-19 System: A transiting 2.2 RE planet and a second planet detected via transit timing variations. The Astrophysical Journal, 743(2). https://doi.org/10.1088/0004637X/743/2/200
Batygin, K., Adams, F. C., Brown, M. E., & Becker, J. C. (2019). The Planet Nine hypothesis. Physics Reports, 805, 1-53. https://doi. org/10.1016/j.physrep.2019.01.009
Batygin, K. & Brown, M. E. (2016). Evidence for a distant giant planet in the solar system. The Astronomical Journal, 151(2). https://doi.org/10.3847/0004-6256/151/2/22
Batygin, K. & Morbidelli, A. (2017). Dynamical evolution induced by Planet Nine. The Astronomical Journal, 154(6). https://doi.org/10.3847/1538-3881/aa937c
Becker, J. C., Khain, T., Hamilton, S. J., Adams, F. C., Gerdes, D. W., Zullo, L., Franson, K., Millholland, S., Bernstein, G. M., Sako, M., Bernardinelli, P., Napier, K., Markwardt, L., Lin, H. W., Wester, W., Abdalla, F. B., Allam, S., Annis, J., Avila, S., … Walker, A. R. (2018). Discovery and dynamical analysis of an extreme trans-Neptunian object with a high orbital inclination. The Astronomical Journal, 156(2). https://doi.org/10.3847/15383881/aad042
Brown, M. E. & Batygin, K. (2019). Orbital clustering in the distant solar system. The Astronomical Journal, 157(2). https:// doi.org/10.3847/1538-3881/aaf051
Brown, M. E., Trujillo, C., & Rabinowitz, D. (2004). Discovery of a candidate inner Oort cloud planetoid. The Astrophysical Journal, 617(1), 645-649. https://doi.org/10.1086/422095
Brown, M. E. (2010). How I killed Pluto and why it had it coming. Spiegel & Grau.
IAU. (2006, August 24). IAU 2006 General Assembly: Result of the IAU Resolution votes. IAU. Retrieved August 26, 2020, from https://www.iau.org/news/pressreleases/detail/iau0603/ Krajnović, D. (2016). The contrivance of Neptune. Astronomy and Geophysics, 57(5), 5.28-5.34. https://doi.org/10.1093/ astrogeo/atw183
LSST. (n.d.). Fact sheets. Rubin Observatory. Retrieved August 26, 2020, from https://www.lsst.org/about/fact-sheets Williams, D. R. (2018, February 23). Sun Fact Sheet. NASA. Retrieved September 24, 2020, from https://nssdc.gsfc.nasa. gov/planetary/factsheet/sunfact.html
Williams, D. R. (2018, September 27). Neptune Fact Sheet. NASA. Retrieved September 24, 2020, from https://nssdc.gsfc. nasa.gov/planetary/factsheet/neptunefact.html
Williams, D. R. (2020, April 02). Earth Fact Sheet. NASA. Retrieved September 24, 2020, from https://nssdc.gsfc.nasa. gov/planetary/factsheet/earthfact.html Scholtz, J. & Unwin, J. (2019). What if Planet 9 is a primordial Sefilian, A. A. & Touma, J. R. (2019). Shepherding in a selfgravitating disk of trans-Neptunian objects. The Astronomical Journal, 157(2). https://doi.org/10.3847/1538-3881/aaf0fc
