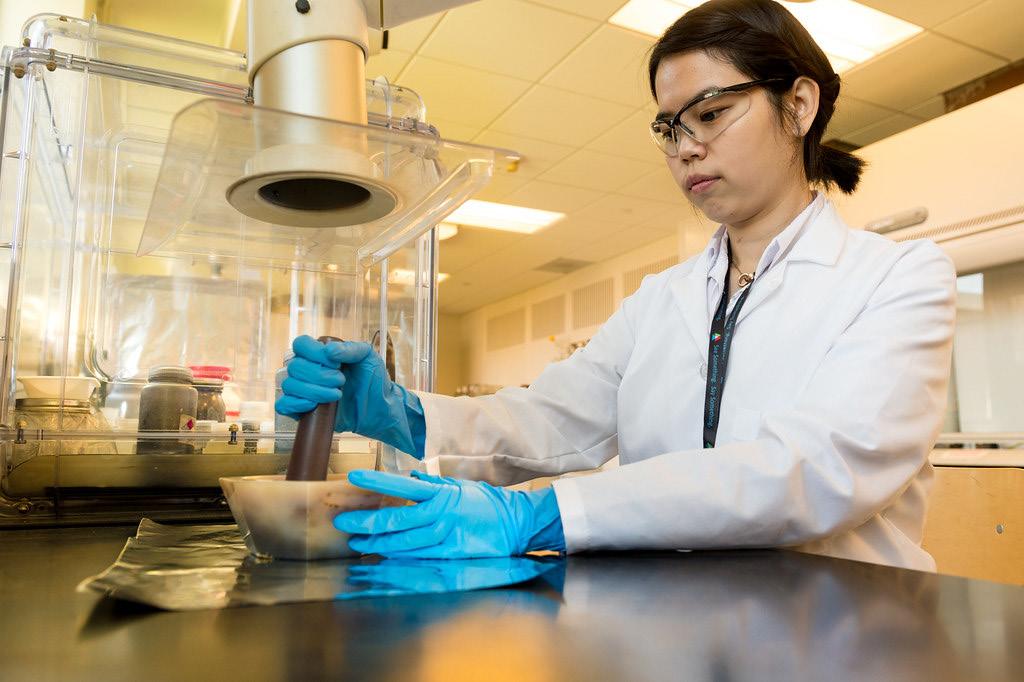
49 minute read
Mechanochemistry – A Powerful and “Green” Tool for Synthesis
BY ANDREW SASSER '23
Cover Image: This scientist is using a mortar and pestle to grind different reagents and facilitate a mechanochemical reaction. Unlike most synthetic reactions, mechanochemical reactions do not require any solvent
Advertisement
Source: Flickr.com Introduction Over the past two centuries, the field of synthetic chemistry has experienced remarkable growth. Prior to the 19th century, scientists accepted the doctrine of vitalism, which suggested that living organisms were fueled by a “vital force” separate from the physical and natural world, and that organic compounds could not be synthesized from inorganic reagents. However, following Friedrich Wohler’s 1828 synthesis of the organic molecule urea from the inorganic molecule ammonia, the field of synthetic chemistry exploded, with chemists producing compounds such as the anti-cancer drug Taxol and the pesticide Strychnine from simple organic and inorganic reagents (Museum of Organic Chemistry, 2011).
Modern synthetic techniques, however, still have some significant drawbacks. For one, synthetic schemes can be highly inefficient. On average, the synthesis of fine chemicals produces 5-50 kilograms(kg) of by-product per kg of product, and the synthesis of pharmaceuticals can generate over 100 kg of waste product per kg. Second, most syntheses use toxic solvents, which often comprise the largest amount of “auxiliary waste.” (Li and Trost, 2008). Third, reactions can also require a large amount of energy, especially if heating is necessary. As a result of these inefficiencies, the field of “Green Chemistry” has been developed to maximize atom economy – the ratio of the mass of the atoms in the product to that of the reagents – and thus promote efficiency (Chen et. al, 2015). In an effort to minimize waste products, some chemists have turned towards solventless reactions to promote higher product recovery. One class of solventless reactions, referred to as mechanochemistry, uses mechanical force to promote chemical reactions. This paper will demonstrate how mechanochemical reactions have not only reduced energy requirements and improved atom economy but have also
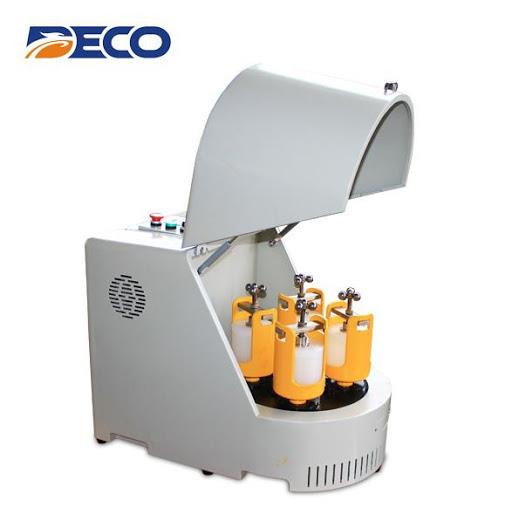
led to new reaction pathways that are not achievable under conditions where solvent is present
History of Mechanochemistry Although mechanochemical techniques have existed since the beginning of recorded history, their use in chemical synthesis has only developed recently. Prior to the late 20th century, mechanochemical techniques relied upon simple pestles and mortars. The famed English chemist Michael Faraday, for instance, used a mixture of zinc, tin, iron, and copper to reduce silver chloride (Takacs, 2013). In the 1880s, M.C. Lea discovered that mechanochemical processes can facilitate reactions not achievable by the heating of solutions. Lea found that mercuric chloride could decompose when ground in a mortar but would sublime (turn to gas) upon heating (Boldyreva, 2013).
Research in mechanochemistry substantially increased over the 20th century due to the evolution of techniques. As the mortar and pestle proved to be too cumbersome, scientists instead turned to ball milling devices that could supply the large amounts of energy needed to initiate chemical reactions. First developed in 1923, small-scale ball mills became increasingly popular throughout the 1940s and 50s (Takacs, 2013). Mill development has since focused on increasing the motion and energy of individual balls. For example, the planetary mill, first developed in 1961, makes use of a centrifuge to increase impact velocity. Similarly, Calka and Radlinski developed the uni-ball mill in 1991, which makes of use of magnets to better control the rate of milling (Calka and Radlinski, 1991).
Modern Techniques Further technical advances have enabled an even greater degree of control over mechanochemical reactions. Of particular importance in mechanochemistry are techniques which add catalytic material to a reaction mixture. One of these methods, called liquid-assisted grinding (LAG), has allowed for reaction pathways not traditionally accessible by neat grinding, or grinding with a mortar and pestle. LAG introduces a small amount of liquid into a reaction mixture, which greatly accelerates reaction rates while also promoting the formation of new isomeric products (Do & Friščić, 2017). LAG has also been shown to increase reaction yield; for example, when water was added in the oxidative addition step of the halogenation of Rhenium (I) complexes, yield increased from 82% to 94%. Additionally, the isomeric excess of the diagonal isomer increased from 45% to 99%, improving reaction selectivity (Hernandez et. al, 2014).
Another modern technique used in mechanochemistry is twin screw extrusion (TSE). In contrast to traditional “batch” mills, TSE results in a “continuous” mode of production as mechanical force is constantly applied to the products. In TSE, the material is passed through a confined barrel assisted by the rotation of two rotating screws. The configuration of these screws can be modified to control shear and compression forces on the molecule. Additionally, the feed rate of material can be modified to directly control the amount of force applied; this allows for greater temperature control than standard ball mills (Crawford et. al, 2017). TSE has been particularly useful in synthetic processes; for instance, Medina et. al produced pharmaceutical cocrystals from caffeine and oxalic acid – a process previously only achieved under solution crystallization (Medina et. al, 2010). As solution crystallization can lead to the formation of solvate products, TSE increases general yield by eliminating the need for solvent.
Figure I: Planetary ball mills in mechanochemistry take advantage of centrifugal force at high speeds. In planetary ball mills, “grinding jars” are arranged on a wheel and spin opposite to the direction of the wheel. The impact of the balls raises reaction rates by decreasing the surface area of the reactants.
Source: Wikimedia Commons
Mechanics of Mechanochemical Reactions With regard to mechanochemical synthesis, the energy required to overcome activation energy barriers is provided by the transformation
Figure 2: MOFs are comprised of metal ions coordinated to specific organic ligands. Normally synthesized under solution conditions, some MOFs have recently been produced through mechanical force Source: Wikimedia Commons
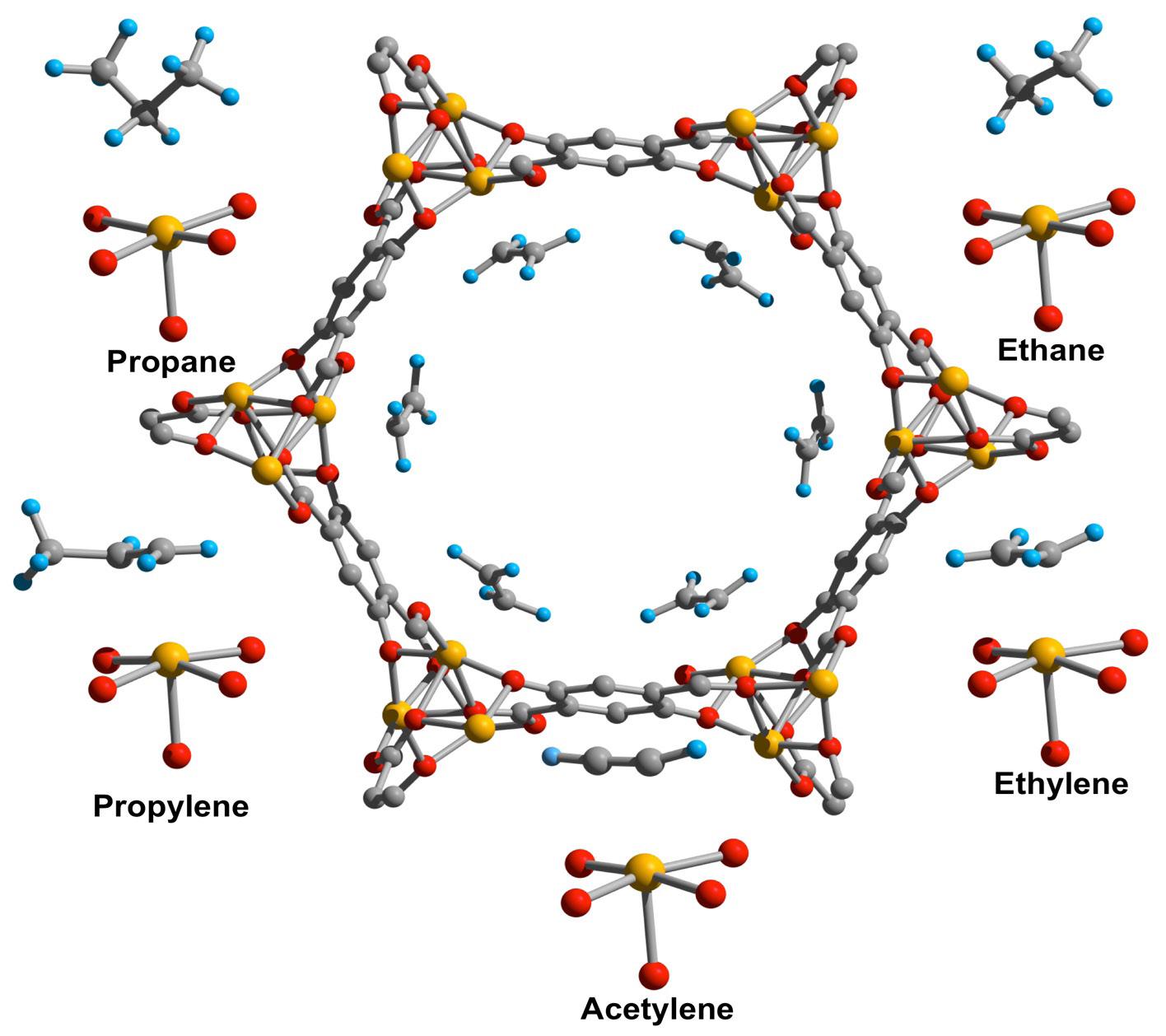
of mechanical work into heat energy, often provided through either deformation or friction forces. As suggested by PG Fox, prolonged direct contact between reactants is necessary for a solid-phase mechanochemical reaction to proceed (Fox, 1975). Evidence also suggests that mechanochemical reactions must occur in a liquid-like phase. The aldol condensation reaction, for instance, has shown to produce at least partial melts, where at least some of the reaction mixture is melted, in 67% of ketone/ aldehyde mixtures (Rothenberg et. al, 2001) Notably, when reactants are ground together, the melting point of the mixture drops significantly. For example, when both alpha glycine and beta-malonic acid were milled together a melt was observed at 60˚C, significantly lower than the standard 112˚C melting point of the salt product. This reduction in melting point significantly lowers the thermal requirement for reactions and generally increases reaction rates (Michalchuck et. al, 2014).
