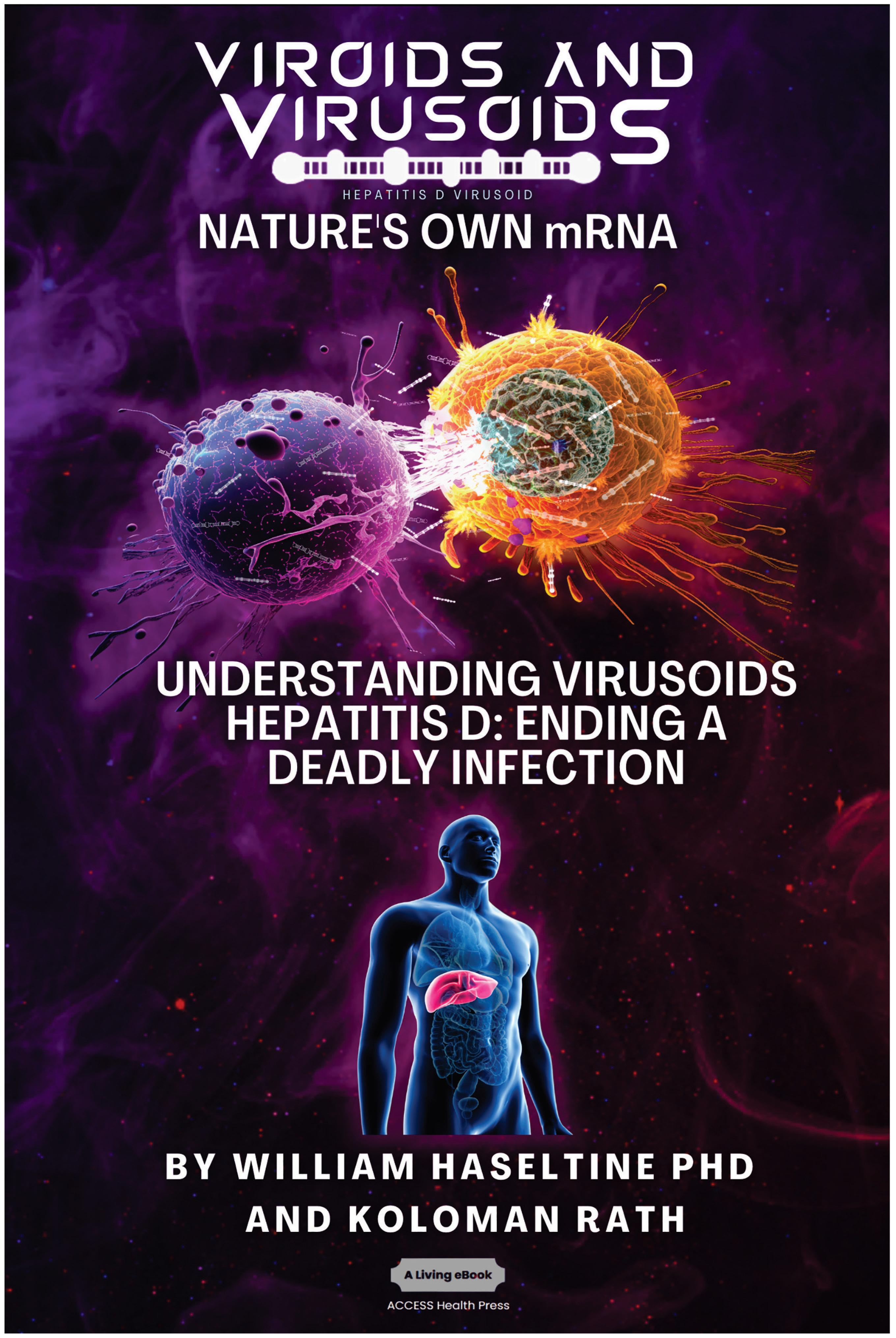
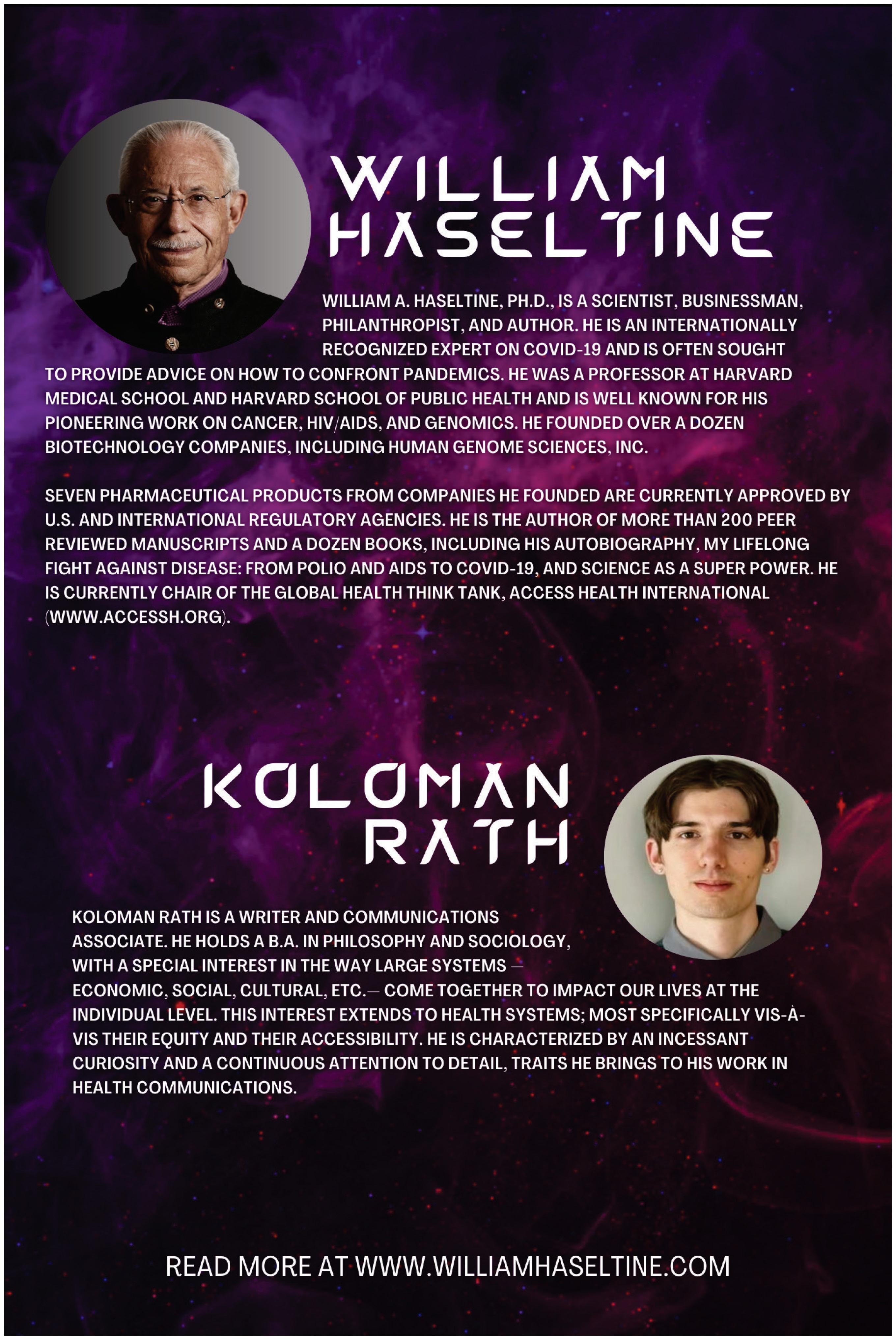
Copyright © 2023 by William A.
Haseltine, PhDCover art by Kim Hazel
All rights reserved. No part of this book may be used or reproduced by any means, graphic, electronic, or mechanical, including photocopying, recording, taping, or by any information storage retrieval system, without the written permission of the publisher except in the case of brief quotations embodied in critical articles and reviews.
All author proceeds from the sale of this book will be donated to the nonprofit global think tank ACCESS Health International.
Affordable Excellence: the Singapore Healthcare Story ; William A Haseltine (2013)
ImprovingtheHealthofMotherandChild:SolutionsfromIndia; Priya Anant, Prabal Vikram Singh, Sofi Bergkvist, William A. Haseltine & Anita George (2014)
Modern Aging: A Practical Guide for Developers, Entrepreneurs, and Startups in the Silver Market ; Edited by Sofia Widén, Stephanie Treschow, and William A. Haseltine (2015)
AgingwithDignity:InnovationandChallengeisSweden-The Voice of CareProfessionals;Sofia Widen and William A. Haseltine (2017)
Every Second Counts: Saving Two Million Lives. India’s Emergency responseSystem.TheEMRIStory;William A Haseltine (2017)
VoicesinDementiaCare;Anna Dirksen and William A Haseltine (2018)
AgingWell ; Jean Galiana and William A. Haseltine (2019)
WorldClass.Adversity,TransformationandSuccessandNYULangone Health ; William A. Haseltine (2019)
Science as a Superpower: My Lifelong Fight Against Disease And The HeroesWhoMadeItPossible ; William A. Haseltine (2021)
The Future of Medicine: Healing Yourself | Regenerative Medicine; William A Haseltine (2023)
A Family Guide to Covid: Questions and Answers for Parents, GrandparentsandChildren ; William A. Haseltine (2020)
ACovidBackToSchoolGuide:QuestionsandAnswersforParentsand Students ; William A. Haseltine (2020)
CovidCommentaries:AChronicleofaPlague,VolumesI,II,III,IV,V, andVI ; William A. Haseltine (2020)
MyLifelongFightAgainstDisease:FromPolioandAIDStoCovid-19 ; William A. Haseltine (2020)
Variants!:TheShape-ShiftingChallengeofCovid-19VaccineEvasion& Reinfection ; William A. Haseltine (2021)
CovidRelatedPost-traumaticStressDisorder(CV-PTSD):WhatItIsAnd WhatToDoAboutIt ; William A. Haseltine (2021)
NaturalImmunityAndCovid-19: What It Is And How It Can Save Your Life ; William A. Haseltine (2022)
Omicron:FromPandemictoEndemic ; William A. Haseltine (2022)
Monoclonal Antibodies: The Once and Future Cure for Covid-19; William A. Haseltine and Griffin McCombs (2023)
Messenger RNA (mRNA) has become a key therapeutic modality. Propelled into the spotlight by the COVID-19 pandemic, vaccines are only the tip of the iceberg: there’s an mRNA revolution on the horizon (Bates, 2021). From cancer treatment to gene therapy to drug development, mRNA will soon be everywhere. At its core, the idea behind mRNA technology is a simple one. Messenger RNAs are transfected into cells and cause a useful phenotypic change. But long before mRNA was thought of as a potential therapeutic drug, nature devised its own transmissible messenger RNA: viroids and virusoids. A study of how these small, naked RNAs can be 1) transmitted from one organism to another, 2) enter target host cells, 3) migrate from the cytoplasm to the nucleus of cells, 4) redirect DNA-dependent RNA polymerases to transcribe RNA, 5) in the case of hepatitis D “virus”, direct the synthesis of its own proteins, and 6) transit the nucleus and cytoplasm to exit the cell and restart the cycle of infection are all lessons we can learn to increase the efficiency of mRNA used as drugs.
In addition to shedding light on potential futures for mRNA, this book also outlines the relevance of virusoids to the fatal human disease of hepatitis D and encourages additional research on the topic.
The format of this book is something that I have dubbed a living ebook. I will continue to update ViroidsandVirusoids:as we learn more. You may view these updates at no additional cost by visiting:
https://www.williamhaseltine.com/viroids/
Password: viroids
Thank you for your interest.
To my wife, Maria Eugenia Maury, my children Mara and Alexander, my stepdaughters Karina, Manuela, and Camila, my three grandchildren Pedro, Enrique, and Carlos, and last but not least our three dogs, Sky, Luna, and Ginger.
Koloman Rath
To my family, my partner, and my friends, who keep me honest and push me to grow.
Why Care About Viroids & Virusoids?
Viroids and virusoids are small, circular infectious RNAs. Think virus, but much simpler. All pathogens have their own “tools of the trade”, which allow them to infect and transmit between different hosts. Of course, some pathogens have more complex toolkits than others. Viruses are quite minimalist in their approach, but viroids and virusoids are more extreme still. Where all viruses encode at least one protein in their genome, used to produce an enzyme that can replicate the virus’ genetic information, viroids and virusoids do not. Instead, they hijack enzymes found within host cells; rather than carrying their own tools, they simply make use of those already present on-site. They are also much smaller than viruses. For comparison, SARS-CoV-2 is made up of nearly 30,000 nucleotides —the basic building blocks of DNA and RNA— yet viroids only contain between 220 to 450 nucleotides (Lee & Koonin, 2022; Sah et al., 2020). Virusoids are generally a similar size, albeit with some larger outliers: the biggest virusoid clocks in at roughly 1700 nucleotides (Taylor, 2015).
So, why should you care about these tiny biological oddities?
First, there’s sheer curiosity. Viroids and virusoids represent a branch of life that was entirely unheard of until 1971 (Viroid: Usda Ars , n.d.). As a result, it has been overlooked and understudied. I call these pathogens living because they express some of the most fundamental properties: they can reproduce themselves and they can mutate, whether at random or in response to external pressures. True, they are a very stripped down form of life —perhaps the most stripped down— but they are living all the same. Indeed, their simplicity is one of the very features that makes them so exciting and may be key to understanding the origins of life as a whole. The RNAworld hypothesis postulates that RNAs with genetic information and catalytic functions constitute the first step in the long journey from primordial soup to complex life (Saito, 2022). Viroids and virusoids are examples of exactly this; they are small, simple RNA molecules
that replicate, and crucially, contain ribozymes, which are active sections of RNA that behave like enzymes, able to cut up and reconnect RNA. Although we have gathered answers to some of the pressing questions about these microbes, many more remain. From what we have learned thus far, however, it is clear that viroids and virusoids hold scores of lessons for us regarding fundamental processes in nature and biology.
Next, these infectious circular RNAs cause major diseases in crops and humans. The first viroid to be identified was the potato spindle tuber viroid (PSTVd) (Diener, 1971). As the name suggests, this viroid infects potatoes, which end up cracked, dumb-bell shaped, and a fraction of their usual size think ginger root, a far cry from what we expect a potato to look like (Figure 2). On top of the damage to individual potatoes, potato spindle tuber viroid infection also causes a drastic decrease in overall yield, in some cases by as much as 64% (Hammond, 2017). Aside from potatoes, which are the fourth-most important crop worldwide, viroids wreak similar havoc on a variety of other important food and textile crops: apples, avocados, citrus fruit, tomatoes, hop, palms, and the list goes on (Group, 2020; Hammond, 2017; Rodriguez et al., 2017; SaucedoCarabez et al., 2014; Scheck, 2020; Sharma et al., 2020; Vernière et al., 2004)
Hemp plants have been especially hard-hit, which is bad news for the skyrocketing cannabis industry (Bektaş et al., 2019) (Figure 3). Considered one of the fastest-growing industries in the U.S., worth more than $26.5 billion in 2022 (Untitled, n.d.), growers across the country have started to struggle with the onslaught of hop-latent viroid (HLVd). In California, the viroid has infiltrated as many as 90% of cultivation sites (Adams, 2022). The result? More than $4 billion annual losses for growers. Speaking to Globe Newswire, Dr. Bryce Falk, an esteemed plant pathologist working at the University of California, Davis, mentions: “Hop Latent Viroid is perhaps the
greatest threat to the legal cannabis industry in the United States. It is very difficult for growers to identify due to its latency and it can spread undetected within a grow, wiping out much of the commercial value.” (Dark Heart Data Shows Hop Latent Viroid Drives $4b Annual Losses to Legal Cannabis Crop, 2021)
In humans, the hepatitis D virusoid causes the most severe form of viral hepatitis (Asselah & Rizzetto, 2023). In general, those suffering from chronic hepatitis D infection experience liver failure within five to ten years, and 15% of people will experience liver failure as quickly as one or two years after initial infection (What Is Hepatitis D - Faq | Cdc, 2020). Compare this to chronic hepatitis B, where liver failure often takes forty plus years to develop. Although this is the only virusoid known to infect humans, many similar virusoids have recently been discovered in other animal species —from termites all the way to deer— indicating that virusoid-induced diseases may be significantly more common than initially suspected (Iwamoto et al., 2021).
Finally, viroids and virusoids may help improve our understanding of RNA dynamics and, in so doing, expand our pharmacopeia. The success of the Covid-19 vaccines helped catapult mRNA technology into the limelight, but the field as a whole is still in its infancy or early childhood. The range of potential applications is growing steadily, from cancer immunotherapy to the treatment of genetic diseases (Qin et al., 2022). Still, a few critical questions persist: what’s the best way to deliver mRNA macromolecules?; what’s the best way to deal with the inherent instability of mRNA?; how do we overcome the immunogenicity of therapeutic mRNA? By studying RNA in all of its forms, we may come closer to finding answers to these lingering questions. Viroids and virusoids are particularly interesting in this regard since they achieve so much with so little. These tiny slivers of RNA which, for the most part, do not encode any proteins somehow manage to transmit between different hosts, hijack host cellular machinery, and cause disease. Despite their small size, they pack a hefty punch. Their functional minimalism may provide us with new insights for the development of sleeker, more efficient RNA therapeutics.
Viroids and virusoids have primarily, and almost exclusively, been considered plant pathogens. A growing body of research challenges this view; not only are they far more common than previously assumed, they are also found in a tremendously diverse range of environments and hosts.
At the heart of these recent discoveries lies a method known as metatranscriptomics. Pioneered in the early 2000s, metatranscriptomics refers to a series of strategies that enable the characterization of the RNA content of large environmental samples (Poretsky et al., 2005). For example, researchers might extract a scoop of soil from a bog in Scotland and analyze that soil for all of the RNA sequences contained within it. The RNA can then be cross-referenced against large databases to establish matches with known RNA sequences. In a sense, metatranscriptomics acts as a giant sieve that filters out everything that isn’t RNA content.
Of course, it’s not quite as simple as that. Metatranscriptomics is a complex process involving multiple steps (Ojala et al., 2023). After initial sample collection, the RNA has to first be extracted and separated from the rest of the contents. The RNA has to then be prepared for high-throughput sequencing, which may include enrichment of desired RNA there are various different types of RNA, some more common than others. Once the desired RNA has been sufficiently enriched, it can be sequenced. And finally, algorithms and other bioinformatic strategies are used to analyze the sequencing data.
A group of researchers from The United States National Library of Medicine (NLM) recently developed a “pipeline” to filter metatranscriptome data for RNA sequences characteristic of viroids and other circular, viroid-like RNAs. Their work sheds light on the global ubiquity of these small infectious RNAs (Lee et al., 2023).
By mining through upwards of 5,000 metatranscriptomes collected from across the globe, the scientists unearthed 11,300 viroid-like
circular RNA sequences. Of these, roughly ten thousand were entirely new viroid-like RNAs, not listed in any of the major viroid databases. Their findings expand the pool of known viroid-like RNAs five times over.
Detection of the viroid-like sequences was observed in numerous locations worldwide, including Colombia, the Czech Republic, Germany, the USA, Japan, Malaysia, and others. Some sequences themselves have been found to cover vast geographic areas as well, such as one particular sequence detected from the northern tip of Alaska all the way down to Florida.
Crucially, the researchers found that the metatranscriptomes they studied were mainly comprised of prokaryotic sequences. In fact, one of the metatranscriptomes that contained viroid-like RNA did not contain any eukaryotic sequences at all, suggesting that the viroid-like RNAs were replicating in prokaryotic hosts. This runs counter to the notion that viroids only infect plants. Indeed, it significantly broadens the potential host range for viroid-like RNAs.
Circular, tiny, and infectious. These three words are frequently used to describe viroids, but they do not really tell us much. This section aims to fill in the gaps, providing an in-depth overview of viroids and everything there is to know about them.
Although viroids are made up of circular RNA, they are rarely circular in practice. This is because of a high rate of sequence selfcomplementarity; the nucleotides that form the genome pair up with their respective bases, folding the RNA into either a rod-like or branched secondary structure (Figure 4) (Moreno et al., 2019).
Areas of the genome that do not exhibit self-complementarity end up as bulges or loops — “dead space”.
FIGURE 4. The viroid is a single-stranded piece of circular RNA that has an unusual structure due to complementary base pairing. Some form a simple rodlike structure, whereas other viroids have a complex branched structure.
SOURCE: ACCESS Health International
These distinct secondary structures correspond to two separate families of viroids: pospiviroidae and avsunviroidae , respectively. But secondary structure is not the only thing that distinguishes these viroid families. Whereas pospiviroidae replicate in the nucleus of the host cell, viroids in the avsunviroidae family instead replicate in host plastids, usually the chloroplast (Di Serio et al., 2018; Di Serio et al., 2021). Also, pospiviroidae have a central conserved region (CCR) that is shared by all viroids in the family; this region is involved in critical interactions with host factors and is essential for viroid replication, processing, and pathogenicity.
Where members of the pospiviroidae family have a central conserved region, those of the avsunviroidae family do not. Instead, the avsunviroidae are characterized by the presence of ribozymes (Figure 5). In short, ribozymes are RNA molecules that have their own catalytic activity. Until the discovery of ribozymes in 1982 by Sidney Altman and Thomas R. Cech, RNA was thought of simply as a store of genetic information (Kruger et al., 1982). Their findings, however, established that RNA can also have catalytic properties. In particular, ribozymes can cut up sections of RNA, known as cleavage, and even glue them back together, known as ligation. Often, these processes are autocatalytic in nature the very genome of which the ribozyme is a part is cleaved and later ligated (Symons, 1999). In 1989, Altman and Cech were awarded the Nobel Prize in chemistry for their efforts (The Nobel Prize in Chemistry 1989, n.d.).
the negative (-) strand viroid genome.
SOURCE: ACCESS Health International
Since pospiviroidae lack ribozymes they cannot self-cleave their genome during replication. Instead, they depend on host enzymes for cleavage. This is one of the most significant differences between the two families and it impacts the replication pathway each
undergoes. Although both rely on the same general mechanism, the nuances of the process vary across the two families.
At its core, viroid replication can be broken down into three main stages: transcription of the circular genome, cleavage, and finally ligation (Wang, 2021). The first step in this process happens by way of rolling-circle replication and is largely identical in both viroid families. From there on, however, their replication pathways diverge.
How does rolling-circle replication work? Since viroids do not encode any proteins of their own, they have to hijack host proteins to do their bidding for them. Specifically, they redirect host DNAdependent RNA polymerases (DdRPs) to transcribe their RNA. This itself is already an astounding feat; DNA-dependent RNA polymerases usually take DNA as a template and transcribe it into RNA, but somehow viroids subvert the polymerases to instead take RNA as a template and transcribe it into complementary RNA (Girbig et al., 2022). Once the viroid has migrated to its replication site either the nucleus or the chloroplast, depending on the family a host cell polymerase binds to the circular genome and, as it moves along the genome, begins to transcribe it. In the case of pospiviroidae, this is done by RNA polymerase II. In the case of avsunviroidae, transcription is thought to be performed by a nuclear-encoded plastid RNA polymerase (NEP).
Instead of stopping after one full rotation around the genome, the host polymerase in charge of transcription continues to circle the genome multiple times over. Each time the host polymerase completes one rotation, it produces one complete RNA transcript.
And each new transcript displaces the one before it, making room for the next. The transcripts build up head-to-tail, forming a long, linear chain called an oligomer. Each individual transcript in the oligomer is a perfect mirror-image of the original template RNA (Figure 6).
For replication to be successful, the mirror-image RNAs —referred to as “negative-sense” RNAs— need to be transcribed a second time so that they are converted back to the original, “positive-sense” genome. Pospiviroidae and avsunviroidae differ in how they achieve this second round of transcription (Figure 7).
When it comes to pospiviroidae, the long oligomer made up of negative-sense RNAs is immediately transcribed a second time, resulting in a long oligomer of positive-sense RNAs. This oligomer
is then cleaved, which cuts the long chain into individual, unit-size RNAs. Since pospiviroidae do not contain a ribozyme, cleavage has to take place with the help of a host enzyme. Exactly which host enzyme cleaves the positive-sense oligomer is not known, but ribonuclease III (RNase III) is considered the most likely candidate (Flores et al., 2009).