Shankman, C., Kavelaars, J. J., Bannister, M. T., Gladman, B. J., Lawler, S. M., Chen, Y., Jakubik, M., Kaib, N., Alexandersen, M., Gwyn, S. D. J., Petit, J., & Volk, K. (2017). OSSOS. VI. Striking biases in the detection of large semimajor axis transNeptunian objects. The Astronomical Journal, 154(2). https:// doi.org/10.3847/1538-3881/aa7aed
Sheppard, S. S., Trujillo, C. A., Tholen, D. J., & Kaib, N. (2019). A new high perihelion trans-Plutonian inner Oort cloud object: 2015 TG387. The Astronomical Journal, 157(4). https://doi. org/10.3847/1538-3881/ab0895
Trujillo, C. A. & Sheppard, S. S. (2014). A Sedna-like body with a perihelion of 80 astronomical units. Nature, 507, 471-474. https://doi.org/10.1038/nature13156
Racial Bias Against Black Americans in the American Healthcare System
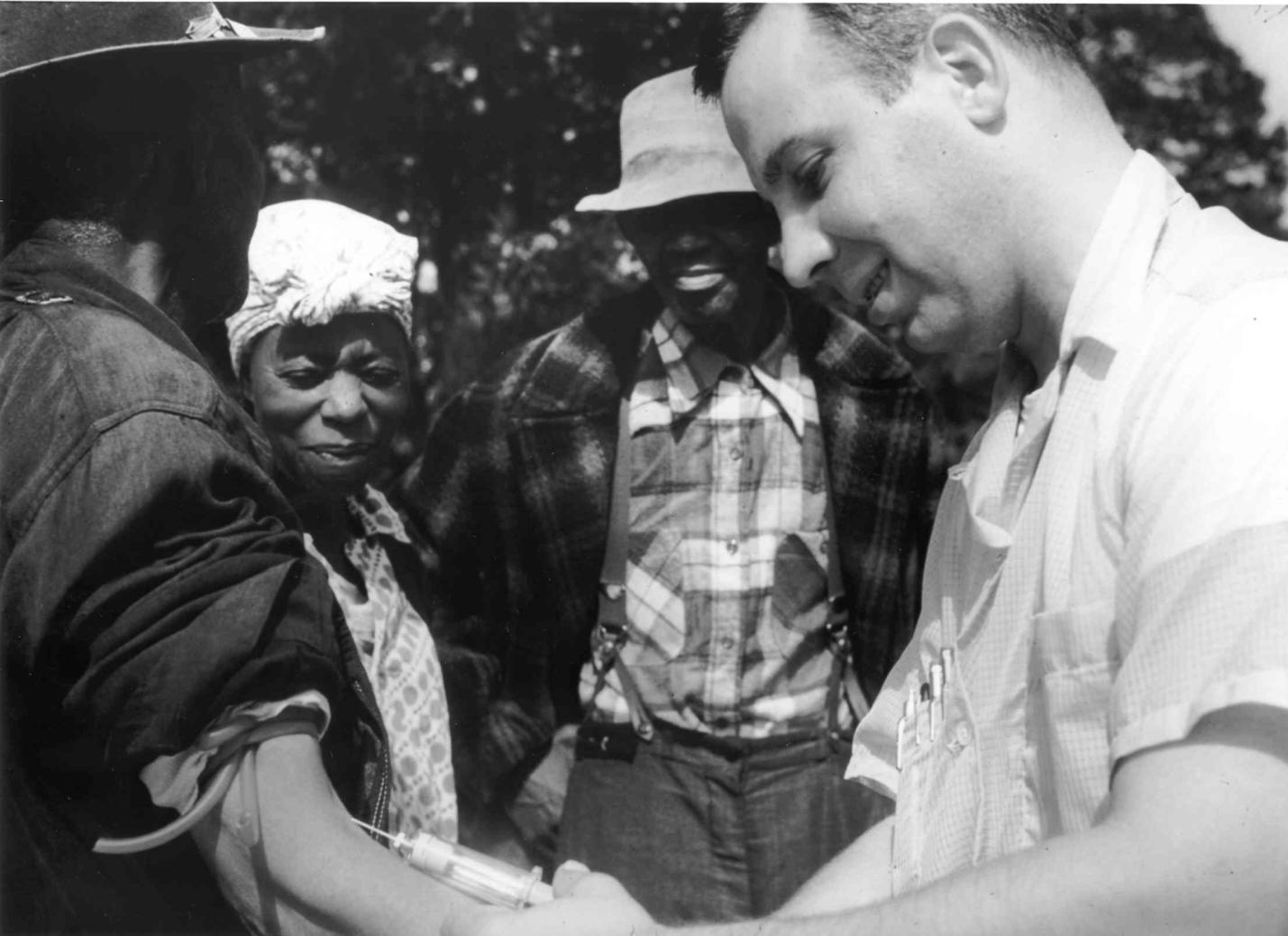
BY ANAHITA KODALI '23
Cover Image: Pictured above is a doctor drawing blood as part of the Tuskegee Syphilis study. The men who were in the study were promised free healthcare as incentive for their participation, but the doctors used a litany of placebos and diagnostic procedures (including blood tests) in place of actual medical procedures that could treat syphilis. The lasting impacts of the study on Black patient trust in the healthcare systems are still felt today. Source: Wikimedia Commons Introduction There are deep historic inequities among America’s different demographic groups. Specifically, Black Americans are at a significant disadvantage in many areas, from wage gaps to discrimination in hiring practices to lack of access to education. The current global pandemic has highlighted yet another area that Black Americans are underserved: the healthcare industry. Black Americans have historically, on average, had poorer health than their White counterparts. Many argue that the disparity in health outcomes was due to genetic differences, cultural practices, or socioeconomic issues. However, even when all factors are held constant, Black individuals consistently have more indicators of poor health than White individuals (Charatz-Litt, 1992). Understanding why necessitates a deeper look at the issues that Black Americans face in medicine. This paper reviews the racist past of American medicine as well as modern racism in physician attitudes to Black people and medical technologies.
