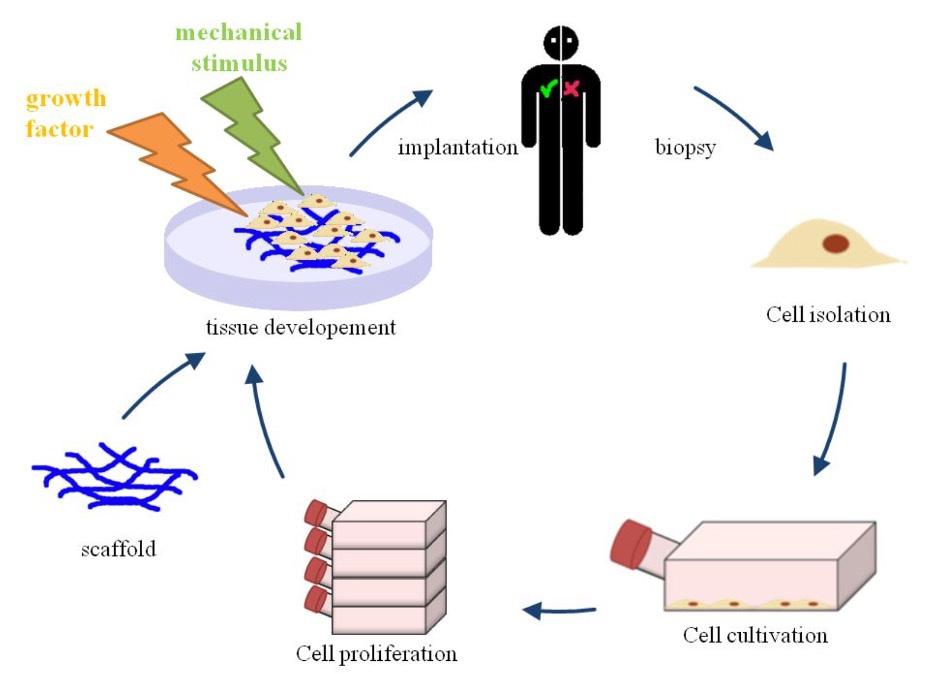
22 minute read
Bibbidi Bobbidy Bone: Exploring the Magic Behind Bone Tissue Engineering Amritha Anup '23
Bibbidi Bobbidy Bone: Exploring the Magic Behind Bone Tissue Engineering
BY AMRITHA ANUP '23
Advertisement
Cover Image: Flowchart depicting foundational steps making up the tissue engineering model. First, a biopsy is used to collect cells and tissue with regenerative potential. This is then cultivated and grown outside of the body under optimal conditions. A scaffold that is compatible with the body is developed. The collected tissue and cells are then integrated with the scaffold that can be stimulated externally to promote organized growth. This is then implanted into the patient Image Source: Wikimedia Commons Musculoskeletal disorders are conditions that impair locomotive abilities. Many factors cause these disorders, including repetitive motions and unnatural body positions exacerbated by the modern-day workplace (Malik et al., 2018). They are estimated to affect every 1 in 2 Americans, with osteoporosis and osteoarthritis becoming more widespread (Briggs et al., 2018). Osteoarthritis is the most common form of arthritis in the U.S. (Neogi, 2013). It involves degeneration of the cartilage that lubricates joints in areas such as the hips, knees, and fingers [Figure 1A]. Another condition, rheumatoid arthritis [Figure 1A], results from an autoimmune disease in which the immune system attacks the joints. The outcome of both conditions is joint syndromes. Osteoporosis causes flash, or unannounced, fractures that are especially common in older women [Figure 1B]. Osteoporosis is estimated to cause 2 million bone fractures per year in the U.S. (Morrell et al., 2021).