Once cleaved, the positive-sense RNAs still need to be recircularized, known as ligation. Again, this is done by a host enzyme: DNA ligase 1. Only after the RNAs have been ligated is the replication cycle complete.
Members of the avsunviroidae family have a different strategy for replication. Since they do contain ribozymes, they do not have to rely on host enzymes for cleavage. Instead, they self-cleave the negative-sense oligomer as soon as it is done forming. The resultant unit-length transcripts are then ligated, producing circular negativesense RNAs: perfect mirror images of the original viroid genome. Ligation occurs either via the ribozyme itself or via a host enzyme called tRNA ligase. To get from these circular, negative-sense RNAs back to positive-sense genomes, the viroids undergo a second round of rolling-circle replication. This time, the negative-sense RNA is used as the initial template, eventually leading to the synthesis of multiple positive-sense RNAs.
FIGURE
potato spindle tuber viroid (PSTVd) replication, which belongs to the pospiviroidae family. On the right, an example of avocado sunblotch viroid (ASBVd) replication, which belongs to the avsunviroidae family. Plus and minus strands are indicated in green and red, respectively.
SOURCE: ACCESS Health International
Despite not encoding a single protein, viroids still cause serious diseases. How do they manage this? Although the exact mechanisms of damage remain elusive, a process known as RNA interference (RNAi) is thought to play a central role (Ramesh et al., 2021).
RNA interference (RNAi) is a natural process that regulates gene expression in most eukaryotic cells. Regulation of gene expression is crucial to keeping vital functions in balance. Insulin regulation, for example, allows us to break down blood sugar into usable energy.
If this malfunctions, we suffer severe consequences including diabetes.
To understand how viroids subvert RNA interference, it helps to understand the basic steps involved in the process more broadly. When cells encounter double-stranded RNA (dsRNA), an enzyme called Dicer cleaves it into small interfering RNAs (siRNAs). These siRNAs are then loaded into the RNA-induced silencing complex (RISC). RISC identifies complementary sequences in messenger RNA (mRNA) and binds to it. This binding either degrades the mRNA or prevents it from producing proteins, effectively silencing the gene.
As it turns out, viroid-induced disease may very well boil down to a simple case of mimicry: the rod-like or quasi-rod-like structure of viroids may trick Dicer into mistaking them for double-stranded RNA (Papaefthimiou, 2001). As a result, Dicer cuts the viroid RNA up into small snippets resembling small interfering RNAs. These are then loaded onto the RISC complex and, from there, go on to silence genes and inhibit the production of important proteins
(Figure 8) (Martínez De Alba et al., 2002). Downregulation of metabolic genes and proteins may then lead to the symptoms commonly associated with viroid infections in plants: stunted growth, distortion of the plant and fruit, skin damage, discoloration, etc.
FIGURE 8. Cutting of precursor microRNA (pre-miRNA) by the nucleases Dicer or Drosha releases the small regulatory RNAs—miRNA. Cutting of viroid RNA by the same nucleases releases small RNA molecules vsRNA. These mimic the action of miRNA. The RISC complex helps both vsRNA and miRNA to bind to complementary sequences on target messenger RNAs. This alters the expression of the mRNA. In the case of the viroid, expression is abnormal and disease results. SOURCE: ACCESS Health International (Adapted from: “Viruses, Viroids, and Prions” CLARK ET AL. 2019)
A great deal about viroid evolution remains unknown. To fill this void, a handful of competing theories have been proposed. One of the first theories was developed by Thomas R. Diener, the discoverer of viroids (Diener, 1981). Diener suggested that the small circular RNAs may actually be derived from introns. Introns are segments of DNA or RNA that do not code for proteins. Genes consist of both coding and non-coding regions, and the coding
regions are called exons. Exons contain the information necessary to produce proteins, while introns do not. Introns were once thought to be "junk DNA" without any functional purpose. However, it is now understood that introns can play essential roles in gene regulation and alternative splicing, influencing how genes are expressed and leading to the production of different protein isoforms from the same gene (Intron, n.d.).
According to Diener’s initial hypothesis, introns may have “escaped” from the genome and continued to evolve independently, eventually giving rise to viroids and viroid-like satellite RNAs (virusoids). Evidence for this is twofold: structural and genetic. The structural evidence comes from the potato spindle tuber viroid (PSTVd), which can bind to a section of U1 spliceosomal RNA (U1 RNA) critical to the act of RNA splicing (Diener, 1981). This interaction is similar to how U1 RNA helps in the removal of introns. On the genetic side of the argument, group I introns and certain viroids share a 16-nucleotide sequence and three sets of additional matching sequences (Dinter-Gottlieb, 1986).
Despite initial traction, this theory has since fallen out of favor the genomes of viroids and their hosts display little-to-no sequence similarity (Flores et al., 2022). In its place, Diener suggested a new theory in 1989, which has since risen to prominence. He posited that viroids are remnants of a primordial, pre-cellular world (Diener, 1989). Essentially, before the advent of DNA, proteins, and cells, there was RNA. This “RNA world”, as it’s called, represents the earliest phase of life as we know it; everything that came afterwards has its roots in a few initial RNAs that could replicate autonomously (Neveu et al., 2013). Diener proposed that viroids are “living fossils”
that have survived from this early RNA world into modern day. For one, viroids have all the features you would expect to see in survivors of the harsh conditions of early Earth (Flores et al., 2014). They are small, circular, and have a compact structure, which protects them from damage and replication issues. Their high cytosine (C) plus guanine (G) content stabilizes their structure and helps combat the subpar fidelity of primitive polymerases. They do not code for proteins, which is consistent with an environment free of ribosomes. And finally, some viroid RNAs are ribozymatic, displaying catalytic abilities crucial to independent replication.
One of the strongest early critiques of this theory was that viroids were not ubiquitous enough to be remnants of a precellular world if they evolved hand-in-hand with more complex life, shouldn’t they be everywhere? Recent metatranscriptomic work has pushed back against this assumption by proving that viroids are, in fact, pervasive across the globe and across a number of different hosts (see section “They Are Everywhere”). This growing body of research suggests that, more likely than not, viroids truly are remnants from a pre-cellular, RNA world.
Virusoids are very similar to viroids. Both are small, circular infectious RNAs that rely on host enzymes for effective replication. Like viroids, virusoids have a highly self-complementary genome, leading to rod-like or branched secondary structures. And, as is the case with viroids, most virusoids do not encode any proteins of their own, although there are exceptions to this. Even their replication processes are very similar, with both adopting a rollingcircle pathway.
What separates the two? While viroids can infect hosts on their own, virusoids rely on a helper virus to transmit between hosts and to enter host cells. Unlike viroids, which are naked RNAs, virusoids borrow the envelope and surface proteins of their helper virus. They then use these surface proteins to gain entry into host cells, following the same steps as their helper virus. Once inside, they go their own way and the rest of the replication cycle is independent of the helper virus.
VirusoidProteins?
One virusoid known to encode proteins is the rice yellow mottle virus satellite RNA (AbouHaidar et al., 2014). Indeed, this virusoid has a very interesting genome that allows it to produce multiple different proteins from overlapping open reading frames — the sections of a genome that can be translated into a protein. Open reading frames are flanked by a “start codon” on one end and a “stop codon” on the other. These help guide ribosomes — the proteinproducing machinery in cells — during translation by telling them
where to begin and where to end. The virusoid associated with rice yellow mottle is peculiar insofar as its start codon, composed of the nucleotides AUG, and stop codons, composed of the nucleotides
UGA, are combined: UGAUG A. Changing reading frames switches the sequence from a start codon to back-to-back stop codons: UGA UGA.
Ribosomes read nucleotide sequences in sets of three, known as codons. Each codon produces a corresponding amino acid, and as the ribosome moves along the genome, the chain of amino acids grows. Eventually, the long chain of amino acids forms a protein. Since all amino acids are made up of three nucleotides, all proteins must be multiples of three. However, the genome of rice yellow mottle virus satellite RNA, at 220-nucleotides long, is not a multiple of three. So instead of stopping after one full rotation around the genome, the ribosome has to do a second loop, this time in a different “frame register” essentially, a one nucleotide difference from the first loop (Figure 9). Because of this one-nucleotide difference, the ribosome successfully hits a stop codon after the second loop, terminating translation and yielding a protein.
Occasionally, a stop codon can end up being suppressed a phenomenon known as “leaky” codon meaning the ribosome just doesn’t stop as it usually would and instead carries on until it hits a different, downstream stop codon. This was shown to happen during translation of the rice yellow mottle virus satellite RNA, leading to even larger proteins being formed.
Hepatitis D virus is the only virusoid currently known to infect humans. It replicates in liver cells, where it causes inflammation and, over time, severe and potentially irreversible damage. Being a virusoid, it depends on a helper virus for transmission and infection.
Hepatitis B virus is the primary helper virus.
Given that hepatitis D virus depends on hepatitis B for transmission and infection, all cases of human hepatitis D occur in conjunction with hepatitis B. This can take on two different forms: co-infection or super-infection. Co-infection happens when hepatitis D virus (HDV) and hepatitis B virus (HBV) infect someone at the same time. Super-infection, on the other hand, refers to a hepatitis D infection that takes hold in someone who is already suffering from a chronic hepatitis B infection. In this scenario, the person initially had a chronic HBV infection, and then HDV infects them on top of the existing HBV infection. The disease outcomes for each are vastly different.
90% of co-infections are acute, meaning patients clear both viruses and make a full recovery (Tseligka et al., 2021). Only less than 5%
of cases lead to chronic illness. Acute hepatitis usually appears 3 to 7 weeks after catching the virus. There are two phases: the first one may not show clear symptoms, but people might feel tired, lose their appetite, or have nausea. Doctors also find elevated blood markers that suggest liver trouble. During co-infection, a patient’s liver enzymes will typically undergo one or two peaks, often separated by a few weeks. This is called 'biphasic hepatitis.' The first peak is related to the hepatitis B infection and comes with specific markers in the blood. The second peak is linked to the hepatitis D infection and has different markers. Sometimes, the order of the peaks can be different, but it depends on the amount of each virus in the body.
Unlike co-infections, which are generally cleared, the majority of super-infections lead to chronic hepatitis D infection upwards of 90% (Farci & Niro, 2012). This is on top of the already present chronic hepatitis B infection. Once a hepatitis D infection transitions to chronicity, disease outcome worsens and the risk of complications increases significantly. In fact, chronic hepatitis D infection is considered the most severe form of all hepatitis infections. Liver tissue from patients with super-infected hepatitis D often shows signs of serious liver inflammation and advanced scarring. Compared to those with only chronic hepatitis B, hepatitis D virus-infected patients progress more quickly to cirrhosis, a serious condition where the liver becomes damaged and scarred. Cirrhosis can also lead to liver failure and liver cancer, which are lifethreatening. In one study of people with chronic hepatitis (carriers of hepatitis B with hepatitis D inside the liver), about 39% developed cirrhosis or liver failure during the following 6 years (Rizzetto, 1983). Overall, in patients who suffer from chronic
hepatitis B and hepatitis D infections at the same time, the risk of developing cirrhosis appears to be twice as high compared to those with only HBV infection (Fattovich et al., 2008).
During both co- and super-infection, hepatitis D virus suppresses replication of hepatitis B virus (Negro, 2014). This is evidenced by a decrease in multiple hepatitis B virus replication markers, including hepatitis B e Antigen (HBeAg), total hepatitis B virus DNA, and pregenomic RNA (Alfaiate et al., 2016). Suppression of hepatitis B virus continues even in instances of chronic hepatitis D virus infection. The precise mechanism by which this happens is still poorly understood. One potential explanation is that the hepatitis D virus antigens repress two important enhancer regions (Enh1 and Enh2) of the hepatitis B genome (Williams et al., 2009). These are critical to successful hepatitis B virus replication. Repression of the two enhancers is considered one of the main mechanisms of hepatitis B virus inhibition.
The various hepatitis D virus sequences gathered globally have been classified into eight distinct genotypes (HDV-1 to HDV-8). HDV-1 is the most widespread genotype, found in major regions like Europe, the Middle East, East Asia, America, and Africa (Usman et al., 2020). The other genotypes (HDV-2 to HDV-8) have specific geographical and ethnic associations. HDV-2 and HDV-4 are found in North and East Asia, respectively. HDV-3 is exclusively found in the northern part of South America (Brazil, Peru, Colombia, Argentina, Ecuador, and Venezuela). HDV-5 to HDV-8 were initially thought to be found only in Africa, but a recent study
discovered HDV-8 in Northeast Brazil, likely introduced through historical slave trades (Makuwa et al., 2008; Santos et al., 2016).
FIGURE 10. Worldwide prevalence of HDV among HBV carriers in 2015.
SOURCE: By Mario Rizzetto, University of Torino, Italy -
v:WikiJournal_Preprints/Epidemiology_of_the_Hepatitis_D_Virus Mario Rizzetto, licensed CC-BY, CC BY 4.0, https://commons.wikimedia.org/w/index.php?curid=84135496
Different HDV genotypes have varying clinical courses and outcomes in human infections. HDV-1 strains show a wide range of virulence, HDV-2 (and HDV-4) cause milder liver disease, while HDV-3 isolates are linked to severe outbreaks of hepatitis in South America. The characteristics of HDV 5-8 isolates are not well understood.
For a long time hepatitis B virus was considered the sole helper virus to hepatitis D, providing the latter with an envelope and surface
proteins. Similarly, humans were thought to be the sole hosts. Both of these axioms are turning out to be mistaken.
Scientists at the Université Claude Bernard Lyon 1, France engineered hepatitis D virions enveloped with vesicular stomatitis virus (VSV) or hepatitis C virus (HCV) surface proteins, instead of the usual hepatitis B virus envelope (Perez-Vargas et al., 2019). They then artificially inserted the new virions into liver cells a process known as transfection to see what would happen. The researchers noticed that the engineered virions were successfully able to enter and exit cells, suggesting the possibility of onward transmission. Indeed, nine days after introducing the genetic material, the team observed significantly elevated levels of HDV RNA in the liquid surrounding the cells. Curiously, the levels of hepatitis D virus RNA were sixfold higher in the liquid surrounding cells infected with the alternative-envelope virions than in the liquid surrounding cells infected with regular, hepatitis B-enveloped virions.
Notably, the virions with alternative envelopes could infect kidney cells as well as the usual liver cells; the “normal” hepatitis D virions could not do so. Clearly, the pool of potential helper viruses is greater than initially suspected. It is possible that many degenerative diseases of unknown etiology are due to this type of co-infection with hepatitis D virus and a helper virus of unknown origin.
In 2018, Australian researchers made a significant discovery during an avian metatranscriptome study (Wille et al., 2018). They found
traces of hepatitis D virus-like agents in ducks captured at a watertreatment plant in Melbourne. Although the nucleotide content of these RNA sequences differed slightly from human hepatitis D virus, they shared several features that made them think the two shared a common ancestry. For one, the avian hepatitis D virus-like RNA formed a circular structure with a similar unbranched rod-like shape observed in human hepatitis D virus. Added to this, the avian virus had an open reading frame in a similar location to that of human hepatitis D virus and both sequences had sections of ribozymatic RNA.
But ducks were only the beginning. A follow-up study by the same researchers discovered that the range of hosts is far wider (Chang et al., 2019). From the more than a billion nucleotides gathered across various metatranscriptome libraries, the researchers were able to isolate four novel hepatitis D virus-like agents. These belonged to Subterranean termite, Asiatic toad, Chinese fire belly newt, and a mixture of fish. Additional studies have since found hepatitis D virus-like RNAs in an even larger range of animals, including: whitetailed deer, passerine birds, and woodchucks (Iwamoto et al., 2021). Importantly, none of the animals were co-infected with hepatitis B virus, suggesting that other helper viruses must be at play.
Combined, these findings force a reevaluation of the notions that hepatitis D virus(oid) can only infect humans and that hepatitis B virus is the sole helper virus. Virusoid-induced diseases may be far more common than we realize.
SOURCE: ACCESS Health International (Based on findings from: 1. Chang et al. 2019, https://doi.org/10.1093/ve/vez021 and 2. Iwamoto et al. 2021, https://doi.org/10.1093/ve/veab003)
As with other virusoids, the genome of hepatitis D virus is highly self-complementary — roughly 74% of the genome is intramolecularly base-paired (Netter et al., 2021). As a result, the circular RNA folds in on itself to form a rod-like secondary structure (Figure 12). Hepatitis D virus also possesses two ribozymes, one in the genomic and another in the antigenomic strand. These ribozymes are similar in secondary structure and in sequence.
Unlike most other virusoids, hepatitis D virus encodes two proteins: the small hepatitis D virus antigen (SHDAg) and the large hepatitis D virus antigen (LHDAg). Both of these proteins are encoded in the antigenomic (+) strand and are produced from the same open reading frame.
The proteins are multipartite and include various different signals. They contain a nuclear localization signal (NLS) and a nuclear export signal (NES), which helps them migrate between the cytoplasm and the nucleus as needed. The small hepatitis protein also contains signals that allow it to redirect the cellular DNADependent RNA polymerases for transcription (Lee et al., 1995). The large protein contains an additional attachment signal for the hepatitis B virus envelope, facilitating assembly of the viral particle.
The two antigen proteins are both integral to the hepatitis D virus life cycle (Zi et al., 2022). The small antigen is produced during the early stages of replication. In the nucleus, the small antigen interacts
with host enzymes called RNA polymerase II. Although usually used to transcribe DNA into RNA, hepatitis D virus manages to hijack the polymerase and direct it to transcribe RNA. By interacting with the polymerase, the small hepatitis D antigen displaces a protein complex known as negative elongation factor (NELF), which usually controls transcription (Yamaguchi et al., 2001). With this obstacle removed, the replication of hepatitis D genomic RNA can start.
The large antigen, in turn, serves to temper replication once sufficient HDV RNA has accumulated in the nucleus. By inhibiting further transcription, the large antigen prepares the newly created genomes for the next step: assembly of the full viral particle in the cytoplasm.
Along with the open reading frame in the antigenomic strand, some strains of hepatitis D virus also contain potential reading frames in the genomic (-) strand. One strain, for example, contains an open reading frame in the genomic RNA that clocks in at 359 aminoacids long (AB118819.1, n.d.). Composed of 1077 nucleotides, it spans almost two thirds of the entire genome. Compare this to the antigen-encoding open reading frame of the antigenomic (+) strand, which is 214 amino-acids-long. This is rarely, if ever, mentioned in the literature. Whether or not this potential open reading frame actually encodes any proteins remains to be determined.
Until recently, the only way to manage hepatitis D was through prevention. The hepatitis D virus relies on hepatitis B to enter and spread between cells, so by preventing the helper virus (hepatitis B), we can also protect against hepatitis D. Vaccination against hepatitis B offers this protection.
Other than prevention through vaccination, interferon alpha is the most commonly used treatment for hepatitis D. It is administered as an injection and helps to boost the body's immune response against the virus. Interferon therapy can lead to a reduction in hepatitis D virus RNA levels and an improvement in liver inflammation in
some patients. That said, it is not a true cure for the disease, instead only diminishing symptoms and slowing progression (Shah et al., 2019). Even then, its effectiveness varies among individuals, and side effects fever, chills, fatigue, muscle aches, thyroid problems, and hair loss can be challenging.
Now, a new pharmaceutical treatment called bulevirtide has entered the scene. Instead of boosting a patient’s immune response, bulevirtide directly targets the virus itself, blocking entry into cells. How? Bulevirtide binds to a specific region on the surface of the hepatitis D particle known as the pre-S1 domain. The pre-S1 domain is part of the hepatitis B virus surface protein, which hepatitis D virus depends on to gain entry into host cells. By binding to pre-S1, bulevirtide blocks hepatitis D virus from attaching to liver cells, and by extension, from being able to enter. By targeting the pre-S1 domain, bulevirtide also interferes with hepatitis D virus’ ability to replicate and produce new viral particles. This disruption in replication further limits the spread and multiplication of the virus within the liver.
Bulevirtide has shown positive results in three real-world studies. In one study, 18 patients with liver cirrhosis and hepatitis D were treated with 2mg of bulevirtide for 48 weeks, resulting in improved liver health and virological response (Degasperi et al., 2022). In a case report, a patient with organ damage from hepatitis D was essentially cured after three years of continuous bulevirtide treatment; hepatitis D virus RNA levels remained undetectable and the patient’s liver recovered significantly (Anolli et al., 2023).
Another study involving 114 patients showed a marked decrease in hepatitis D virus RNA levels with 2mg of bulevirtide. Overall, these
studies demonstrate the effectiveness of bulevirtide in managing hepatitis D (Dietz-Fricke et al., 2023).
Despite the clear efficacy, it is important to note a potential limitation: bulevirtide inhibits replication by way of a middle man. It works because hepatitis D virus depends on hepatitis B virus for cell entry. The latter provides the surface proteins needed to bind and enter host liver cells. Bulveritide interferes with these proteins. But as is becoming increasingly evident, hepatitis D virus is able to transmit by hijacking other viruses as well (see section: “Alternative Envelope Proteins”). Hepatitis B virus may not be the only helper. We need to expand our repertoire of treatment options to combat hepatitis D virus as a whole, not just in conjunction with hepatitis B virus.
What are Viroids? Understanding the World’s Smallest Pathogen
Viruses, bacteria, and fungi; most people will have heard of these three major types of disease-causing microbes. Some will also have heard about protozoa, which are larger and more complex than the aforementioned three. The particularly studious may even know of prions, which are “infectious”, mis-folded proteins that cause neurodegenerative diseases, including mad cow disease. But few will know about the smallest infectious pathogens discovered to date: viroids (Diener et al., 1993).
These biological oddities are to blame for a host of different crop diseases, causing huge losses across many different agricultural industries. In humans, the viroid-like hepatitis D virus (HDV) can cause joint pain, inflammation of the liver, and extreme fatigue. Although people can overcome the acute phase of infection, for many the disease becomes chronic. Coinfection of hepatitis B and D is considered the most severe form of hepatitis, with patients frequently developing serious complications ranging from extreme fatigue to full-scale liver cirrhosis (Hepatitis d-Niddk , n.d.).
So, what are viroids and how do they replicate? What follows is a brief overview of what we know so far.
Every pathogen carries with it a toolkit for infection the types of tools in the kit, and the size of the kit, however, can vary significantly.
Viruses generally travel quite light. They take only what they need and depend on the host cell for the rest. Hijacking the host cell translation system permits viruses to produce the proteins encoded in their genetic material. Some of these proteins will be used to further copy the viral genome, enabling effective replication every virus encodes at least one protein to help with this process. Others give a virus its structure and protective coating. These are necessary for viral entry into host cells. And in some cases, a virus may also carry proteins that help give it a competitive edge over our immune system, letting it go undetected or actively suppressing our defenses. Regardless, the function of the genetic material whether in the form of DNA (deoxyribonucleic acid) or RNA (ribonucleic acid) is to serve as a blueprint for the production of proteins. DNA and RNA do the instructing, proteins do the actual legwork.
Viroids take this lightweight approach to the extreme: they are small, covalently closed circular (ccc) RNA molecules that, crucially, do not encode any proteins. Where a virus such as SARSCoV-2 is made up of nearly 30,000 nucleotides the basic building blocks of DNA and RNA viroids only contain between 220 to 450 nucleotides (Lee & Koonin, 2022; Sah et al., 2020). This is more than five times smaller than the smallest known virus. And since viroids lack a protein “coat” they are completely naked, nothing but RNA.
The minimalism of viroids does come with the drawback of being entirely dependent on passive mechanisms of transmission. Since they lack a protein coat, they do not have any surface proteins and cannot recognize, bind to, and enter healthy cells. The only way for a viroid to get into a cell is if there is pre-existing damage to the cell membrane, affording it an opportunity for entry. Otherwise, it is stuck outside the door.
If viruses rely on their proteins for replication, how is it that viroids manage without? They take advantage of a process called “rollingcircle replication” (Figure 1). This mechanism was discovered by my PhD mentor, Walter Gilbert, and fellow graduate student, David Dressler, in the 1970s (Potter, 2014). For the most part, rolling-circle replication serves to create duplicates of DNA, but viroids and some RNA viruses —neither of which possess any DNA— have managed to appropriate the task for their own benefit.
