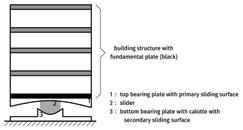
15 minute read
Passive Vibration Reduction by Curved Surface Slider Systems
Schwingungsreduktion durch den Einsatz passiver Systeme Passive Vibration Reduction by Curved Surface Slider Systems
by Christian Bucher
Advertisement
Schwingungen von Bauwerken durch äußere Anregungen wie Verkehr, Wind oder Erdbeben können häufig zu schweren Schäden führen, die entweder auf Überlastung oder auf Ermüdungseffekte zurückzuführen sind. Es ist daher wichtig, die Auswirkung solcher unvermeidbaren Vibrationen abzuschwächen. Eine weitverbreitete Möglichkeit ist der Einbau von passiven Systemen, die entweder die übertragenen Kräfte sofort reduzieren oder durch zusätzliche Dämpfung die Energie abführen. Natürlich sollte ein gutkonzipiertes System beide Prinzipien kombinieren. Passive Systeme wie Curved Surface Slider (CSS) oder Ähnliches haben sich im Zusammenhang mit Erdbebenlasten als sehr effektiv für den Schutz von Gebäuden erwiesen. Dieser Aufsatz konzentriert sich auf die Frage, ob solche Systeme für Brücken unter Erdbebenbelastung gleichermaßen wirksam sein können. Zu diesem Zweck wird ein veranschaulichendes Beispiele numerisch analysiert. Aus dieser Analyse werden einfache Entwurfsformeln abgeleitet und die Ergebnisse verglichen. Dies mit dem Ziel, ein einfaches Werkzeug zur Entscheidungsfindung bereitzustellen. 1 Introduction Curved surface sliders (CSS) are effective base isolators to reduce the effect of earthquake accelerations on civil structures. These isolators can be built in different configurations, i.e. with one (Single CSS) or two (Double CSS) or even multiple primary sliding surfaces (e.g. [1]). The secondary sliding surface of the calotte joint in case of Single CSS is lubricated to ensure high rotation capability of the calotte joint but must not be considered in the CSS design to produce damping ( Fig. 1). Common to all types of CSS is that their effective radius of curvature, which is the radius of an equivalent single pendulum, isolates the structure from the shaking ground by its low restoring stiffness and their damping, which is generated by friction on the primary sliding surfaces, augments the structural damping. [2] [3] In addition, the design of CSS must guarantee the minimum required re-centring of the primary structure which is achieved by appropriate designs of effective radius and friction. [4] In many papers dealing with CSS, the focus is on the effective seismic protec tion of structural and non-structural elements [3] [5] for which also the variation of axial loads on the isolators during the earthquake are considered. [6] In the studies [7] [8] also semi-active (tuneable) base isolation systems are considered in order to further enhance the structural isolation. Also, the residual displacement and re-centring error, respectively, of CSS have been investigated for different base isolators [9] and the method of sensitivity analysis is adopted in [3] [10] to identify the influence of the CSS design parameters on the resulting structural isolation performance. Earthquakes are considered to be highly random processes with non-deterministic magnitude and frequency contents which also applies to the Maximum Considered Earthquake (MCE). As an inherent consequence of this fact, the CSS displacement capacity requires to be computed in a probabilistic way. In this paper the characteristic displacement of the CSS is determined such that it is not exceeded with the probability of 99,99 %, which is a 0,9999 quantile, for the assumed MCE. Due to the high level of randomness it is reasonable to formally represent the loading as a non-stationary random process. In order to match the analysis with typical seismic design procedures the analysis focuses on earthquakes with site-specific average peak ground accelerations (PGA) which are selected here to be 2 and 5 m/s 2 . The solution approach in this paper, which is partially based on the earlier research results [11] [12] but further developed here, is as follows: – The space of all sensible variations of effective radius of curvature R eff and friction coefficient μ is scanned by a so-called Design of Experiments (DOE) which utilizes 50 randomly sampled values of these parameters. – For each of these combinations, 128 different realizations of earthquakes are digitally generated, and from the results the statistical properties of the responses in terms of peak support displacement and CSS displacement are computed. – Generally valid functions for these statistical parameters valid for arbitrary numerical values of the system parameters are generated using a meta-modeling technique. – Based on mean values and standard deviations, the 0.9999-quantiles of the peak CSS displacement are computed assuming an extreme value (Gumbel) distribution. – All the previous steps are carried out for two levels of the ground accelera tions, i.e. vor PGA values of 2 and 5 m/s 2 and simple engineering approximations are derived.