The chronic forms of these conditions do not have cures; treatments only work for some people, and they provide only short-term relief. Surgeries, such as joint replacement and bone grafting procedures, come with concerns of immune rejection, are not widely available, and have a high risk of infection due to potential pathogen transfer (Seong et al., 2010; Henkel et al., 2013). Bone tissue engineering introduces a personalized approach to treating these conditions and aims to replicate and stimulate the body’s healing process through the introduction of biocompatible materials. These are materials that mimic or come from living tissues and they do not cause undesirable effects (Kowalczuk, 2020). These cells are collected from the patient and grown in an organized manner outside of the body using a scaffold [Figure 2]. A scaffold is critical in supporting the transformation from a twodimensional layer of cells to a three-dimensional tissue (Swenson, 2013). They are typically made of a biocompatible material, or a material that is safe to put into the body, such as the polyester scaffold poly(ε-caprolactone), or PCL (Dwivedi et al., 2020). This tissue is then implanted into the patient. The final tissue product requires the integration of three components (Quarto & Giannoni, 2016): 1. an osteogenic or osteoinductive environment – this is an environment that supports bone formation and communication between
A
B
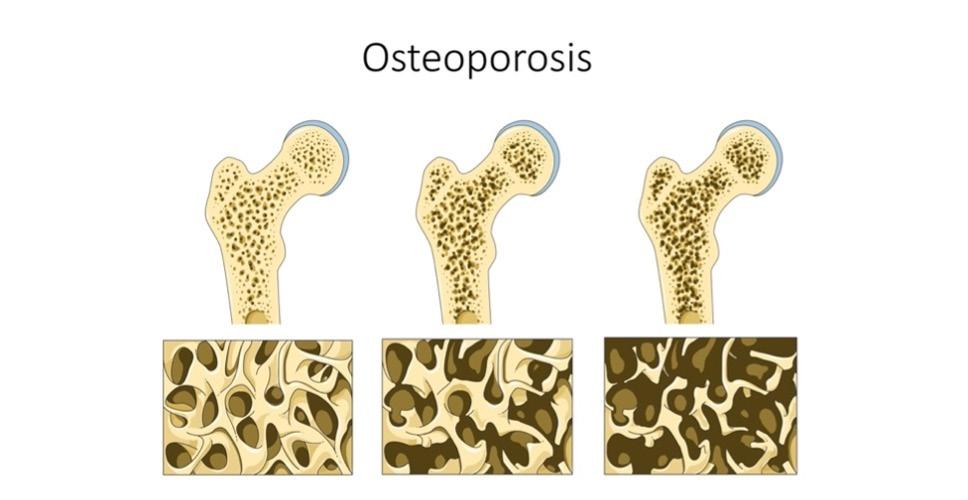
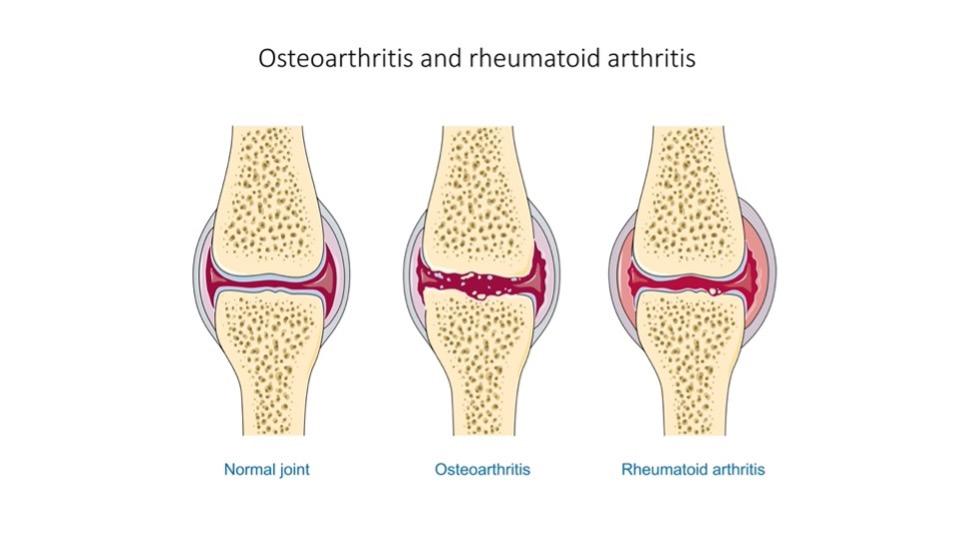
different cells (Albrektsson & Johansson, 2001); 2. growth factors, or molecules that can stimulate cell growth; 3. and a biomaterial matrix that supports osteoconduction (Albrektsson & Johansson, 2001), which means bone growth on the matrix surface through processes such as cellular attachment, migration, and proliferation.
Why Bone Tissue Engineering?
Bone is the second most transplanted material and about 3.5 million bone grafts are performed each year (Henkel et al., 2013). Bone, unlike most other types of tissue, can heal and regenerate itself to fix minor fractures and defects without forming fibrous scar tissue (Sheen & Garla, 2021). This process consists of the formation of a hematoma, or blood clot, followed by collagenous tissue formation. This collagenous growth will become more calcified over time, and the bone undergoes a process called remodeling. Remodeling consists of a balance between removing damaged bone and forming the new bone, and the process can span years. Large bone defects, on the other hand, cannot be repaired by bones alone (Qu et al., 2019). Since bones have the potential to self-regenerate in some capacity, they are a great organ system to explore bone tissue engineering. The field aims to develop external scaffolds, harvest and apply cells, and use biologically active molecules to induce proper healing of the bone tissue when the damage is severe.