First, the single-stranded viroid RNA is copied by a piece of hostcell machinery called RNA polymerase II. This is already unusual, since RNA polymerase II traditionally transcribes DNA into RNA, not RNA into RNA. Somehow, viroids manage to subvert this and hijack the enzyme. RNA polymerase II produces a long, fused chain made up of multiple copies of the viroid genome. But since the genomes are fused together, they need to be cleaved at the appropriate places before they are fully functional.
Cleavage of the long, multi-genome chains can happen in one of two ways, and viroids are classified into families depending on the method they use (Figure 2). The first family of viroids, called Pospiviroidae, are rod shaped. These rely on conserved genetic motifs in their RNA being recognized and cleaved by host enzymes current consensus flags RNA polymerase III as the most likely candidate. Once the long chain of viroid genomes has been chopped up into single, free-floating pieces, another host enzyme, called DNA ligase I, fuses the ends of each strand together, shaping them back into their usual circular form. Again, DNA ligase I is actually meant for DNA replication, but the viroids manage to subvert the mechanism to suit their needs.
SOURCE: ACCESS Health International
The second family of viroids, called Avsunviroidae, does not depend on host-cell machinery for cleavage. Instead, these viroids make use of so-called “hammerhead” ribozymes (HHRz). Although this may sound like a protein, it is not; a ribozyme is a strand of RNA that is capable of acting like an enzyme, catalyzing biochemical reactions such as RNA splicing. Rather than simply serving as a set of genetic instructions for protein synthesis, RNA in the form of a ribozyme does the manual labor itself. So, viroids of the Avsunviroidae family carry with them the tools to self-cleave, which is integral to the replication process. Unlike their counterparts, Avsunviroidae have a branched, hammerhead-like shape (Figure 3).
FIGURE 3. The viroid is a single-stranded piece of circular RNA that has an unusual structure due to complementary base pairing. Some form a simple rodlike structure, whereas other viroids have a complex branched structure.
SOURCE: Viruses, Viroids, and Prions CLARK ET AL. 2019
ThisarticleoriginallyappearedinForbeson February17,2023and is available online here: What are Viroids? Understanding the World’sSmallestPathogen
Whether you’re a carnivore, omnivore, or a vegetarian, either you or what you eat depends on crops somewhere along the way. Food systems form the backbone of all societies; no matter how complex or technologically advanced a culture, its people still need to eat. And for now, crops continue to be an integral part of this equation. But as farmers know all too well, crops are susceptible to a range of diseases and issues. As we are quickly finding out, viroids are an especially pesky agricultural scourge. These are small, circular strands of RNA that can replicate and damage host cells all while possessing no proteins of their own. Think virus, but much tinier and much simpler — a virus reduced down to nothing but the essentials.
For the longest time, viroids flew under the radar, known only by the damage they left in their wake. As early as the 1920s, farmers in the United States had been struggling with a mysterious pathogen that rendered their potatoes cracked, dumbbell-shaped, and a fraction of their normal size (Figure 1) (Singh, 2014). Along with the damage, the yield was also lower than usual. And the issue was not restricted solely to the United States, it was more global than that. In the 1960s and 1970s, upwards of half of the potato plants in some states of Ukraine and China were also afflicted by what seemed to be the same disease.
In spite of its pervasiveness, scientists were stumped as to what was causing the issue. They suspected it was viral in nature, but nobody had been able to isolate the culprit. In 1971, Theodor O. Diener, a plant pathologist working in the Agricultural Research Service branch of the U.S. Department of Agriculture, made a breakthrough, but it wasn’t what everyone expected (Viroid : Usda Ars, n.d.). Instead of a virus, he had determined that the disease was being caused by small, circular RNA with no protein coat; virus-like, but not a true virus. He dubbed it a “viroid”.
Since then, viroids have been discovered to infect an assortment of different plants (Table 1), with a geographic distribution that spans
practically all continents. As things stand, we know of 33 different viroid species (Ortolá & Daròs, 2023).
Potatoes
SOURCE: EPPO Global Database
Potato spindle tuber viroid (PSTVd)
Reference
T. O. Diener, 1971
https://doi.org/10.1016/ 0042-6822(71)90342-4
Tomatoes
Tomato apical stunt viroid
Tomato planta macho viroid
Mexican papita viroid (now a strain of tomato planta macho viroid)
Tomato chlorotic dwarf viroid (TCDVd)
Rosemarie W. Hammond, 2017
https://doi.org/10.1016/ B978-0-12-8014981.00001-2
SOURCE: Shanga et al. 2015
Columnea latent viroid
Indian tomato bunchy top viroid (a distinct strain of CEVd)
Eggplants
Eggplant latent viroid (ELVd)
José-Antonio Daròs, 2015
https://doi.org/10.1111/ mpp.12358
Vernière et al., 2007
Citrus Fruits
Citrus exocortis viroid (CEVd)
https://doi.org/10.1094/ PDIS.2004.88.11.1189
SOURCE: Ali et al. 2020
Apples
Citrus bent leaf viroid (CBLVd)
Hop stunt viroid (HSVd)
Citrus viroid III (CVd-III)
Citrus viroid IV (CVd-IV)
Apple scar skin viroid (ASSVd)
Apple fruit crinkle viroid
Apple dimple fruit viroid.
Sharma et al., 2020
https://doi.org/10.1007/s 13337-020-00625-8
SOURCE: Di Serio et al. 2017
Peaches
Peach latent mosaic viroid (PLMVd)
Hop stunt viroid
Di Serio et al., 2018
https://doi.org/10.3390/ v10110612
SOURCE: PestinfoWiki
Pea rs
Pear blister canker viroid (PBCVd) Apple scar skin viroid
Di Serio et al., 2018
https://doi.org/10.3390/ v10110612
SOURCE: Kyriakopoulou et al. 2001
Hemp (Cannabis Sativa)
Hop latent viroid (HLVd)
Bektaş et al., 2019
https://doi.org/10.1094/ PDIS-03-19-0459-PDN
SOURCE: A2LA
Palms (Coconut / Oil Palm Fruit)
Coconut cadangcadang viroid (CCCVd)
Coconut tinangaja viroid (CTiVd)
Rodriguez et al., 2017
https://doi.org/10.1016/ B978-0-12-8014981.00004-8
SOURCE:
Vadamalai et al.
2017
Avocados
Avocado sunblotch viroid (ASBV)
Saucedo-Carabez et al., 2013
https://doi.org/10.1007/s 10658-013-0354-9
SOURCE: SaucedoCarabez et al. 2013
TABLE 1. A list of agricultural crops infected by viroids (not comprehensive). SOURCE: ACCESS Health International
From its humble origins in the Andes, between southern Peru and northern Bolivia, the potato has spread across the world. It is now a staple food for more than a billion people and has risen to become the fourth-most important crop, right behind rice, wheat, and maize (Group, 2020). Yearly, this amounts to more than 300 million metric tons of potato equivalent in weight to around two million blue whales, give or take (Potato | Land & Water | Food and AgricultureOrganizationoftheUnitedNations , n.d.).
So how do viroids impact this agricultural giant? Studies indicate that a potato spindle tuber viroid (PSTVd) infestation can cut yields by as much as 64% (Hammond, 2017). In the saco variety, even mild strains of the viroid can cause a yield-reduction of 24% (Singh et al., 1971). In more severe cases, this number can soar up to 60%. Another study, this one performed in the United States, suggests that as little as 4% of infected crops can lead to a 3% loss in yield (Verhoeven, 2022).
It should come as no surprise that the potato spindle tuber viroid (PSTVd) is listed as a pest in many countries. This is exacerbated by the difficulty of removing the viroid once it has “taken root” contaminated farm equipment, contaminated pollen, and contaminated seeds can all spread the disease onwards. Only strict quarantining and, in worst case scenarios, complete eradication of infected potatoes have proven successful. And although some countries have managed to fully eliminate potato spindle tuber viroid (PSTVd) infection in potatoes, the top four producers
China India, Russia, and Ukraine continue to grapple with the issue (Verhoeven, 2022).
Avocados
Viroids are also bad news for America’s favorite fruit, the avocado (Ferdman, 2015). Since 2010, avocado consumption has more than doubled, from 1300 million pounds to upwards of 3000 million pounds in 2021 (Shahbandeh, 2022). And that is in the United States alone. The avocado craze has made its way to East Asia as well, with South Korea especially partial to the creamy, green fruit. Like the potato, however, avocados are prone to viroid infection. Indeed, there’s a viroid that specifically targets avocados: the avocado sunblotch viroid (ASBV).
Despite being the smallest known viroid, it causes extensive damage. Avocados from viroid-infected trees often suffer from discoloration, their skin turning yellow, white, or even red. Such discoloration is usually accompanied by scarring of the skin or in the fruit itself. Fruit from infected trees is often also significantly smaller than fruit from their uninfected counterparts, known as dwarfing. Some trees are symptomless which, although may sound like a good thing, is actually quite dangerous, as farmers can unwittingly transmit viroids from the symptomless trees to healthy trees. As with potatoes, getting rid of viroids in an avocado orchard is difficult and may require full eradication of all trees a complete restart.
As far as economic losses are concerned, avocado sunblotch viroid (ASBV) is merciless. A study from Mexico the largest global producer of avocados found that symptomatic Hass avocado trees can suffer a 75% reduction in total fruit weight (Saucedo-Carabez
et al., 2014). This loss was more pronounced still in Mendez avocados, with a 83% reduction in yield. Even asymptomatic trees produced a loss in yield, with a reduction of between 30% to 58%, depending on the variety.
Palm oil. You’ve heard of it, I’ve heard of it, everyone’s heard of it. That’s because it’s practically ubiquitous at this point, present in everything from shampoos and cosmetics to foods and biodiesel. Its appeal lies in its versatility, and its versatility has seen it quickly rise to top-spot, now far and away the most common vegetable oil (Ritchie & Roser, 2021).
As the name suggests, the oil is derived from a type of palm tree called African oil palm. These palms are susceptible to one of the nastier viroids, the coconut cadang-cadang viroid (CCCVd).
Infected oil palms develop a condition known as orange spotting (OS) which significantly stunts growth and yield. Palms afflicted with orange spotting produce 25%-50% less fruit, leading to an estimated yearly loss of $25.6–256.2 million in Malaysia alone (Rodriguez et al., 2017).
Cadang-cadang disease also impacts coconut palms, where it is extremely lethal; the name is derived from the Bikol word “gadangadan”, which means dead or dying. In the Philippines, the world’s largest producer of coconuts, cadang-cadang has led to the death of 40 million palm trees over the past decade, with an estimated economic cost of $4 billion USD (Vadamalai et al., 2017).
The cannabis industry is one of the fastest-growing industries in the United States, worth $26.5 billion in 2022 (Morrissey, 2022). With more States likely to shift to an adult-use, or recreational, cannabis status, this number is projected to soar to $70 billion by 2030. This increase is driven by an uptick in consumers, again predicted to steadily increase by around four percent every year, potentially hitting 70 million by 2030 (50+ Cannabis Industry Statistics for 2023|Embroker,2023).
All this demand requires supply, and as cannabis farmers have found out, the hemp crop is not immune to viroids. Although not its primary host, hop latent viroid (HLVd) can infect hemp plants, leading to many of the same symptoms viroids have come to be known for: diminished growth, lower yield, discolored leaves, and brittle stems (Warren et al., 2019). But it’s not only the yield quantity that is affected, yield quality also suffers; the few cannabis flowers produced by infected plants are dull in smell and low in oils. As a result, infection by viroids has come to be known as the “dudding disease”.
To determine the scope of the issue, a leading cannabis genetics company performed over 200,000 tissue tests for 100 different growers between 2018 and 2021. They discovered that for 90% of cultivation sites, 33% of the tests they took came back positive for hop latent viroid (HLVd) infection. The impact? More than $4 billion annual losses for growers (Adams, 2022).
Speaking to Globe Newswire, Dr. Bryce Falk, an esteemed plant pathologist working at the University of California, Davis, mentions:
“Hop Latent Viroid is perhaps the greatest threat to the legal
cannabis industry in the United States. It is very difficult for growers to identify due to its latency and it can spread undetected within a grow, wiping out much of the commercial value. For cannabis to achieve its potential as a commercial agricultural crop, the industry needs this type of large scale testing and treatment platform.” (Dark Heart Data Shows Hop Latent Viroid Drives $4b Annual Losses to Legal Cannabis Crop, 2021)
This articleoriginallyappeared in Forbes on March1, 2023 and is availableonlinehere:Viroids,AFarmer'sNightmare
Viroids are small, circular slivers of ribonucleic acid (RNA) that do not encode any proteins. Despite this, they can self-replicate and damage hosts. Think of proteins as tools: they have specific functions that help achieve some end goal. Most viruses, for example, make use of proteins not only to enter host cells, but also to replicate once inside. Some of the nastier viruses also use proteins to evade or even suppress host immune responses. How do viroids cause disease without any help from proteins? Although the exact mechanisms of damage remain elusive, a process known as RNA interference (RNAi) is thought to play a central role.
Many plants and animals use RNA interference (RNAi) as a means of regulating gene expression it helps them switch certain genes, and by extension proteins, “off.” (Agrawal et al., 2003) Genes encode information, and when genes are expressed, this information is turned into a functional product with a specific purpose. Modulating which genes are expressed, and when they are expressed, helps organisms keep their vital functions in balance. For example, gene expression of insulin increases following a meal in response to a spike in blood sugar; insulin acts as a key that allows blood sugar to enter our cells, where it can be used as energy. Depending on the meal, more or less insulin may be produced.
Gene expression is also vital to successful immune responses. During infection, the host immune system is constantly upregulating increasing the expression of and downregulating decreasing the expression of a variety of different proteins. These proteins contribute to inflammation, help direct immune cells to where they need to be, and so on. But this is a very delicate
dance; too much inflammation can be just as damaging as too little inflammation, for instance. So organisms are constantly modulating gene expression in order to keep things “just right”.
RNA interference, as mentioned, is one mechanism of gene expression. How does it work? Broadly speaking, small snippets of RNA shut down protein synthesis by binding to, and interfering with, the messenger RNAs that code for those proteins. The slivers of RNA that enable the gene silencing can be broken down into two classes: microRNA (miRNA) and small interfering RNA (siRNA). Both of these small RNA types are usually between 20 and 24 nucleotides long tiny. For comparison, SARS-CoV-2 is made up of roughly 30,000 nucleotides (Sah et al., 2020). MicroRNAs are derived from a double-stranded region of a hairpin-shaped precursor (Figure 1), whereas small interfering RNAs are generated from longer regions of double-stranded RNA (dsRNA) (MacFarlane &
Despite the subtle differences between the two types, the general pathway through which microRNAs and small interfering RNAs induce gene silencing remains the same (What Is Rnai - Rnai
Biology , 2013). First, their precursors —pre-microRNA and doublestranded RNA, respectively — are ferried from the nucleus into the cytoplasm of the cell. Once there, the precursors are cut into siRNAs or miRNAs by a protein called Dicer. Next, the small RNA pieces are incorporated into something called the RNA-induced silencing complex (RISC). RISC uses miRNA or siRNA as a template and begins to search for a complementary strand of messenger RNA. After recognizing and binding to the complementary messenger RNA, the complex can interfere with gene expression in two ways: it either uses a protein called Argonaute to “slice up” the mRNA, after which it gets degraded by the cell, or it simply inhibits mRNA from being translated by physically blocking the machinery used to do so (Figure 2).
FIGURE 2. A schematic diagram of RNA interference, either through cleavage of messenger RNA (right) in the case of a perfect match, or through physical inhibition (left) in the case of an imperfect match.
SOURCE: ACCESS Health International (Adapted from: National Library of Medicine, NIH)
MicroRNAs generally do not perfectly match up to messenger RNA strands; because they are not specific to any one mRNA, they can inhibit many different, sequentially-similar messenger RNAs. In contrast, small interfering RNAs are highly specific, each binding to and silencing only one particular messenger RNA target. Regardless, both successfully silence gene expression.
Immune systems and pathogens are involved in a constant, ongoing chess match: immune systems are exposed to pathogens, develop mechanisms to block and destroy them, and the pathogens, in turn, “learn” to evade these mechanisms. Sometimes, pathogens manage to use those selfsame defense mechanisms to their advantage. Although RNA interference is generally used by plants to target and suppress foreign RNA, viroids may in fact be hijacking the process, using it to instead damage their hosts.
All of this begins with a case of mistaken identity. Recall that RNA interference makes use of small interfering RNAs and microRNAs, both of which are derived from precursor double-stranded RNA. During replication, viroids depend on an intermediate step where their RNA briefly takes on a double-stranded structure. As such, the viroid RNA may erroneously be recognized by Dicer as a precursor and chopped up into small fragments, called viroid short RNAs (vsRNAs). Given their similarity to the small RNAs used for gene silencing, viroid short RNAs may get incorporated into RNAinduced silencing complex (RISC) and, from there, interfere with host gene expression (Figure 3).
FIGURE 3. Cutting
by the nucleases Dicer or Drosha releases the small regulatory RNAs—miRNA. Cutting of viroid RNA by the same nucleases releases small RNA molecules vsRNA. These mimic the action of miRNA. The RISC complex helps both vsRNA and miRNA to bind to complementary sequences on target messenger RNAs. This alters the expression of the mRNA. In the case of the viroid, expression is abnormal and disease results. SOURCE: ACCESS Health International (Adapted from: “Viruses, Viroids, and Prions” CLARK ET AL. 2019)
Evidence for viroid-induced RNA interference comes from a number of sources. For one, deep sequencing of viroid short RNAs from tomato plants infected with potato spindle tuber viroid (PSTVd) has shown that most viroid short RNAs are derived from a part of the viroid known to be related to disease symptoms, called the pathogenicity domain (Diermann et al., 2010). Another study found that proteins associated with disease resistance in plants — serine/threonine kinases— were negatively affected by viroid
infection (Adkar-Purushothama & Perreault, 2018). Importantly, exposing plants to just the viroid short RNAs, without the rest of the viroid, was enough to cause damage similar to that seen during infection. Similarly, researchers have found that infection with the tomato planta macho viroid (TPMVd) leads to the downregulation of gene networks associated with plant development and growth (Aviña-Padilla et al., 2018).
Although additional mechanisms of viroid-induced disease may still surface, current evidence suggests RNA interference, and the subsequent downregulation of host genes, plays a central role.
ThisarticleoriginallyappearedinForbeson March10,2023andis available online here: No Proteins, No Problem: Viroids Cause DiseaseBySilencingRNA
Virusoids: Viruses’ Very Own Parasites
Greatfleashavelittlefleasupon theirbacksto bite'em,Andlittle fleashavelesserfleas,andso adinfinitum.Andthegreat fleas themselves,inturn, havegreater fleastogo on; Whiletheseagain havegreaterstill,andgreaterstill,andso on.
Viroids are tiny strands of circular, infectious RNA. They differ from viruses in that they do not have a protective protein coat and that they do not encode any proteins of their own. They are also much, much smaller than the average virus. Think of viroids as extreme minimalists, traveling as lightweight as possible. Viruses are generally more complex, with larger molecular “toolboxes” — all viruses produce at least one protein, but most produce a whole array of them, used for replication, and in some cases, suppression and evasion of the host immune system.
Virusoids, in turn, straddle the line between viroids and viruses: like viroids, they are small circular RNAs that, for the most part, do not encode any proteins; like viruses, they are encapsidated in a protein coat, albeit not of their own making. The hallmark difference between viroids and virusoids is that the latter cannot transmit between hosts and host cells without assistance from a helper virus, which provides it with its protein coat and the necessary
“machinery” for infection. So in order for a virusoid to infect a host, it needs to be carried in “upon the back of” a virus. If there is no helper virus to hitch a ride on, the virusoid is stuck outside, with no way into the host and no way to replicate. Curiously, virusoids share little to none of their genetic material with their helper viruses, which means they evolved independently of them and are not simply maladapted offshoots. This clearly differentiates them from another type of subviral agent, defective interfering particles (DIPs), which are small mutants of a parent virus generated in moments of defective replication.
To summarize, virusoids (also called “viroid-like satellite RNAs”) are circular, single-stranded RNA molecules that exhibit the following traits (Rao & Kalantidis, 2015): “(1) satRNA parasitizes on helper virus (HV) replication machinery (2) satRNA is encapsidated by the HV capsid protein to promote transmission and (3) satRNA and the HV genomic RNAs have no nucleotide sequence similarity.”
Virusoids can be separated into groups according to the genus of their helper virus (Table 1). In plant virusoids, five are linked to the sobemovirus genus, three are linked to the nepovirus genus, and one is linked to the polerovirus genus. In animals, we know of multiple viroid-like satellite RNAs, all of which resemble the human virusoid hepatitis D virus.
Arabis mosaic virus small satellite RNA
Tobacco ringspot virus satellite RNA (satTRsV)
Chicory yellow mottle virus satellite RNA (satCYMoV)
Solanum nodiflorum mottle virus satellite RNA
Subterranean clover mottle virus satellite RNA
Velvet tobacco mottle virus satellite RNA
Lucerne transient streak virus satellite RNA (satLTSV)
Rice yellow mottle virus satellite RNA
Cereal yellow dwarf
Arabis mosaic virus
Nepovirus 300–301 Kaper and Collmer (1988)
https://doi.org/10.101
6/0006291X(88)90687-0
Tobacco ringspot virus
Nepovirus 359 Buzayan et al. (1986) https://doi.org/10.107 3/pnas.83.23.8859
Chicory yellow mottle virus
Nepovirus 457 Rubino et al. (1990)
https://doi.org/10.109 9/0022-1317-71-91897
Solanum nodiflorum mottle virus
Subterranean clover mottle virus
Velvet tobacco mottle virus
Sobemovirus 377 Haseloff and Symons (1982)
https://doi.org/10.109 3/nar/10.12.3681
Sobemovirus 332 and 328 Davies et al. (1990)
https://doi.org/10.101
6/00426822(90)90475-7
Sobemovirus 366 Haseloff and Symons (1982)
https://doi.org/10.109 3/nar/10.12.3681
Lucerne transient streak virus
Sobemovirus 322 and 324 Keese et al. (1983) https://eurekamag.co m/research/017/474/0 17474103.php
Rice yellow mottle virus
Sobemovirus 220 Collins et al. (1998) https://doi.org/10.100
6/viro.1997.8962
Cereal yellow dwarf virusRPV
Polerovirus 322 Miller et al. (1991) doi: 10.1016/00426822(91)91000-7
virus-RPV satellite RNA
Hepatitis Delta Virus (HDV)
Hepatitis B Virus (HBV)
Orthohepad navirus ~ 1,679 Alves et al. (2013) doi: 10.1155/2013/560105
TABLE 1. Overview of known virusoids (“viroid-like satellite RNAs”); sc-satRNA = small circular satellite RNA; nt = nucleotides.
SOURCE: Adapted from Navarro et al. (2017), “Chapter 61 - Small Circular Satellite RNAs”
Like viroids, virusoid replication is a three-step affair, making use of the rolling-circle mechanism (Davies et al., 1990). First, the circular RNA is replicated into a long chain of RNAs containing multiple copies of the original genome; helper virus polymerases are likely hijacked to this end. Second, the long chain of “oligomeric” RNAs to use technical speak is then cut up into its individual units by host enzymes or, occasionally, by ribozymes encoded in the virusoid genome. Ribozymes are RNA sequences that, despite not encoding a protein, are “active” they can themselves perform catalytic tasks, such as cleavage, usually expected of protein enzymes. Finally, the individual strands of RNA have to be circularized again to fully form the virusoid particles. Known as ligation, this takes place either through the appropriation of host enzymes, or in rare instances, through self-ligation catalyzed by the ribozyme.
Replication can take place in one of two ways: symmetric or asymmetric (Figure 1). The circular genome that viroids and virusoids start out with is positive-sense (+). During rolling-circle replication, however, the RNA is copied into a negative (-) strand
the mirror image of the original. In order to get the negative-strand RNA back to its original sense, it has to be copied a second time, flipping the mirror image again. How the negative strand is copied back to the positive sense is what differentiates symmetric from asymmetric replication. In symmetric replication, this happens via a second instance of rolling-circle replication, aided by ribozyme cleavage. In the case of asymmetric replication, the negative-sense RNA gets copied back to its positive polarity through the help of host enzymes, which also serve to cleave the RNA into its unit-length strands.