While mechanochemical reactions occur at significantly lower temperatures than solvent reactions, they are still governed by many of the same principles. For instance, the success of any reaction depends heavily on the nucleophilic or electrophilic character of the involved reagents. As described by Machucha and Juaristi, the yield for the mechanochemical dipeptide-catalysed aldol-condensation reaction increased from 7% for p-methoxybenzaldehyde to 84% for o-chlorobenzaldehyde. The increased electronwithdrawing effects associated with the o-chlorobenzaldehyde raised the reaction rate due to increased pi-stacking, as the electronrich napthyl ring on the catalyst formed a more rigid transition state with the electron-poor benzaldehyde (Machucha and Juaristi, 2015). Similarly, the mechanochemical synthesis of thioureas required 9 hours of milling when a 4 methoxy thiocyanate derivative was used as the electrophile, as reported by Štrukil. In contrast, only 3 hours of milling were required when the methoxy group was replaced with the more electron-poor nitro group, as the benzyl ring became more electron deficient and thus susceptible to nucleophilic attack by the aniline (Štrukil, 2017).
Mechanochemical reactions are also more sensitive than their solvent counterparts to changes in the stoichiometric ratio of reactants and temperature. As reported by Užarević et. al, the dry milling of cyanoguanidine and cadmium chloride produced a 1-dimensional polymer when ground in a 1:1 ratio, whereas a 3-dimensional polymer was formed when the reagents were ground in a 1:2 ratio (Užarević et. al, 2016). Notably, the group found that when the temperature of the milling reaction
Figure 3: This is the Reaction mechanism for Suzuki-Miyaura Cross Coupling, a common organometallic reaction used for producing carbon-carbon single bonds
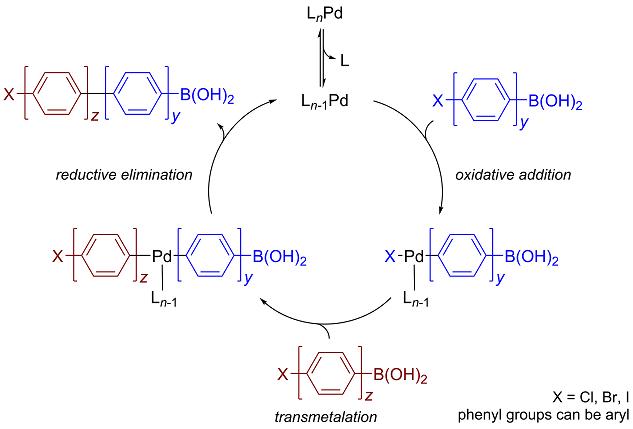
Source: Wikimedia Commons
was raised to 60˚C, there was an almost immediate formation of the 3-dimensional polymer, followed by rapid conversion to the 1-dimensional polymer once the cadmium chloride had been consumed. In contrast, at room temperatures, an amorphous intermediate phase that lasted for almost 20 minutes of ball-milling was observed (Užarević et. al, 2016).
Applications of Mechanochemical Synthesis Due to modern technical achievements, mechanochemistry has proven to be a versatile synthetic tool. Recent studies have demonstrated the potential use of mechanochemistry in metal-catalysed reactions. For instance, Fulmer et. al found that the Sonogashira coupling reaction could be replicated via high speed ball-milling without the need for a traditional copper iodide catalyst (Fulmer et. al, 2009). Instead, the group used a copper vial and copper balls as a catalytic surface for the reaction, which produced yields of up to 89% - comparable to the solvent reaction11. Similarly, Chen et. al found silver foil to be an effective catalyst in the cyclopropanation of alkenes with diazoacetates. In comparison to the standard catalyst for the reaction (dirhodium (II) salts), silver foil is both significantly more abundant and recyclable (Chen et. al, 2015). Another important application of mechanochemical reactions is in the synthesis of metal-organic frameworks, or MOFs. As porous materials made up of metal ions and organic ligands, MOFs have been proven to be potential candidates for fuel storage, carbon capture, and catalysis (Furukawa et. al, 2013). Pichon et. al demonstrated the potential for solvent free synthesis of MOFs through the reaction of copper acetate mononhydride, Cu(O2CCH3)2·H2O, with isonicotinic acid under ball milling conditions (Pichon et. al, 2006). Similarly, Friščić & Fábián synthesized Zinc fumarate, an MOF commonly used in functional materials, from Zinc oxide via liquid-assisted grinding (Friščić & Fábián, 2009). Significantly, the simple metal oxides in the mechanochemical synthesis of MOFs can replace the more expensive and toxic metal nitrates commonly used in current MOF synthesis (Do & Friščić, 2017).
Benefits of Mechanochemistry over Solvent Reactions Although mechanochemistry is a stillemerging field, it has already demonstrated significant advantages over traditional solution synthesis methods. Some of these advantages are explored below.
Reaction Energetics and Efficiency: Compared to common methods of microwave irradiation and solution heating, “Recent studies have demonstrated the potential use of mechanochemistry in metal-catalyzed reactions.”
mechanochemical reactions may be significantly more efficient at transferring energy for comparable yield. Experimental studies suggest that ball mills can deliver anywhere between 95.4 and 117.7 kJ mol-1 (McKissic et. al, 2014) As ball mills can deliver such a large quantity of energy, it is not surprising to see a corresponding increase in reaction rate compared to standard methods. A study conducted by Rodriguez et. al suggests that, on average, high-energy ball milling can drastically reduce reaction time for an enantioselective aldol reaction by over 50%. This reduction was achieved with similar yields and enantiomeric excess for both the stirring reactions and aldol reactions (Rodriguez et. al, 2006). Similarly, a study by Schneider et. al suggests that ball-milling has significant energy efficiency advantages over traditional microwave processes in Suzuki-Miyaura coupling reactions. Traditional microwave irradiation techniques were found to require 7.6 kWh mol-1 to produce 5 mmol of product, whereas planetary mills generated 100 mmol of product using just 5 kWh mol-1 of electrical energy (Schenider et. al, 2009).
Reaction Selectivity:
Mechanochemical reactions also enhance the selectivity of desired products as compared to solution conditions. Products with a higher degree of thermodynamic stability are generally preferentially selected due to the different thermodynamic environment associated with solid-state mechanochemical reactions. For instance, Balema et. al observed that the mechanochemical Wittig reaction preferentially selected for the more thermodynamically stable E-stilbenes; in contrast, solution environments normally produce mixtures of Z and E-stilbene isomer (Balema et. al, 2002). Similarly, Belenguer et. al observed that mechanochemical conditions drove the thermodynamic equilibration of a disulfide dimerization reaction from two homodimers (reactants) to heterodimers (products). The group suggested that this may be due to the fact that forcing one of the homodimers into an energetically unstable lattice structure raises the lattice energy by 12.7 kJ mol-1 compared to an isolated molecule in solution, thus reducing the activation energy and raising reaction rate (Belenguer et. al, 2011).
De Novo Synthesis:
Finally, some mechanochemical reactions have enabled the synthesis of products that could not be synthesized using other methods. One of these products is a dimer of C6o, better known as fullerene. Previously believed to be thermally impossible to produce due to the highly strained and electron-deficient double bonds, Shiro et. al successfully produced a C120 dimer using a high-speed vibration mill and potassium cyanide as a catalyst (Shiro et. al, 2009). In addition to circumventing the problems of hindered stereochemistry, mechanochemical pathways also avoid the common problem of solvent interference. As reported by Rheingold et. al, the synthesis of tris(allyl)aluminum complexes was only possible via ball-milling; attempts to conduct the synthesis in hexane solutions were entirely unsuccessful due to solvent interference (Rheingold et. al, 2014).
Conclusion While mechanochemical techniques have been used for thousands of years, only recently have they evolved to become a potential tool for synthesis. Thanks to modern technological innovations, synthetic chemists can more easily control the energy input of reactions, while also drastically speeding up the reaction rates. Additionally, mechanochemistry has promoted greater atom economy and reduced solvent waste in modern syntheses, making it an excellent example of green chemistry. Furthermore, due to the different chemical environment associated with solidstate chemistry, synthetic pathways can be fine-tuned to raise reaction speed, change selectivity and even synthesize “impossible” molecules. Though the physical underpinnings of mechanochemical techniques are still being explored, evidently, they present an incredibly viable alternative to traditional reaction pathways.