Bone is a connective tissue network made of a complex mixture of inorganic and organic materials such as hydroxyapatite and collagen proteins (Feng, 2009; Henkel et al., 2013). The skeleton is a dynamic system that provides structural integrity, protection, and acts as a chemical reservoir. These characteristics are made possible by the structural organization and mechanical properties of bone. The outer surface of bone is made of a hard shell called compact, or cortical bone [Figure 3]. Making up the insides of the shell is spongy, trabecular bone, which forms a network through the core of the bone that consists of bone marrow. Bone is known to be mechanoreceptive, which means it adapts to applied mechanical stimuli (Qu et al., 2019). The exterior cortical layer and porous trabecular bone adapt or remodel in response to mechanical stresses and strains (Manzini et al., 2021) by the actions of highly specialized cells.
Remodeling, or coupled bone removal and formation, begins with the activation of
Figure 1: (A) Progression of osteoporosis and its effect on bones. As osteoporosis becomes more severe, excessive bone loss and/or reduced bone growth causes the bone microstructure to become more brittle. This results in bones that are prone to fracturing under stress (Image Source: Wikimedia Commons). (B) How osteoarthritis and rheumatoid arthritis affect the joint. A healthy joint consists of intact cartilage (shown in blue). Osteoarthritis develops when the cartilage at the joint becomes damaged due to mechanical wear. Rheumatoid arthritis is characterized by joint inflammation caused by an overactive immune system that attacks the joints Image Source: Wikimedia Commons
Figure 2: Here, the crisscross patterns are made of polycaprolactone or poly(εcaprolactone), also known as PCL, which is a material that can be used for bone tissue engineered scaffolds. It was introduced as a reference material by the National Institute of Standards and Technology (NISD) in 2013. The fluorescent regions on the image mark cells that have proliferated. Image Source: Wikimedia Commons
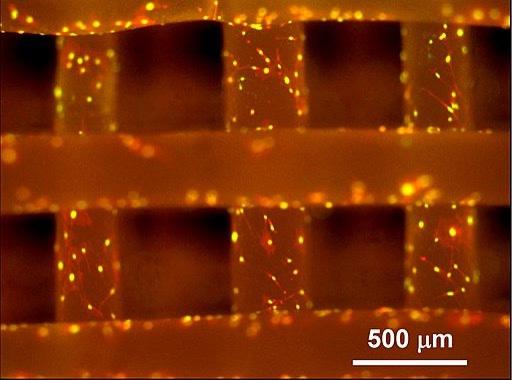
osteoclasts, which are cells that resorb or dissolve bone. Once osteoclasts undergo apoptosis, osteoblasts begin forming new bones (Langdahl et al., 2016). Osteocytes are star-shaped cells derived from osteoblasts, and they work with osteoclasts to regulate bone repair (Creecy et al., 2021). Resorption and formation must be balanced for bone structures to retain their quality and function. When bone experiences a small injury, such as a hairline fracture, the bone can heal on its own. For larger injuries, or if the patient has a condition that prevents the natural bone regenerative process, bone cannot fix itself (Dimitriou et al., 2011). While attempting the healing process, the injured region succumbs to issues such as insufficient blood supply due to vascular damage and healing cannot be completed.
A Snapshot of the Rich History of Bone Tissue Engineering and Regeneration
The idea of bone regeneration is not new, and the field of bone tissue engineering has a rich history. In Peru, a skull from 2000 BC was discovered with a 1 mm thick plate of gold masking a bone defect that was likely caused by trepanation, which involves drilling a hole into the skull to relieve symptoms of neurological conditions (Henkel et al., 2013). During 600 BC, ancient Egyptians developed skills used in orthopedic surgeries as mummies have been found to have iron prostheses implanted for knee joint replacement (Henkel et al., 2013). Around the same period, the Indian surgeon Susruta wrote an account of skin graft techniques for reconstructing the nose using skin from the forehead, and this account was included in one of the first textbooks on surgery (Willyard, 2016). In 1668, Dutch surgeon Job Janszoon van Meerkenen performed the first bone xenograft. A xenograft is a piece of tissue that originates from a species different from the recipient. Janszoon van Meerkenen repaired a skull defect using a bone xenograft from a deceased dog and the graft was successfully incorporated into the patient. In the 1800s, Plaster of Paris, also known as calcium sulfate, was used to fill bone cavities.
Philips von Walters performed the first bone autograft in 1820 using tibial bone wedges from three donors to reconstruct the humerus bone in a 3-year-old affected by rickets, which is a debilitating musculoskeletal disorder that causes weak bones due to reduced calcium or phosphate levels in the blood. These reduced levels can contribute to delayed bone mineralization (Carpenter et al., 2017). During the later years of the 19th century, Louis Léopold Ollier coined the term ‘bone graft’, and he along with German surgeon Arthur Barth contributed to the foundation of current bone grafting procedures.