2 Principles of CSS and mechanical model The curved slide surface protection system (CSS, an example is shown in Fig. 1) allows horizontal relative displacement between the top and bottom plates. This effectively reduces the magnitude of the horizontal forces transmitted and thus protects the structural elements such as columns or walls. Due to the curvature of the two sliding surfaces, any horizontal relative motion also induces a relative vertical motion, i.e. an uplift of the top plate. The motion is comparable to that of a pendulum with a length R eff (effective radius). For a horizontal displacement x of the pendulum, a weight N Sd resting on the top plate is lifted up by an amount z. Considering the kinematic relations as shown in Fig. 2, the vertical motion can be easily determined. At x = 0, the radius of curvature of z(x) is R eff , therefore the second derivative is z´´ = 1/R eff . By integration we obtain approximately (for small values of x) z´ = x/R eff and z(x) = x 2 1/2R eff . In the offset position x there is a re-centering force F r ≈ N Sd z´ = x N Sd /R eff which acts in tangential direction. In addition to the influence of the effective radius R eff , the force is also affected by the magnitude of the friction in the sliding surfaces. In the following analysis, a Coulomb friction model with a constant dynamic coefficient of friction μ is assumed.
3 Earthquake excitation model The ground excitation model used in this paper loosely follows the model given by [13]. It is based on an evolutionary random process generated by passing an amplitude-modulated white noise (shot noise) through a second order filter of the Kanai-Tajimi type which is described by the ground frequency ω g and the ground damping ratio ζ g . Based on this model, the earthquake excitation a g (t) is generated as
in which y f is a filter variable governed by the second-order equation
Here w(t) is white noise with intensity D 0 , i.e. its autocorrelation function is R ww (τ) = D 0 δ(τ), and e(t) is a deterministic dimensionless modulating function, here chosen as
1
Sketch of CSS of type Single with building structure © Aus [12]
2
Simplified kinematics of CSS © Christian Bucher
The modulating function parameters are chosen as t 1 = 1.10 s and t 2 = 12.18 s. ω g and ζ g denote characteristic site-dependent ground parameters. In the following example, the numerical values ω g = 20 rad/s and ζ g = 0.60 are chosen together with D 0 = 0.1347 m 2 /s 4 . This choice leads to an average PGA of 5 m/s 2 . Different values of the PGA can easily be obtained by scaling the generated time series accordingly. Some sample records based on this model are shown in Fig. 3.
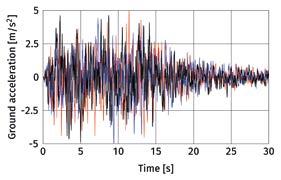
As mentioned previously, peak ground accelerations are of the order of 5 m/s 2 . The Kanai-Tajimi model as used in this paper actually compares quite well to the response spectra as specified by EC8 (Soil A, ground acceleration 5 m/s 2 , structural damping ratio ζ = 0.05). Computing the average response spectra for a set of 100 digitally simulated earthquake records it can be seen that there is very close agreement (for structural damping ratio of 5%, cf. Fig 4).
4 Dynamic response analysis As discussed above, the structural model is assumed to be given. The CSS-system is characterized by an effective radius R eff and a friction coefficient μ. These two parameters are considered to be nominal values, but they may be actually different in the final structure, e.g. due to manu facturing tolerances and other random effects. This possible difference is modeled as stochastic uncertainty of all parameters with a defined coefficient of variation. For the CSS system it is assumed that the effective radius R has almost no uncertainty because it can be manufactured very precisely, but its friction coefficient μ is assumed to have a rather large stochastic variability. In the following analysis, all stochastic uncertainties are described by log-normal distributions. The design parameters are varied together with the earthquake intensity expressed by the average peak ground acceleration PGA. The analysis consists of nested loops. In the outer loop, the mean values of the design parameters R eff and μ are varied in a pre-defined range (Fig. 5). This variation is done in terms of a Design of Experiments (DOE) based on the Latin Hypercube Sampling method (e.g. [14]) using 50 sample vectors. For each of these designs, an inner-loop analysis is carried out. This inner loop considers the random variability of the system and design parameters. It is realized in terms of a Monte-Carlo simulation with 128 samples per design point. In each simulation, a new random earthquake record is generated based on the Kanai-Tajimi model as outlined earlier. Also, for each sample, random values for the system and design parameters are generated using the previously generated mean values. For each complete set of Monte-Carlo samples, the second order statistics (mean values and standard deviations) are computed and stored.