References
Balema, V. P., Wiench, J. W., Pruski, M., & Pecharsky, V. K. (2002). Mechanically Induced Solid-State Generation of Phosphorus Ylides and the Solvent-Free Wittig Reaction. Journal of the American Chemical Society, 124(22), 6244–6245. https://doi. org/10.1021/ja017908p
Belenguer, A. M., Friščić, T., Day, G. M., & Sanders, J. K. M. (2011). Solid-state dynamic combinatorial chemistry: reversibility and thermodynamic product selection in covalent mechanosynthesis. Chemical Science, 2(4), 696–700. https://doi.org/10.1039/C0SC00533A
Boldyreva, E. (2013). Mechanochemistry of inorganic and organic systems: what is similar, what is different? Chemical Society Reviews, 42(18), 7719–7738. https://doi.org/10.1039/ C3CS60052A
Calka, A., & Radlinski, A. P. (1991). Universal high performance ball-milling device and its application for mechanical alloying. Materials Science and Engineering: A, 134, 1350–1353. https://doi.org/10.1016/0921-5093(91)90989-Z
Cano-Ruiz, J. A., & McRae, G. J. (1998). Environmentally conscious chemical process design. Annual Review of Energy and the Environment, 23(1), 499–536. https://doi. org/10.1146/annurev.energy.23.1.499
Chen, L., Bovee, M. O., Lemma, B. E., Keithley, K. S. M., Pilson, S. L., Coleman, M. G., & Mack, J. (2015). An Inexpensive and Recyclable Silver-Foil Catalyst for the Cyclopropanation of Alkenes with Diazoacetates under Mechanochemical Conditions. Angewandte Chemie International Edition, 54(38), 11084–11087. https://doi.org/10.1002/ anie.201504236
Crawford, D. E., Miskimmin, C. K. G., Albadarin, A. B., Walker, G., & James, S. L. (2017). Organic synthesis by Twin Screw Extrusion (TSE): continuous, scalable and solvent-free. Green Chemistry, 19(6), 1507–1518. https://doi.org/10.1039/ C6GC03413F
Do, J.-L., & Friščić, T. (2017). Mechanochemistry: A Force of Synthesis. ACS Central Science, 3(1), 13–19. https://doi. org/10.1021/acscentsci.6b00277
Fox, P. G. (1975). Mechanically initiated chemical reactions in solids. Journal of Materials Science, 10(2), 340–360. https:// doi.org/10.1007/BF00540358
Friščić, T., & Fábián, L. (2009). Mechanochemical conversion of a metal oxide into coordination polymers and porous frameworks using liquid-assisted grinding (LAG). CrystEngComm, 11(5), 743–745. https://doi.org/10.1039/ B822934C
Fulmer, D. A., Shearouse, W. C., Medonza, S. T., & Mack, J. (2009). Solvent-free Sonogashira coupling reaction viahigh speed ball milling. Green Chemistry, 11(11), 1821–1825. https://doi.org/10.1039/B915669K
Furukawa, H., Cordova, K. E., O’Keeffe, M., & Yaghi, O. M. (2013). The Chemistry and Applications of Metal-Organic Frameworks. Science, 341(6149). https://doi.org/10.1126/ science.1230444
Hernández, J. G., Macdonald, N. A. J., Mottillo, C., Butler, I. S., & Friščić, T. (2014). A mechanochemical strategy for oxidative addition: remarkable yields and stereoselectivity in the halogenation of organometallic Re(I) complexes. Green Chemistry, 16(3), 1087–1092. https://doi.org/10.1039/ C3GC42104J
Kinne-Saffran, E., & Kinne, R. K. H. (1999). Vitalism and Synthesis of Urea. American Journal of Nephrology, 19(2), 290–294. https://doi.org/10.1159/000013463
Li, C.-J., & Trost, B. M. (2008). Green chemistry for chemical synthesis. Proceedings of the National Academy of Sciences of the United States of America, 105(36), 13197–13202. https://doi.org/10.1073/pnas.0804348105
Machuca, E., & Juaristi, E. (2015). Organocatalytic activity of α,α-dipeptide derivatives of (S)-proline in the asymmetric aldol reaction in absence of solvent. Evidence for noncovalent π–π interactions in the transition state. Tetrahedron Letters, 56(9), 1144–1148. https://doi.org/10.1016/j. tetlet.2015.01.079 McKissic, K. S., Caruso, J. T., Blair, R. G., & Mack, J. (2014). Comparison of shaking versus baking: further understanding the energetics of a mechanochemical reaction. Green Chemistry, 16(3), 1628–1632. https://doi.org/10.1039/ C3GC41496E
Medina, C., Daurio, D., Nagapudi, K., & Alvarez-Nunez, F. (2010). Manufacture of pharmaceutical co-crystals using twin screw extrusion: a solvent-less and scalable process. Journal of Pharmaceutical Sciences, 99(4), 1693–1696. https://doi. org/10.1002/jps.21942
Michalchuk, A. A. L., Tumanov, I. A., Drebushchak, V. A., & Boldyreva, E. V. (2014). Advances in elucidating mechanochemical complexities via implementation of a simple organic system. Faraday Discussions, 170(0), 311–335. https://doi.org/10.1039/C3FD00150D
Pichon, A., Lazuen-Garay, A., & James, S. L. (2006). Solventfree synthesis of a microporous metal–organic framework. CrystEngComm, 8(3), 211–214. https://doi.org/10.1039/ B513750K
Rightmire, N. R., Hanusa, T. P., & Rheingold, A. L. (2014). Mechanochemical Synthesis of [1,3-(SiMe 3 ) 2 C 3 H 3 ] 3(Al,Sc), a Base-Free Tris(allyl)aluminum Complex and Its Scandium Analogue. Organometallics, 33(21), 5952–5955. https://doi.org/10.1021/om5009204
Rodríguez, B., Rantanen, T., & Bolm, C. (2006). Solvent-Free Asymmetric Organocatalysis in a Ball Mill. Angewandte Chemie, 118(41), 7078–7080. https://doi.org/10.1002/ ange.200602820
Rothenberg, G., Downie, A. P., Raston, C. L., & Scott, J. L. (2001). Understanding Solid/Solid Organic Reactions. Journal of the American Chemical Society, 123(36), 8701–8708. https://doi. org/10.1021/ja0034388
Schneider, F., Szuppa, T., Stolle, A., Ondruschka, B., & Hopf, H. (2009). Energetic assessment of the Suzuki–Miyaura reaction: a curtate life cycle assessment as an easily understandable and applicable tool for reaction optimization. Green Chemistry, 11(11), 1894–1899. https://doi.org/10.1039/ B915744C
Štrukil, V. (2017). Mechanochemical synthesis of thioureas, ureas and guanidines. Beilstein Journal of Organic Chemistry, 13(1), 1828–1849. https://doi.org/10.3762/bjoc.13.178
Takacs, L. (2013). The historical development of mechanochemistry. Chemical Society Reviews, 42(18), 7649–7659. https://doi.org/10.1039/C2CS35442J
Taxol – The Drama behind Total Synthesis. (2011, July 27). https://web.archive.org/web/20110727152818/http://www. org-chem.org/yuuki/taxol/taxol_en.html
Užarević, K., Štrukil, V., Mottillo, C., Julien, P. A., Puškarić, A., Friščić, T., & Halasz, I. (2016). Exploring the Effect of Temperature on a Mechanochemical Reaction by in Situ Synchrotron Powder X-ray Diffraction. Crystal Growth & Design, 16(4), 2342–2347. https://doi.org/10.1021/acs. cgd.6b00137
Wang, G.-W., Komatsu, K., Murata, Y., & Shiro, M. (1997). Synthesis and X-ray structure of dumb-bell-shaped C 120. Nature, 387(6633), 583–586. https://doi.org/10.1038/42439
Fast Fashion and the Challenge of Textile Recycling
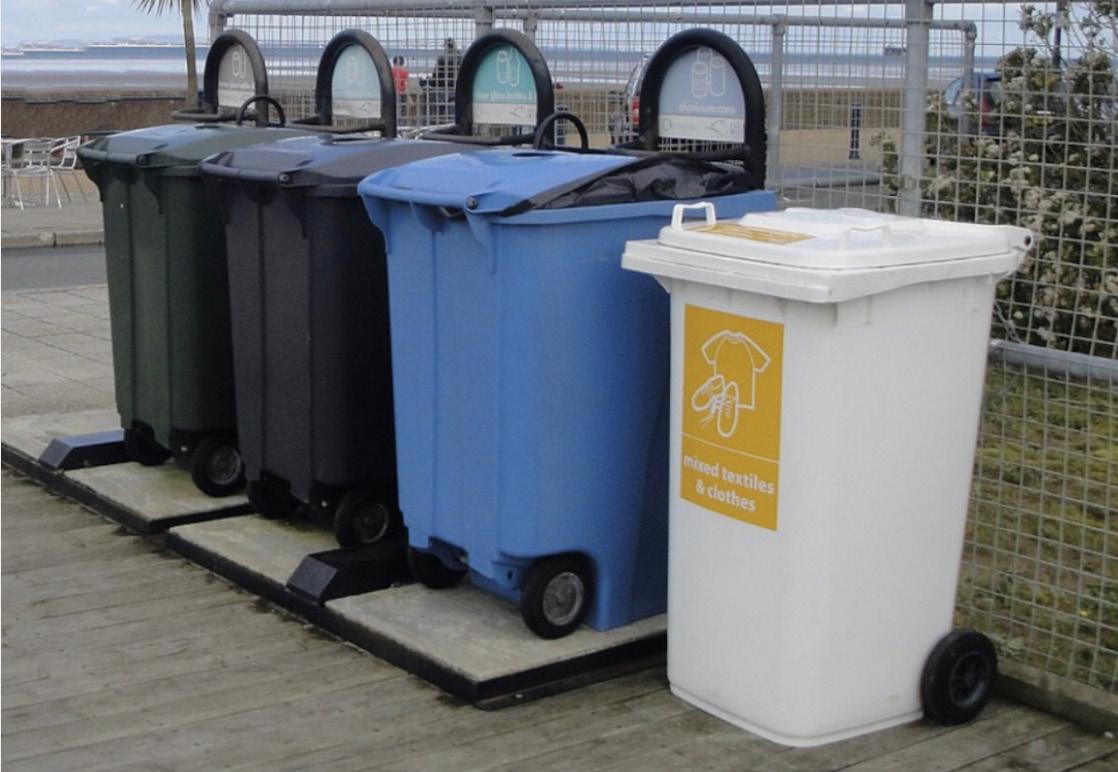
BY ARUSHI AGASTWAR, MONTA VISTA HIGH SCHOOL SENIOR
Cover Image: Mixed textiles and clothing recycling bin alongside bins for plastic, glass, and aluminum bottles
Source: Wikimedia Commons Introduction In recent decades, the textile industry has seen tremendous growth due to an increase in general population size, a growing middle class in particular, and the presence of higher disposable income. Alongside these broader trends, this growth has also been influenced by a new surge in textile and apparel consumption referred to as Fast Fashion. Consumer fashion brands like Zara, Forever 21, and H&M are disrupting the seasonal design cycles of clothes. Traditional legacy brands like Tommy Hilfiger, Levis, and Dolce and Gabbana used to have around two or three seasonal lines in a year. Now, there is an entirely new collection of apparel put out on a biweekly basis. Fast Fashion brands leverage the urgency created by short supply cycles, causing people to either purchase immediately or miss out on the latest trends. The business model of these brands is to swiftly make clothes that are inexpensive and expendable - and the quick turnaround of raw material to finished apparel has been facilitated greatly by social media platforms (Caro & Martínez-de-Albéniz, 2015). Social media influencers constantly affect social media feeds with new styles, creating an apparent psychological need to keep up with new trends. Unfortunately, however, this Fast Fashion model is neither cost-effective nor sustainable for the environment.
Today, the average individual is purchasing more clothes than ever before. Due to a steady rise in clothing consumption during the past three decades, more than 80 billion garments were produced worldwide in 2019 (Thomas, 2019). For the American Market, this increase translates to the average American consumer purchasing more than 63 pieces of clothing per year, or 1.2 garments a week, which is more than 6 times the global average (Bick et al, 2018). Such large-scale consumption of clothing has led to significant problems, such
Figure 1: The long-term environmental impacts of the textile industry is often overlooked.