In 1986, the Masquelet technique for bone reconstruction was introduced. This technique leads to a particular immune system reaction that favors the healing process (Henkel et al., 2013). This process is commonly used for treating long bone defects that are greater than 5 cm in length (Bosemark et al., 2015; Sivakumar et al., 2016). First the damaged bone tissue is removed. Then the defect site is filled with a polymethylmethacrylate (PMMA) plastic spacer. The spacer triggers a ‘foreign body reaction’ and the formation of a thick membrane. This membrane has desirable properties for bone regeneration (Henkel et al., 2013), such as rich vascularization and the presence of growth factors. Once the soft tissue in the damaged area has regenerated, the PMMA spacer can be removed. A bone graft, more specifically an autograft, is inserted into the cavity and the body’s healing process begins expanding on the graft.
Current bone regeneration treatments fall into one of two main categories: autografts and allografts. These types of grafts provide mechanical support and a source of regenerative cells (Goldberg & Stevenson, 1987). For bone defects that are smaller than 5 cm, an autograft is commonly used (Bosemark et al., 2015). An autograft procedure is a two-surgery process. First, the patient’s tissue is surgically transferred from one part of a patient’s body to another part of the patient’s body. Bone cells are commonly harvested from the iliac crest, located on the pelvis, because the region has a high amount of bone (Robinson et al., 2018), and the cancellous bone in this region contains many osteoblasts, or bone forming cells. These cells are then implanted at the site of bone injury. Autografts are the gold standard of currently available bone
tissue engineering techniques because it is nonimmunogenic. This means the patient’s immune system is unlikely to reject the implant since the graft tissue is not foreign to the patient (Amini et al., 2012). However, the additional surgery to extract graft material for the treatment takes an added toll on the patient’s body, and the amount of available bone graft varies per patient. There is an 8.6% chance patients will develop serious conditions during or due to the graft collection procedure. One example of a condition is a hematoma (Baldwin et al., 2019), which occurs when there is a buildup of blood caused by ruptured blood vessels.
Allografts, on the other hand, involve taking tissue from another person’s body and transferring this to the patient implantation site. They account for about one-third of all bone grafts in the U.S. (Baldwin et al., 2019). Allografts are an optimal solution for patients who cannot undergo a second surgery or those who do not have enough graft tissue in their bodies. The main concern with this procedure is reduced efficacy due to storage and sanitation limitations. The grafts are stored by freeze drying, which is a process that removes all water from the tissue. This method makes the graft mechanically weaker and can destroy cells (Baldwin et al., 2019). In addition to autografts and allografts, another bone regeneration treatment is xenografts. These are grafts derived from animal tissue. The major disadvantages of xenografts are that they will likely cause graft rejection similar to allografts, however, consequences may be more drastic with xenografts because the graft comes from another species. These also have the risk of transmitting zoonotic diseases (Oryan et al., 2014) to both the patient and the wider human population (Fishman et al., 2012). The risk of infection depends on the graft species, amount of tissue grafted, and the patient immune system. A benefit of xenografts is that they may support a timely procedure, especially when allografts are not available.