4
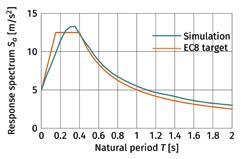
Comparison of EC 8 response spectrum to averaged response spectrum values of Kanai-Tajimi model © Christian Bucher
Parameter R eff [m] μ [%]
Lower Bound 1.5 3
Upper Bound 4.0 6
Coefficient of Variation 0.02 0.25
5 Range and variability of parameters considered © Christian Bucher
The main goal of the analysis is to develop a simple tool for estimating the maximum CSS displacement x* and column displacement y* depending on the CSS design (effective radius R eff and dynamic coefficient of friction μ) for a given earthquake intensity (peak ground acceleration). The rationale behind it is that the values of x* and y* are chosen such that they are exceeded only with a very small probability P^* (here we choose P* = 10 -4 ). This requires to obtain a probability distribution function of the CSS peak displacement x and the structural displacement y from a Monte-Carlo simulation run. This distribution function is assumed to be an Extreme-Type I (Gumbel) distribution whose parameters are computed from the mean value and standard deviation of the quantity under consideration. Given the mean value μ X and the standard deviation σ X of a Gumbel-distributed quantity X, its probability distribution functions is given by
From this equation, a characteristic value (quantile q) x q_ can be readily computed by solving
For a 99.99% quantile this results in
Finally, the dependence of these statistical data μ X and σ X on the currently used mean values of structural and design parameters is expressed in terms of a meta-model (cf. section 5.2).
5 Numerical Example 5.1 Bridge Model A simple bridge model as previously described in [15] is utilized to demonstrate the applicability of the approach as suggested. This skewed bridge is supported by a total of 8 CSS systems. The fundamental period of vibration of the structure on the 8 CSS is T = 4.03 s if the effective radius is chosen to be R eff = 4 m. This period corresponds to a longitudinal rigid body translation of the bridge deck. Due to its large in-plane stiffness, the bridge deck essentially behaves like a rigid block concerning all horizontal motions. The earthquake excitation a(t) is assumed to be acting in longitudinal direction of the bridge only. Neither lateral nor vertical components are considered for the present analysis. The response quantities of interest are on one hand the maximum displacement occurring in the CSS systems (this is relevant for the proper choice of the size of the slider) and the maximum displacement of the column heads (this is relevant for the design of the column and its footing).
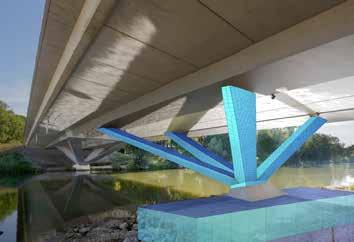
6
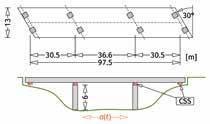
Bridge Model (after [15]) © Christian Bucher
5.2 Establishing the meta-model For this structure equipped with CSSsystems, the analysis is carried out as described earlier. Meta-models for the mean values and the standard deviations of the peak support displacement and the CSS peak displacement are developed for earthquake records with PGA values of 2 and 5 m/s 2 . Here, meta-models based on a combination of polynomials and radial basis functions as described in [16] are used. These meta-models are then used to perform parametric studies showing the influence of the structural and the CSS system design parameters on the response. This is explained in more detail in [12]. The required 99.99% quantile values are the calculated from the means and the standard deviations according to Eqs. 4–6. 5.3 Approximate Solution In order to obtain an estimate for the performance of a CSS system, a simple pre-design rule can be derived from the numerical results. This design rule includes the effective radius of curvature R and the friction μ in the CSS sliding surface. The formula is derived by regression on the meta-models for different values of the PGA and it expresses the characteristic value of the peak CSS displacement x* for PGA of 2m/s 2 as x 2 * = 0.069 + 0.0335R eff – 2.350μ – 0.00197R 2 eff + 24.42R eff μ – 0.149μ 2 and for the PGA of 5 m/s 2 as x 5 * = 0.108 + 0.128R eff – 3.378μ – 0.0094R 2 eff + 28.84R eff μ – 0.346μ 2
30 Tage testen www.sofistik.de/trial
Brückenbau
Bleichinselbrücke Heilbronn (Deutscher Brückenbaupreis 2018) Auftraggeber: Amt für Straßenwesen Heilbronn Statik & Architektur: sbp GmbH - schlaich bergermann partner
inf o @ sofistik.de www.sofistik.de
7 8
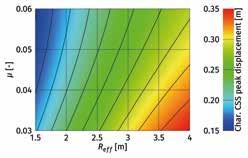
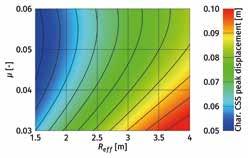
Approximation for characteristic values (99.99% quantile) of peak CSS displacement for PGA of 2 m/s 2 (left) and 5 m/s 2 (right) © Christian Bucher
Note that in these equations, the numerical values R in m, and values for μ are considered to be numbers (e.g. 0.04). As noted earlier, these characteristic values correspond to an exceedance probability of 10 -4 . The results based on the simple formula are shown in Figs. 6. The results für the peak support displacements are similar. For the final design of the CSS system, of course, a full dynamic analysis is highly recommendable.