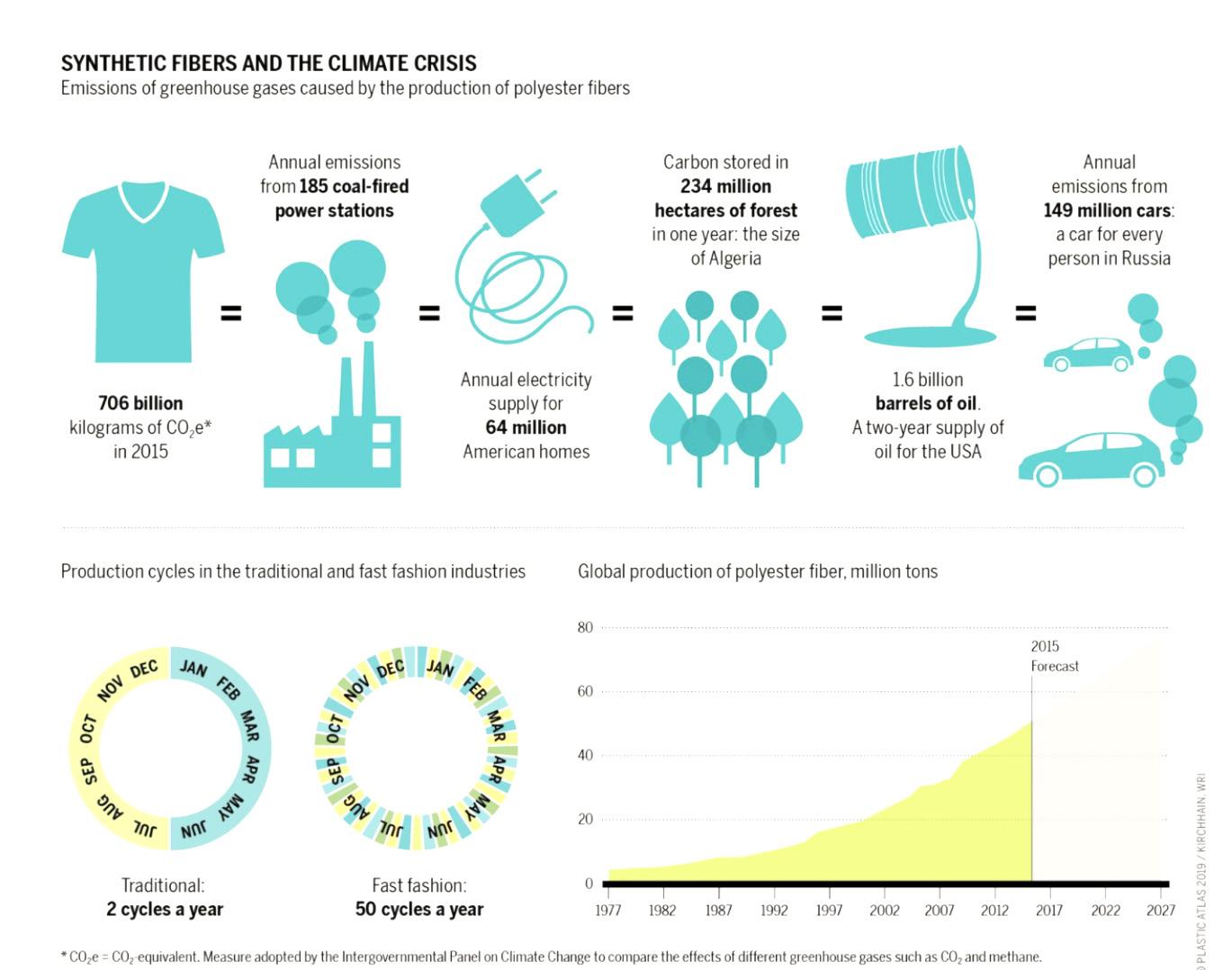
Source: Flickr
as unwanted garments and textiles being prematurely discarded. Due to consumers holding onto clothing for only half as long as they used to, around 82 pounds of clothing are typically thrown away each year. Of this, around 85 percent ends up in the landfill and only half of the other remaining 15 percent is reused, whilst the other half is recycled (Bick et al., 2018; Wicker, 2016).
Why We Need Recycling According to the Ellen MacArthur Foundation, the textile production process is often highly resource-intensive. In 2015, the total Greenhouse Gas Emissions (GHG) for the textile and apparel industry was 1,200 million tons. Additionally, water usage by the industry was 93 billion cubic meters, fertilizers for cotton growth were 8 million tons, and pesticides for cotton were 200,000 tons (Ellen MacArthur Foundation, 2017).
In the textile industry, there are dozens of types of fabrics. Synthetic fabrics like polyester, nylon, rayon, acrylic, and elastane are based on plastics and designed to last longer. This causes them to take hundreds of years to decompose. This is also the case with natural fabrics including cotton, linen, wool, and silk. During the fabric manufacturing process, the fibers go through a series of processes like dyeing and finishing, which renders these fabrics difficult to decompose (Mukhopadhyay, n.d). Once in landfills, an apparel made of natural fibers is several hundred times more slow to degrade compared to unprocessed raw fiber which has not been converted to yarn or fabrics. Additionally, their decomposition can lead to the release of certain greenhouse gases in the atmosphere which contribute to increasing global temperatures.
Recycling textiles can have a substantial environmental benefit, including a 53% reduction in greenhouse gas emissions, a 45% reduction in chemical pollution, and a 95% reduction in water pollution (ECOSIGN, 2017). And Textile recycling also substantially reduces the dependence on virgin natural resources and allows us to extract the maximum value out of fibers. This is a strong positive because the globe is currently facing increasingly high population projections that demand resources for settlement and sustenance. As a result, societies will have to choose between using land for living, land for food cultivation, land for growing cotton, and land for grazing sheep for
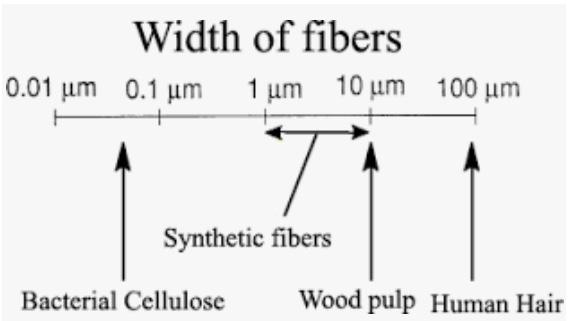
Figure 2: Width of a synthetic fiber as compared to other materials
Source: Wikimedia Commons
Figure 3: How mechanical recycling compares to other forms of municipal waste Source: Flickr
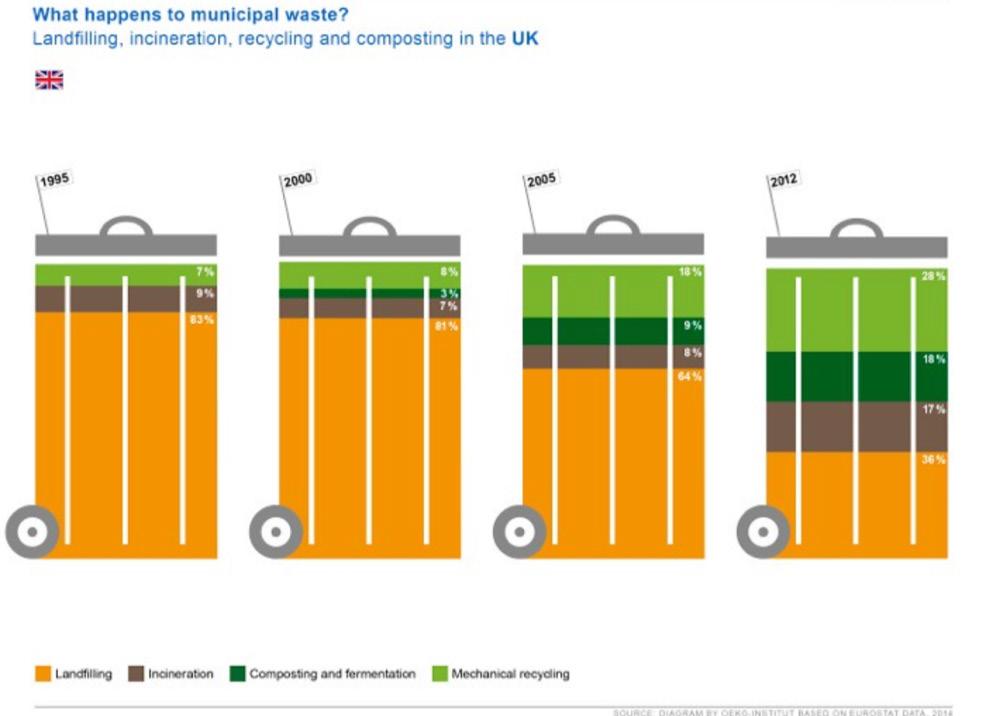
wool. Textile recycling is a major way to lessen the desperateness of the situation. For every piece of cloth recycled, the amount of resources used to produce virgin fabric is significantly reduced (Watson, 2017).
The processes leading up to recycling include the collection of textiles from various sources, consolidation of the textiles, classification according to fabric type and color, and transportation from the collection sites to the sorting facilities. As each of these individual steps is highly labor-intensive, they generate a multitude of employment opportunities. This, in addition to the environmental benefits of reduced landfill and incineration (which helps reduce air pollution), are further positive aspects of plastic recycling (Tai J, et al., 2017).
The Recycling Process Textiles are recycled in two prominent ways today: mechanical and chemical.
During mechanical recycling, the fabrics are shredded into shorter fibers. However, these shorter fibers yield a yarn of lower quality and strength and are difficult to turn back into fabrics; shredded materials are optimum for “non-woven” fabrics. As non-woven fabrics are formed directly from fibers, there is no need for yarn formation and weaving or knitting the yarn. As shown by the data in Figure 3, the percentage of municipal waste produced by mechanical recycling has been increasing over the past few decades. The most recent data point shows that the percentage of municipal waste produced by mechanical recycling is relatively similar to the percentage of municipal
waste produced by landfilling (Payne, 2013).
During chemical recycling, natural fibers such as cotton, linen, or bamboo, which are made of cellulose, can be dissolved much like paper. Nonetheless, after every successive dissolution, the length of the cellulosic polymer chains gets shorter, resulting in a weak yarn and substandard fabric. Even after the first round of recycling, the cotton fibers are not long enough to be properly knitted or woven. To obtain a higher fabric strength, textile industries need to bundle these fibers with virgin fibers (Payne, 2013).
Challenges in Textile Recycling Globally, these textile fibers are often blended together to achieve special optical effects or to improve performance by maximizing the beneficial properties of each fiber type. However, these blends pose significant challenges when it comes to the recycling of the fabrics – each fiber has a different recycling process. Since the blending is done at the fiber stage, the mechanical separation process of these blended fibers has not yet been developed, making it difficult to recycle a blended fabric (Ellen MacArthur Foundation, 2017).
Some other issues in recycling are:
1. The processing, dyes, and finishes on the raw textile fabric make it very difficult to recycle the material. This is because dyes and finishes are chemically bonded to the surface of the fabric. The chemical bonds between the dyes, finishes and fiber polymers like cellulose, polyester,
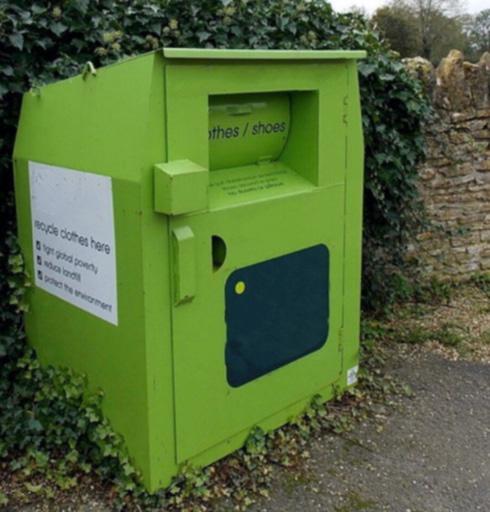
nylon are specifically engineered for each type of dye and fabric. This allows the fabrics to hold onto the dyes and finishes for a long time, preventing damage with successive washing or due to sunlight. As a result, they are particularly hard to separate from the fabrics (Marconi et al., 2018).
2. Some apparel is now supplemented with metal wires and various polymer materials (such is the case with technical textiles). These blends of electrical components are done at the weaving or the knitting stage of the fabric production and pose a serious challenge to fabric recycling. Mechanical recycling, which is done using shredding of fabric, will not be able to separate these wires from the fabric (Tai et al., 2011).
3. The fibrous material collected after mechanical recycling fabric by shredding is often inconsistent. It is difficult to change the process parameters for each new batch that comes in. This is because the machine settings used for the yarn production from this recycled fiber is dependent on the fiber length. For the recycling process to run seamlessly, there has to be a consistent increase in the amount of acceptable textile waste as input for the facility to be profitable (Xiao et al., 2018).
Discussion Consumers are becoming increasingly aware of the consequences of human consumption. As a result, clothing brands are introducing new and more ‘conscious’ lines to cater to this market segment. However, these eco-friendly brands and clothing lines are often priced at a premium and are therefore largely inaccessible to the vast majority of the population who have lesser disposable incomes to spend on clothes. Some companies are even criticized for the practice of ‘greenwashing’, or misleading ecoconscious consumers into thinking that their products are environmentally friendly when they really are not any more so than other fabrics on the market.