It is important to note that for the purposes of simplicity and clarity, much of this paper discusses the differences between the treatment of Black and White Americans as a dichotomy. However, in reality, racism in American healthcare effects other people of color as well. Current rising racial tensions and protests across the United States highlight the struggles of Black Americans with predominantly White American institutions, which is why this paper chooses to focus on their experience with the healthcare system.
Medicine's Ugly History Slavery was the first major barrier to Black Americans receiving proper healthcare. The American Medical Association (AMA) was formed in 1847 as a result of years of
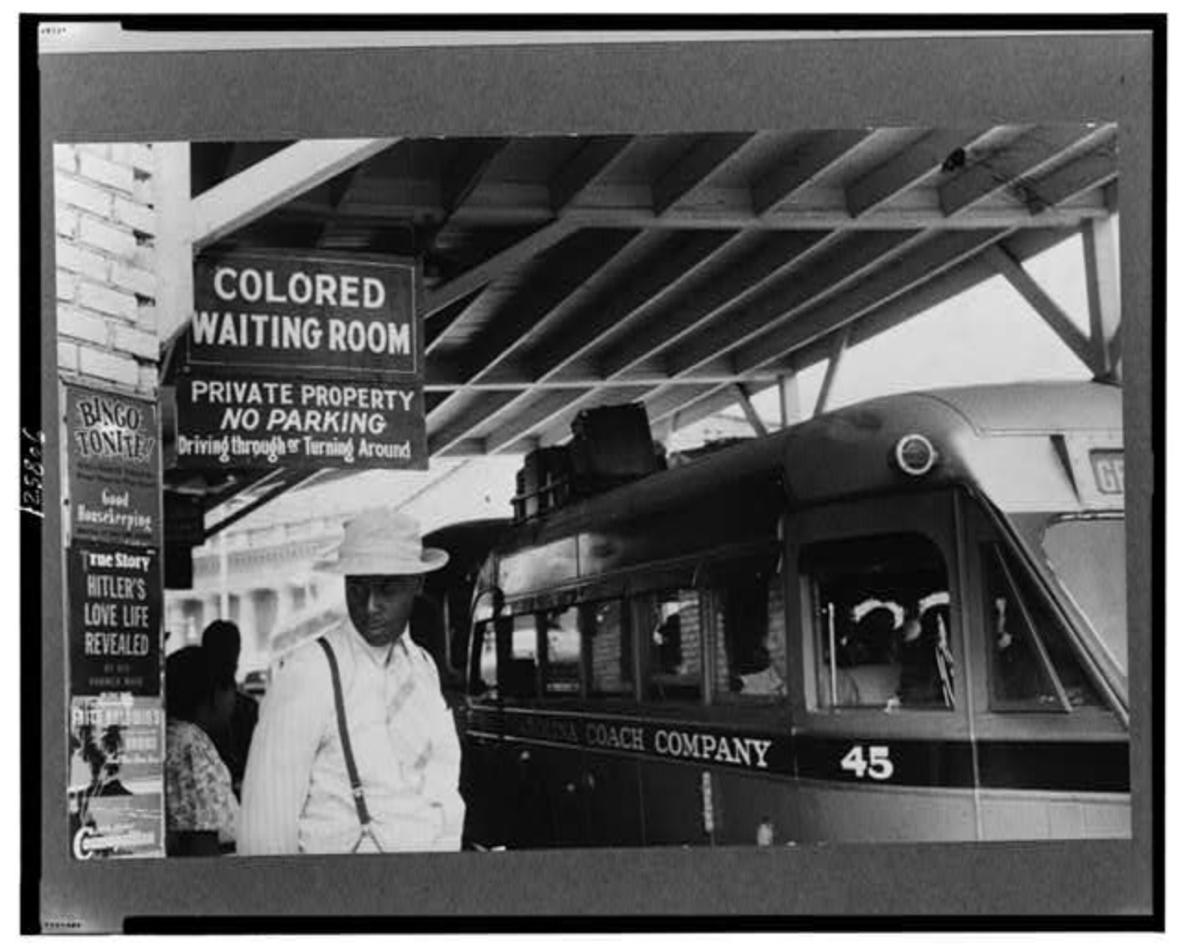
Figure 1: Generally, people only consider the obvious effects of the segregation laws in the Jim Crow codes. However, one critical piece often ignored – Black people were often denied access to the best hospitals in the country, forcing them to instead either go to subpar healthcare facilities or treat illnesses themselves at home. This resulted in overall poorer health for America’s Black demographic relative to America’s Whites.