6 Conclusions The design of CSS systems for seismic protection of structures subjected to earthquake excitations needs to account for sufficient displacement capacity of the device in order to prevent accidental slip-off and potentially structural collapse. The essential quantity for the design is the peak relative motion of the CSS that occurs during an earthquake. Due to the random nature both of magnitude and frequency content of earthquakes it is understood that this quantity is of statistical nature. The numerical solution for this problem is shown in the paper. To make this solution applicable to the daily work of engineers an approximate solution based on a polynomial regression formula with high accuracy is presented. This approximation allows that engineers can do a quantitative probabilistic design of CSSs with respect to their displace ment capacity for a prescribed high level of safety against larger relative motions than their displacement capacity and therefore against structural collapse.
Autor: Prof. Dipl.-Ing. Dr. techn. Christian Bucher Research Unit of Structural Dynamics and Risk Assessment Technische Universität Wien, Wien, Österreich References [1] Fenz, D. M.; Constantinou, M. C.: Modeling triple friction pendulum bearings for responsehistory analysis. Earthquake Spectra, 2008, 24, 1011–1028. [2] Kumar, M.; Whittaker, A. S.; Constantinou, M. C.: Characterizing friction in sliding isolation bearings. Earthquake Engineering & Structural Dynamics, 2015, 44, 1409–1425. [3] Weber, F.; Meier, L.; Distl, J.; Braun, C. (n.d.): Optimum design of curved surface sliders based on site-specific seismic input and its sensitivity. Geosciences, 8. [4] Quaglini, V.; Gandelli, E.; Dubini, P.: Experimental investigation of the re-centring capability of curved surface sliders. Structural Control and Health Monitoring, 2017, 24, e1870. [5] Rangid, R. S.: Optimum friction pendulum system for near-fault motions. Engineering Structures, 2005, 27, 349–359. [6] Calvi, G. M.; Ceresa, P.; Casarotti, C.; Bolognini, D.; Auricchio, F.: Effect of axial force variation in the seismic response of bridges isolated with friction pendulum systems. Journal of Earthquake Engineering, 2004, 8, 187–224. [7] Nagarajaiah, S.; Sahasrabudhe, S.: Seismic response control of smart sliding isolated buildings using variable stiffness systems. An experimental and numerical study. Earthquake Engineering & Structural Dynamics, 2006, 35, 177–197. [8] Weber, F.; Distl, J.; Meier, L.; Braun, C.: Curved surface sliders with friction damping, linear viscous damping, bow tie friction damping and semi-actively controlled properties. Structural Control and Health Monitoring, 2018, 25. [9] Mazza, F.; Vulcano, A.; Mazza, M.: Nonlinear dynamic response of rc buildings with different base isolation systems subjected to horizontal and vertical components of near-fault ground motions. The Open Construction and Building Technology Journal, 2012, 373–383. [10] Mazza, F.; Mazza, M.: Sensitivity to modelling and design of curved surface sliding bearings in the nonlinear seismic analysis of base-isolated r.c. framed buildings. Soil Dynamics and Earthquake Engineering, 2017, 100, 144–158. [11] Bucher, C.: Probability-based optimization of friction damping devices. Structural Safety, 2009, 31, 500–507. [12] Bucher, C.; Weber, F.; Braun, C.: Displacement capacity of curved surface sliders. Probabilistic design. In C. Adam, G. Achs, & T. Furtmüller (Eds.), D-A-CH Tagung Erdbebeningenieurwesen & Baudynamik, 2019, 499–506. [13] Fan, F.-G.; Ahmadi, G.: Nonstationary Kanai-Tajimi models for El Centro 1940 and Mexico City 1985 earthquakes. Probabilistic Engineering Mechanics, 1990, 5(4), 171–181. [14] Bucher, C.: Computational analysis of randomness in structural mechanics (D. M. Frangopol, Ed.). Structures and Infrastructures Book Series, 2009, Vol. 3, London. [15] Constantinou, M. C.; Whittaker, A. S.; Fenz, D. M.; Apostolakis, G.: Seismic isolation of bridges. State University of New York, 2007. [16] Bucher, C.: Metamodels of optimal quality for stochastic structural optimization. Probabilistic Engineering Mechanics, 2018, 54, 131–137.