Several brands have implemented approaches that do not directly address the issue of textile biodegradation and recycling. H&M stores, for instance, have recollection bins where people can discard their clothes and receive H&M cash coupons in exchange
To be more environmentally sustainable, the textile industry needs to make significant changes regarding its use of resources. In the past, there has not been an adequate consideration of the environmental impacts of the textile production process. With fast fashion changing the way people purchase and interact with their clothes, the dangers of textile production to the environment have become increasingly pertinent.
References
Wicker On 09/01/16 at 6:40 AM EDT, A. (2017, March 16). The earth is covered in the waste of your old clothes. https:// www.newsweek.com/2016/09/09/old-clothes-fashionwaste-crisis-494824.html.
Caro, F., & Martínez-de-Albéniz, V. (1970, January 1). Fast Fashion: Business Model Overview and Research Opportunities. https://link.springer.com/ chapter/10.1007/978-1-4899-7562-1_9.
Circular Fashion - A New Textiles Economy: Redesigning fashion's future. (2017, November 28). https://www. ellenmacarthurfoundation.org/publications/a-new-textileseconomy-redesigning-fashions-future.
Claudio, L., Siegle, L., MA. Sant'Ana, F. K., S. Akhter, S. R., G. Gebremichael, A. K., & TP. Lyon, A. W. M. (1970, January 1). The global environmental injustice of fast fashion. https://doi. org/10.1186/s12940-018-0433-7.
Cuc, S., & Vidović, M. (1970, January 1). [PDF] Environmental Sustainability through Clothing Recycling: Semantic Scholar. undefined. https://www.semanticscholar.org/paper/ Environmental-Sustainability-through-Clothing-Cuc-Vidovi% C4%87/81069e26c688be1d475a1337f40fe68e6414969f.
Elander, M., & Ljungkvist, H. (2016). Critical aspects in design for fiber-to-fiber recycling of ...http:// mistrafuturefashion.com/wp-content/uploads/2016/06/MFFreport-2016-1-Critical-aspects.pdf.
Marconi, M., Landi, D., Meo, I., & Germani, M. (2018, April 18). Reuse of Tires Textile Fibers in Plastic Compounds: Is this Scenario Environmentally Sustainable? Procedia CIRP. https://www.sciencedirect.com/science/article/pii/ Figure 4: Public Recycling bin for textiles and shoes
Source: Geograph
Mukhopadhyay, S. Textile Engineering - Textile Fibres. NPTEL. https://nptel.ac.in/courses/116/102/116102026/.
Payne, A. (2015, August 7). Open- and closed-loop recycling of textile and apparel products. Handbook of Life Cycle Assessment (LCA) of Textiles and Clothing.https://www. sciencedirect.com/science/article/pii/B978008100169100006X.
Tai, J., Zhang, W., Che, Y., & Feng, D. (2011). Municipal solid waste source-separated collection in China: A comparative analysis. Waste management (New York, N.Y.). https://pubmed.ncbi.nlm. nih.gov/21504843/.
Textile recycling as a contribution to circular economy and production waste enhancement. View Cart. http://www. ecosign-project.eu/news/textile-recycling-as-a-contributionto-circular-economy-and-production-waste-enhancement/.
Thomas, D. (2019, August 29). The High Price of Fast Fashion. The Wall Street Journal. https://www.wsj.com/articles/thehigh-price-of-fast-fashion-11567096637.
Watson, D., Gylling, A., Andersson, T., & Heikkilä, P. (1970, January 1). Textile-to-textile recycling: Ten Nordic brands that are leading the way. VTT's Research Information Portal. https:// cris.vtt.fi/en/publications/textile-to-textile-recycling-tennordic-brands-that-are-leading-t.
Women's Clothing & Fashion - shop the latest trends: H&M GB. H&M. https://www2.hm.com/en_gb/ladies.html.
Xiao, S., Dong, H., Geng, Y., & Brander, M. (2018, March 22). An overview of China's recyclable waste recycling and recommendations for integrated solutions. Resources, Conservation and Recycling. https://www.sciencedirect.com/ science/article/pii/S0921344918300910.
Zara.com. JOIN LIFE: ZARA United States. JOIN LIFE | ZARA United States. https://www.zara.com/us/en/ sustainability-l1449.html.
The Cellular Adhesion and Cellular Replication of SARS-COV-2
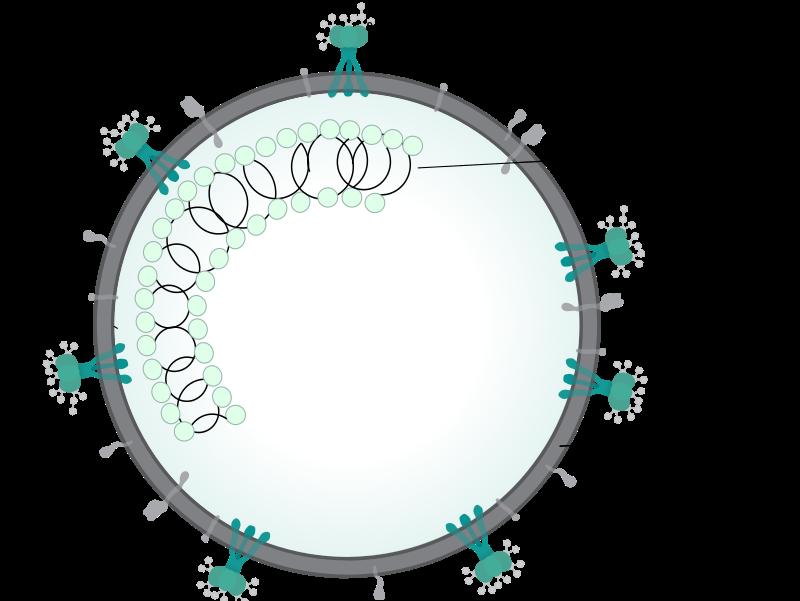
BY ARYA FAGHRI, UNIVERSITY OF DELAWARE '24
Cover Image: A depiction of the extracellular component of the SARS-COV-2 cell. All the membrane proteins have specific functions in both entry to the human cell and activity within the human cell.
Source: Wikimedia Commons Introduction COVID-19 is a viral disease caused by an infection from the Severe Acute Respiratory Syndrome Coronavirus or SARS-COV-2. The coronavirus itself is a sphere-shaped virus containing a plasma membrane with various proteins that protect its single-stranded RNA genome (Patel, 2020). This virus is zoonotic, meaning that it initially jumped from another species to a human host, most likely a pangolin or a bat (Patel, 2020). Once in the human body, the coronavirus enters cells so that it can multiply. It enters via the process of cellular adhesion in which it attaches its surfacelevel proteins to a specific human receptor enzyme called Angiotensin Converting Enzyme II (ACE-2) (Patel, 2020). ACE-2 is a type I integral membrane enzyme categorized as a carboxypeptidase, responsible for the conversion of angiotensin II to Angiotensin (17) (Warner, 2004). Once inside the human cell, the coronavirus looks to hijack the biosynthetic machinery within that cell to reproduce its genomic RNA and proteins to form new copies of itself. Having undergone this cellular replication, the newly produced coronavirus is then released into the extracellular fluid and can travel to other cells to complete this same process.
Severe Acute Respiratory Syndrome Coronavirus 2 (SARS-COV-2) and its mechanics Coronaviruses are positive-strand (5’ to 3’) RNA viruses that have a variety of proteins embedded within their plasma membrane, a set of intracellular proteins, and a 30,000 nucleotide-long RNA genome also packed within the cell (Schelle et al., 2005). Proteins within the plasma membrane bind ACE-2 receptor enzymes on human cells to facilitate viral uptake (National Institute of Health NIAD Team, 2020). Spike (S) glycoproteins and
hemagglutinin esterase dimers specifically facilitate this bonding, while membrane (M) proteins form much of the protein coat that contains the virus interior. Envelope (E) proteins regulate viral assembly once the virus is inside the cell. Lastly, novel structure proteins (nsp’s) are proteins of very small size that exhibit an assisting role in the coronavirus replication and assembly processes (Schelle et al., 2005, Zeng et al., 2008, Boopathi et al., 2020). The coronavirus genome is highly protected within the viral capsid (protein coat). One set of protective proteins are the nucleocapsid (N) proteins, which form the intracellular component of the virus (Schelle et al., 2005). The viral RNA genome is constantly replicated, transcribed, and translated to multiply and spread throughout the human body. The virus can be compared to other viruses such as influenza; however, the timing and the degree of effects toward organ systems produced by the coronavirus is distinctive. Particularly, the coronavirus strategically ceases the activity of any organs associated with the immune system which allows it to be mobile and capable of severe harm.
Angiotensin Converting Enzyme II (ACE-2) and its mechanics Angiotensin Converting Enzyme II, more commonly known as ACE-2, is related to Angiotensin Converting Enzyme, ACE, which is an enzyme in the human body that helps maintain blood pressure (among other roles) (Warner, 2004, Turner, 2015). The main cellular function ACE-2 possesses is to convert the protein Angiotensin II to Angiotensin (1-7) (R&D Systems, 2015).
3.1 Structure:
ACE-2 is a wrench-shaped receptor protein consisting of an N-terminal signal sequence, a transmembrane domain, a single catalytic domain, and a C-terminal cytosolic domain (Turner, 2020). The single catalytic domain is the outermost portion of the protein, the N-terminal signal sequence is also found outside the cell (extracellular), and the C-terminal signal sequence is found inside the cell (intracellular) (Clarke et al., 2011).
3.2 Location:
ACE-2 is a receptor protein located on the plasma membranes of lung cells, skin cells, stomach cells, liver cells, kidney cells, bone marrow cells, and much of the respiratory tract (Hamming el at., 2004). Recent studies have found that ACE-2 is also located on the hypothalamus, brainstem, and cerebral cortex, leading to the concern that the brain is also in danger of having its cells affected by the coronavirus (Kabbani el at., 2020).
3.3 Biological Function:
The primary function of ACE-2 is to split the protein Angiotensin II into the protein Angiotensin (1-7) which serves to block organ damage and helps control blood pressure (R&D Systems, 2015, Hiremath, 2020, Sriram et al., 2020). The splitting occurs in the extracellular fluid and also acts in an anti-inflammatory capacity by reducing the activity of bradykinin, a peptide that instigates inflammation (Cyagen Newsletter, 2020, UniProt, 2020). Furthermore, ACE-2 regulates the human gastrointestinal microbiome as well by primarily defending the digestion and metabolization processes of the large intestine from inflammation (Kuba, 2013). "The primary functino of ACE-2 is to split the protein Angiotensin II into the protein Angiotensin(1-7), which serves to block organ damage and helps control blood pressure.”
Affinity of the Coronavirus for ACE-2 The coronavirus is most likely to enter the body through the respiratory system because respiratory droplets from afflicted people contain viral particles (Ghose, 2020). These droplets can travel through the air a remarkable distance and enter a healthy person’s body (Atkinson, 2009, Ghose, 2020) Within several hours, the coronavirus particles will cross the mucous membranes of the respiratory system and enter the cells of the human body (Anderson et al., 2020).
When the coronavirus enters through the respiratory tract, it makes its way towards healthy cells containing ACE-2 receptors to begin the attachment process. Why does the coronavirus have such a high affinity for ACE2? First, the structural features of ACE-2 make it favorable for the coronavirus. Specifically, ACE2 has a more compact conformation in its single catalytic domain which gives it a strong affinity for the coronavirus. It also has an S protein that has a structural binding complementary to ACE-2 (Shang, 2020).