FIGURE 2. Overview of viroid replication. On the left, an example of potato spindle tuber viroid (PSTVd) replication, which belongs to the pospiviroidae family. On the right, an example of avocado sunblotch viroid (ASBVd) replication, which belongs to the avsunviroidae family. Plus and minus strands are indicated in green and red, respectively. Cleavage is catalyzed either by host enzymes (pospiviroidae) or by hammerhead ribozymes (avsunviroidae)
SOURCE: ACCESS Health International
Although plant virusoid replication follows this general process, there are some individual nuances. Flores et al. note that three virusoids from sobemoviruses replicate asymmetrically, and although they contain ribozymes, they do so only in the positive strand (Flores et al., 2011). The remaining two sobemovirus virusoids replicate via the symmetrical rolling-circle pathway and contain ribozymes in both the negative and positive strand. The virusoids associated with nepoviruses also replicate symmetrically, but they make use of two different classes of ribozymes, depending on the strand polarity: so-called “hammerhead” ribozymes in the positive strand and hairpin ribozymes in the negative strand. The polerovirus virusoids, on the other hand, have a hammerhead ribozyme but it is blocked in the unit-length RNA; only when the RNA has been copied into a long oligomeric chain does it become unblocked and can it help mediate self-cleavage.
Unlike viroids, which are split into two families depending on the site of their replication, either the chloroplast or the nucleus, virusoids most likely replicate in membranous vesicles connected to the cytoplasm. Hepatitis Delta virus follows a different replication pathway, which will be covered in a later piece. This articleoriginallyappeared in Forbes on April 3, 2023 and is availableonlinehere:Virusoids:Viruses’VeryOwnParasites
It’s axiomatic in reviews of virusoids that they are small circular RNAs that do not encodeany proteins . Studies with the virusoid known as small circular satellite of rice yellow mottle virus (scRYMV) challenge this notion: not only does the virusoid produce proteins from its positive (+) strand RNA, it may also produce proteins from its negative (-) strand RNA, which is used as a template during replication. This opens the much broader possibility that other virusoids produce peptides and proteins of varying descriptions. As we shall soon see, that is certainly true of the virusoid most directly involved in human disease, the hepatitis delta virusoid.
Viroids and virusoids are some of the smallest transmissible pathogens discovered thus far. They are tiny strands of circular RNA roughly between 220 and 450 nucleotides long — for reference, SARS-CoV-2 clocks in at 30,000 nucleotides. What sets the two apart is the fact that virusoids require a helper virus to infect and replicate, whereas viroids do not. Helper viruses also provide virusoids with a protein coat, which helps keep them safe and facilitates entry into host cells. Some viroids and most virusoids have the unusual feature that their RNA includes a section that has catalytic activity, known as a ribozyme. Essentially, it is RNA that, instead of encoding a protein to do a job, does that job itself. In the
case of viroids and virusoids, the ribozymes help cleave and occasionally rejoin the circular RNA genomes during replication.
When Abouhaidar et al. first discovered and described the virusoid associated with rice yellow mottle virus, they noticed that it contained various possible open reading frames (ORFs) in its genetic sequence (Collins et al., 1998). Open reading frames are segments of RNA that have the potential to be translated into proteins. They are flanked on one side by a “start codon” and on the other end by a “stop codon”. These codons, each made up of three nucleotides, help tell the ribosome the protein-producing machinery in cells where to begin and where to end. The RNA between the two codons is turned into a protein.
Virusoids replicate via rolling-circle replication. Replication of this kind involves the formation of a long chain of negative (-) strand RNAs, known as a concatemer. Each negative-strand RNA is a mirror image of the original, positive-strand genome. The long chain of negative-strand RNAs is then cut up into unit-length segments, either by the ribozymes contained within the virusoid RNA or by host and viral polymerases. Finally, the negative strands are then used as templates for the production of new positive (+) strands, completing replication.
Abouhaidar and his colleagues found that the (-) strand contains three small open reading frames. The (+) strand also contains two or even three, totally overlapping open reading frames. Curiously, the start codon in the positive strand, made up of the three nucleotides AUG, is embedded right between two stop codons:
UGAUG A. Changing reading frames switches the sequence from a start codon to back-to-back stop codons: UGA UGA.
Another peculiarity of virusoids is that they lack clear directionality. Most protein-encoding RNA is linear , with two distinct sides: a fiveprime end and a three-prime end. Ribosomes orient themselves by binding to the five-prime end and scanning the RNA until they hit a start codon, at which point they begin translation. Translation comes to an end once they hit a stop codon. Since virusoids are circular , their two ends are fused together, making the usual approach impossible for ribosomes. Even if they occasionally take on a linear form during replication, they still do not have a fiveprime and three-prime end. Instead, the small circular satellite RNA of rice yellow mottle virus likely has an internal ribosome entry site (IRES) think of this as an artificial docking station that grants the ribosome access to the circular genome. This is not without precedent, as many RNA viruses make use of internal ribosome entry sites (IRES) to initiate translation independently of a fiveprime cap, skipping over the scanning step (Mailliot & Martin, 2018) (Figure 1).
Simply because a sequence contains a potential reading frame does not necessarily mean it actively encodes any proteins. To find out, Abouhaidar et al. generated a linear copy of the putative (+) strand open reading frame by joining three strands of the unit-length RNA head-to-tail, thus mimicking the overlapping reading frames present in the virusoid (AbouHaidar et al., 2014).
The RNA construct was then inserted into an Escherichia coli (E. Coli) cell. If the reading frame were operational, you would expect the ribosomes in the E.Colicell to translate the RNA sequence and produce a corresponding protein. Indeed, a 19-kilodalton (kDA) protein was made. The amino-acid sequence of this 19-kDA protein
mostly matched the predicted amino-acid sequence of the virusoid RNA.
Next, the 19-kDa protein was used to raise specific antibodies that would recognize and bind to the protein whenever it was present. The researchers then invitrotranslated the RNA construct and used the antibodies to double check whether the protein had been synthesized. The antibodies revealed two distinct proteins, one weighing 19 kDa and the other weighing 16 kDa. Based on the amino-acid sequence of the 16-kDa protein, translation must have started at the initiation-termination site of the first virusoid RNA monomer and must have ended at the initiation-termination site of the last virusoid RNA monomer (Figure 2). Removing the last virusoid RNA monomer led to the loss of the 16-kDA protein but did not interfere with the synthesis of the 19-kDa protein, which began initiation outside of the first virusoid RNA monomer.
FIGURE
Small Circular Satellite RNA of Rice Yellow Mottle Virus. SOURCE: ACCESS Health International (Adapted from “Novel coding, translation, and gene expression of a replicating covalently closed circular RNA of 220 nt”, Abouhaidar et al. 2014)
The next step was to determine whether the 16-kDa protein was made in plants infected with the virusoid. To this end, total viral RNA was extracted from the plants, translated, and then exposed to the virusoid-specific antibodies raised in earlier experiments. The same 16-kDa protein was present (Figure 3). Supplementing total viral RNA with additional purified circular RNA generated a much stronger signal of the 16-kDa protein. In contrast, total RNA extracted from healthy plants did not generate any such proteins.
FIGURE 3. In vitro translation reaction depicting the 16-kDa protein from the scRYMV circular RNA (purified from denaturing polyacrylamide gels) is shown in lane 3, whereas that of the reaction from total RNA of RYMV-infected rice is shown in lane 2. Lane 5 demonstrates the 16-kDa product from reaction using total viral RNA extracted from RYMV virus particles, and lane 4 shows the enhanced 16-kDa signal from reaction using RYMV total viral RNA but supplemented with the same amount of gel-purified circular RNA as that used in the reaction of lane 3. Lanes 1 and 6 represent negative control reactions using healthy rice and the endogenous empty lysate, respectively. Arrows on the right depict the position of molecular weight markers 25, 16, and 14 kDa from top to bottom, respectively. SOURCE: ACCESS Health International (Adapted from: Abouhaidar et al. 2014)
The 16-kDa protein produced by the small circular satellite RNA of rice yellow mottle virus requires a ribosome to loop around its RNA twice. The reason the ribosome doesn’t stop after the first loop is because the virusoid RNA is 220 nucleotides long, which is not a multiple of three. Since each codon is made up of three nucleotides, after one full loop, instead of hitting the stop codon, the ribosome slips into a new reading frame and continues on, now off by one nucleotide compared to the first loop. This one nucleotide difference in reading means new amino acids are synthesized during the second loop (Figure 4). It also means the ribosome now hits one of the stop codons in the initiationtermination sequence, which it missed the first time around (Figure 5).
Along with the 16-kDa protein, translation of total viral RNA from infected plants also yielded larger proteins: 18 kDa, 23 kDa, 32 kDa, and even 39 kDa. The researchers suspect these are “read-through” proteins, formed when the ribosome does not stop at the initiationtermination codon after the second loop, but instead keeps going around the circular RNA of the virusoid, shifts into a different reading frame, and eventually hits another stop codon. Sometimes even these secondary or tertiary stop codons may prove to be “leaky”,
in which case the ribosome continues on until it hits the next reading frame and continues translation. For example, the 32-kDa protein seemed to be a perfect dimer of the 16-kDa protein.
translation. Two loops (red spiral) marks the production of the 16-kDa protein. Two loops plus 18 additional amino acids marks the production of the 18-kDa read-through protein (red + green). Three full loops marks the production of the 23-kDa protein (red + green +blue). Full amino-acid sequence:
MSQEELGGTQEFHPGRPGRLGAVHRASLWSEPGLQGAWRRNRSVGT TRTISHRAPAASSCAGGGDFVSSLTDTDE –RGTWRHPGISPGSTWAARSRAQGVAVERAWPPRGLEAKPVCWDHSDH QSSCSGSFQLRRGRRFCFEPYRH – AKRNLEARPNFTRVDLGG –EPCTGRRCGASLASKGPGGETGLLGPLGPSVIVLRQLPAAQGAEILFRAL PT SOURCE: ACCESS Health International (Adapted from Abouhaidar et al. 2014)
As mentioned above, the negative (-) strand of the virusoid RNA also contains open reading frames. Whereas the positive (+) strand only has one clear initiation codon across all three reading frames, the negative strand contains two possible start codons, one in the first reading frame and one in the third reading frame. Although the researchers did not address the negative strand, there is no clear
reason why it shouldn’t also produce functional proteins. All the necessary components are in place. Barring experimental data, we cannot draw any firm conclusions, but it is possible that the negative strand encodes a long, 72-amino-acid sequence. This sequence may begin in the third reading frame before frameshifting into the second sequence and eventually hitting a downstream stop codon (Figure 6).
ACCESS Health International.
Here, we described the proteins and extraordinary coding capacity of a virusoid known as small circular satellite RNA of ring yellow mottle virus (scRYMV). This opens the much broader possibility that other virusoids produce peptides and proteins of varying descriptions. As we shall soon see, that is certainly true of the
virusoid most directly involved in human disease, the hepatitis delta virusoid.
This articleoriginallyappeared in Forbes on April 3, 2023 and is available online here: Contrary To Conventional Wisdom, Some VirusoidsDoProduceProteins
In 1977, a gastroenterologist by the name of Mario Rizzeto, working out of the University of Turin, Italy, discovered the first evidence of hepatitis Delta virus (Rizzetto et al., 1977). While caring for chronically ill hepatitis B patients, Rizzetto and his colleagues noticed something unusual during routine liver biopsies: in some samples, antibodies against the hepatitis B core antigen (HBcAg) were reacting even when there was no hepatitis B core antigen present. Clearly something was triggering an immune reaction. The antibodies would only react in samples which contained the hepatitis B surface antigen (HBsAg); samples containing the core antigen but no surface antigen did not bring about any response. Further experiments revealed that the mystery antigen was also distinct from the hepatitis B e antigen (HBeAg) — samples containing the surface antigen and the mystery antigen did not react when anti-e antibodies were added to the mix. Having discounted all known antigens, the culprit was given the name of δ (delta). It is now referred to as hepatitis delta antigen (HDAg).
Two years later, in 1980, Mario Rizzetto and his team returned with new findings (Rizzetto et al., 1980). By studying blood samples of chimpanzees positive for the delta antigen, they noticed that, along with the hepatitis B surface antigen, delta was also associated with a small, low-weight RNA. Although they could not yet confirm it at
the time, they had succeeded in identifying the genetic material of the hepatitis Delta virus.
That same year, in a follow-up study, the delta agent was proven to be transmissible, albeit only in the presence of hepatitis B virus (Rizzetto et al., 1980). By 1986, the RNA associated with the delta antigen had been cloned and fully sequenced, giving researchers an in-depth look at the genome (Wang et al., 1986). There was no overlap in the genetic sequence of the hepatitis Delta virus RNA (HDV RNA) and that of hepatitis B virus, on which it relied for transmission. This suggested that the delta agent was not simply a defective interfering particle (DIP) a mutant snippet of a parent virus that forms as a result of defective replication but instead its very own entity. Nor was it a classic example of a virus, given its small and circular RNA. Despite this, it was given the name hepatitis D virus (HDV).
Hepatitis D virus more closely resembles viroids and virusoids than traditional RNA viruses: it is a small single-stranded circular RNA, it has a double-stranded rod-like secondary structure, and it encodes ribozymes (Figures 1 & 2). Like virusoids, it also depends on a helper virus for transmission and infection at least in humans.
up
roughly 1700 nucleotides. 74% ribonucleotides form consecutive base-paired regions, interspersed by small loops or bulges formed by the unpaired ribonucleotides. All ribonucleotides fold into a quasi-double-stranded, unbranched rod-like structure. SOURCE: ACCESS Health International
Hepatitis D virus also contains an open reading frame, which is a section of RNA that can encode a protein. Although virusoids are not usually thought to produce any proteins of their own, there are exceptions to the rule. In fact, hepatitis D uses this open reading frame to produce three different versions of its antigen protein: a
large one, a medium one, and a small one. Each of these versions has slightly different functions in the hepatitis D lifecycle.
The large and the small hepatitis D antigens bind to the genome to form a ribonucleoprotein (RNP) complex; the final result resembles beads (the antigens) on a string (the RNA). This ribonucleoprotein complex is then encapsulated in the envelope protein of hepatitis B virus, offering it protection and enabling entry into host cells (Figure 3).
FIGURE 3. Illustration of a hepatitis D virion. Abbreviations: ssRNA, singlestranded RNA; S-HDAg, small hepatitis D antigen protein; L-HDAg, large hepatitis D antigen protein; S-HBsAg, small hepatitis B surface antigen; MHBsAg, medium hepatitis B surface antigen; L-HBsAg, large hepatitis B surface antigen. SOURCE: Ypna, CC BY-SA 4.0
<https://creativecommons.org/licenses/by-sa/4.0>, via Wikimedia Commons
Viral hepatitis is a potentially life-threatening disease characterized by inflammation of the liver. It is caused by the hepatitis viruses, of
which there are five main strains: A, B, C, D, and E. Hepatitis A and E are primarily spread through the gut, when food or water contaminated by an infected person’s stool is ingested. Even the tiniest, trace amounts can cause illness. A and E also mainly tend to cause acute disease, which usually resolves after four to eight weeks. The remaining three strains B, C, and D are most commonly transmitted through contact with the blood of an infected person. This can happen in a number of ways: sharing needles or other items (razors, toothbrushes, etc.) with an infected individual, contact with open wounds from an infected person, unprotected sex with an infected person, and, albeit rarely, birth from an infected mother. Hepatitis B, C, and D are also more likely to cause chronic infection, leading to severe liver cirrhosis and liver cancer. Despite the severity of the disease, many chronic hepatitis infections remain asymptomatic for extended periods; by the time you realize something is amiss, the disease has already put down deep roots. When symptoms do appear, it is usually in the form of fever, tiredness, nausea, abdominal pain, vomiting, and occasionally jaundice (yellowing of the skin and eyes) and confusion.
A 2021 report by the World Health Organization (WHO) estimates that 350 million people globally are living with either chronic hepatitis B or C infections (296 million with hepatitis B; 58 million with hepatitis C) (GlobalProgressReport on HIV, ViralHepatitis and Sexually Transmitted Infections, 2021, 2021). As mentioned above, in humans hepatitis D virus relies on hepatitis B as its helper virus, using it to transmit between hosts and to infect cells. This means all cases of hepatitis D are either co-infections, which happens when the two viruses infect an individual simultaneously, or super-infections, which happens when someone already infected
with hepatitis B contracts hepatitis D (Figure 4). Because of these very particular conditions, hepatitis D infections are rarer. Still, they are generally thought to affect around 15-30 million people, or nearly 5% to 10% all people with chronic hepatitis B infections (Wedemeyer & Negro, 2019). A recent meta-analysis suggests the actual number may be far higher, somewhere in the range of 67-72 million people (Chen et al., 2019).
FIGURE 4. HDV-HBV co-infection or HDV super-infection of a chronically infected HBV patient can progress towards viral clearance or chronic infection. Blue and red arrows demonstrate the major clinical outcome upon co- or superinfection, respectively. SOURCE: ACCESS Health International (Adapted from: Tseligka et al. 2021 https://doi.org/10.3390/v13050778)
Although less common than its helper virus, hepatitis D infection is much more severe. Superinfection accelerates disease progression in up to 90% of people, leading to liver cirrhosis onset almost a decade earlier than in cases of hepatitis B infection alone (What Is Hepatitis d - Faq | Cdc, 2020). In general, those suffering from chronic hepatitis D infection experience liver failure within five to
ten years, and 15% of people will experience liver failure as quickly as one or two years after initial infection. People who acquire hepatitis B in childhood, in contrast, usually do not have issues with cirrhosis until roughly 40 years after infection. In fact, around 18% of the cirrhosis and 20% of the liver cancer associated with chronic hepatitis B are likely to be caused directly by hepatitis D virus (Stockdale et al., 2020).
Since hepatitis D virus depends on hepatitis B virus for transmission, their geographic distribution overlaps significantly. And although they can both be found worldwide, there are some clear hotspots, including Western and Middle Africa, Eastern Europe, the Mediterranean Basin, and Central Asia (Mentha et al., 2019) (Figure 5). The Republic of Moldova and Mongolia, in particular, have the highest rates of hepatitis D — up to 60% of chronic hepatitis B patients in Mongolia are also positive for hepatitis D.
SOURCE: By Mario Rizzetto, University of Torino, Italy -
v:WikiJournal_Preprints/Epidemiology_of_the_Hepatitis_D_Virus Mario
Rizzetto, licensed CC-BY, CC BY 4.0, https://commons.wikimedia.org/w/index.php?curid=84135496
We know that hepatitis D virus is responsible for the most severe form of hepatitis, but we still don’t know exactly how it causes such damage. Currently, there are two dominant theories. The first posits that damage to the liver is caused directly by the hepatitis D virus antigen (HDVAg). The second posits that, as is the case with other viral hepatitis infections, liver damage is primarily a result of our own immune response to the virus. Note that these two views needn’t be mutually exclusive; it could be that both play a role. Indeed, it may be that the former is the primary mechanism of action during acute hepatitis D infections, whereas the latter occurs during chronic infections (Abbas & Afzal, 2013).
Evidence for direct damage comes primarily from studies performed in test tubes, known as invitro studies. When exposed to the hepatitis D virus antigen, human liver cells suffer a serious reduction in the rate of RNA synthesis (Cole et al., 1991). Over time, this leads to cell death cells depend on RNA for the production of proteins, which perform vital functions required for the well being and longevity of the cells. Under the microscope, the antigen-exposed liver cells look exactly like the liver cells of individuals suffering from a hepatitis D infection. And the amount of antigen in the dead cells matches the amount of antigen seen in the liver cells of chimpanzees suffering from acute hepatitis D infections. Research in chimpanzees and some human case studies have yielded similar findings (Canese et al., 1984; Lefkowitch et al., 1987). However, a long-term study of transgenic mice found no
evidence of direct liver damage from exposure to the hepatitis D virus antigen (Guilhot et al., 1994).
If not from direct injury via the viral antigen, it is likely that liver damage seen in hepatitis D infections is instead a byproduct of immune responses intended to clear the pathogen (Abbas & Afzal, 2013). Once hepatitis D virus enters our cells, it triggers an inflammatory response, rallying various immune cells and signaling proteins together to combat the infection. These immune cells destroy infected cells in an attempt to stop the spread of hepatitis D virus. Think of inflammation as a necessary evil: immune cells damage their own host tissue in the short run so that they can eliminate a pathogen in the long run. If the pathogen is successfully eliminated, inflammation subsides and the damaged areas can recover. The problem with chronic hepatitis D virus is that our immune system fails to fully clear the infection, leading to a continuous state of inflammation. Since hepatitis D exclusively infects liver cells, the extended inflammatory response slowly wears down the liver as a whole. The large hepatitis D virus antigen (LHDVAg), in particular, has been associated with the activation of transforming growth factor-β (TGF-β) cascades (Choi et al., 2007).
Transforming growth factor beta is a well-known signaling protein involved in the regulation of various important cellular processes. But dysregulation or overexpression can lead to serious issues, including fibrosis and cirrhosis, especially in the liver (Fabregat et al., 2016).
It was generally understood that hepatitis D virus depends entirely on hepatitis B virus for initial infection. It was also understood that,
like the other hepatitis viruses, delta exclusively infects liver cells. Novel research indicates this may not be so. Published in Nature Communications , the work revealed that hepatitis D virus can be packaged by vesicular stomatitis virus and hepatitis C virus (PerezVargas et al., 2019). Hepatitis D ribonucleoproteins encapsulated in the envelope proteins of these viruses could successfully leave host liver cells and, using the appropriate surface receptors, bind and enter new liver cells, effectively spreading infection. Crucially, hepatitis D virus particles packaged in the stomatitis virus envelope were able to infect not only liver cells but also kidney cells.
This opens the possibility that the hepatitis D virus may actually be the cause of a number of diseases other than just hepatitis and hepatic cancer, including across different organ systems. Future studies might do well to search for hepatitis D genomes in a broader range of disease tissues, expanding beyond only the liver.
This articleoriginallyappeared in Forbes on April26, 2023 and is availableonlinehere:TheMightyVirusoid:Hepatitis D
Hepatitis D “virus” is a virus in name only. Underneath the hood, it more closely resembles viroids and virusoids: it is a small circular RNA that, due to high sequence complementarity, folds in on itself to form a rod-like structure. Like other virusoids, hepatitis D depends on a helper virus to infect cells and to migrate from host to host. Usually this help comes from hepatitis B virus, although recent findings suggest that other viruses may also be able to contribute, raising the possibility that hepatitis D virus may contribute to disease other than fulminant hepatitis (Perez-Vargas et al., 2019).
The hepatitis D virus genome consists of roughly 1670 to 1700 nucleotides, depending on the genotype. Currently, there are eight known genotypes, spread across the globe in geographic clusters (Figure 1). There are further minor variants within each genotype. Changes in the genome contribute to noticeable differences in pathogenesis, with some genotypes causing more serious disease than others (Spaan et al., 2020).
SOURCE: By Mario Rizzetto, University of Torino, Italy -
v:WikiJournal_Preprints/Epidemiology_of_the_Hepatitis_D_Virus Mario Rizzetto, licensed CC-BY, CC BY 4.0, https://commons.wikimedia.org/w/index.php?curid=84135496
Around 74% of the nucleotides in the hepatitis D virus genome are complementary, meaning they bind to one another. This compresses the circular genome into a rod-like form (Figure 2). Both the genomic and the antigenomic strands of hepatitis D virus encode ribozymes; these are active sequences of RNA that both cleave and rejoin the RNA during replication, as is the case for all viroids and virusoids (Figure 2).
ACCESS Health International (Adapted from FieldsVirology:DNAViruses , 7th Edition)
Strikingly, both complementary genomic strands have large open reading frames that have the potential to encode proteins. The antigenomic strand of the hepatitis D virus open reading frame encodes two distinct proteins: the small hepatitis D virus antigen (SHDAg) and the large hepatitis D virus antigen (L-HDAg) (Figure 3). These play a critical role in replication. There is no report of the even larger open reading frame of the genomic strand, which spans 352 amino acids, in the literature.