Source: Wikimedia Commons
conversations between White physicians wanting to protect their practice from homeopathic, or alternative, medicine. They established standards of science and professionalism while securing for themselves the elite status of physicians in society, resulting in a boom of White physician practice. Unfortunately, these standards did not apply to slaves (“AMA History, ” 2018).
Slave masters actually often tried to give slaves professional medical care when they fell ill or got injured; though perhaps counterintuitive at first, this proved to be more economically viable than having to pay for more slaves if one became too ill to work (Lander & Pritchett, 2009). However, slave masters had to contend with several issues. For one, physicians were less available in the South than they were in the North, meaning that slave masters often could not find a doctor close enough to treat their slaves quickly. Secondly, even though it made economic sense to treat slaves for illness, services were often too expensive for individual owners to give to slaves. Thirdly, even when slave masters had access to doctors and could afford to pay them, it was difficult to find a doctor willing to work on Black patients; those that did, often did shoddy work as the standards set by the AMA did not apply to slaves. Finally, even doctors who attempted to do adequate work on slaves would give slaves different treatments than they would a White patient because they did not understand how Black people presented different illnesses (Charatz-Litt, 1992). Several doctors believed that Black people had less sensitivity to pain than White people, and some doctors still hold the belief today (CharatzLitt, 1992). Others believed that Black people’s brains were smaller than brains of other races, while their senses of smell, taste, and hearing were stronger. Additionally, physicians applied certain theories and disease models specifically to slaves, including “Drapetomania,” a theory that slaves ran away because of mental illness, and “Cachexia Africana,” a pathology in which Black people would eat dirt; this further worsened the level of care given to slaves (Sullivan, n.d.).
This lack of understanding of Black anatomy (as well as a general lack of knowledge about human biology) led to the horrific practice of experimentation on slaves. As medicine was quickly advancing, demands for human specimens for physician training and research increased. Slaves represented a very unique and attractive group for researchers; from a scientific perspective, they could be used as good research models, and from a legal perspective, they had no autonomy. Thus, slaves were often used as practice for surgeons, ways to study autopsy, and experimental models for new techniques (Savitt, 1982). Slaves had to endure amputations, electric shocks, tumor removals, and more terrible experiments, often without using anesthesia (Kenny).
The improper treatment of Black Americans by doctors persisted even after the emancipation of slaves. Though constitutionally equal to White Americans, Black people were “This lack of understanding of Black anatomy (as well as a general lack of knowledge about human biology) led to the horrific practice of experimentation on slaves.”
Figure 2: Maternal mortality rate (per 100,000 births) across the United States.
Source: Wikimedia Commons (published in 2015).