Once the coronavirus has identified a host cell, there are a series of reactions that allow it to move intracellularly, beginning with action from the S protein (Zeng et al., 2008, Boopathi et al., 2020), The S protein itself consists of a receptorbinding domain known as subunit S1, an intracellular backbone, and a transmembrane anchor known as the S2 subunit (Li, 2016). The
Figure 1: The initial translation process completed to produce pp1a and pp1ab.
Image created by author. Inspiration derived from: (Sino Biological, 2006) (Burkard, 2014) (Smirnov, 2018)
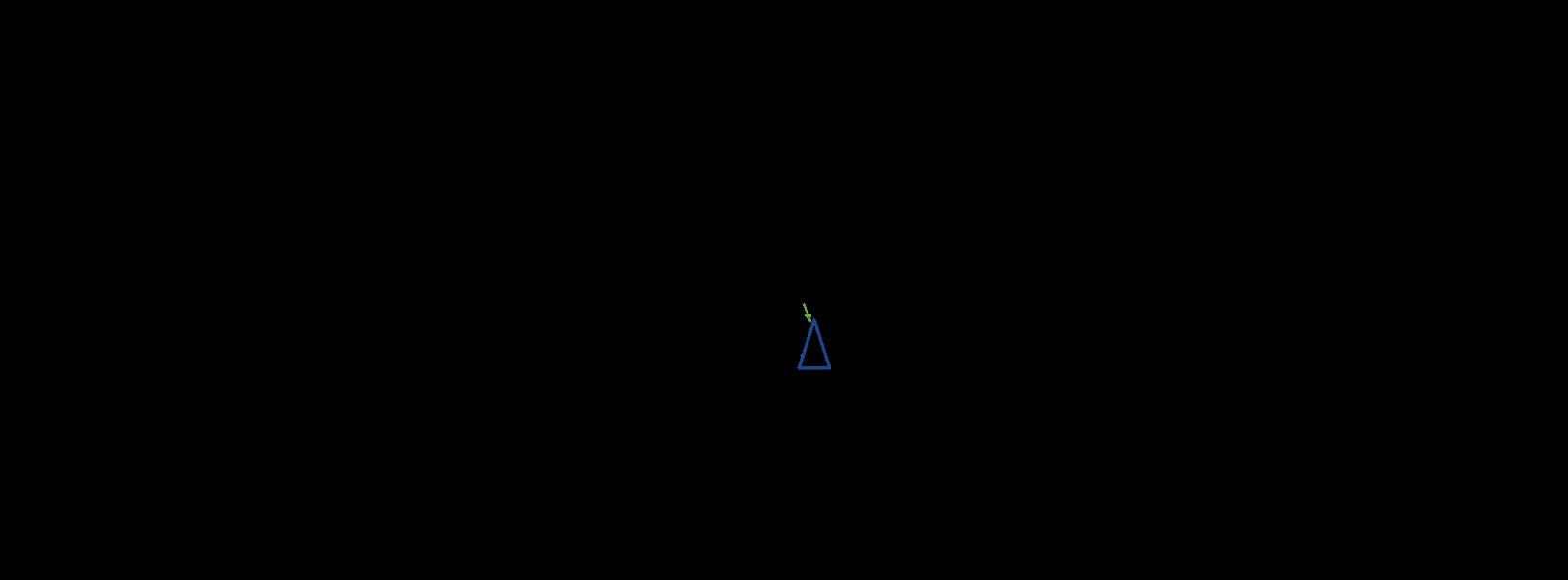
S1 subunit makes up the outer surface of the S protein and it is the boundary that faces ACE-2 during attachment (Lee, 2020). Fusion begins when the N-terminal of the S1 subunit on the S protein of the coronavirus binds to the ACE-2 receptor in a complementary fashion (Verdecchi et al., 2020, Lee, 2020). Once that initial binding occurs, a structural change in the S protein takes place which activates another receptor known as transmembrane protease serine 2 receptor (TMPRSS2) which shares a very close position on the plasma membrane with ACE-2 and plays a large role in viral entry in general (Hiremath, 2020, Verdecchi et al., 2020, Lee, 2020). TMPRSS2 cleaves the S protein between the S1 and S2 subunits (Verdecchi et al., 2020). Once the S protein is cleaved, the S2 subunit completes the attachment process by exposing a fusion peptide that is attached to the phospholipid bilayer (Verdecchi et al., 2020, Lee, 2020, Di Giorgio et al., 2020). Once the fusion occurs, the coronavirus travels through the phospholipid bilayer of the cell and is ready to go through a series of reactions and changes to complete its goal of replicating (Lee, 2020).
Post-fusion, ACE-2 is bound by the S protein and is therefore unable to perform its designated function (Han et al., 2006, Xu et al., 2017, National Heart, Lung, and Blood Institute, 2020). This is dangerous because ACE-2 protects the pulmonary system from inflammation (Zhang, 2020, Povlsen et al., 2020). Specifically, ACE-2 has a protective role in acute-lung failure as it delivers key contributions to lung protection and security which prompts that the loss of ACE-2 function will instigate inflammatory contusions on the lungs and the components of the respiratory tract (Kuba, 2005, Imai, 2005, Verdecchi et al., 2020). coronavirus The coronavirus has a pre-programmed objective of growing and generating an abundant supply of exact copies in a process known as viral replication (Wessner, 2010). Viral replication allows the virus to survive and thrive, eventually invading organ systems. Because the coronavirus can’t divide and replicate on its own, it hijacks and utilizes the organelles, molecular reactions, and metabolic processes of a human host cell to carry out the synthesis of necessary nucleic acids and proteins (Boundless Microbiology, 2020). This allows the coronavirus to exploit host cell capabilities in membrane fusion, translation, proteolysis, replication, and transcription (Shereen et al., 2020). These processes are all different operations that the RNA can conduct in order to modify, edit, and produce a new RNA strand. Additionally, the coronavirus will utilize organelles as well, namely the rough endoplasmic reticulum, which is involved with ribosomes in protein synthesis, the golgi apparatus, which modifies the newly produced proteins and ships them to the appropriate location in the cell, vesicles, which serve as transporters of material across the cell, and the cytosol which is the fluid between the plasma membrane and the organelles (Shereen et al., 2020). The coronavirus will spend most of its time and effort in the ribosomes and the cytosol with the rough endoplasmic reticulum, golgi apparatus, and vesicles mostly serving as preparation for exocytosis, or exit from the cell. As a whole, the entirety of the coronavirus replication process can be broken down into a four-step process: attachment and fusion as described in the preceding section, virus disassembly, viral biosynthesis, and virus assembly (Hammer, 2020).
Virus Disassembly:
Figure 2: The replicase/ transcriptase complex completed to produce the mRNA template Image created by author. Inspiration derived from: (Sino Biological, 2006) (Burkard, 2014)
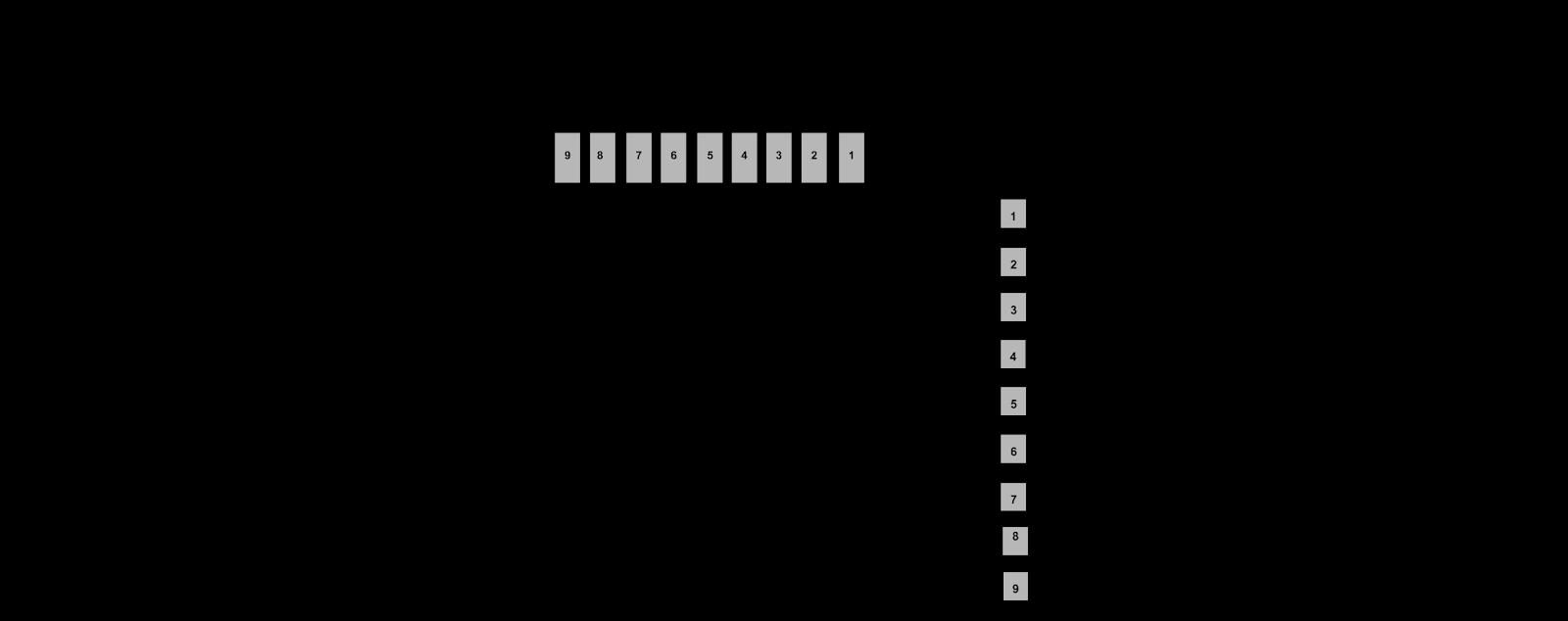
(Smirnov, 2018)
The coronavirus, consisting of all its proteins and RNA once in the cytosol, is ready to be disassembled via membrane uncoating (Haywood, 2010). Membrane uncoating is completed by lysosomes —groups of enzymes that hydrolyze and recycle excess material in the cell— within the host cell and involves separating the viral membrane and exposing the intracellular single-stranded RNA for it to be utilized throughout the replication process (Haywood, 2010). With this process complete, the 30,000-nucleotide genomic RNA is now uncoated and prepared to begin the viral biosynthesis step of replication.
Viral Biosynthesis:
The positive genomic RNA strand (5’ to 3’) will now begin a series of biosynthetic steps to produce new viral RNA and viral proteins. First, the RNA will undergo translation. The translation step begins with the coronavirus RNA attaching and fusing into a nearby ribosome in the cytosol (Sino Biological, 2006). Then, ORF1a, one region of the viral RNA genome, is translated to polyprotein 1a (pp1a) before being halted by an RNA pseudoknot located right before the termination codon of ORF1a (Ziebuhr et al., 1999, Fehr et al., 2015). The RNA pseudoknot on the slippery sequence alters the reading frame by using the translating ribosome to shift the reading frame one nucleotide in the negative direction (-1) of RNA (Lim et al., 2016). This process is known as programmed -1 ribosomal frameshifting (-1 PRF) and it causes this shift of a nucleotide to allow the ribosome to bypass the ORF1a termination codon and keep translation going for the ORF1b (Plant et al., 2008). The translation process is then complete at the end of ORF1b and results in a larger hybrid protein that covers two open reading frames known as polyprotein 1ab (pp1ab) (Ziebuhr et al., 1999). With pp1a and pp1ab now produced through translation, the two polyproteins will undergo proteolysis, a process in which proteins are degraded to smaller component polypeptides, which results in the production of fifteen novel structure proteins (nsp) (Wit et al., 2016, Nature, 2020).