SOURCE: ACCESS Health International (Adapted from: Magnius et al. 2018, https://doi.org/10.1099/jgv.0.001150)
Hepatitis D virus is carried between hosts and into cells using the hepatitis B virus coat proteins. Once inside the cell, the hepatitis D genome wends its way into the nucleus via nuclear localization signals (NLS) embedded in the hepatitis D antigens there, replication begins. With the exception of the final assembly stage of the infectious particle —at which point the hepatitis B surface proteins are reintroduced— the replication cycle of hepatitis D virus is thought to be independent of hepatitis B. To exit the cell, hepatitis D virus once again makes use of the hepatitis B virus coat proteins (Figure 4).
Three kinds of hepatitis D RNA are involved in replication: genomic RNA, antigenomic RNA, and messenger RNA (mRNA). The genomic strand acts as a template for the production of the other two.
Upon entry into the nucleus, the first step of hepatitis D replication is the synthesis of mRNAs. Down the line, these will be used to produce the antigen proteins. Unlike most RNA viruses, hepatitis D does not encode an RNA-dependent RNA polymerase (RdRp). This is an enzyme that allows viruses to produce multiple copies of their RNA from an original RNA template. Instead, hepatitis D virus hijacks the cellular DNA-dependent RNA polymerase II (Pol-II), normally used to produce messenger RNAs from genomic DNA .
Exactly how hepatitis D virus manages to appropriate the host polymerases remains unclear, but it’s likely that its rod-like double-
stranded structure confuses the cell into mistaking it for a strand of DNA. This is another respect in which hepatitis D resembles viroids and virusoids, which depend on the same strategy.
The newly synthesized HDV mRNAs are then exported back into the cytoplasm, where they are translated into HDV antigen proteins (Figure 5) before being transported back into the nucleus.
Abbreviations: 5’, five-prime end; pA, poly-A tail; Pol-II, DNA-dependent RNApolymerase II; L-HDAg, large hepatitis D antigen; S-HDAg, small hepatitis D antigen. SOURCE: ACCESS Health International
With mRNA synthesis in full swing, the next step of the replication process can begin: amplification of genomic RNA. For this, the genomic RNA must first travel to the nucleolus, a special subcompartment of the nucleus. The specifics of this migration are not yet understood, but it is thought that the newly synthesized
antigens play a role in triggering and assisting the journey (Zi et al., 2022).
Once in the nucleolus, hepatitis D relies on a rolling-circle replication pathway (Figure 6A). The key mechanism behind this form of replication is the creation of a large chain of RNAs using the original circular RNA as a template. This chain, called an oligomer, is made up of many unit-length RNAs tethered together, but each is the mirror image of the original RNA. Such “mirror-image” RNA is referred to as antigenomic ; it is the inverse version of the genome. Unlike mRNA synthesis, creation of hepatitis D antigenomic RNAs relies on DNA-dependent RNA-polymerase I (Pol-I), which can only be found in the nucleolus.
As soon as the long antigenomic chain has been produced, the hepatitis D ribozymes spring into action, cleaving the long chain into unit-length RNAs. The antigenomic unit-length RNAs, currently in linear form, still need to be turned back into circular RNA. This process, known as ligation, happens either through the assistance of yet-unknown host enzymes or through the ribozymes themselves, which self-ligate the RNA back into circular form.
The antigenomic circular RNAs can then be used as a template to produce new genomic RNAs in a second round of rolling-circle replication; the mirror image is reflected once more, bringing it back to its original state (Figure 6B).
replication of antigenomic HDV RNA in
nucleolus,
Rolling-circle replication of genomic HDV RNA in the nucleus, with antigenomic RNA as template.
Following synthesis of HDV antigens and amplification of HDV RNA, all that is left is for the various pieces to be assembled and packaged in a protein coat containing the hepatitis B virus surface antigens. The finished viral particle is then ready to exit the host cell and infect neighboring cells.
This articleoriginallyappeared in Forbes on May 10,2023 and is availableonlinehere:RNAGymnastics:HowDoesHepatitisDelta VirusReplicate?
Hepatitis D virus is a small circular satellite RNA that can cause serious disease — despite its tiny size, it is responsible for the most severe form of viral hepatitis. Being a satellite RNA, it depends on a helper virus to infect cells and transmit between hosts. In humans, the usual accomplice is hepatitis B virus, which “lends” hepatitis D its protein coat, including surface proteins. Once inside a host cell, however, the two viruses go their separate ways and hepatitis D replicates independently. Vital to this replication process are the two proteins that hepatitis D virus produces: the small antigen and the large antigen.
Indeed, the hepatitis D antigens was the first clue in the mystery that ultimately led to the discovery of hepatitis D virus. In 1977, a gastroenterologist by the name of Mario Rizzetto, working out of the University of Turin, Italy, noticed some unusual activity in liver biopsies of chronically ill hepatitis B patients: some samples showed antibody activity against the hepatitis B core antigen even when there was none present (Rizzetto et al., 1977). After a series of followup experiments, Rizzetto and his colleagues realized that they had happened upon the antigen protein of an entirely different hepatitis virus. They named the new pathogen δ, delta. Later work revealed the presence of another, larger antigen, now called the large hepatitis D antigen. What follows is a detailed overview of these two proteins and their role in the hepatitis D virus life cycle.
As mentioned, the hepatitis D virus encodes two different proteins from the same open reading frame (ORF): the small hepatitis D antigen (S-HDAg), 195 amino acids, and the large hepatitis D antigen (L-HDAg), 214 amino acids. The open reading frame that encodes the proteins is found in the hepatitis D antigenomicstrand. The genomic strand also contains a large open reading frame, but whether it actively encodes any proteins has yet to be addressed in the current literature.
The difference between the two antigen proteins is a 19-amino-acid extension at the end of the small antigen (Figure 1). Despite sharing the majority of their amino acids, the functions of these two proteins within the hepatitis D life cycle are vastly different.
FIGURE 1. S-HDAg and L-HDAg are characterized by three RNA-binding domains (RBD), a coiled-coil sequence (CCS), a nuclear localization signal (NLS) in the N-terminal identity region (olive green box), and a nuclear export signal (NES), a farnesylation signal (pink star) in the C-terminal extension region (dark green box). SOURCE: ACCESS Health International (Adapted from: Zi et al. 2022)
The antigens produced by the hepatitis D virus are integral to replication. They are also integral to the structure of the complete “viral” particle. Eight single units of the small hepatitis D antigen
bind together to form one big entity, known as an octamer. The same happens with the large hepatitis D antigen. Some of the octamers are also formed via a mix-match of the large and small antigens. Four or five of these octamers are then grouped together and bound by the hepatitis D virus RNA (AbeywickramaSamarakoon et al., 2020). Based on the amount of hepatitis D virus antigens present per viral particle roughly between 70 and 200 it is likely that somewhere between two to six such bundles get wrapped up by the RNA. The finished product, known as a ribonucleoprotein complex, resembles beads on a string (Figure 2).
FIGURE 2. Structure of HDAg multimers and HDV ribonucleoprotein (RNP) complex. One eighth of the sphere represents a HDAg monomer, the blue one represents S-HDAg, the pink one represents L-HDAg. A HDAg octamer containing 8 S-HDAg or 8 L-HDAg is called homomultimer, and is called heteromultimer when it contains a mix of S-HDAg and L-HDAg. Quasi-doublestranded RNA (quasi-dsRNA) wraps around four or five HDAg octamers as a nucleosome-like core particle, and the whole HDV RNP contains 2–6 such core particles. SOURCE: Zi et al. 2022 https://doi.org/10.3389/fmicb.2022.838382
Finally, the whole ribonucleoprotein complex is encapsulated by a protein coat dotted with the hepatitis B virus surface antigen. This protein coat protects the genetic material of the hepatitis D virus and enables entry into new host cells. The hepatitis B antigen protein comes in three versions: a small version, a medium version, and a large version. All three can be found in the protein coat surrounding the hepatitis D virus genome.
The first 195 amino acids, corresponding to the small hepatitis D antigen, contain three crucial functional domains (Zi et al., 2022). The RNA-binding domain (RBD) allows the antigen to bind to the hepatitis D virus genome and form a ribonucleoprotein complex. There are three RNA-binding-domain regions in the antigen: amino acids two to 27, 97 to 107, and 136 to 146. The next crucial domain is the “coiled-coil” sequence (CCS), which spans from amino acid 31 to amino acid 52. This helps mediate the polymerization of the antigen. Finally, amino acids 68 to 88 represent the nuclear localization signal (NLS), which serves to catalyze movement of the antigen from one part of the cell to another; from the cytoplasm to the nucleus.
When hepatitis D virus first enters a host cell, the nuclear localization signal in the small antigen protein ferries it to the nucleus. There, the virus appropriates a host enzyme, RNA polymerase II (Pol-II), to create multiple copies of its messenger RNA sequence. Traditionally, host cells use this enzyme to transcribe DNA into RNA, but hepatitis D virus manages to hijack it to reproduce RNA from RNA. Although it is still not fully understood how this happens, researchers suspect it has to do with
the quasi-double-stranded structure of the hepatitis D virus genome, which the cell enzyme might mistake for DNA.
The mRNAs exit the nucleus and travel into the cytoplasm, where they are translated into small hepatitis D virus antigens (Figure 3). These antigens then migrate back into the nucleus, ready to assist with replication.
Abbreviations: 5’, five-prime end; pA, poly-A tail; Pol-II, DNA-dependent RNApolymerase II; L-HDAg, large hepatitis D antigen; S-HDAg, small hepatitis D antigen. SOURCE: ACCESS Health International
Once in the nucleus, the newly synthesized small antigens bind to RNA polymerase II. In doing so, the antigens displace a protein complex called negative elongation factor (NELF), which usually acts as a gatekeeper of transcription (Yamaguchi et al., 2001). Having cleared the way, amplification of hepatitis D genomic RNA can begin. By binding to RNA polymerase II, the small antigens also loosen the grip that the polymerase “clamp” —think of this as a hand that allows the polymerase to latch onto DNA or RNA— has
on the hepatitis D virus RNA (Yamaguchi et al., 2007). Loosening the grip that the polymerase has on the RNA allows it to move along more quickly than it usually would, accelerating the transcription process.
The small hepatitis D antigens also bind, via amino acids two to 67, to the central globular domain of human histone 1E (H1E) (Lee & Sheu, 2008). Histones are proteins in the nucleus which bind to and wrap up long strands of DNA, compacting them into structures called chromosomes. They also help regulate gene activity. Exactly how this helps the replication process of hepatitis D is unknown, but it is crucial: eliminating the hepatitis D binding site on histones completely inhibits replication.
Aside from RNA polymerase II and histone 1E, small hepatitis D antigens interact with Yin Yang 1 (Huang et al., 2008). This is a transcription factor, meaning it plays a role in regulating the speed of transcription. The RNA-binding domain of the small hepatitis D antigen binds to part of Yin Yang 1 as well as two enzymes associated with Yin Yang 1, CREB-binding protein (CRB) and p300. The resulting protein complex helps enhance RNA amplification, accelerating the hepatitis D replication process.
Finally, small hepatitis D antigens also bind to a chromatin remodeling protein called bromodomain adjacent to zinc finger domain 2B (BAZ2B) (Abeywickrama-Samarakoon et al., 2020). Chromatin is a DNA-protein complex that condenses the long strands of DNA into more compacted structures if our DNA wasn’t wrapped up like this, it would be far too long to fit in our cells. Chromatin remodeling proteins influence gene expression by modulating the chromatin architecture. Although it is not completely clear how, hepatitis D interaction with the
bromodomain adjacent to zinc finger domain 2B protein is critical to sustained RNA replication. Interfering with the interaction between the two inhibits RNA amplification.
Whereas the small hepatitis D antigen kickstarts and accelerates replication of hepatitis D RNA, the large hepatitis D antigen acts as a kill switch, inhibiting further replication and instead facilitating a shift towards viral assembly.
To this end, the 19-amino-acids-long extension of the large hepatitis D antigen contains a nuclear export signal (NES), which helps shuttle the ribonucleoprotein complex from the nucleus of the host cell into the cytoplasm, where it can be wrapped up in its protein coat. The last four amino acids of the large hepatitis D antigen make up a farnesylation signal. This signal recruits a host protease, farnesyl transferase, to add a small molecule called a farnesyl lipid group to the end of the large antigen. The addition of the farnesyl lipid group is critical to viral assembly, as it acts like a “hook” that enables the hepatitis D ribonucleoprotein complex to combine with the hepatitis-B-antigen-containing envelope (Figure 4) (Hwang & Lai, 1993). Only once it has been enveloped can the hepatitis D virus particle go on to infect new cells and transmit to new hosts.
Since the same stretch of RNA encodes both the small and large hepatitis D antigens, there has to be some mechanism that shifts production from the small antigen to the large antigen at just the right time. Here, hepatitis D virus employs a nifty bit of sleight of hand.
To transition from production of the small delta antigen to the large delta antigen, a single mutation of the mRNA sequence takes place, catalyzed by a protein called the host adenosine deaminase acting on RNA 1 (ADAR1). After a sufficient amount of small hepatitis D antigens have gathered in the nucleus, adenosine deaminase acting on RNA 1 binds to the hepatitis D RNA and changes the UAG stop codon, which usually marks the end of the open reading frame, to UGG. This mutation extends the open reading frame by 57
nucleotides, and thus adds a 19-amino-acids-long extension to the end of the small delta antigen (Figure 5).
Curiously, this point mutation doesn’t occur directly on the HDAg mRNA, but rather on the full-length antigenomic strand used as an intermediate during RNA replication. As the antigenomic strand is transcribed back into its genomic counterpart, the mutation carries over. The mRNA sequences derived from this mutated HDV genome will from then on produce the 214-amino-acid large delta antigen.
FIGURE 5. HDV RNA editing. Before editing (left), there is an amber codon (UAG, blue oval) at amber/W site of mRNA, translation will stop here to produce S-HDAg. During editing, adenosine deaminases acting on RNA 1 (ADAR1) converts UAG of agRNA to UIG (right). When Pol-II use these editedagRNA as a template to replicate edited-gRNA, and use edited-gRNA as a template to transcribe edited-mRNA, there is a W codon (UGG, pink oval) at amber/W site of edited-mRNA, the translation won’t stop until next termination codon is met, producing an L-HDAg. gRNA, genomic RNA. agRNA, antigenomic RNA. SOURCE: Zi et al. 2022
Over time, the large hepatitis D antigen catches up to the small hepatitis D antigen, and the proportion of the two antigens in the
ribonucleoprotein complex equalizes. After equilibrium of the two antigen proteins has been reached, the nuclear export signal of the large hepatitis D antigen becomes the dominant message. This acts as a trigger for migration of the ribonucleoprotein complex out of the nucleus and into the cytoplasm, where it can be wrapped up in a protein coat before finally exiting the cell.
This articleoriginallyappeared in Forbes on May 17, 2023 and is available online here: The Many Functions Of The Hepatitis D AntigenProteins
Viral hepatitis is a disease characterized by inflammation of the liver. Over time, this inflammation can lead to severe and often-irreversible scarring, interfering with the liver’s ability to perform its necessary functions. At its most extreme, the spread of scar tissue causes complete failure of the liver.
Although there are multiple hepatitis viruses, all of which cause disease, one of them is particularly pernicious: hepatitis D virus. Despite the name, hepatitis D virus more closely resembles a class of subviral pathogens called virusoids — small, circular RNAs that lack a protective protein coat and depend on a helper virus to transmit between hosts. In cases of human infection, hepatitis D is usually carried in by hepatitis B virus. The latter lends hepatitis D a protective coat replete with surface proteins, which enable entry into host cells.
Somewhat unusual for virusoids, hepatitis D produces two proteins. These are encoded by a stretch of RNA called an open reading frame (ORF); in brief, this is a section of the genome that can be “read” by host ribosomes, special cellular machines that translate RNA into proteins. A genome can contain multiple such open reading frames, each of which might encode a different protein. Indeed, aside from the open reading frame that encodes its two antigen proteins, hepatitis D virus contains a few additional open
reading frames. Discussion of these open reading frames, and their potential protein-producing capacity, is oddly absent from the literature. What follows is an attempt to fill this gap by providing a preliminary analysis of the additional open reading frames.
Every RNA genome is made up of an elaborate mix of four basic building blocks, called nucleotides, which are defined according to their nitrogenous base: adenine (A), guanine (G), cytosine (C), or uracil (U). Each base has its complementary pair; Adenine links up with uracil, and guanine links up with cytosine.
As part of the hepatitis D virus replication process, the RNA genome is used as a template for the production of a complementary, antigenomic copy (Figure 1). This copy is the exact inverse of the original genome — a mirror image. Wherever there’s an adenine in the genome, the antigenome instead contains a uracil, and so on.
(ORFs) clearly marked. SOURCE: ACCESS Health International (Adapted from: Magnius et al. 2018, https://doi.org/10.1099/jgv.0.001150)
In the case of hepatitis D, the open reading frame that encodes the two antigen proteins is located in the antigenome. But the genomic RNA also contains open reading frames of course, there is plenty of variation from strain to strain, with some containing longer open reading frames than others. Occasionally, you’ll find a strain with a very large open reading frame. Indeed, one strain contains an open reading frame in the genomic RNA that clocks in at 359 aminoacids-long. Composed of 1077 nucleotides, it spans almost two thirds of the entire genome. Compare this to the antigen-encoding open reading frame of the antigenomic strand, which is 214 aminoacids-long.
Whether or not the open reading frames found in the genomic RNA of hepatitis D actually produce any proteins is unclear; all the pieces are in place, but that doesn’t necessarily mean they are being put to use. For example, there is no evidence at present that a messenger RNA corresponding to the positive strand is made, still less that it is transported to the cytoplasm for translation. Perhaps it is only present at some stage of the life cycle and disease process.
Still, it seems extremely unlikely that such a long open reading frame would be present and yet remain unused. This is especially true as the constraints to maintain such a long open reading frame overlapping the minus strand coding sequence is severe. This observation calls for an intense search for both positive strand messenger RNAs and proteins.
In addition to the long open reading frame, there are also a number of other, shorter open reading frames scattered throughout the
genome. This is also true of the antigenome, which aside from the antigen-producing open reading frame, has multiple other open reading frames that could potentially encode proteins. Whether or not any of these additional open reading frames are functional remains to be seen.
We recommend that a serious effort be mounted to test for the presence of the hypothetical proteins of the genomic-strand RNA. This can be achieved by creating antibodies that target the hypothetical protein. Testing should be done not only for the entire replication cycle of hepatitis D virus, but for all stages of infection and disease progression.
Assuming the aforementioned 359 amino-acids-long open reading frame did encode a protein, what functions might it have? By comparing the amino acid sequence of the open reading frame to sequences listed in the GenBank database, we were able to identify matches to three proteins: phosphohistidine phosphatase, GDP-Lfucose synthase, and pectate lyase (Genbank Overview, n.d.).
It is worth noting that these matches are mainly partial matches, meaning only a small section of the open reading frame overlaps with a small section of the protein sequence. Extrapolating what function a particular snippet of a protein sequence might have is an inexact science; the regions of similarity may have particular functions that do not necessarily match the overall functions after which the full proteins are named. What follows should be understood as laying the initial groundwork for further, more detailed research.
It is also important to keep in mind that the open reading frame discussed above is found in one strain of hepatitis D virus, but how conserved it is across strains is unclear. It would be prudent to first establish whether there are any open reading frames in the genomic RNA that are highly conserved, and then use these highly conserved sequences for future analysis.
PhosphohistidinePhosphatase
Phosphohistidine phosphatase is a protein involved in “dephosphorylation”, the removal of a phosphate group from a molecule. Phosphorylation and dephosphorylation are crucial processes in the regulation of cell signaling and in the regulation of gene expression. The addition or removal of a phosphate group can contribute to whether a certain gene, and by extension protein, is expressed or suppressed.
It is not clear exactly how this would influence the hepatitis D life cycle, but considering the importance of de/phosphorylation to virtually every cellular process including transcription and translation it could be an important part of the puzzle.
As implied by its name, GDP-L-fucose synthase is a protein that catalyzes the production of GDP-L-fucose. GDP-L-fucose, in turn, is a critical player in a process known as fucosylation, which is characterized by the addition of fucose sugar units to molecules. Fucosylation of proteins is relevant to both cell signaling and immune regulation (Li et al., 2018).
That said, fucosylation has also been associated with various cancers, including liver cancer (Zhou et al., 2017). It is well known
that chronic hepatitis D infection can lead to liver cancer, and it does so more frequently than hepatitis B monoinfection (Farci et al., 2021). The mechanisms underlying this increased risk for liver cancer are poorly understood. Perhaps the open reading frames of the hepatitis D genomic RNA contribute to disease state, rather than replication and life cycle? Additional research is needed to confirm whether this may be the case.
Pectate lyases are enzymes involved in the degradation of plant cell walls (Hugouvieux-Cotte-Pattat et al., 2014). Although employed in certain plant tissues to help remodel the cell wall during growth, they are most commonly found in plant pathogens, including bacteria. Here, they help the pathogens break down pectin, which plays a key role in the rigidity of the cell wall; think of a battering ram breaking down a castle gate. Once the cell wall is weakened, entry becomes easy.
The use of pectate lyase-like functions to hepatitis D is unclear. Viroids and most virusoids, with which hepatitis D shares many similarities, are plant pathogens. Yet, they rarely encode any of their own proteins. This makes it unlikely that the similarities between the large genomic-strand open reading frame and pectate lyase are remnants of a shared evolutionary history between hepatitis D and viroids.
Since hepatitis D, per current understanding, exclusively infects non-plant hosts, it seems unlikely that the pectate lyase-like sequence of the open reading frame contributes heavily to the viral life cycle.
Given the severity of hepatitis D infection, gaining a full understanding of the pathogen is critical; the better we understand hepatitis D virus, the better-equipped we are to combat it. It would be wise to confirm whether open reading frames in the genomic RNA are active. If they are, it may yield new avenues for pharmaceutical intervention. If they are not, then at least we know with certainty that we are not overlooking something, allowing us to focus any drug-development efforts on other parts of the genome.
This articleoriginallyappeared in Forbes on May 25, 2023 and is available online here: Does The Hepatitis D Genome Encode ProteinsFrom BothThe“Plus”And“Minus”Strands?
All viruses encode an enzyme capable of replicating their genome, be it DNA or RNA. Without such an enzyme, the virus is rendered impotent — even if it manages to infiltrate a host cell, it cannot replicate. Viroids and virusoids, including hepatitis D, are the exception. These small, circular RNA pathogens do not encode replication enzymes of their own. Despite this, they manage to replicate without issue. How? The simple answer is that they hijack the replication machinery of the host cell. Although most viroids and virusoids do not encode proteins, hepatitis D virus does. The hepatitis D protein —known as hepatitis D virus antigen (HDAg)— modifies the relevant cellular machinery to its own advantage. In particular, it interacts with host DNA-dependent RNA polymerases (Pol I, Pol II, and Pol III). What follows is an overview of this strategy and its importance to the hepatitis D life cycle (Figure 1). Understanding these interactions opens the opportunity for new hepatitis-D-specific antiviral drugs.
DNA-dependent RNA polymerases are enzymes that a cell uses to copy the information in DNA to RNA. There are three human RNA polymerases, designated RNA polymerase I, II, and III.
RNA polymerase I is a large protein composed of 14 different subunits, known as polypeptides. It contributes to cell division by creating many of the components of ribosomes, the proteinproducing machinery of cells. Think of ribosomes as translators; as they traverse a strand of RNA, they convert the nucleotides that make up the RNA into amino acids. RNA in, proteins out.
RNA polymerase I produces these ribosomal pieces —ribosomal ribonucleic acids (rRNAs), to use the technical term by
transcribing a special kind of DNA called ribosomal DNA (rDNA). The ribosomal RNA transcribed by RNA polymerase I joins up with ribosomal proteins to form a complete ribosome.
Transcription of ribosomal DNA into ribosomal RNA by RNA polymerase I happens in a separate subcompartment of the nucleus known as the nucleolus.
RNA polymerase II is also a multi-protein complex, made up of 12 different subunits. Five of its subunits are shared with RNA polymerase I and III (White, 2013). Despite this, its function is very different; where RNA polymerase I exclusively transcribes ribosomal DNA, RNA polymerase II transcribes the protein-coding sections of ordinary DNA into messenger RNA. These messenger RNAs are then used as blueprints for the synthesis of proteins.