Figure 2: Having a more diverse medical field allows doctors and medical researchers to understand better how diseases (like Lupus) present themselves differently in different demographics of patients. This allows doctors to better and more precisely serve all of their patients. Source: Wikimedia Commons
systemically denied access to proper healthcare due to the implementation of segregation and the Jim Crow Laws (see Figure 1). As a result, Black people were unable to receive necessary medical treatments or go to the best medical practices in the nation (Hunkele, n.d.). At the same time, Black people were exploited by White doctors to advance medicine. One of the most well-known examples is the Tuskegee Syphilis Experiment (see Cover Figure). The US Public Health Service conducted the Tuskegee Syphilis Experiment from 1930 into the 1952 in order to see the effects of untreated syphilis on 600 Black men; even when penicillin treatments (which cured syphilis) became widely available in the 1950s, doctors did not give the men adequate care. By the end, at least 100 Black men had died from syphilis related complications (Brandt, 1978). The tale of Henrietta Lacks, a poor Black southern woman who got treated for cervical cancer at Johns Hopkins University in the 1950s, is another clear case of the American healthcare system failing its Black patients. Doctors took samples of the cells in Mrs. Lacks’s tumor cells without informed consent, which ultimately led to an immortal cell line that has opened the pathway for innovation in countless scientific and medical pursuits, including research into cancer, AIDS, radiation therapy, and genomic mapping. Mrs. Lacks’s family was not immediately informed and never received compensation for her contributions (Khan, 2011).
While, these two stories highlight the historic improper care of Black Americans and caused Black Americans to lose trust in the healthcare system, there are many more specific reasons for this lack of trust. Unfortunately, as medicine improves and the inequities in healthcare for Black people persists, the level of disparity between White and Black Americans has stayed stagnant (National Center for Health Statistics, 2006). Though there are more legal protections in place for Black Americans today than there were decades ago, there are still significant barriers to Black people receiving adequate care.
Physician Attitudes Towards Black Patients There still exists a significant degree of bias in the American healthcare system that causes physicians to treat Black patients unfairly. These issues begin in physician training and education. Despite receiving a more comprehensive education than their counterparts in the 1800s did, a significant number of physicians share a fundamental and dangerous misunderstanding
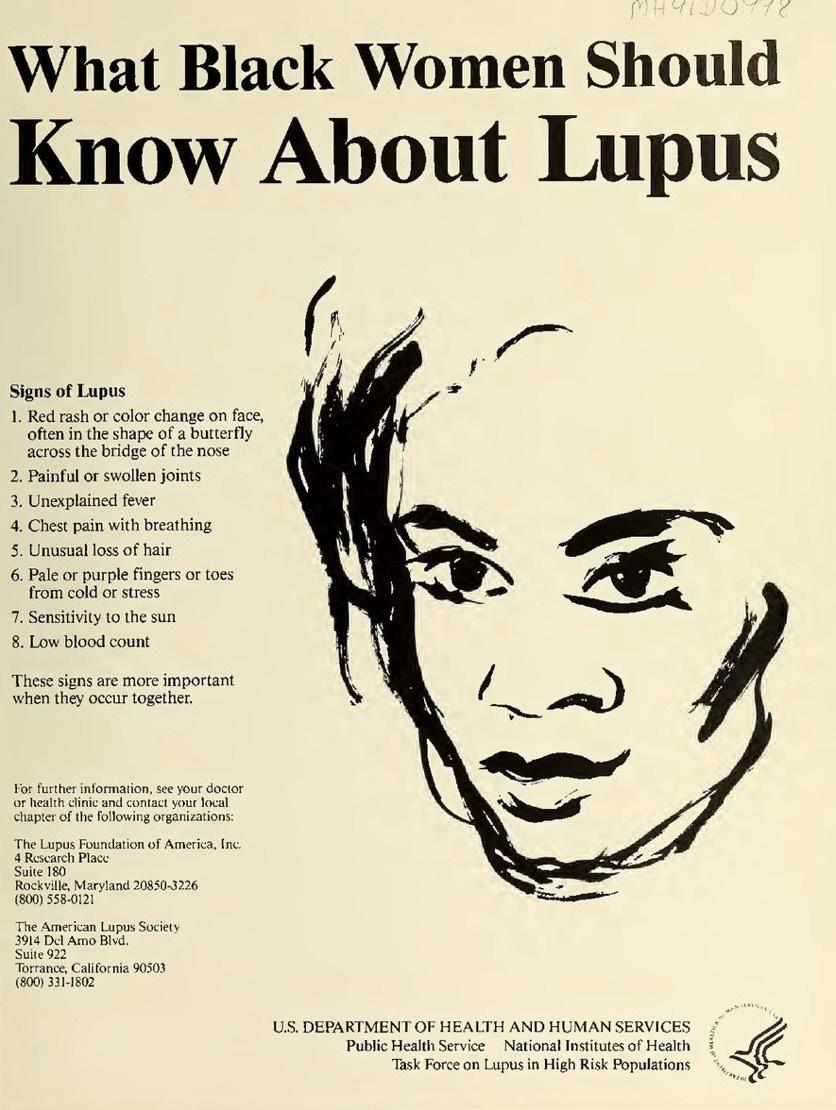
of pain and pain management for Black patients (see Figure 2). Half of White medical students and residents surveyed in 2016 held one or more incorrect beliefs of Black biology and pain tolerance, including: Black people have thicker skin than White people; Black people’s nerves are less sensitive than White people’s nerves; and Black people’s blood coagulates more quickly than White people’s. These beliefs have all been proven to be wrong (Hoffman et. al., 2016). Issues in training and misunderstanding of Black anatomy and biology translate directly to problems in the hospital. Generally, physicians are much more unlikely to believe their Black patient’s descriptions of pain and therefore have skewed perceptions of the pain of their patient (Staton et. al., 2007). This perception has resulted in White patients consistently receive more pain medications than Black patients. Researchers found that 74% of White patients received pain medication for injuries related to bone fractures while only 50% of Black patients received pain medications for similar injuries (Josefson, 2000). The disparity can be specifically found in adolescent patients. For example, White children are much more likely than their Black counterparts to receive pain treatment for appendicitis (Goyal et. al., 2015).