Second, the initial positive genomic coronavirus RNA strand (5’ to 3’) will go through a replicasetranscriptase complex which begins with the initial coronavirus RNA traveling down the cytosol to link and combine with the fifteen novel structure proteins that were produced in the translation phase (Wit et al., 2016). With the initial RNA covered with novel structure proteins, it will now replicate itself and produce a negative genomic RNA strand (3’ to 5’) in the opposite order compared to the initial positive genomic RNA strand (Sino Biological, 2006, Wit et al., 2016).
The first molecular process of replication will use the negative RNA strand to produce a positive genomic coronavirus RNA strand (5’ to 3’) - one that is very similar to the initial RNA from the attached coronavirus that originally fused into the cytosol (Sino Biological, 2006, Wit et al., 2016). This newly produced positive genomic RNA strand will make up the intracellular aspect of the new coronavirus (Sino Biological, 2006). The RNA strand will travel further down the cytosol where it will wait until the assembly stage. The second “The positive genomic RNA strand (5' to 3') will now begin a series of biosynthetic steps to produce new viral RNA and viral proteins.”
Figure 3: The final translation process completed to produce the viral proteins that are constructed along with the RNA to produce the new coronavirus cell.
Image created by the author.
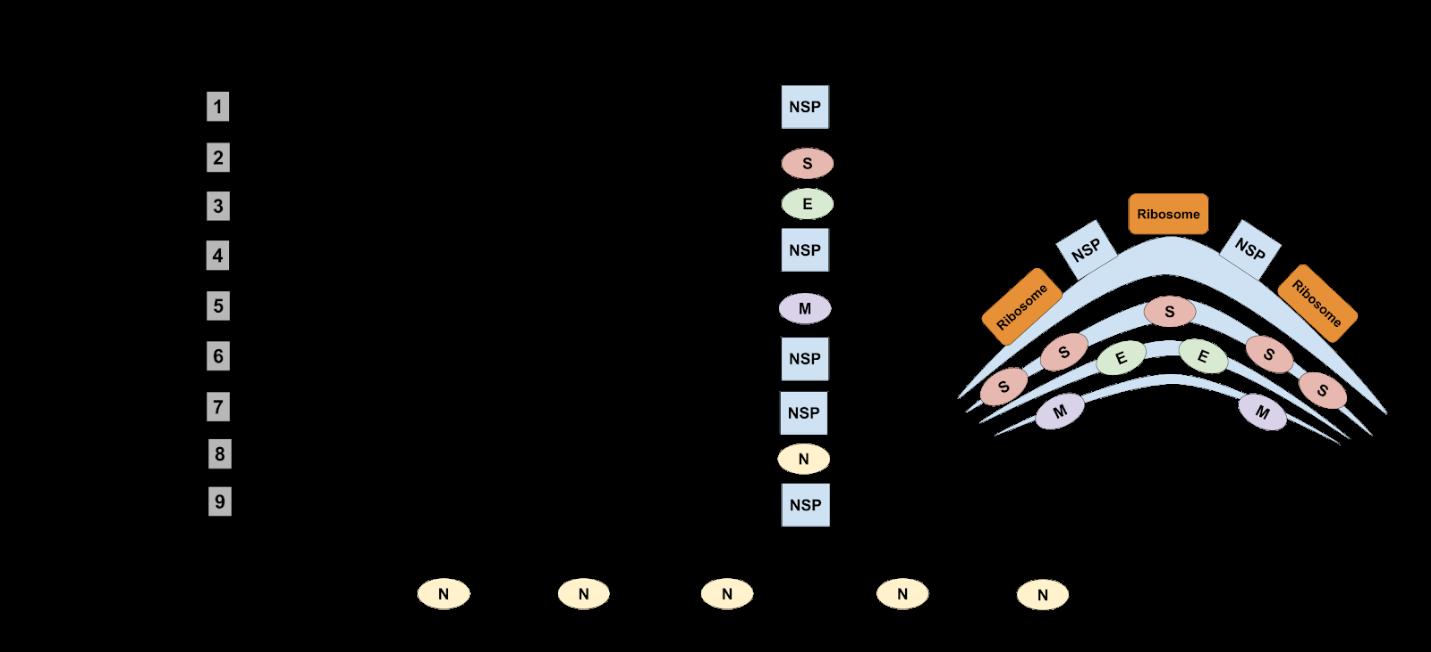
Inspiration derived from: (Sino Biological, 2006)
molecular process is discontinuous transcription using an enzyme called RNA polymerase that transcribes the negative genomic RNA strand to produce a template of subgenomic mRNA strands (Wit et al., 2016, Smirnov et al., 2018). The negative genomic RNA strand consists of a leader transcription regulatory sequence (TRS), and around nine body transcription regulatory sequences (TRSs) all with very small distances from each other to make up a specific portion near the 5’ end of the RNA strand (Marle et al., 1999). RNA polymerase then transcribes from the 5’ end until it arrives at the first body TRS (Sawicki et al., 2003). RNA polymerase stops here and instantly jumps to the leader TRS located at the 3’ end (Komissarova et al., 1996). The segment of the RNA that was jumped over from is not included in the mRNA transcript (Wit et al., 2016). Once transcribed, the small mRNA strand is sent to a template right below the negative genomic RNA strand, in the cytosol (Komissarova et al., 1996, Sawicki et al., 2003). The RNA polymerase then transcribes the RNA again from the 5’ end, until it arrives at the second body TRS which is located at a further position than the first TRS (Marle et al., 1999, Sawicki et al., 2003). RNA polymerase will stop here and again, jump to the leader TRS where it will perform transcription, and produce another mRNA strand that will be sent to the template (Komissarova et al., 1996). The process is identical to the first body TRS; however, since the distance of the second body TRS is farther than the first body TRS, RNA polymerase will transcribe more RNA to arrive at the second body TRS. This makes the second mRNA strand produced slightly bigger than the first. The same process will be used for the next several body TRSs successively with each mRNA transcript coming out slightly longer than the previous body TRS that was transcribed. Discontinuous transcription will lead to the production of a template of nine total subgenomic mRNAs which gradually increase in size and exert their functions in the next phase of viral biosynthesis (Sino Biological, 2006).
Virus Assembly:
Assembly of the coronavirus occurs largely in the endoplasmic reticulum-golgi intermediate component (ERGIC) as follows: (Fehr et al., 2015, Lodish, 2020).
M protein: The M protein is the commander of virus assembly in the ERGIC (Siu et al., 2008). To begin, the M protein will bind the E protein to form artificial microproteins which maintain the bond with the E protein, and ultimately organize, mature, and produce the viral envelope of the new coronavirus (Siu et al., 2008, Lim et al., 2016). With the viral envelope now produced, there is an opening for the extracellular viral proteins to attach and initiate their positioning on the viral envelope. Next, the M protein binds the S protein to configure the proper locations and amount of S proteins that will be placed on the viral membrane of the new coronavirus (de Haan et al., 2005, Fehr et
al., 2015). If there is an oversupply of S proteins, then the M proteins will release the extra into the cytosol which lysosomes will digest.
E protein: The E protein manipulates the plasma membrane to produce membrane curvature and ensure the coronavirus is a stable sphereshaped virus (Raamsman et al., 2000, Fehr et al., 2015). The membrane curvature is particularly valuable as it provides the mobility and transportability it requires to travel through the body. The E protein also has a function of thoroughly evaluating the M protein to ensure it is accurately completing its functions and progressing the assembly process (Boscarino et al., 2008).
S protein: The S protein, while extremely active in fusion, has a very limited role in the assembly of the coronavirus, only functioning as a trafficking and regulating agent in the ERGIC (Fehr et al., 2015).
N protein: The N protein progresses viral-like proteins to the ERGIC which then promotes a more stable viral envelopment when it binds with the other viral proteins to stabilize the newly constructed virus (Lim et al., 2016). The N protein is responsible for placing the genomic RNA strand in the accurate position within the new coronavirus, which is the final step in viral assembly (Krijnse-Locker et al., 1994).
Altogether, the four viral proteins complete all their functions which slowly, but accurately, completes the assembly of the coronavirus. However, there are still a series of steps the coronavirus goes through to ensure that it can leave the human cell accurately. For instance, the golgi apparatus scans and packages the newly produced coronavirus to place it into golgi vesicles (Sino Biological, 2006, Antibodies Online, 2020). The golgi vesicles act as transporters of the coronavirus from the golgi apparatus to the plasma membrane of the human cell. When the vesicle arrives at the plasma membrane, it will be released into the extracellular fluid, a process known as exocytosis (Antibodies Online, 2020). Exocytosis completes the process of coronavirus replication, and results in another virulent particle that can now travel to other organ systems and continue hijacking cells to repeat this process.
Overall, it may be said that the coronavirus is novel, which is valid; although, the biological forethought and resistance that the coronavirus wields is a representation that the coronavirus is a truly unparalleled biological virus that can gain immediate imperious control all through the entirety of an organism.