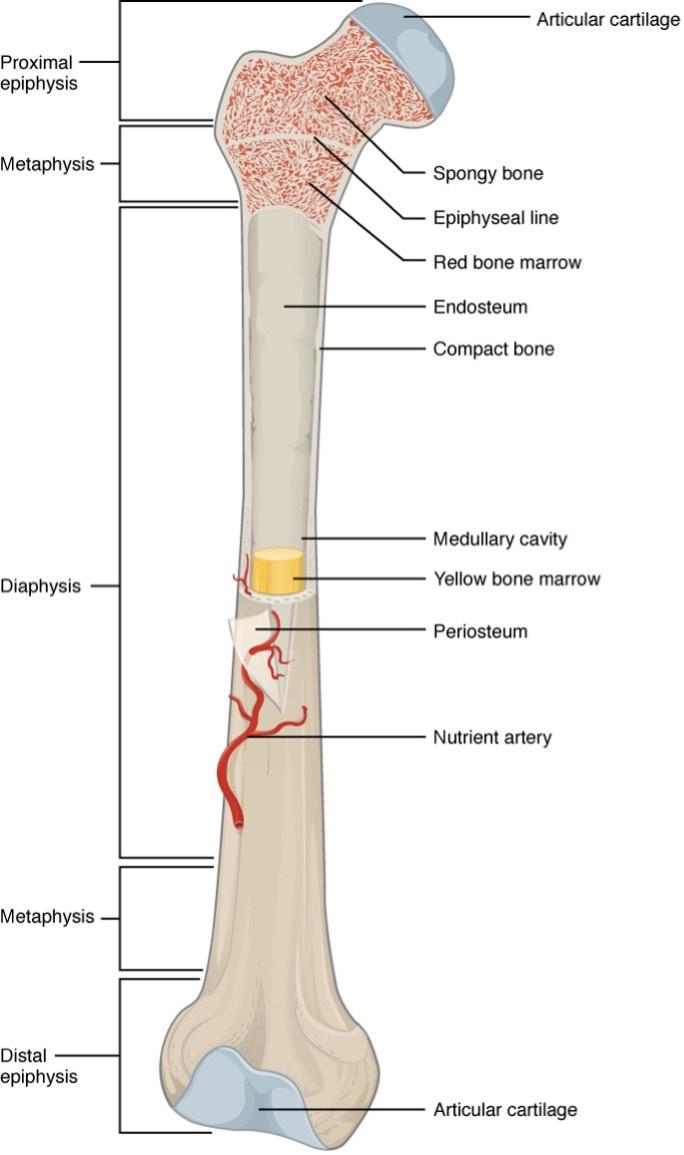
Tissue Engineering is Filling Voids in Current Bone Regeneration Treatments
Tissue engineering combines the use of engineered scaffolds, growth factors, and cells from the patient to bolster the body’s natural healing process. Scaffolds or artificial extracellular matrices are foundational in tissue engineering. They are responsible for supporting a system that promotes proliferation, differentiation, and growth of cells. They must be biocompatible, or not cause ill effects to the body, to ensure safe implantation into the host as well as porous to support the spreading of cells and development of a vascular network and made in a geometry that allows cells to interact with each other. These scaffolds are temporary (Abbasi et al., 2020); the scaffold degradation rate must be fast enough to make room for newly growing tissue, though slow enough to provide enough support during the tissue regeneration process.
The process of engineering scaffolds requires significant biomaterial and architectural design. The development of biomaterials has evolved in three generations in the last 30 years. A goal of the first generation of biomaterials was to have them closely match the host’s body to reduce the chances of triggering an immune system response. Examples of biomaterials include titanium metal, synthetic polymers such as (PMMA), and ceramics (Henkel et al., 2013). Second generation materials are more biocompatible, and they positively interacted with the host environment. For example, these bioactive materials can degrade in vivo, or in the patient’s body. Third generation materials are more powerful, and they can stimulate the treatment site in response to biological factors and external stimuli.
One of the challenges facing third generation, bioactive materials is that they are less
Figure 3: Long bone biology. The outer shell of bone is compact or cortical bone. The periosteum is a thin sheet that covers compact bone. Near the top and bottom ends of the bone, there is a large volume of cancellous or spongy bone. Integrated into this spongy matrix is red bone marrow. Near the middle of the bone, the core is made up of yellow bone marrow Image Source: Wikimedia Commons
Figure 4: The top left image shows the lower jaw which contains the ramus-condyle unit; highlighted in blue is the temporomandibular joint condyle. The lower left image shows a decellularized bone (DCB) and polycaprolactone (PCL) hybrid scaffold. Both images on the right are zoomed in images of the scaffold, with the top right image showing bone cells stained in red.
Image Source: NIH Image Gallery
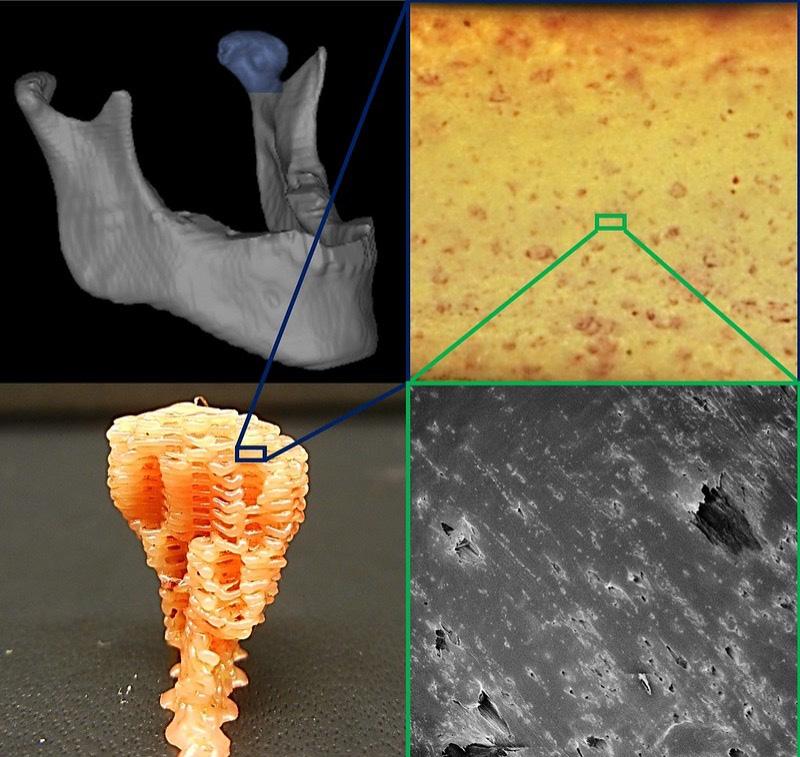
manufacturable than synthetics, such as those that fall under first generation materials (Hung et al., 2016). There has been limited success in incorporating growth factors into synthetics to make them more bioactive, but in 2016, a group of researchers at Johns Hopkins University combined decellularized bone (DCB) matrix particles with the synthetic polyester polycaprolactone (PCL) to make a manufacturable and bioactive alternative for bone tissue engineering applications [Figure 4]. DCB’s are a type of allogeneic source of osteoinductive and osteoconductive properties that work well for bone tissue engineering applications. The group then implanted these scaffolds into mouse models with defects on the calvaria or top part of the skull. It was observed that the hybrid DCB and PCL material grew about double the volume compared to the PCL only control.