Issues with pain management have led to significant trust issues between patients and physicians. Researchers have found that there are significant differences in physician-patient
trust that are related to racial differences. Interactions between Black patients and nonBlack physicians are relatively unfavorable when compared to interactions between Black patients and Black physicians. (Martin et. al., 2013). Black patients tend to trust Black doctors more than White doctors; similarly, when Black men receive care from Black physicians, they are significantly more likely to receive effective care. Specifically, the Black men are more likely to receive preventative care with these findings most pronounced for men who had strong distrust in the medical system (Alsan et. al., n.d.). Thus, emphasis on creating more diversity in the medical field could help alleviate some of the issues caused by biased physician training.
Bias in Medical Technologies Researchers and entrepreneurs alike have increasingly voiced their concerns about racial bias in technology over the past few years. Biotechnology in medicine is no exception. As the medical field becomes more technology driven, the existing issues with algorithms and biotechnology will continue to grow, which could aggravate the already dangerous racial disparities in medicine.
Healthcare centers around the country use commercial algorithms to guide help guide clinical decisions and decide which patients receive additional care. These algorithms affect the healthcare that millions of Americans receive. Researchers studied one algorithm that is representative of the majority of popular algorithms employed by hospitals and found that Black patients were significantly sicker than their White counterparts for any given risk score (risk scores are the way that doctors decide what course of treatment to give to their patients). Unwell Black patients were given the same risk score as healthy White patients, resulting in a reduction of Black patients that were identified for extra care by more than half. This bias results from the algorithms predicting costs of health instead of health needs directly. Costs and healthcare needs are directly correlated (sicker patients require more care and therefore more money), so at first glance, using cost as a proxy for needs makes sense. However, historically, the healthcare system spends significantly less money on Black patients because of several issues, including direct discrimination and challenges to the patient-physician relationship. Because less money is spent on Black patients, the algorithms conclude that Black patients are healthier than White patients. By solving the issue, the team estimated that the number of Black patients receiving extra care would jump from 17.7% to 46.5% (Obermeyer et. al., 2019).
The advent of precision medicine also represents a major problem for Black patients. While precision medicine has the potential to greatly improve care by allowing for hyperindividualized care, unchecked, it will also propagate a host of biases towards Black patients. There are three main areas that precision medicine can derive this bias. The first is collection of biased data; Black people are historically underrepresented in research datasets; this underrepresentation results in wider variability and less accurate results, as well as a lack of understanding of the nuances in Black patient presentation of certain diseases. This results in biased conclusions being drawn. The second is integration of biased data into precision medicine algorithms; as previously discussed, datasets are already biased. These biases are then reinforced when biased AI technologies are used to create clinical algorithms. The third is influence of preexisting structural racism while precision medicine is being adopted by hospitals; structural racism affects which hospitals adopt precision medicine and which patients will receive access to it and will ultimately hurt Black patients, who are relatively underprioritized when compared to White patients because of both algorithm bias and direct discrimination (Geneviève et. al., 2020).