Transcription of DNA by RNA polymerase II occurs in the nucleoplasm.
RNA polymerase III is the largest of the three RNA polymerases, composed of 17 subunits. Again, it shares five of its subunits with the other two RNA polymerases. And like the other two polymerases, it also serves to facilitate transcription of DNA into particular kinds of RNA. Specifically, it transcribes DNA to produce 5S ribosomal RNA the only ribosomal RNA molecule not transcribed by RNA polymerase I and to produce transfer RNA (tRNA).
In addition to the aforementioned RNA polymerases, there are two more (Pol IV and Pol V). However, these are exclusively found in plants. Since hepatitis D is an animal pathogen, the two plant RNA polymerases are not relevant.
Recall that DNA-dependent RNA polymerases usually take DNA as a template and convert it into one or another form of RNA. Hepatitis D virus, however, is made up exclusively of RNA. This means that the pathogen somehow appropriates the polymerases into using RNA as a template — RNA to RNA, instead of DNA to RNA. How does it achieve this act of subversion?
Macnaughton and Lai propose two possible explanations, which, it should be noted, are not mutually exclusive (Macnaughton & Lai,
2006). The first is that the small protein of hepatitis D virus binds directly to the RNA polymerases, initiating replication and transcription. The other possibility is that the quasi-double stranded secondary structure of hepatitis D virus a result of 70% sequence self-complementarity is mistaken for DNA, thereby hijacking RNA polymerases and transcription factors that usually would not bind to RNA (Nagata et al., 2021).
Evidence for the first explanation is abundant. For one, the small hepatitis D protein interacts with nine of the 12 RNA polymerase II subunits (Cao et al., 2009). Along with these nine subunits, the small protein was also found to interact with 65 other host proteins involved in transcription. Many of these are also known to bind RNA polymerase II. It is possible that, after binding to RNA polymerase II, these proteins then also link to the small protein to further replication.
Another point in favor of this possibility is that the small protein of hepatitis D binds to a section of RNA polymerase II known as the “clamp”, which acts as a claw that allows the polymerase to latch onto DNA (Figure 3). In binding to this region, the small protein loosens the grip of the clamp. The effect of this is twofold. On the one hand, loosening the clamp speeds up the rate of transcription, and by extension, the rate of replication. On the other hand, it decreases fidelity of transcription. This is not necessarily a bad thing. On the contrary, it may be the mechanism by which the small protein is able to hijack RNA polymerase II: decreased fidelity may represent a relaxation of the usual template requirements. Indeed, Yamaguchi et al. note, “By reducing transcriptional fidelity in terms
of not only discrimination of incoming nucleotides but also recognition of templates, HDAg may facilitate the unusual RNAdependent RNA synthesis by Pol II.” (Yamaguchi et al., 2007)
Finally, the small hepatitis D protein shares some of its sequence with a region of a protein complex called negative elongation factor (NELF). Negative elongation factor binds to RNA polymerase II and represses transcription. In order for transcription to begin, negative elongation needs to first be removed — think of the complex as a kind of gatekeeper, preventing RNA polymerase II from activating in instances it shouldn’t. As the small protein binds to RNA polymerase II, it displaces the negative elongation factor, kick-starting the polymerase into transcription mode (Yamaguchi et al., 2001).
This articleoriginallyappeared in Forbes on June 9, 2023 and is available online here: How Hepatitis D Virus Hijacks Host ReplicationMachinery
Viroids and virusoids are small, circular RNA pathogens. They have a very unique ability: with only a tiny amount of genetic information, they are able to transmit between hosts, infect cells, and cause disease. Viruses and other microbes also manage to do this, but by comparison they are veritable giants. The virus that causes Covid-19, for example, depends on roughly 30,000 nucleotides —the building blocks of ribonucleic acid (RNA)— to achieve its dirty work. The average viroid or virusoid, in contrast, weighs in at only 200 to 450 nucleotides
As well as being larger, most viruses encode multiple proteins to help them replicate and, in especially nefarious cases, evade and suppress the host immune system — they come prepared, toolbox in hand. The vast majority of viroids and virusoids do not encode any proteins of their own. Instead, they depend entirely on the host to help them replicate. Where viruses bring their own tools, viroids and virusoids simply appropriate the tools already present.
Some of the most important cellular tools for viroid and virusoid replication are host enzymes called DNA-dependent RNA polymerases. These are proteins that usually copy sections of DNA into RNA; DNA in, RNA out. Somehow, viroids and virusoids manage to hijack these polymerases, fooling the proteins into transcribing viroid RNA genomes instead of their usual DNA targets; RNA in, RNA out. This act of molecular acrobatics allows
the pathogens to create multiple new copies of their genome, each of which can then go on to become a fully infectious particle.
Hepatitis D “virus”, despite the name, is a virusoid. Like most other virusoids, it depends on host RNA polymerases for replication. Unlike most other virusoids, it encodes proteins of its own. These proteins contribute to the replication process. In a previous article, we reviewed how the small hepatitis antigen protein (S-HDAg) binds to and alters RNA polymerase II, kickstarting replication. Here, we look at an alternative possibility: perhaps sections of the hepatitis D genome alone, independently of the small hepatitis D protein, suffice to pirate the host RNA polymerases. This would line up with what we know about other viroids and virusoids, which manage to replicate without encoding any proteins.
For a long time it was suspected that RNA polymerase II one of three DNA-dependent RNA polymerases present in humans was associated with the replication of hepatitis D virus. Anytime alpha (
α) amanitin, a toxin that interrupts RNA polymerase II, was introduced, replication of hepatitis D virus was also inhibited (Fu & Taylor, 1993). Exactly how and where polymerase II recognizes the hepatitis D genome, however, was unclear.
As with many viroids and virusoids, the hepatitis D virus genome has a high degree of self-complementarity around 70% of the nucleotides pair up (Nagata et al., 2021). Since the genome is circular, the pairing of nucleotides causes the circle to fold in on itself, resulting in a rod-like structure (Figure 1). In areas of low or no sequence self-complementarity, single bulges or loops remain. This includes stem loop structures at each end.
SOURCE: ACCESS
To find out which parts of the hepatitis D virus genome interact with RNA polymerase II, Greco-Stewart et al. broke the genome down into three domains: a 213-nucleotide-long section corresponding to the left stem-loop region (nucleotides 687 to 900); a section corresponding to the quasi-double-stranded middle section (nucleotides 681 to 54 and nucleotides 900 to 1540); and a 199-nucleotide-long section corresponding to the right stem-loop region (nucleotides 1540 to 60) (Greco-Stewart et al., 2007). This was done for both the positive-strand RNA as well as the negativestrand RNA.
Exposing these three regions to RNA polymerase II yielded a surprising result: only the stem-loop regions at the ends of the hepatitis D genome interacted with the host enzyme (Figure 2B). This was the case for both polarities of the genome. The middle section, on the other hand, showed no indication of interacting with the host enzyme. This suggests that the RNA polymerase II interaction sites are between nucleotides 1540 to 60 and nucleotides 687 to 900 of the hepatitis D virus RNA. These observations also suggest that the cellular DNA-dependent RNA-polymerase recognizes the specific structure, not the nucleotide sequences themselves as the sequences differ not only between the two ends
but also between the stem loop corresponding to the positive and negative versions of the genomic RNA.
FIGURE
Interaction of RNA polymerase II with: hepatitis D virus genome (HDVG); hepatitis D virus antigenome (HDVAG); right stem-loop section, 199 nucleotides, genomic strand (R199G); central section, genomic strand (CENTRG); left stem-loop section 213 nucleotides, genomic strand (L213G); right stem loop, 199 nucleotides, antigenomic strand (R199AG); central section, antigenomic strand (CENTRAG); left stem-loop section 213 nucleotides, antigenomic strand (L213AG). (C) Interaction of RNAP II with the extremities of the rod-like HDV secondary structure of both polarities. SOURCE: GrecoStewart et al. 2007 https://doi.org/10.1016/j.virol.2006.08.010
To hone in on the exact location of the interaction sites, the researchers broke down the stem-loop regions into smaller sections, testing each for their ability to interact with RNA polymerase II. They found that a 38-nucleotide sequence on the tip of the right stem-loop region and a 48-nucleotide sequence on the tip of the left stem-loop region were particularly integral to successful binding (Figure 2C). Indeed certain nucleotide sequences within these regions proved to be fully conserved across 81 different variants of
the hepatitis D virus and there seems to be high selective pressure to keep the rod-like secondary structure of these regions.
Along with RNA polymerase II, RNA polymerases I and III are also implicated in hepatitis D replication. Building on their previous work, Greco-Stewart et al. confirmed that hepatitis D virus interacts with RNA polymerases I and III; it associates with transcription initiation factor TFIID subunit 1 (TAF1) and with the DNAdirected RNA polymerase III subunit RPC10 (POLR3K) (GrecoStewart et al., 2009). Transcription initiation factor TFIID subunit 1 is a subunit of a protein complex called selective factor 1 (SL1). Selective factor 1 binds to the target DNA and attracts another protein to which RNA polymerase I can bind, initiating transcription. POLR3K —also known as RPC10— is a subunit of RNA polymerase III that copies cellular DNA into transfer RNAs. It also plays an important role in termination and initiation of transcription.
As with RNA polymerase II, polymerases I and III associate with both polarities of hepatitis D RNA in liver cell lines. And the two polymerases also interact exclusively with the stem-loop regions of the hepatitis D genome (Figure 3).
FIGURE 3. Compilation of the interaction of various HDV-derived RNAs with both TAF1 and POLR3K. Stem-loop domains: R199G, R199AG, L213G, and L213AG. Central domains: CENTRG and CENTRAG. G= genomic, AG = antigenomic. SOURCE: Greco-Stewart et al. 2009
https://doi.org/10.1016/j.virol.2009.02.007
Connecting the Dots: TATA-Binding Protein?
All three human RNA polymerases interact with the stem-loop regions of the hepatitis D genome; might there be some common feature shared by the polymerases that enables this interaction?
The answer may lie in the TATA-binding protein (TBP). This protein is common to all three of the RNA polymerases and plays a central role in initiating transcription. Indeed, binding of the TATAbinding protein to the target DNA is the first step in transcription; it provides an anchor site for additional “transcription factors” other proteins involved in transcription and it recruits these proteins into action.
Greco-Stewart et al. mention, “[TATA-binding protein] can recognize both TATA and TATA-less promoters dependent on accessory transcription factors, and its affinity for DNA templates is mediated by electrostatic interactions with the phosphate backbone, which could account for its ability to bind HDV RNA despite the absence of known DNA promoter features.”
Clearly, viroids and virusoids punch well above their weight. With a few hundred nucleotides, they achieve what other viruses need tens or hundreds of thousands of nucleotides to do. Understanding these fascinating entities, and how they achieve so much with so
little, may help us design more efficient drugs and inform novel messenger RNA therapies.
This articleoriginallyappeared in Forbes on June14, 2023 and is availableonlinehere:MolecularAcrobatics:ALookAtHepatitisD VirusReplication
Virusoids are strands of circular infectious RNA. These biological oddities are much smaller than the smallest viruses and have far fewer moving pieces. Most virusoids do not encode proteins, relying entirely on host cellular machinery for replication — what they don’t bring with them, they hijack as needed. Along with depending on the host cell, virusoids also depend on a helper virus to shuttle them between cells and between hosts. This is because virusoids do not come with an envelope, and by extension, any surface proteins of their own. Surface proteins act as a sort of key, allowing viruses to bind to cell membranes and gain access into the interior of the cell. Only once inside can a virus begin replicating.
Hepatitis D virus, despite the name, behaves like a virusoid: it is a small, circular RNA molecule that relies on a helper virus to be shuttled into host cells and relies on host machinery for replication. It is also responsible for the most aggressive form of viral hepatitis, causing severe liver scarring and liver cancer. Hepatitis B virus was long considered its sole helper virus, providing it with the envelope and surface proteins necessary for infection. This also meant the liver was considered the sole site of infection. Novel research challenges both of these assumptions. Published in Nature , the work by Perez-Varga et al. provides evidence of successful hepatitis D virus transmission via a number of different viral envelopes
(Perez-Vargas et al., 2019). The findings indicate hepatitis D virus
may be able to persist in a far larger range of tissues than the liver and may contribute to diseases of unknown etiology, including degenerative neurological disease.
Non-Human Hosts: IsHepatitis B VirusNecessary?
The origins of hepatitis D virus are still poorly understood. So too is the range of species it can infect. Although initially thought to be restricted to human hosts, a growing number of studies report the presence of hepatitis D virus-like RNAs in non-human animals. This includes snakes, birds, fish, insects, and beyond (Chang et al., 2019;Hetzel et al., 2019; Iwamoto et al., 2021). Granted, the hepatitis D virus-like agents vary significantly from animal to animal, but they retain key characteristics of the pathogen: a small circular genome, a rod-like secondary structure, and hepatitis D antigen proteins (HDAgs).
Crucially, none of the animals infected with the hepatitis D viruslike RNAs were found to carry hepatitis B virus, or any of the other hepadnaviruses. How did they get into the diverse animal cells?
Alternative ViralEnvelopes?
The presence of hepatitis D virus-like agents in animals otherwise free of hepadnaviruses raised the possibility that they may have been transmitted with the help of envelopes from other viruses.
To test this, Perez-Varga et al. exposed liver cells to specially engineered hepatitis D virions; instead of the usual hepatitis B virus envelope and surface proteins, they wrapped hepatitis D virions in the envelope of vesicular stomatitis virus (VSV) or hepatitis C virus (HCV). The envelopes included the surface proteins of each. As a
control group, the researchers also created virions with dud envelopes that did not encode any proteins.
Three days after initial infection of the liver cells, hepatitis D virus RNA had accumulated outside of the cells. Usually hepatitis D virus depends on the hepatitis B virus surface proteins for cell exit essentially, the inverse of the entry process. Yet, the engineered virions with the alternative surface proteins proved just as capable of exiting the cell, as evidenced by the amassment of extracellular RNA. In fact, by day nine the amount of extracellular hepatitis D virus RNA in cell cultures derived from virions coated in the vesicular stomatitis virus envelope was more than sixfold higher than in cell cultures using hepatitis B viral envelopes.
Having determined that hepatitis D virions coated in unconventional envelope proteins could successfully exit the cell, Perez-Varga et al. next tested to see if the virions could also gain entry into cells and begin replicating. The engineered virions had no trouble, with intracellular levels of hepatitis D virus RNA confirming not only the presence of the virus, but also its successful replication. Indeed, the virions with alternative envelopes could infect kidney cells as well as the usual liver cells. The “normal” hepatitis D virions could not do so.
As is the case with normal hepatitis D virions, the engineered virions entered cells in the same way that their helper viruses did, using the same pathways. Blocking the helper viruses with targeted antibodies also blocked the engineered virions from entry.
The team of researchers then performed the same experiments with an even larger group of helper viruses, creating hepatitis D virions enveloped with the surface proteins of: RD114 cat endogenous
virus, murine leukemia virus (MLV), human immunodeficiency virus (HIV), avian influenza virus (AIV), lymphocytic choriomeningitis virus (LCMV), human metapneumovirus (HMPV), dengue virus (DENV), and West Nile virus (WNV). Human metapneumovirus, dengue virus, and West Nile virus were all able to act as helper viruses; levels of hepatitis D virus particle secretion for these alternative envelopes matched those of normal hepatitis D virions. Lymphocytic choriomeningitis virus also managed to act as a helper virus, but the levels of hepatitis D virus particle secretion were lower than when using hepatitis D virions enveloped with hepatitis B virus surface proteins. The remaining viruses failed to enable cell exit and entry.
The above experiments were all performed in cell cultures would the results hold in live animal models?
To find out, mice were co-infected with hepatitis D virus RNA and hepatitis C virus. They were split into three groups: mice first infected with hepatitis C virus and then, four weeks later, with “helper-free” hepatitis D virus; mice first infected with “helper free” hepatitis D virus and then, four weeks later, with hepatitis C virus; and finally, mice co-infected with the two viruses simultaneously.
A few weeks after co-infection, the mice were tested for hepatitis D virus RNA. Hepatitis D virus RNA was detected in all three groups; all mice infected with hepatitis C virus were also positive for hepatitis D virus and significant amounts of hepatitis D RNA was found in their blood. These results suggest that hepatitis C virus can effectively act as a helper virus to hepatitis D virus even in vivo.
Not only can hepatitis D virus be propagated by a variety of helper viruses other than just hepatitis B virus, it can also infect a range of different tissues because of this. This raises the possibility that hepatitis D virus is actually responsible for a far larger number of diseases than initially thought, beyond inflammation of the liver. This articleoriginallyappeared in Forbes on June16, 2023 and is available online here: Might Hepatitis D Virus Cause More DiseasesThan InitiallyThought?
Hepatitis D virus has generally been considered an exclusively human pathogen, but a fast-growing body of research suggests otherwise. Hepatitis D virus-like agents seem to be active in a range of different animal species, from fish all the way to termites. Such findings call for a reevaluation of previous assumptions and a recognition that hepatitis D-like viruses may be significantly more pervasive than initially thought.
Hepatitis D “virus” is a tiny, infectious RNA pathogen. Far smaller than any known virus, it more closely resembles subviral agents known as viroids and virusoids. These are characterized by their circular genomes and lack of replication proteins: where viruses encode proteins to help them replicate their genetic information, viroids and virusoids instead hijack host-cell proteins. The same is true of hepatitis D virus. And like other virusoids, it is also understood to be dependent on the envelope proteins of a helper virus —in this case hepatitis B virus— for transmission between hosts and between cells.
Central to these new findings is a technique called metatranscriptomics. Pioneered in the early 2000s, metatranscriptomics refers to a series of strategies that enable the characterization of the RNA content of large environmental
samples (Poretsky et al., 2005). For example, researchers might extract a scoop of soil from a bog in Scotland and analyze that soil for all of the RNA sequences contained within it. The RNA can then be cross-referenced against large databases to establish matches with known RNA sequences
Metatranscriptomics allows researchers to extract massive amounts of RNA from environmental samples that would otherwise be difficult to study; in a sense, it acts as a giant sieve that filters out everything that isn’t RNA content.
One of the first traces of hepatitis D virus-like agents in non-human hosts was discovered in 2018 by a group of researchers based in Australia (Wille et al., 2018). As part of a broader avian metatranscriptome study, Wille et al. analyzed throat and anal swabs collected from ducks caught at a water-treatment plant in Melbourne. They noticed that some of the RNA resembled that of human hepatitis D virus.
The nucleotide contents didn’t quite match up human hepatitis D virus has a guanine (G) and cytosine (C) content of 60%, whereas the guanine and cytosine content of the new sequences was only around 51%. This aside, the two RNA sequences shared many features suggestive of common ancestry. For one, the avian hepatitis D virus-like RNA was circular and folded in on itself to form an unbranched rod-like secondary structure, a key characteristic of human hepatitis D virus. The hepatitis D virus-like genome discovered in the duck samples also had an open reading frame with the capacity to express an 185-amino-acid-long protein. The location of this open reading frame within the genome very closely
mirrors the location of the open reading frame seen in human hepatitis D virus (Figure 1). On top of this, certain sequences of the avian hepatitis D virus-like agents resemble those corresponding to the genomic and antigenomic ribozymes found in human hepatitis D virus. Indeed, both of these potential avian ribozymes had secondary structures very similar to those of human hepatitis D virus ribozymes (Figure 2).
Curiously, none of the duck samples showed any signs of infection with duck hepatitis B virus. One possible explanation for this is that non-human hepatitis D virus-like agents may take advantage of other viruses to make infectious particles and to transmit from cell to cell. This explanation is supported by later work, which showed that, even in humans, hepatitis B virus is not the only one that can function as a helper virus.
FIGURE 1. Location of genomic and antigenomic ribozyme sequences, and the predicted ORF of the delta antigen in the avian HDV-like genome compared to their location in the HDV genome sequence. SOURCE: ACCESS Health International (Adapted from: Wille et al. 2018
https://doi.org/10.3390/v10120720)
Spurred on by these findings, Wille and colleagues revisited data collected during previous metatranscriptomic work, now with an eye towards identifying potential new hepatitis D virus-like agents (Chang et al., 2019). This earlier data included samples from amphibians, fish, reptiles, and invertebrates.
From the more than a billion nucleotides gathered across the various libraries, the researchers were able to isolate four novel hepatitis D virus-like agents. These belonged to Subterranean termite, Asiatic toad, Chinese fire belly newt, and a mixture of fish (Table 1).
SOURCE: ACCESS Health International (Adapted from: Chang et al. 2019
https://doi.org/10.1093/ve/vez021)
As with the duck hepatitis D virus-like agents, the guanine/cytosine contents of these newly discovered varieties were also lower than in human hepatitis D virus. Still, all of the genomes were circular with unbranched rod-like secondary structures and their sizes consistent with other hepatitis D virus-like agents (1500-1700 nucleotides). Additionally, all of the newly discovered agents encoded a putative hepatitis D virus-like antigen protein, which, when cross-referenced against large protein databases, proved to be most closely related to the human delta antigen proteins. Even for the distinct domains within each of the putative antigen proteins, all of the “top scoring hits” matched human hepatitis D antigen protein.
Again, none of the data libraries contained any hepatitis B virus RNA. In fact, they didn’t contain any hepadnavirus RNA of any kind. They did, however, contain sequences from other viruses which may have served a functionally similar role (Table 2).
TABLE 2. Details of the HDV-like agents and associated viruses discovered previously. SOURCE: ACCESS Health International (Adapted from: Chang et al. 2019)
This adds credence to the notion that hepatitis D virus and viruslike agents are not exclusively tethered to the hepatitis B virus and
may instead be able to use other helper viruses to assist with transmission and infection.
Since the completion of these early studies, the list of potential hosts has been growing steadily. Aside from ducks, newts, toads and the rest of the abovementioned animals, hepatitis D virus-like agents have now also been found in white-tailed deer, passerine birds, and woodchucks (Iwamoto et al., 2021). Clearly, the assumption that hepatitis D virus is a strictly human pathogen has reached its limit the virus is far more widespread than initially thought. Not only this, but the range of helper viruses is also likely far more diverse than previously understood. None of the animal samples showed any indication of co-infection with hepatitis B virus, and yet hepatitis D virus persisted in great numbers. We have to begin acknowledging that hepatitis D virus-like agents likely adopt envelope proteins from a variety of sources. Different helper viruses may enable the infection of different tissues, and with this, cause diseases never previously associated with hepatitis D virus. We are only just beginning to scratch the surface. This articleoriginallyappeared in Forbes on June22, 2023 and is availableonlinehere:HepatitisDVirus-LikeAgentsAreUbiquitous ThroughoutTheAnimalKingdom
Viroids and virusoids are a special kind of pathogen: small, circular strands of RNA that can infect hosts and cause disease. Think of a virus, but far more minimalist. All viruses encode at least one protein to help them transcribe and replicate their genetic material. Many others contain additional proteins that serve to evade, or even suppress, the host immune system. Barring a few exceptions, viroids and virusoids do not encode any such proteins. Despite this, they are a serious agricultural scourge, causing multimillion-dollars-worth of damage each year. Others, including hepatitis D-like agents, can infect humans and animals. New research indicates that these biological oddities may be far more common than initially thought. Published in the journal Cell , the study has led to a five-fold expansion of the known diversity of viroids and viroid-like agents (Lee et al., 2023).
Transcriptomics refers to the analysis of all the RNA content in a cell (NCIDictionaryofCancerTerms , 2011). This allows scientists to get a sense of which genes are turned on or off in cells at different points in time, including during states of infection or disease.
Metatranscriptomics takes this same idea but broadens it: instead of studying the RNA content within a single cell, metatranscriptomics studies the RNA content of a much larger environmental sample (Sutherland et al., 2022). For example, marine biologists might
collect a sample of seawater from a particular region to determine its RNA makeup, giving them a better sense of the microbes active in that area.
Of course, it’s not quite as simple as that. Metatranscriptomics is a complex process involving multiple steps (Ojala et al., 2023). After initial sample collection, the RNA has to first be extracted and separated from the rest of the contents. The RNA has to then be prepared for high-throughput sequencing, which may include enrichment of desired RNA there are various different types of RNA, some more common than others. Once the desired RNA has been sufficiently enriched, it can be sequenced. And finally, algorithms and other bioinformatic strategies are used to analyze the sequencing data.