After the scaffold has been designed, cells that are osteogenic, or capable of forming bone, are seeded into the matrix material (Henkel et al., 2013). In the past 20 years, significant progress has been made in gathering information about stem cells. Stem cells are unspecialized cells that have self-renewing capabilities (Seong et al., 2010), and they can be stimulated to specialize into osteogenic, bone forming cells. Therefore, stem cells can be used in bone tissue engineering. Osteoprogenitor stem cells can be collected from the periosteum (the thin outer layer of bone), bone marrow, spleen, or thymus. These cells are then isolated, cultured, and stimulated to develop into bone.
Modern Day Technical Applications
Over the past decade, the need for bone grafting has become more pronounced and this trend is continuing. With an aging Baby Boomer population, cases of joint diseases, back pain, osteoporotic fractures are rapidly increasing, and there is a significant demand for joint and bone restoring procedures (Baroli, 2009). Each year about 0.5 million bone grafts surgeries are performed in the United States (Baldwin et al., 2019). Bone tissue engineering research continues to evolve, and there have been several significant modern-day developments in the field. Two prominent examples of bone tissue engineering
epiBone is a biomedical engineering startup that originated from Columbia University and specializes in bone reconstruction. The epiBone technology was developed from tissue engineered grafts for facial bone reconstruction (Bhumiratana et al., 2016). Facial bones have complex geometries, so using standard auto or allografts to treat facial deformities come with significant comorbidities. The aim of work at epiBone is to engineer the ramus-condyle unit bone in the skull making up the jaws. This bone was engineered using stem cells and a scaffold, using the Yucatan minipig as a preclinical animal model. The novel workflow starts with a CT scan of the bone region that needs to be replicated. Then, a scaffold is prepared from bovine bone. The scaffold is shaped into the complex geometry of the facial bone using micromilling technology, which involves detailed milling of the scaffold. Milling is guided by computer tomography (CT) images of the pig’s jaw. Stem cells derived from adipose tissue of the minipigs were then collected and cultured within these scaffolds using a perfusion bioreactor. The bioreactor serves to provide an optimal environment for the growing tissue engineered graft. The system supports the exchange of nutrients and oxygen between the tissue and the surrounding environment. This work was the first to demonstrate the reconstruction of a large load bearing facial bone as well as the possibility of implanting the tissue engineered construct into the minipig animal model. epiBone is currently running Phase 1 and Phase 2 clinical trials that are expected to be completed in 2023 (Epibone, Inc., 2021).
Cerapedics is another bone tissue engineering company, and they have developed a new bone graft called i-FACTOR. i-FACTOR is made of a material that combines the inorganic bone mineral hydroxyapatite with P-15, a polypeptide that can attach to osteogenic cells to stimulate bone formation (Lauweryns & Raskin, 2015). It synergistically works with the body’s natural healing process to stimulate bone formation, and ensures bone formation is restricted only to the injury site since the polypeptide is anchored to the surface of the hydroxyapatite (Science, n.d.). Originally this product was developed as a dental application, but there are several clinical trials that are testing its use for treating other conditions such as those that affect the spine.
Challenges
Despite the advancements in bone tissue engineering, there are still no readily available clinical solutions for doctors to use to treat their patients. One reason for the lack of treatments is the increased number of variables in clinical settings. For example, in the emergency room, different patients can have varying health histories, causes of the incident, and affected locations on the body; bone tissue engineering treatment eligibility depends on all these factors. For companies like epiBone and Cerapedics, the road to obtaining FDA approval is a long, complex process that requires time to ensure medical products are safe. There are also challenges in designing optimal scaffolds that support bone tissue regeneration throughout the scaffold; this is an issue that is exacerbated by achieving less vascularization than desired (Amini et al., 2012). To circumvent this problem, scientists have been applying growth factors and anti-inflammatory agents to the scaffold to support a more seamless integration of the tissue engineered material to the patient’s body.