Conclusions and Future Directions It is abundantly clear that Black people are not cared for properly in the current American healthcare system relative to White counterparts. From historic inequities to issues with modern physician attitudes and growing concerns about the prevalence of biased technology, there are a myriad of problems that need to be solved to ensure Black people receive equal, accessible, and adequate healthcare. By bringing more diversity to medicine, the American healthcare system could see immediate benefits with physicianpatient trust. However, in the long term, significant steps need to be taken to dismantle the systemic issues that exist that are the root causes of unequal access to medicine. “As the medical field becomes more technologyu driven, the existing issues with algorithms and biotechnology will continue to grow, which could aggravate the already dangerous racial disparities in medicine.”
Alsan, M., Garrick, O., & Graziani, G. C. (n.d.). Does Diversity Matter for Health? Experimental Evidence from Oakland. 56.
AMA History. (2018, November 20). Retrieved July 22, 2020 from https://www.ama-assn.org/about/ama-history/amahistory
Brandt, A. M. (1978). Racism and Research: The Case of the Tuskegee Syphilis Study. The Hastings Center Report, 8(6), 21. https://doi.org/10.2307/3561468
Charatz-Litt, C. (1992). A chronicle of racism: The effects of the White medical community on Black health. Journal of the National Medical Association, 84(8), 717–725.
Geneviève, L. D., Martani, A., Shaw, D., Elger, B. S., & Wangmo, T. (2020). Structural racism in precision medicine: Leaving no one behind. BMC Medical Ethics, 21(1), 17. https://doi.org/10.1186/s12910-020-0457-8
Goyal, M. K., Kuppermann, N., Cleary, S. D., Teach, S. J., & Chamberlain, J. M. (2015). Racial Disparities in Pain Management of Children With Appendicitis in Emergency Departments. JAMA Pediatrics, 169(11), 996. https://doi. org/10.1001/jamapediatrics.2015.1915
Hoffman, K. M., Trawalter, S., Axt, J. R., & Oliver, M. N. (2016). Racial bias in pain assessment and treatment recommendations, and false beliefs about biological differences between Blacks and Whites. Proceedings of the National Academy of Sciences, 113(16), 4296–4301. https://doi. org/10.1073/pnas.1516047113
Hunkele, K. L. (n.d.). Segregation in United States Healthcare: From Reconstruction to Deluxe Jim Crow. 51.
Josefson, null. (2000). Pain relief in US emergency rooms is related to patients’ race. BMJ (Clinical Research Ed.), 320(7228), 139A.
Kenny, S. C. (2015). Power, opportunism, racism: Human experiments under American slavery. Endeavour, 39(1), 10–20. https://doi.org/10.1016/j.endeavour.2015.02.002
Khan, F. A. (2011). The Immortal Life of Henrietta Lacks. Journal of the Islamic Medical Association of North America, 43(2). https://doi.org/10.5915/43-2-8609
Lander, K., & Pritchett, J. (2009). When to Care: The Economic Rationale of Slavery Health Care Provision. Social Science History, 33(2), 155–182. https://doi.org/10.1215/014555322008-018
Martin, K. D., Roter, D. L., Beach, M. C., Carson, K. A., & Cooper, L. A. (2013). Physician communication behaviors and trust among Black and White patients with hypertension. Medical Care, 51(2), 151–157. https://doi.org/10.1097/ MLR.0b013e31827632a2
National Center for Health Statistics. Health United States 2006 with chartbook on trends in the health of Americans. US Government Printing Office, Hyattsville, MD (2006)
Obermeyer, Z., Powers, B., Vogeli, C., & Mullainathan, S. (2019). Dissecting racial bias in an algorithm used to manage the health of populations. Science, 366(6464), 447–453. https://doi. org/10.1126/science.aax2342
Savitt, T. L. (1982). The Use of Blacks for Medical Experimentation and Demonstration in the Old South. The Journal of Southern History, 48(3), 331. https://doi. org/10.2307/2207450
Staton, L. J., Panda, M., Chen, I., Genao, I., Kurz, J., Pasanen, M., Mechaber, A. J., Menon, M., O’Rorke, J., Wood, J., Rosenberg, E., Faeslis, C., Carey, T., Calleson, D., & Cykert, S. (2007). When race matters: Disagreement in pain perception between patients and their physicians in primary care. Journal of the National Medical Association, 99(5), 532–538.
Sullivan, G. (n.d.). Plantation Medicine and Health Care in the Old South. 21.