Rather than collect their own samples for analysis, Lee et al. drew upon readily-available metatranscriptome “libraries.” That is, data collected and made public by other researchers. Before they analyzed these libraries, however, they would need to establish a solid method to sift through the massive amounts of RNA and single out only those strands belonging to viroids and virusoids. Think needle in the haystack, but the haystack is the size of a football field and the lights are off.
The method or pipeline starts by filtering out any non-circular RNAs. This is achieved by honing in on “head-to-tail repeats”, motifs characteristic of circular RNAs and their replication intermediates. The replication intermediates are long chains of unit-length RNA tethered together, head-to-tail. These need to be
cleaved from one another, so that only the unit-length RNA remains.
All of the putative circular RNAs then need to be filtered again, with a more fine-toothed comb; non-viroid circular RNAs are abundant in nature, and although we are still figuring out their exact functions, it is clear that they play an important role in gene regulation (Greene et al., 2017). Like most viroids and virusoids, circular RNAs do not encode any proteins. So to differentiate viroidlike circular RNAs from plain old circular RNAs, Lee et al. analyzed the sequences for the presence of self-cleaving ribozymes. They examined both the sequence itself as well as the secondary structure of the RNA molecule. Ribozymes are special sequences of RNA that, instead of encoding proteins for catalytic functions, are able to perform these functions themselves; in the case of viroids, ribozymes help cleave the long chain of tethered RNAs down to unit length, thus furthering the replication process. Think of ribozymes as “active” RNA sequences. Any potential ribozyme structures were cross-referenced against a database of known self-cleaving ribozymes
But not all viroids contain ribozymes. Members of the pospiviroidae family one of the two main viroid families, the other being avsunviroidae are not known to harbor ribozymes. Instead, they have a characteristic RNA section called the RY motif stem loop. It is not clear what exactly it does, but it is known to be critical to successful infection (Gozmanova, 2003). To account for this, Lee et al. supplemented the database of known ribozymes with known RY motifs.
The last stage of the pipeline involves cross-referencing any newly discovered viroid-like RNAs against reference databases for direct comparison of sequence similarity.
To test their method, Lee et al. processed and searched a plant dataset composed of 1,344 transcriptomes, made up of more than 103 million full-length RNA transcripts. All viroids and viroid-like RNAs, with the exception of hepatitis D virus, infect plants. If their pipeline proved accurate, it would be able to recover known viroidlike RNAs from the transcriptomes. Using their pipeline, they predicted roughly 164,000 full-length circular transcripts. Of these, 42 were identified as viroid-like agents; 15 sequences were deemed viroid-like by virtue of having ribozymes, 33 were deemed viroidlike after cross-reference against viroid databases, and six sequences met both criteria. Their method did its job, filtering out all non viroid-RNA sequences.
Next, the group of researchers applied their pipeline to 5000 diverse metatranscriptomes, totalling 1.5 billion full-length RNA transcripts. Roughly 8.5 million RNA sequences were predicted to belong to circular RNAs, with an average size of 165 nucleotides. For reference, known viroids are between 220 and 450 nucleotides long (Lee & Koonin, 2022). Indeed, almost three million of the putative circular RNAs fell within the known size range of viroids. Of these, 11 thousand were classified as viroid-like because they contained a self-cleaving ribozyme. Curiously, none of the putative circular RNAs matched the RY motif of the pospiviroidae family. Ten thousand of the identified circular RNAs showed no detectable
sequence similarity to known viroids, suggesting that they were newly discovered. The remaining thousand sequences were very similar to known viroids, displaying high nucleotide sequence similarity. All in all, the metatranscriptome study yielded a 5.9-fold increase in viroid-like RNA diversity.
Not only are viroid-like RNAs far more diverse than initially thought, they are also a lot more widespread. Samples that came back positive for viroid-like sequences stretched the world over: Colombia, Czech Republic, Germany, USA, Japan, Malaysia, and the list goes on. Some individual sequences also spanned large geographic regions, with one sequence, for example, being found in the northern tip of Alaska, and also all the way south, in Florida.
The geographic diversity came hand-in-hand with a diversity of ecosystems. Although the vast majority of viroid-like RNAs were found in soil samples, some were also found in freshwater, in seawater, and in animal microbiomes. It is difficult to deduce host species from ecosystem samples, but using a bioinformatics pipeline called “IMG annotation”, Lee et al. were able to determine that the majority of the metatranscriptomes were dominated by prokaryotic sequences. Still, most contained at least a small portion of eukaryotic sequences as well. This indicates that viroid-like RNAs can replicate in both obscure eukaryotes and in common prokaryotes, significantly expanding the potential host range.
The work by Lee et al. helps resolve a long-standing puzzle: if viroids and viroid-like RNAs are relics from a time when RNA was the
dominant nucleic acid, why aren’t they more common? Well, as was made clear by this study, they are actually very common. In fact, many of the newly discovered viroid-like RNAs were among the most abundant sequences in their respective metatranscriptomes. As if a five-fold increase weren’t enough, the authors argue that this number is “likely a substantial underestimate of the true span of the viroid-like domain of the replicator space because among the millions of the predicted cccRNAs, in which no ribozymes were confidently identified, some, and possibly many, could be viroidlike agents containing unknown ribozymes or lacking ribozymes altogether, like pospiviroids.”
This articleoriginallyappeared in Forbes on June29, 2023 and is availableonlinehere:ViroidsandVirusoids:HidingInPlainSight
Chronic hepatitis D is the most severe form of viral hepatitis. Although it leads to similar complications as other viral hepatitis infections —scarring of the liver, liver cancer, and in extreme cases, full liver failure and death— it does so far more quickly: generally those suffering from chronic hepatitis D infection experience liver failure within five to ten years of initial infection, and 15% of people will experience liver failure as quickly as one or two years (Yurdaydın et al., 2010). Despite the severity of hepatitis D infections, many patients remain asymptomatic for an extended period. By the time you realize something is amiss, the disease has already put down deep roots.
Up until recently, the only treatment for hepatitis D consisted of prevention. As a virusoid-like pathogen, hepatitis D virus depends on a helper virus for entry into, and transmission between, cells; block the helper virus and you block hepatitis D. Thus, vaccination against hepatitis B virus —the helper virus— also offers protection against hepatitis D virus. Now, a new pharmaceutical treatment called bulevirtide has entered the scene. What follows is an overview of this novel drug, including its mechanism of action and real-world trial results.
To understand how bulevirtide works, you have to understand how hepatitis D enters host cells. Since hepatitis D is a virusoid-like
pathogen, it does not have an envelope or surface proteins of its own. An envelope protects the genetic material of a virus from damage and helps it evade recognition by the immune system. Embedded in the envelope you find surface proteins, also known as glycoproteins. These proteins act as harpoons of sorts, allowing the virus to bind to the surface of target host cells. From there, the genetic information of the virus is absorbed into the cell and the virus can begin replicating.
If hepatitis D virus lacks both of these crucial components, how does it infect cells? Well, it borrows the envelope and surface proteins of hepatitis B virus (Figure 1). Wrapped up in the jacket of another, it can successfully infiltrate host cells. This means the mechanism of entry for both hepatitis D virus and hepatitis B virus is largely identical, since they share the same envelope and surface proteins.
HBsAg, medium hepatitis B surface antigen; L-HBsAg, large hepatitis B surface antigen. SOURCE: Ypna, CC BY-SA 4.0 <https://creativecommons.org/licenses/by-sa/4.0>, via Wikimedia Commons
When a hepatitis D virion approaches a human liver cell, a region of the hepatitis B surface antigen attaches to tendril-like proteins that extend from the surface of the host cell. These tendrils, formally called heparan sulfate proteoglycans (HSPGs), pull the virion closer to the cell membrane. Once at the membrane, the large hepatitis B surface antigen hijacks and tightly binds sodium taurocholate cotransporting polypeptide (NTCP), a receptor on the surface of the liver cells (Yan et al., 2012). Usually, NTCP regulates the uptake of bile acids, which help with digestion by breaking down fats into fatty acids, but the large hepatitis B surface antigen subverts this receptor to suit its own needs.
In addition to NTCP, epidermal growth factor receptor (EGFR) is also a key player (Iwamoto et al., 2019). This receptor interacts with NTCP and triggers endocytosis, a process by which the cell membrane folds in on itself and forms a small bubble, called a vacuole, around the HDV-NTCP-EGFR complex (Figure 5). The vacuole carrying the complex is brought into the cell. A specific section of the hepatitis B surface antigen then acts as a translocation motif (TLM); essentially, a set of instructions that allow the hepatitis D viral particle to escape the membrane bubble through which it was brought into the cell (Stoeckl et al., 2006). Escaping the vacuole lands the particle in the cytoplasm of the cell, one step closer to where it wants to be: the nucleus. Once in the nucleus, hepatitis D virus can begin replicating.
Bulevirtide works by mimicking the surface protein of hepatitis B virus, thus competing with the protein for the sodium taurocholate
cotransporting polypeptide (NTCP) receptor (Cheng et al., 2021).
If bulevirtide binds the receptor first, then the hepatitis B surface proteins cannot attach themselves to the cell membrane, and by extension, cannot enter the cell — only one item can bind to the receptor at a time. By blocking cell entry, bulevirtide blocks replication as a whole.
FIGURE 2.
representation
the hepatitis D virus (HDV) entry pathway. Since hepatitis D virus uses the surface proteins of hepatitis B, cell entry for the two is thought to be similar. First, HDV particles attach to heparan sulfate proteoglycans (HSPGs) on the cell surface. The attachment is weak, but helps move the protein along to the entry main receptor: sodium taurocholate cotransporting polypeptide (NTCP). Once attached, the viral particle is absorbed into the cell via endocytosis. Next, the vesicle membrane and the virion membrane fuse together, creating an opening from which the HDV genome can escape. Finally, the HDV genome migrates into the cell nucleus where it can begin replication.
SOURCE: ACCESS Health International (Adapted from: Koichi Watashi and Takaji Wakita 2015)
Real-world studies represent the gold standard for gauging how well a pharmaceutical product works. So far, bulevirtide has proven up to the challenge, with positive results across three different realworld studies.
The first of these was performed by researchers at the Center for Liver Disease at the University of Milan. Degasperi et al. treated 18 patients with 2mg a day of bulevirtide for a period of 48 weeks (Degasperi et al., 2022). All of the patients displayed signs of liver cirrhosis and clinically significant portal hypertension, elevated pressure in the veins that lead to the liver. For five of the patients, hepatitis D RNA levels became entirely undetectable during the course of treatment. For all but two of the patients, there was a clear virological response accompanied by a decrease in hepatitis D RNA. Levels of aspartate aminotransferase and gammaglutamyltransferase two key markers of liver health also improved markedly. The only side effect experienced by the patients was an asymptomatic increase in bile acid, which is to be expected since bulevirtide targets the receptor in charge of bile acid regulation. None of the patients discontinued treatment.
The same group of researchers released a case report on a three-year course of bulevirtide treatment (Anolli et al., 2023). As before, this was a patient who already showed signs of hepatitis D-related organ damage, including liver cirrhosis and abnormal veins in the lower part of the tube running from the throat to the stomach, known as esophageal varices. Following continuous treatment for three years, levels of hepatitis D RNA and hepatitis D antigen protein dropped to undetectable levels. Again, all relevant markers of liver health showed significant improvement, including: normal liver enzymes,
preserved synthetic function, normalization of immunoglobulin G and gamma-globulins, and regression of the esophageal varices. Both the virological response and the improved liver health were maintained for the duration of a 72-week follow-up period during which the patient did not receive any bulevirtide. Barring any later flare ups, the three-year treatment essentially cured the patient of chronic hepatitis D.
Finally, scientists at the Hannover Medical School gathered data from 114 patients treated with 2mg of bulevirtide (Dietz-Fricke et al., 2023). A clinically significant decrease in hepatitis D virus RNA was observed in 76% of patients. The mean time to virologic response was 23 weeks. Again, there was noticeable improvement in liver function, even in those patients that did not achieve a significant virologic response.
The development of bulevirtide is a major step forwards; for the first time since the discovery of hepatitis D virus in 1977, patients have a treatment available to them that effectively challenges chronic infection. Not only does bulevirtide suppress the virus to undetectable levels, it also helps heal the liver damage associated with infection.
Although a victory, we would do well to remember that it is better to be overprepared than underprepared. Yes, having a functional drug to combat chronic hepatitis D is cause for celebration, but redundancy is crucial. We need to expand our quiver to ensure we stay one step ahead. This is best done by diversifying our lines of attack, making it harder for hepatitis D virus to evade treatment. For example, novel research indicates that hepatitis D may also be
transmitted by different helper viruses (Perez-Vargas et al., 2019). Since bulevirtide works by interfering specifically with the surface proteins of hepatitis B virus, these alternative helper viruses would likely be impervious.
The hepatitis D antigen proteins may prove promising targets for future drug development as they are central to the life cycle of the virus. Human RNA polymerases enzymes that help with genetic transcription are another area of interest. Hepatitis D virus hijacks these host proteins to make copies of its genome. Interrupt this process and you interrupt replication. As always, the best way forward is rigorous research: the more we know about hepatitis D virus, the better equipped we are to neutralize it.
This article originally appeared in Forbes on July 6, 2023 and is available online here: New Treatment Gives Hope to Chronic
William Haseltine, PhD
Iwould like to thank Koloman Rath for his tireless work and good cheer. I could not have attempted this book without the dedicated assistance of the ACCESS Health New York team: Courtney Biggs, Griffin McCombs, Kim Hazel, Amara Thomas, and Roberto Patarca.
I would also like to thank my Boston area colleagues at Mass CPR; the Variants Group; and the Vaccine Group.
This work is supported by ACCESS Health International (www.accessh.org).
Koloman Rath
I want to extend my sincere appreciation to Dr. William Haseltine for giving me the opportunity to work alongside him on this project. I am equally grateful to the rest of my colleagues —present and former— at ACCESS Health New York, all of whom have provided me with valuable counsel, warm words of encouragement, and unrelenting support: Courtney Biggs, Kim Hazel, Griffin McCombs, Amara Thomas, Dr. Roberto Patarca, and Jo Gurch. And of course, I cannot fail to express my gratitude to my parents, siblings, and loving partner — a building is only as solid as its foundation.
Introduction
AB118819.1. (n.d.). [dataset].
https://viroids.org/sequences/AB118819.1
AbouHaidar, M. G., Venkataraman, S., Golshani, A., Liu, B., & Ahmad, T. (2014). Novel coding, translation, and gene expression of a replicating covalently closed circular RNA of 220 nt. ProceedingsoftheNationalAcademyofSciences, 111(40), 14542–14547.
https://doi.org/10.1073/pnas.1402814111
Adams, D. (2022, December 3). An insidious blight that is ravaging West Coast marijuana crops has landed in Massachusetts. BostonGlobe.Com.
https://www.bostonglobe.com/2022/12/03/marijuana/aninsidious-blight-that-is-ravaging-west-coast-marijuana-cropslands-massachusetts-threatening-cannabis-sector/
Alfaiate, D., Lucifora, J., Abeywickrama-Samarakoon, N., Michelet, M., Testoni, B., Cortay, J.-C., Sureau, C., Zoulim, F., Dény, P., & Durantel, D. (2016). HDV RNA replication is associated with HBV repression and interferon-stimulated genes induction in super-infected hepatocytes. Antiviral Research,136 , 19–31.
https://doi.org/10.1016/j.antiviral.2016.10.006
Anolli, M. P., Degasperi, E., Allweiss, L., Sangiovanni, A., Maggioni, M., Scholtes, C., Oberhardt, V., Neumann-
Haefelin, C., Dandri, M., Zoulim, F., & Lampertico, P. (2023). A 3-year course of bulevirtide monotherapy may cure HDV infection in patients with cirrhosis. Journalof Hepatology,78(4), 876–880.
https://doi.org/10.1016/j.jhep.2022.12.023
Asselah, T., & Rizzetto, M. (2023). Hepatitis d virus infection. NewEnglandJournalofMedicine,389(1), 58–70.
https://doi.org/10.1056/NEJMra2212151
Bektaş, A., Hardwick, K. M., Waterman, K., & Kristof, J. (2019). Occurrence of hop latent viroid in cannabis sativa with symptoms of cannabis stunting disease in california. Plant Disease,103(10), 2699. https://doi.org/10.1094/PDIS-03-190459-PDN
Chang, W.-S., Pettersson, J. H.-O., Le Lay, C., Shi, M., Lo, N., Wille, M., Eden, J.-S., & Holmes, E. C. (2019). Novel hepatitis D-like agents in vertebrates and invertebrates. Virus Evolution,5(2), vez021. https://doi.org/10.1093/ve/vez021
Dark heart data shows hop latent viroid drives $4b annual losses to legal cannabis crop. (2021, August 16). GlobeNewswire News Room.
https://www.globenewswire.com/newsrelease/2021/08/16/2281335/0/en/Dark-Heart-Data-ShowsHop-Latent-Viroid-Drives-4B-Annual-Losses-to-LegalCannabis-Crop.html
Degasperi, E., Anolli, M. P., Uceda Renteria, S. C., Sambarino, D., Borghi, M., Perbellini, R., Scholtes, C., Facchetti, F., Loglio, A., Monico, S., Fraquelli, M., Costantino, A., Ceriotti, F., Zoulim, F., & Lampertico, P. (2022). Bulevirtide monotherapy for 48 weeks in patients with HDV-related
compensated cirrhosis and clinically significant portal hypertension. JournalofHepatology,77(6), 1525–1531.
https://doi.org/10.1016/j.jhep.2022.07.016
Di Serio, F., Li, S.-F., Matoušek, J., Owens, R. A., Pallás, V., Randles, J. W., Sano, T., Verhoeven, J. Th. J., Vidalakis, G., Flores, R., & ICTV Report Consortium. (2018). Ictv virus
taxonomy profile: Avsunviroidae. JournalofGeneralVirology, 99(5), 611–612.
https://doi.org/10.1099/jgv.0.001045
Di Serio, F., Owens, R. A., Li, S.-F., Matoušek, J., Pallás, V., Randles, J. W., Sano, T., Verhoeven, J. Th. J., Vidalakis, G., Flores, R., & ICTV Report Consortium. (2021). Ictv virus
taxonomy profile: Pospiviroidae. JournalofGeneralVirology, 102(2).
https://doi.org/10.1099/jgv.0.001543
Diener, T. O. (1971). Potato spindle tuber “virus”: IV. A replicating, low molecular weight RNA. Virology,45(2), 411–428.
https://doi.org/10.1016/0042-6822(71)90342-4
Diener, T. O. (1981). Are viroids escaped introns? Proceedingsof theNationalAcademyofSciences,78(8), 5014–5015.
https://doi.org/10.1073/pnas.78.8.5014
Diener, T. O. (1989). Circular RNAs: Relics of precellular evolution? ProceedingsoftheNationalAcademyofSciences, 86(23), 9370–9374.
https://doi.org/10.1073/pnas.86.23.9370
Dietz-Fricke, C., Tacke, F., Zöllner, C., Demir, M., Schmidt, H. H., Schramm, C., Willuweit, K., Lange, C. M., Weber, S., Denk, G., Berg, C. P., Grottenthaler, J. M., Merle, U., Olkus, A., Zeuzem, S., Sprinzl, K., Berg, T., Van Bömmel, F., Wiegand, J., Deterding, K. (2023). Treating hepatitis D with bulevirtide – Real-world experience from 114 patients.
JHEPReports,5(4), 100686.
https://doi.org/10.1016/j.jhepr.2023.100686
Dinter-Gottlieb, G. (1986). Viroids and virusoids are related to group I introns. ProceedingsoftheNationalAcademyof SciencesoftheUnitedStatesofAmerica,83(17), 6250–6254.
https://www.ncbi.nlm.nih.gov/pmc/articles/PMC386480/
Farci, P., & Niro, G. A. (2012). Clinical features of hepatitis D. Seminars inLiverDisease,32(3), 228–236.
https://doi.org/10.1055/s-0032-1323628
Fattovich, G., Bortolotti, F., & Donato, F. (2008). Natural history of chronic hepatitis B: Special emphasis on disease progression and prognostic factors. JournalofHepatology,48(2), 335–352.
https://doi.org/10.1016/j.jhep.2007.11.011
Flores, R., Gago-Zachert, S., Serra, P., Sanjuán, R., & Elena, S. F. (2014). Viroids: Survivors from the rna world? AnnualReview ofMicrobiology,68(1), 395–414.
https://doi.org/10.1146/annurev-micro-091313-103416
Flores, R., Gas, M.-E., Molina-Serrano, D., Nohales, M.-Á., Carbonell, A., Gago, S., De La Peña, M., & Daròs, J.-A. (2009). Viroid replication: Rolling-circles, enzymes and ribozymes. Viruses, 1(2), 317–334.
https://doi.org/10.3390/v1020317
Flores, R., Navarro, B., Serra, P., & Di Serio, F. (2022). A scenario for the emergence of protoviroids in the RNA world and for their further evolution into viroids and viroid-like RNAs by modular recombinations and mutations. VirusEvolution,8(1), veab107. https://doi.org/10.1093/ve/veab107
Girbig, M., Misiaszek, A. D., & Müller, C. W. (2022). Structural insights into nuclear transcription by eukaryotic DNAdependent RNA polymerases. Nature ReviewsMolecularCell Biology,23(9), 603–622. https://doi.org/10.1038/s41580-02200476-9
Group, A. (2020, September 16). Why the humble potato is the worlds 4th most important crop. ACIT Group.
https://acitgroup.com.au/why-the-humble-potato-is-the-worlds4th-most-important-crop/
Hammond, R. W. (2017). Chapter 1 Economic significance of viroids in vegetable and field crops. In A. Hadidi, R. Flores, J. W. Randles, & P. Palukaitis (Eds.), Viroids and Satellites (pp. 5–13). Academic Press. https://doi.org/10.1016/B978-0-12801498-1.00001-2
Intron. (n.d.). Genome.Gov. Retrieved August 10, 2023, from
https://www.genome.gov/genetics-glossary/Intron
Iwamoto, M., Shibata, Y., Kawasaki, J., Kojima, S., Li, Y.-T., Iwami, S., Muramatsu, M., Wu, H.-L., Wada, K., Tomonaga, K., Watashi, K., & Horie, M. (2021). Identification of novel avian and mammalian deltaviruses provides new insights into deltavirus evolution. VirusEvolution,7(1), veab003.
https://doi.org/10.1093/ve/veab003
Kruger, K., Grabowski, P. J., Zaug, A. J., Sands, J., Gottschling, D. E., & Cech, T. R. (1982). Self-splicing RNA: Autoexcision and autocyclization of the ribosomal RNA intervening sequence of tetrahymena. Cell,31(1), 147–157.
https://doi.org/10.1016/0092-8674(82)90414-7
Lee, B. D., & Koonin, E. V. (2022). Viroids and viroid-like circular rnas: Do they descend from primordial replicators?