Despite the many developments in the field, no routine clinical applications exist and most surgeons still use more traditional treatments for musculoskeletal injuries such as autografts and allografts (Quarto & Giannoni, 2016). Time also limits the usefulness of these therapies. Cells need time to grow in an organized manner and communicate with the cells around them to elicit the appropriate body responses that allow for the successful integration of the implanted tissue with the host tissue.
Concluding Remarks
Bone tissue engineering holds potential in solving the shortcomings of current grafting procedures. It can be personalized or made highly compatible with the patient’s body with the combination of patient stem cells, thus reducing the probability of evoking an immunogenic response. It also avoids the problem of bone graft shortages. Future advancements in the field are necessary to bring these bone tissue engineered technologies to the clinical space.
References
Abbasi, N., Hamlet, S., Love, R. M., & Nguyen, N.-T. (2020). Porous scaffolds for bone regeneration. Journal of Science: Advanced Materials and Devices, 5(1), 1–9. https://doi.org/10.1016/j.jsamd.2020.01.007
Albrektsson, T., & Johansson, C. (2001). Osteoinduction, osteoconduction and osseointegration. European Spine Journal: Official Publication of the European Spine Society, the European Spinal Deformity Society, and the European Section of the Cervical Spine Research Society, 10 Suppl 2, S96-101. https://doi.org/10.1007/ s005860100282
Amini, A. R., Laurencin, C. T., & Nukavarapu, S. P. (2012). Bone Tissue Engineering: Recent Advances and Challenges. Critical Reviews in Biomedical Engineering, 40(5), 363.
Baldwin, P., Li, D. J., Auston, D. A., Mir, H. S., Yoon, R. S., & Koval, K. J. (2019). Autograft, Allograft, and Bone Graft Substitutes: Clinical Evidence and Indications for Use in the Setting of Orthopaedic Trauma Surgery. Journal of Orthopaedic Trauma, 33(4), 203–213. https://doi.org/10.1097/BOT.0000000000001420
Baroli, B. (2009). From natural bone grafts to tissue engineering therapeutics: Brainstorming on pharmaceutical formulative requirements and challenges. Journal of Pharmaceutical Sciences, 98(4), 1317–1375. https://doi.org/10.1002/jps.21528
Bhumiratana, S., Bernhard, J. C., Alfi, D. M., Yeager, K., Eton, R. E., Bova, J., Shah, F., Gimble, J. M., Lopez, M. J., Eisig, S. B., & Vunjak-Novakovic, G. (2016). Tissue-Engineered Autologous Grafts for Facial Bone Reconstruction. Science Translational Medicine, 8(343), 343ra83. https://doi.org/10.1126/scitranslmed.aad5904
Bosemark, P., Perdikouri, C., Pelkonen, M., Isaksson, H., & Tägil, M. (2015). The masquelet induced membrane technique with BMP and a synthetic scaffold can heal a rat femoral critical size defect. Journal of Orthopaedic Research, 33(4), 488–495. https://doi. org/10.1002/jor.22815
Briggs, A. M., Woolf, A. D., Dreinhöfer, K., Homb, N., Hoy, D. G., Kopansky-Giles, D., Åkesson, K., & March, L. (2018). Reducing the global burden of musculoskeletal conditions. Bulletin of the World Health Organization, 96(5), 366. https://doi.org/10.2471/BLT.17.204891
Creecy, A., Damrath, J. G., & Wallace, J. M. (2021). Control of Bone Matrix Properties by Osteocytes. Frontiers in Endocrinology, 0. https://doi. org/10.3389/fendo.2020.578477
Dimitriou, R., Jones, E., McGonagle, D., & Giannoudis, P. V. (2011). Bone regeneration: Current concepts and future directions. BMC Medicine, 9(1), 66. https://doi.org/10.1186/1741-7015-9-66
Dwivedi, R., Kumar, S., Pandey, R., Mahajan, A., Nandana, D., Katti, D. S., & Mehrotra, D. (2020). Polycaprolactone as biomaterial for bone scaffolds: Review of literature. Journal of Oral Biology and Craniofacial Research, 10(1), 381. https://doi.org/10.1016/j.jobcr.2019.10.003
Epibone, Inc. (2021). An Open-Label, First-in-Human, Single Intervention Study for Evaluation of EpiBone-CMF Engineered Living Bone Graft for Mandibular Ramus Reconstruction (Clinical Trial Registration No. NCT03678467). clinicaltrials.gov. https://clinicaltrials.gov/ ct2/show/NCT03678467
Fishman, J. A., Scobie, L., & Takeuchi, Y. (2012). Xenotransplantation-associated infectious risk: A WHO consultation. Xenotransplantation, 19(2), 72. https://doi.org/10.1111/j.1399-3089.2012.00693.x
Goldberg, V. M., & Stevenson, S. (1987). Natural history of autografts and allografts. Clinical Orthopaedics and Related Research, 225, 7–16.