References
Anderson, E. L., Turnham, P., Griffin, J., & Clarke, C. (2020, May 1). Consideration of the Aerosol Transmission for COVID-19 and Public Health. PubMed. https://pubmed.ncbi.nlm.nih. gov/32356927/
Antibodies Online. (2020, March 31). SARS-CoV-2 Life Cycle: Stages and Inhibition Targets. https://www.antibodies-online. com/resources/18/5410/sars-cov-2-life-cycle-stages-andinhibition-targets/
Atkinson, J. (2009). Respiratory droplets - Natural Ventilation for Infection Control in Health-Care Settings - NCBI Bookshelf. National Institute of Health (NIH). https://www.ncbi.nlm.nih. gov/books/NBK143281/
Boopathi, S., Poma, A., & Kolandaivel, P. (2020, April 30). Novel 2019 coronavirus structure, mechanism of action, antiviral drug promises and rule out against its treatment. Taylor & Francis. https://www.tandfonline.com/doi/full/10.1080/0739 1102.2020.1758788
Boscarino, J. A., Logan, H. L., Lacny, J. J., & Gallagher, T. M. (2008, January 9). Envelope Protein Palmitoylations Are Crucial for Murine Coronavirus Assembly. PubMed Central (PMC). https://www.ncbi.nlm.nih.gov/pmc/articles/ PMC2258982/
Burkard, C. (2014, November 6). Coronavirus Entry Occurs through the Endo-/Lysosomal Pathway in a ProteolysisDependent Manner. PLOS Pathogens. https://journals.plos. org/plospathogens/article?id=10.1371/journal.ppat.1004502
Clarke, N. & Turner, A. (2011, November 10). AngiotensinConverting Enzyme 2: The First Decade. PubMed Central (PMC). https://www.ncbi.nlm.nih.gov/pmc/articles/ PMC3216391/
Cyagen Newsletter. (2020, April 1). What Roles Does ACE2 Play? | ACE2 Mice | Cyagen US Inc. Cyagen. https://www. cyagen.com/us/en/community/technical-bulletin/ace2.html
de Haan, C. A. M., & Rottier, P. J. M. (2005, August 31). Molecular Interactions in the Assembly of Coronaviruses. PubMed Central (PMC). https://www.ncbi.nlm.nih.gov/pmc/ articles/PMC7112327/
Di Giorgio, S., Martignano, F., Torcia, M. G., Mattiuz, G., & Conticello, S. G. (2020). Evidence for host-dependent RNA editing in the transcriptome of SARS-CoV-2 in humans. ScienceAdvances. https://advances.sciencemag.org/content/ advances/early/2020/05/15/sciadv.abb5813.full.pdf
Fehr, A., & Perlman, S. (2015, February 12). Coronaviruses: An Overview of Their Replication and Pathogenesis. PubMed Central (PMC). https://www.ncbi.nlm.nih.gov/pmc/articles/ PMC4369385/
Ghose, T. (2020, April 7). How are people being infected with COVID-19? Live Science. https://www.livescience.com/howcovid-19-spreads-transmission-routes.html
Hammer, S. M. (2020) Viral Replication. Columbia Viral Replication. http://www.columbia.edu/itc/hs/medical/
Hamming, I., & Timens, W. (2004, June). Tissue distribution of ACE2 protein, the functional receptor for SARS coronavirus. A first step in understanding SARS pathogenesis. PubMed. https://pubmed.ncbi.nlm.nih.gov/15141377/
Han, D., Penn-Nicholson, A., & Cho, M. (2006, February 28). Identification of critical determinants on ACE2 for SARS-CoV entry and development of a potent entry inhibitor. PubMed Central (PMC). https://www.ncbi.nlm.nih.gov/pmc/articles/ PMC7111894/
Haywood, A. M. (2010, November 1). Membrane Uncoating of Intact Enveloped Viruses. Journal of Virology. https://jvi.asm. org/content/84/21/10946
Hiremath, S. (2020, March 14). Should ACE-inhibitors and ARBs be stopped with COVID-19? NephJC. http://www.nephjc.com/ news/covidace2
Imai, Y. (2005, July 7). Angiotensin-converting enzyme 2 protects from severe acute lung failure. Nature. https:// www.nature.com/articles/nature03712?error=cookies_not_ supported&code=89ced488-272f-47f4-ac2b-24e8bf2b5e94
Jia, H. P., Look, D., & Tan, P. (2009, May 1). Ectodomain shedding of angiotensin converting enzyme 2 in human airway epithelia. PubMed Central (PMC). https://www.ncbi.nlm.nih.gov/pmc/ articles/PMC2711803/
Kabbani, N., & Olds, J. (2020, March 27). Does COVID19 Infect the Brain? If So, Smokers Might Be at a Higher Risk. Molecular Pharmacology. http://molpharm.aspetjournals.org/content/ molpharm/97/5/351.full.pdf
Komissarova, N., & Kashlev, M. (1996, December 26). RNA Polymerase Switches between Inactivated and Activated States By Translocating Back and Forth along the DNA and the RNA*. Journal of Biological Chemistry. https://www.jbc.org/ content/272/24/15329.full.pdf
Krijnse-Locker, J., Ericcson, M., Rottier, P., & Griffiths, G. (1994, January 1). Characterization of the budding compartment of mouse hepatitis virus: evidence that transport from the RER to the Golgi complex requires only one vesicular transport step | Journal of Cell Biology | Rockefeller University Press. Rockefeller University Press. https://rupress.org/jcb/ article/124/1/55/56282/Characterization-of-the-buddingcompartment-of
Kuba, K. (2005, July 10). A crucial role of angiotensin converting enzyme 2 (ACE2) in SARS coronavirus–induced lung injury. Nature Medicine. https://www.nature.com/articles/ nm1267?error=cookies_not_supported&code=7ab7aa6e385f-417c-a2af-11908b669599
Kuba, K. (2013, January 18). Multiple functions of angiotensinconverting enzyme 2 and its relevance in cardiovascular diseases. PubMed. https://pubmed.ncbi.nlm.nih. gov/23328447/
Lee, J. (2020, April 1). How the SARS-CoV-2 Coronavirus Enters Host Cells and How To Block It. Promega Connections. https:// www.promegaconnections.com/how-the-coronavirus-entershost-cells-and-how-to-block-it/
Li, F. (2016, September 29). Structure, Function, and Evolution of Coronavirus Spike Proteins. PubMed Central (PMC). https:// www.ncbi.nlm.nih.gov/pmc/articles/PMC5457962/ Lim, Y. X., Ng, Y. L., & Tam, J. P. (2016, July 25). Human Coronaviruses: A Review of Virus–Host Interactions. PubMed Central (PMC). https://www.ncbi.nlm.nih.gov/pmc/articles/ PMC5456285/
Lodish, H. (2020, August). Overview of the Secretory Pathway - Molecular Cell Biology - NCBI Bookshelf. National Institute of Health (NIH). https://www.ncbi.nlm.nih.gov/books/ NBK21471/
Marle, G., Dobbe, J., Gultyaev, A., Luytjes, W., Spaan, W., & Snijder, E. (1999, October 12). Arterivirus discontinuous mRNA transcription is guided by base pairing between sense and antisense transcription-regulating sequences. PNAS. https:// www.pnas.org/content/96/21/12056.figures-only
National Heart, Lung, and Blood Institute. (2020). Respiratory Failure | NHLBI, NIH. National Institute of Health. https://www. nhlbi.nih.gov/health-topics/respiratory-failure
Nature. (2020, August 21). Cell density-dependent proteolysis by HtrA1 induces translocation of zyxin to the nucleus and increased cell survival. https://www.nature.com/subjects/ proteolysis?error=cookies_not_supported&code=867889c6b015-41ae-a853-b94272da3f45
Patel, N. (2020, April 15). How does the coronavirus work? MIT Technology Review. https://www.technologyreview. com/2020/04/15/999476/explainer-how-does-thecoronavirus-work/
Plant, E., & Dinman, J. (2008, May 1). The role of programmed-1 ribosomal frameshifting in coronavirus propagation. PubMed Central (PMC). https://www.ncbi.nlm. nih.gov/pmc/articles/PMC2435135/
Povlsen, A. L., Grimm, D., Wehland, M., Infranger, M., & Kruger, M. (2020, January 18). The Vasoactive Mas Receptor in Essential Hypertension. MDPI. https://www.mdpi.com/20770383/9/1/267/htm
Raamsman, M. J. B., Locker, J. K., de Hooge, A., de Vries, A. A. F., Griffiths, G., Vennema, H., & Rottier, P. J. M. (2000, March 1). Characterization of the Coronavirus Mouse Hepatitis Virus Strain A59 Small Membrane Protein E. PubMed Central (PMC). https://www.ncbi.nlm.nih.gov/pmc/articles/PMC111715/
R&D Systems, a biotech brand. (2015). ACE-2: The Receptor for SARS-CoV-2. https://www.rndsystems.com/resources/ articles/ace-2-sars-receptor-identified
Sawicki, S., Sawicki, D., & Siddell, S. (2003, August 23). A Contemporary View of Coronavirus Transcription. PubMed Central (PMC). https://www.ncbi.nlm.nih.gov/pmc/articles/ PMC1797243/
Schelle, B., Karl, N., Ludewig, B., Siddell, S., & Thiel, V. (2005, June 1). Selective Replication of Coronavirus Genomes That Express Nucleocapsid Protein. PubMed Central (PMC). https:// www.ncbi.nlm.nih.gov/pmc/articles/PMC1112145/
Shang, J. (2020, March 30). Structural basis of receptor recognition by SARS-CoV-2. Nature. https://www.nature. com/articles/s41586-020-2179-y?error=cookies_not_ supported&code=c040882d-a7b9-4224-b9b5-2b2ca6ff8da8
Shereen, M., Khan, S., Kazmi, A., Bashir, N., & Siddique, R. (2020, July 1). COVID-19 infection: Origin, transmission, and characteristics of human coronaviruses. ScienceDirect. https://www.sciencedirect.com/science/article/pii/ S2090123220300540
Sigma Aldrich. (2020). Coronavirus (SARS-CoV-2) Viral Proteins. https://www.sigmaaldrich.com/technicaldocuments/protocols/biology/ncov-coronavirus-proteins. html
Sino Biological, Biological Solution Specialist. (2006). Coronavirus Replication. Sino Biological. https://www. sinobiological.com/research/virus/coronavirus-replication
Siu, Y. L., Teoh, K. T., Lo, J., Chan, C. M., Kien, F., Escriou, N., Tsao, S. W., Nicholls, J. M., Altmeyer, R., Pieris, J. S. M., Bruzzone, R., & Nal, B. (2008, November 15). The M, E, and N Structural Proteins of the Severe Acute Respiratory Syndrome Coronavirus Are Required for Efficient Assembly, Trafficking, and Release of Virus-Like Particles. Journal of Virology. https:// jvi.asm.org/content/82/22/11318
Smirnov, E., Hornacek, M., Vacik, T., Cmarko, D., & Raska, I. (2018, January 30). Discontinuous transcription. PubMed Central (PMC). https://www.ncbi.nlm.nih.gov/pmc/articles/ PMC5973254/
Sriram, K., Insel, P., & Loomda, R. (2020, May 14). What is the ACE2 receptor, how is it connected to coronavirus and why might it be key to treating COVID-19? The experts explain. The Conversation. https://theconversation.com/what-isthe-ace2-receptor-how-is-it-connected-to-coronavirusand-why-might-it-be-key-to-treating-covid-19-the-expertsexplain-136928
Turner, A. (2015, January 1). ACE2 Cell Biology, Regulation, and Physiological Functions. ScienceDirect. https://www.sciencedirect.com/science/article/pii/ B9780128013649000250?via%3Dihub
Turner, A. J. (2020, April). ACEH/ACE2 is a novel mammalian metallocarboxypeptidase and a homologue of angiotensinconverting enzyme insensitive to ACE inhibitors. PubMed. https://pubmed.ncbi.nlm.nih.gov/12025971/
UniProt. (2020). ACE2 - Angiotensin-converting enzyme 2 precursor - Homo sapiens (Human) - ACE2 gene & protein. https://www.uniprot.org/uniprot/Q9BYF1
Verdecchi, P., Cavallini, C., Spanevello, A., & Angeli, F. (2020). The pivotal link between ACE2 deficiency and SARS-CoV-2 infection. European Journal of Internal Medicine. https://www.ejinme.com/action/showPdf?pii =S0953-6205%2820%2930151-5
Viral Replication | Boundless Microbiology. (2020) Lumen Learning. https://courses.lumenlearning.com/boundlessmicrobiology/chapter/viral-replication/
Warner, F. J. (2004, November). Angiotensin-converting enzyme-2: a molecular and cellular perspective. PubMed. https://pubmed.ncbi.nlm.nih.gov/15549171/
Wessner, D. (2010). Origin of Viruses | Learn Science at Scitable. Nature. https://www.nature.com/scitable/ topicpage/the-origins-of-viruses-14398218/?error=cookies_ not_supported&code=996434bb-dc5a-49f8-b0c65f250616315b
Wit, E., van Doremalen, N., Falzarano, D., & Munster, V. (2016, June 27). SARS and MERS: recent insights into emerging coronaviruses. Nature Reviews Microbiology. https://www. nature.com/articles/nrmicro.2016.81?error=cookies_ not_supported&code=f8f66712-0405-4bc7-908e58cb5d96d03f#Sec2 Xu, J., Fan, J., & Wu, F. (2017, May 8). The ACE2/ Angiotensin-(1–7)/Mas Receptor Axis: Pleiotropic Roles in Cancer. Frontiers. https://www.frontiersin.org/ articles/10.3389/fphys.2017.00276/full
Zeng, Q., Langereis, M., van Vliet, A. L. W., Huizinga, E., & de Groot, R. J. (2008, July 1). Structure of coronavirus hemagglutinin-esterase offers insight into corona and influenza virus evolution. PubMed Central (PMC). https:// www.ncbi.nlm.nih.gov/pmc/articles/PMC2449365/
Zhang, H. (2020, March 3). Angiotensin-converting enzyme 2 (ACE2) as a SARS-CoV-2 receptor: molecular mechanisms and potential therapeutic target. Intensive Care Medicine. https://link.springer.com/article/10.1007/s00134-020-059859?error=cookies_not_supported&code=d9b9c760-3c954b58-a03f-668954481245
Ziebuhr, J., & Siddell, S. (1999, January). Processing of the Human Coronavirus 229E Replicase Polyproteins by the VirusEncoded 3C-Like Proteinase: Identification of Proteolytic Products and Cleavage Sites Common to pp1a and pp1ab. PubMed Central (PMC). https://www.ncbi.nlm.nih.gov/pmc/ articles/PMC103821/