Henkel, J., Woodruff, M. A., Epari, D. R., Steck, R., Glatt, V., Dickinson, I. C., Choong, P. F. M., Schuetz, M. A., & Hutmacher, D. W. (2013). Bone Regeneration Based on Tissue Engineering Conceptions—A 21st Century Perspective. Bone Research, 1(1), 216–248. https:// doi.org/10.4248/BR201303002
Hung, B. P., Naved, B. A., Nyberg, E. L., Dias, M., Holmes, C. A., Elisseeff, J. H., Dorafshar, A. H., & Grayson, W. L. (2016). Three-Dimensional Printing of Bone Extracellular Matrix for Craniofacial Regeneration. ACS Biomaterials Science & Engineering, 2(10), 1806. https://doi.org/10.1021/acsbiomaterials.6b00101
Kowalczuk, M. (2020). Intrinsically Biocompatible Polymer Systems. Polymers, 12(2). https://doi.org/10.3390/polym12020272
Lauweryns, P., & Raskin, Y. (2015). Prospective Analysis of a New Bone Graft in Lumbar Interbody Fusion: Results of a 2- Year Prospective Clinical and Radiological Study. International Journal of Spine Surgery, 9. https://doi.org/10.14444/2002
Malik, K. M., Beckerly, R., & Imani, F. (2018). Musculoskeletal Disorders a Universal Source of Pain and Disability Misunderstood and Mismanaged: A Critical Analysis Based on the U.S. Model of Care. Anesthesiology and Pain Medicine, 8(6). https://doi. org/10.5812/aapm.85532
Morrell, A. E., Robinson, S. T., Ke, H. Z., Holdsworth, G., & Guo, X. E. (2021). Osteocyte mechanosensing following short-term and long-term treatment with sclerostin antibody. Bone, 149, 115967. https:// doi.org/10.1016/j.bone.2021.115967
Neogi, T. (2013). The Epidemiology and Impact of Pain in Osteoarthritis. Osteoarthritis and Cartilage / OARS, Osteoarthritis Research Society, 21(9), 1145. https://doi.org/10.1016/j.joca.2013.03.018
Oryan, A., Alidadi, S., Moshiri, A., & Maffulli, N. (2014). Bone regenerative medicine: Classic options, novel strategies, and future directions. Journal of Orthopaedic Surgery and Research, 9(1), 18. https://doi. org/10.1186/1749-799X-9-18
Qu, H., Fu, H., Han, Z., & Sun, Y. (2019). Biomaterials for bone tissue engineering scaffolds: A review. RSC Advances, 9(45), 26252–26262. https:// doi.org/10.1039/C9RA05214C
Quarto, R., & Giannoni, P. (2016). Bone Tissue Engineering: Past–Present–Future. In M. Gnecchi (Ed.), Mesenchymal Stem Cells: Methods and Protocols (pp. 21–33). Springer. https://doi.org/10.1007/978-14939-3584-0_2
Robinson, B. T., Metcalfe, D., Cuff, A. V., Pidgeon, T. E., Hewitt, K. J., Gibbs, V. N., Rossiter, D. J., & Griffin, X. L. (2018). Surgical techniques for autologous bone harvesting from the iliac crest in adults. The Cochrane Database of Systematic Reviews, 2018(4). https://doi. org/10.1002/14651858.CD011783.pub2
Science. (n.d.). Cerapedics. Retrieved August 23, 2021, from https://cerapedics.com/science
Seong, J. M., Kim, B.-C., Park, J.-H., Kwon, I. K., Mantalaris, A., & Hwang, Y.-S. (2010). Stem cells in bone tissue engineering. Biomedical
Materials, 5(6), 062001. https://doi.org/10.1088/17486041/5/6/062001
Sheen, J. R., & Garla, V. V. (2021). Fracture Healing Overview. In StatPearls [Internet]. StatPearls Publishing. https://www.ncbi.nlm.nih.gov/books/ NBK551678/
Sivakumar, R., Mohideen, M. G., Chidambaram, M., Vinoth, T., Singhi, P. K., & Somashekar, V. (2016). Management of Large Bone Defects in Diaphyseal Fractures by Induced Membrane Formation by Masquelet’s Technique. Journal of Orthopaedic Case Reports, 6(3), 59. https://doi.org/10.13107/jocr.2250-0685.508
swenson. (2013, June 25). NIST Announces New Scaffold Reference Material for Tissue Engineering Research [Text]. NIST. https://www.nist.gov/newsevents/news/2013/06/nist-announces-new-scaffold-referencematerial-tissue-engineering-research
Willyard, C. (2016). Timeline: Regrowing the body. Nature, 540(7632), S50–S51. https://doi.org/10.1038/540S50a