Life,12(1), 103.
https://doi.org/10.3390/life12010103
Lee, B. D., Neri, U., Roux, S., Wolf, Y. I., Camargo, A. P., Krupovic, M., Simmonds, P., Kyrpides, N., Gophna, U., Dolja, V. V., & Koonin, E. V. (2023). Mining metatranscriptomes reveals a vast world of viroid-like circular RNAs. Cell,186(3), 646-661.e4.
https://doi.org/10.1016/j.cell.2022.12.039
Lee, C. Z., Chen, P. J., & Chen, D. S. (1995). Large hepatitis delta antigen in packaging and replication inhibition: Role of the carboxyl-terminal 19 amino acids and amino-terminal sequences. JournalofVirology,69(9), 5332–5336.
https://www.ncbi.nlm.nih.gov/pmc/articles/PMC189372/
Makuwa, M., Caron, M., Souquière, S., Malonga-Mouelet, G., Mahé, A., & Kazanji, M. (2008). Prevalence and genetic diversity of hepatitis B and delta viruses in pregnant women in Gabon: Molecular evidence that hepatitis delta virus clade 8 originates from and is endemic in central Africa. Journalof ClinicalMicrobiology,46(2), 754–756.
https://doi.org/10.1128/JCM.02142-07
Martínez De Alba, A. E., Flores, R., & Hernández, C. (2002). Two chloroplastic viroids induce the accumulation of small rnas associated with posttranscriptional gene silencing. Journalof Virology,76(24), 13094–13096.
https://doi.org/10.1128/JVI.76.24.13094-13096.2002
Moreno, M., Vázquez, L., López-Carrasco, A., Martín-Gago, J. A., Flores, R., & Briones, C. (2019). Direct visualization of the native structure of viroid RNAs at single-molecule resolution
by atomic force microscopy. RNABiology,16(3), 295–308.
https://doi.org/10.1080/15476286.2019.1572436
Negro, F. (2014). Hepatitis d virus coinfection and superinfection. ColdSpringHarborPerspectivesinMedicine,4(11), a021550.
https://doi.org/10.1101/cshperspect.a021550
Netter, H. J., Barrios, M. H., Littlejohn, M., & Yuen, L. K. W. (2021). Hepatitis delta virus (Hdv) and delta-like agents: Insights into their origin. Frontiers inMicrobiology,12 .
https://www.frontiersin.org/articles/10.3389/fmicb.2021.652962
Neveu, M., Kim, H.-J., & Benner, S. A. (2013). The “strong” rna world hypothesis: Fifty years old. Astrobiology,13(4), 391–403.
https://doi.org/10.1089/ast.2012.0868
Ojala, T., Kankuri, E., & Kankainen, M. (2023). Understanding human health through metatranscriptomics. Trendsin MolecularMedicine,29(5), 376–389.
https://doi.org/10.1016/j.molmed.2023.02.002
Papaefthimiou, I. (2001). Replicating potato spindle tuber viroid RNA is accompanied by short RNA fragments that are characteristic of post-transcriptional gene silencing. Nucleic AcidsResearch,29(11), 2395–2400.
https://doi.org/10.1093/nar/29.11.2395
Perez-Vargas, J., Amirache, F., Boson, B., Mialon, C., Freitas, N., Sureau, C., Fusil, F., & Cosset, F.-L. (2019). Enveloped viruses distinct from HBV induce dissemination of hepatitis D virus in vivo. NatureCommunications,10(1), 2098.
https://doi.org/10.1038/s41467-019-10117-z
Poretsky, R. S., Bano, N., Buchan, A., LeCleir, G., Kleikemper, J., Pickering, M., Pate, W. M., Moran, M. A., & Hollibaugh, J. T. (2005). Analysis of microbial gene transcripts in environmental samples. AppliedandEnvironmentalMicrobiology,71(7), 4121–4126.
https://doi.org/10.1128/AEM.71.7.4121-4126.2005
Qin, S., Tang, X., Chen, Y., Chen, K., Fan, N., Xiao, W., Zheng, Q., Li, G., Teng, Y., Wu, M., & Song, X. (2022). Mrna-based therapeutics: Powerful and versatile tools to combat diseases. SignalTransductionandTargetedTherapy,7(1), 1–35.
https://doi.org/10.1038/s41392-022-01007-w
Ramesh, S. V., Yogindran, S., Gnanasekaran, P., Chakraborty, S., Winter, S., & Pappu, H. R. (2021). Virus and viroid-derived small rnas as modulators of host gene expression: Molecular insights into pathogenesis. Frontiers inMicrobiology,11
https://www.frontiersin.org/articles/10.3389/fmicb.2020.614231
Rizzetto, M. (1983). Chronic hepatitis in carriers of hepatitis b surface antigen, with intrahepatic expression of the delta antigen: An active and progressive disease unresponsive to immunosuppressive treatment. AnnalsofInternalMedicine, 98(4), 437. https://doi.org/10.7326/0003-4819-98-4-437
Rodriguez, M. J. B., Vadamalai, G., & Randles, J. W. (2017). Economic significance of palm tree viroids. In Viroids and Satellites (pp. 39–49). Elsevier . https://doi.org/10.1016/B978-012-801498-1.00004-8
Sah, R., Rodriguez-Morales, A. J., Jha, R., Chu, D. K. W., Gu, H., Peiris, M., Bastola, A., Lal, B. K., Ojha, H. C., Rabaan, A. A., Zambrano, L. I., Costello, A., Morita, K., Pandey, B. D., & Poon, L. L. M. (2020). Complete genome sequence of a 2019
novel coronavirus (SARS-CoV-2) strain isolated in nepal. MicrobiologyResourceAnnouncements,9(11), e00169-20.
https://doi.org/10.1128/MRA.00169-20
Saito, H. (2022). The RNA world ‘hypothesis.’ Nature Reviews MolecularCellBiology,23(9), 582–582.
https://doi.org/10.1038/s41580-022-00514-6
Santos, M. D. C., Gomes-Gouvêa, M. S., Nunes, J. D. C., Barros, L. M. F., Carrilho, F. J., Ferreira, A. de S. P., & Pinho, J. R. R. (2016). The hepatitis delta genotype 8 in Northeast Brazil: The North Atlantic slave trade as the potential route for infection. VirusResearch,224 , 6–11.
https://doi.org/10.1016/j.virusres.2016.08.003
Saucedo-Carabez, J. R., Téliz-Ortiz, D., Ochoa-Ascencio, S., Ochoa-Martínez, D., Vallejo-Pérez, M. R., & Beltrán-Peña, H. (2014). Effect of Avocado sunblotch viroid (Asbvd) on avocado yield in Michoacan, Mexico. EuropeanJournalofPlant Pathology,138(4), 799–805. https://doi.org/10.1007/s10658013-0354-9
Scheck, H. (2020, June 1). Hop latent viroid. Pest Rating Proposals and Final Ratings.
https://blogs.cdfa.ca.gov/Section3162/?p=7262
Shah, P. A., Choudhry, S., Reyes, K. J. C., & Lau, D. T. Y. (2019). An update on the management of chronic hepatitis D. GastroenterologyReport,7(6), 396–402.
https://doi.org/10.1093/gastro/goz052
Sharma, U., Watpade, S., Gupta, B., Raigond, B., Kumari, N., Bhardwaj, P., Handa, A., & Gupta, P. (2020). Economic losses due to infection by apple scar skin viroid in Himachal Pradesh,
India. VirusDisease,31(4), 490–496.
https://doi.org/10.1007/s13337-020-00625-8
Symons, R. H. (1999). Ribozymes. In Encyclopedia of Virology (pp. 1551–1559). Elsevier.
https://doi.org/10.1006/rwvi.1999.0254
Taylor, J. M. (2015). Hepatitis d virus replication. ColdSpring HarborPerspectivesinMedicine,5(11), a021568.
https://doi.org/10.1101/cshperspect.a021568
The nobel prize in chemistry 1989. (n.d.). NobelPrize.Org.
Retrieved August 10, 2023, from
https://www.nobelprize.org/prizes/chemistry/1989/summary/
Tseligka, E. D., Clément, S., & Negro, F. (2021). Hdv pathogenesis: Unravelling ariadne’s thread. Viruses,13(5), 778.
https://doi.org/10.3390/v13050778
Urban, S., Neumann-Haefelin, C., & Lampertico, P. (2021). Hepatitis D virus in 2021: Virology, immunology and new treatment approaches for a difficult-to-treat disease. Gut,70(9), 1782–1794.
https://doi.org/10.1136/gutjnl-2020-323888
Usman, Z., Velkov, S., Protzer, U., Roggendorf, M., Frishman, D., & Karimzadeh, H. (2020). Hdvdb: A comprehensive hepatitis d virus database. Viruses, 12(5), 538.
https://doi.org/10.3390/v12050538
Vernière, C., Perrier, X., Dubois, C., Dubois, A., Botella, L., Chabrier, C., Bové, J. M., & Vila, N. D. (2004). Citrus viroids: Symptom expression and effect on vegetative growth and yield of clementine trees grafted on trifoliate orange. PlantDisease,
88(11), 1189–1197.
https://doi.org/10.1094/PDIS.2004.88.11.1189
Viroid: Usda ars. (n.d.). Retrieved August 10, 2023, from
https://www.ars.usda.gov/oc/timeline/viroid/ Wang, Y. (2021). Current view and perspectives in viroid replication. CurrentOpinioninVirology,47 , 32–37.
https://doi.org/10.1016/j.coviro.2020.12.004
What is hepatitis D - faq | cdc. (2020, December 3).
https://www.cdc.gov/hepatitis/hdv/hdvfaq.htm
Wille, M., Netter, H., Littlejohn, M., Yuen, L., Shi, M., Eden, J.S., Klaassen, M., Holmes, E., & Hurt, A. (2018). A divergent hepatitis d-like agent in birds. Viruses, 10(12), 720.
https://doi.org/10.3390/v10120720
Williams, V., Brichler, S., Radjef, N., Lebon, P., Goffard, A., Hober, D., Fagard, R., Kremsdorf, D., Dény, P., & Gordien, E. (2009). Hepatitis delta virus proteins repress hepatitis B virus enhancers and activate the alpha/beta interferon-inducible MxA gene. JournalofGeneralVirology,90(11), 2759–2767.
https://doi.org/10.1099/vir.0.011239-0
Yamaguchi, Y., Filipovska, J., Yano, K., Furuya, A., Inukai, N., Narita, T., Wada, T., Sugimoto, S., Konarska, M. M., & Handa, H. (2001). Stimulation of rna polymerase ii elongation by hepatitis delta antigen. Science,293(5527), 124–127.
https://doi.org/10.1126/science.1057925
Zi, J., Gao, X., Du, J., Xu, H., Niu, J., & Chi, X. (2022). Multiple regions drive hepatitis delta virus proliferation and are
therapeutic targets. Frontiers inMicrobiology,13 .
https://www.frontiersin.org/articles/10.3389/fmicb.2022.838382 (N.d.). Retrieved August 10, 2023, from
https://newfrontierdata.com/cannabis-insights/new-statemarkets-could-boost-u-s-legal-cannabis-sales-to-72b-by-2030/
Viroids
50+cannabisindustrystatistics for2023|embroker.(2023, January 30). https://www.embroker.com/blog/cannabisindustry-statistics/
Adkar-Purushothama, C. R., & Perreault, J.-P. (2018). Alterations of the viroid regions that interact with the host defense genes attenuate viroid infection in host plant. RNA Biology, 15(7), 955–966.
https://doi.org/10.1080/15476286.2018.1462653
Agrawal, N., Dasaradhi, P. V. N., Mohmmed, A., Malhotra, P., Bhatnagar, R. K., & Mukherjee, S. K. (2003). Rna interference: Biology, mechanism, and applications. Microbiology and Molecular Biology Reviews, 67(4), 657–685.
https://doi.org/10.1128/MMBR.67.4.657-685.2003
Aviña-Padilla, K., Rivera-Bustamante, R., Kovalskaya, N. Y., & Hammond, R. W. (2018). Pospiviroid infection of tomato regulates the expression of genes involved in flower and fruit development. Viruses, 10(10), 516.
https://doi.org/10.3390/v10100516
Diener, T. O., Owens, R. A., & Hammond, R. W. (1993). Viroids: The smallest and simplest agents of infectious disease. How do they make plants sick? Intervirology, 35(1–4), 186–195.
https://doi.org/10.1159/000150309
Diermann, N., Matoušek, J., Junge, M., Riesner, D., & Steger, G. (2010). Characterization of plant miRNAs and small RNAs derived from potato spindle tuber viroid (Pstvd) in infected tomato. 391(12), 1379–1390.
https://doi.org/10.1515/bc.2010.148
Ferdman, R. A. (2015, January 22). The rise of the avocado, America’s new favorite fruit. Washington Post.
https://www.washingtonpost.com/news/wonk/wp/2015/01/22/th e-sudden-rise-of-the-avocado-americas-new-favorite-fruit/
Hepatitis d Niddk.(n.d.).National Institute of Diabetes and Digestive and Kidney Diseases. Retrieved August 11, 2023, from https://www.niddk.nih.gov/health-information/liverdisease/viral-hepatitis/hepatitis-d
MacFarlane, L.-A., & Murphy, P. R. (2010). Microrna: Biogenesis, function and role in cancer. Current Genomics, 11(7), 537–561. https://doi.org/10.2174/138920210793175895
Morrissey, K. (2022). New State Markets Could Boost U.S. Legal Cannabis Sales to $72B by 2030.
https://newfrontierdata.com/cannabis-insights/new-statemarkets-could-boost-u-s-legal-cannabis-sales-to-72b-by-2030/
Ortolá, B., & Daròs, J.-A. (2023). Viroids: Non-coding circular rnas able to autonomously replicate and infect higher plants.
Biology, 12(2), 172.
https://doi.org/10.3390/biology12020172
Potato|land&water|foodandagricultureorganization ofthe UnitedNations. (n.d.). Retrieved August 11, 2023, from
https://www.fao.org/land-water/databases-and-software/cropinformation/potato/en/
Potter, H. (2014). David H. Dressler 1941–2014. Nature Genetics, 46(10), 1044–1044. https://doi.org/10.1038/ng.3099
Ritchie, H., & Roser, M. (2021). Forests and deforestation. Our World in Data. https://ourworldindata.org/palm-oil
Sah, R., Rodriguez-Morales, A. J., Jha, R., Chu, D. K. W., Gu, H., Peiris, M., Bastola, A., Lal, B. K., Ojha, H. C., Rabaan, A. A., Zambrano, L. I., Costello, A., Morita, K., Pandey, B. D., & Poon, L. L. M. (2020). Complete genome sequence of a 2019 novel coronavirus (SARS-CoV-2) strain isolated in nepal.
Microbiology Resource Announcements, 9(11), e00169-20.
https://doi.org/10.1128/MRA.00169-20
Shahbandeh, M. (2022, July 27). Domestic avocado consumption U.S. 2021. Statista.
https://www.statista.com/statistics/591263/average-avocadoconsumption-us-per-week/
Singh, R. P. (2014). The discovery and eradication of potato spindle tuber viroid in Canada. VirusDisease, 25(4), 415–424.
https://doi.org/10.1007/s13337-014-0225-9
Singh, R. P., Finnie, R. E., & Bagnall, R. H. (1971). Losses due to the potato spindle tuber virus. American Potato Journal, 48(7), 262–267.
https://doi.org/10.1007/BF02861592
Vadamalai, G., Thanarajoo, S. S., Joseph, H., Kong, L. L., & Randles, J. W. (2017). Chapter 25 Coconut cadang-cadang viroid and coconut tinangaja viroid. In A. Hadidi, R. Flores, J. W. Randles, & P. Palukaitis (Eds.), Viroids and Satellites (pp. 263–273). Academic Press. https://doi.org/10.1016/B978-0-12801498-1.00025-5
Verhoeven, J. Th. J. (2022). Potato spindle tuber viroid (Spindle tuber of potato). CABI Compendium, CABI Compendium, 43659.
https://doi.org/10.1079/cabicompendium.43659
Warren, J. G., Mercado, J., & Grace, D. (2019). Occurrence of hop latent viroid causing disease in cannabis sativa in california. Plant Disease, 103(10), 2699–2699.
https://doi.org/10.1094/PDIS-03-19-0530-PDN
Whatis rnai Rnaibiology.(2013, November 3). UMass Chan Medical School.
https://www.umassmed.edu/rti/biology/rna/how-rnai-works/
AbouHaidar, M. G., Venkataraman, S., Golshani, A., Liu, B., & Ahmad, T. (2014). Novel coding, translation, and gene expression of a replicating covalently closed circular RNA of 220 nt. ProceedingsoftheNationalAcademyofSciences, 111(40),14542–14547.
https://doi.org/10.1073/pnas.1402814111
Collins, R. F., Gellatly, D. L., Sehgal, O. P., & Abouhaidar, M. G. (1998). Self-cleaving circular rna associated with rice yellow mottle virus is the smallest viroid-like rna. Virology,241(2), 269–275.https://doi.org/10.1006/viro.1997.8962
Davies, C., Haseloff, J., & Symons, R. H. (1990). Structure, selfcleavage, and replication of two viroid-like satellite RNAs (Virusoids) of subterranean clover mottle virus. Virology, 177(1),216–224.https://doi.org/10.1016/0042-6822(90)904757
Flores, R., Grubb, D., Elleuch, A., Nohales, M.-Á., Delgado, S., & Gago, S. (2011). Rolling-circle replication of viroids, viroid-like satellite RNAs and hepatitis delta virus: Variations on a theme.
RNABiology,8(2),200–206.
https://doi.org/10.4161/rna.8.2.14238
Mailliot, J., & Martin, F. (2018). Viral internal ribosomal entry sites: Four classes for one goal. WIREsRNA,9(2).
https://doi.org/10.1002/wrna.1458
Rao, A. L. N., & Kalantidis, K. (2015). Virus-associated small satellite RNAs and viroids display similarities in their replication strategies. Virology,479–480,627–636.
https://doi.org/10.1016/j.virol.2015.02.018
Abbas, Z., & Afzal, R. (2013). Life cycle and pathogenesis of hepatitis D virus: A review. WorldJournalofHepatology , 5(12), 666–675. https://doi.org/10.4254/wjh.v5.i12.666
Abeywickrama-Samarakoon, N., Cortay, J.-C., Sureau, C., Müller, S., Alfaiate, D., Guerrieri, F., Chaikuad, A., Schröder, M., Merle, P., Levrero, M., & Dény, P. (2020). Hepatitis Delta Virus histone mimicry drives the recruitment of chromatin remodelers for viral RNA replication. Nature
Communications,11(1), 419. https://doi.org/10.1038/s41467020-14299-9
Anolli, M. P., Degasperi, E., Allweiss, L., Sangiovanni, A., Maggioni, M., Scholtes, C., Oberhardt, V., NeumannHaefelin, C., Dandri, M., Zoulim, F., & Lampertico, P. (2023). A 3-year course of bulevirtide monotherapy may cure
HDV infection in patients with cirrhosis. Journalof Hepatology,78(4), 876–880.
https://doi.org/10.1016/j.jhep.2022.12.023
Canese, M. G., Rizzetto, M., Novara, R., London, W. T., & Purcell, R. H. (1984). Experimental infection of chimpanzees with the HBsAg-Associated delta (Δdl) agent: An ultrastructural study. JournalofMedicalVirology,13(1), 63–72.
https://doi.org/10.1002/jmv.1890130108
Cao, D., Haussecker, D., Huang, Y., & Kay, M. A. (2009). Combined proteomic–RNAi screen for host factors involved in human hepatitis delta virus replication. RNA,15(11), 1971–1979. https://doi.org/10.1261/rna.1782209
Chen, H.-Y., Shen, D.-T., Ji, D.-Z., Han, P.-C., Zhang, W.-M., Ma, J.-F., Chen, W.-S., Goyal, H., Pan, S., & Xu, H.-G. (2019). Prevalence and burden of hepatitis D virus infection in the global population: A systematic review and meta-analysis.
Gut,68(3), 512–521. https://doi.org/10.1136/gutjnl-2018316601
Cheng, D., Han, B., Zhang, W., & Wu, W. (2021). Clinical effects of NTCP‐inhibitor myrcludex B. JournalofViral Hepatitis, 28(6), 852–858. https://doi.org/10.1111/jvh.13490
Choi, S., Jeong, S., & Hwang, S. B. (2007). Large hepatitis delta antigen modulates transforming growth factor-β signaling cascades: Implication of hepatitis delta virus–induced liver fibrosis. Gastroenterology,132(1), 343–357.
https://doi.org/10.1053/j.gastro.2006.10.038
Cole, S. M., Gowans, E. J., Macnaughton, T. B., Hall, P. D., & Burrell, C. J. (1991). Direct evidence for cytotoxicity associated
with expression of hepatitis delta virus antigen. Hepatology (Baltimore,Md.),13(5), 845–851.
Degasperi, E., Anolli, M. P., Uceda Renteria, S. C., Sambarino, D., Borghi, M., Perbellini, R., Scholtes, C., Facchetti, F., Loglio, A., Monico, S., Fraquelli, M., Costantino, A., Ceriotti, F., Zoulim, F., & Lampertico, P. (2022). Bulevirtide monotherapy for 48 weeks in patients with HDV-related compensated cirrhosis and clinically significant portal hypertension. JournalofHepatology,77(6), 1525–1531.
https://doi.org/10.1016/j.jhep.2022.07.016
Dietz-Fricke, C., Tacke, F., Zöllner, C., Demir, M., Schmidt, H. H., Schramm, C., Willuweit, K., Lange, C. M., Weber, S., Denk, G., Berg, C. P., Grottenthaler, J. M., Merle, U., Olkus, A., Zeuzem, S., Sprinzl, K., Berg, T., Van Bömmel, F., Wiegand, J., … Deterding, K. (2023). Treating hepatitis D with bulevirtide – Real-world experience from 114 patients. JHEPReports,5(4), 100686.
https://doi.org/10.1016/j.jhepr.2023.100686
Fabregat, I., Moreno-Càceres, J., Sánchez, A., Dooley, S., Dewidar, B., Giannelli, G., Ten Dijke, P., & the IT-LIVER Consortium. (2016). TGF-β signalling and liver disease. The FEBSJournal,283(12), 2219–2232.
https://doi.org/10.1111/febs.13665
Farci, P., Niro, G. A., Zamboni, F., & Diaz, G. (2021). Hepatitis d virus and hepatocellular carcinoma. Viruses,13(5), 830.
https://doi.org/10.3390/v13050830
Fu, T. B., & Taylor, J. (1993). The RNAs of hepatitis delta virus are copied by RNA polymerase II in nuclear homogenates.
JournalofVirology,67(12), 6965–6972.
https://doi.org/10.1128/jvi.67.12.6965-6972.1993
Genbank overview. (n.d.). Retrieved August 16, 2023, from
https://www.ncbi.nlm.nih.gov/genbank/ Global progress report on HIV, viral hepatitis and sexually transmitted infections, 2021. (2021, July 15). World Health Organization. https://www.who.int/publications-detailredirect/9789240027077
Gozmanova, M. (2003). Characterization of the RNA motif responsible for the specific interaction of potato spindle tuber viroid RNA (Pstvd) and the tomato protein Virp1. Nucleic AcidsResearch,31(19), 5534–5543.
https://doi.org/10.1093/nar/gkg777
Greco-Stewart, V. S., Miron, P., Abrahem, A., & Pelchat, M. (2007). The human RNA polymerase II interacts with the terminal stem–loop regions of the hepatitis delta virus RNA genome. Virology,357(1), 68–78.
https://doi.org/10.1016/j.virol.2006.08.010
Greco-Stewart, V. S., Schissel, E., & Pelchat, M. (2009). The hepatitis delta virus RNA genome interacts with the human RNA polymerases I and III. Virology,386(1) , 12–15.
https://doi.org/10.1016/j.virol.2009.02.007
Greene, J., Baird, A.-M., Brady, L., Lim, M., Gray, S. G., McDermott, R., & Finn, S. P. (2017). Circular rnas: Biogenesis,
in MolecularBiosciences,4
https://www.frontiersin.org/articles/10.3389/fmolb.2017.00038
function and role in human diseases. Frontiers
Guilhot, S., Huang, S. N., Xia, Y. P., La Monica, N., Lai, M. M., & Chisari, F. V. (1994). Expression of the hepatitis delta virus large and small antigens in transgenic mice. Journalof Virology,68(2), 1052–1058.
https://doi.org/10.1128/jvi.68.2.1052-1058.1994
Hetzel, U., Szirovicza, L., Smura, T., Prähauser, B., Vapalahti, O., Kipar, A., & Hepojoki, J. (2019). Identification of a novel deltavirus in boa constrictors. mBio,10(2), e00014-19.
https://doi.org/10.1128/mBio.00014-19
Huang, W.-H., Mai, R.-T., & Wu Lee, Y.-H. (2008). Transcription factor yy1 and its associated acetyltransferases cbp and p300 interact with hepatitis delta antigens and modulate hepatitis delta virus rna replication. JournalofVirology,82(15), 7313–7324.
https://doi.org/10.1128/JVI.02581-07
Hugouvieux-Cotte-Pattat, N., Condemine, G., & Shevchik, V. E. (2014). Bacterial pectate lyases, structural and functional diversity. EnvironmentalMicrobiologyReports, 6(5), 427–440.
https://doi.org/10.1111/1758-2229.12166
Hwang, S. B., & Lai, M. M. (1993). Isoprenylation mediates direct protein-protein interactions between hepatitis large delta antigen and hepatitis B virus surface antigen. Journalof Virology,67(12), 7659–7662.
https://doi.org/10.1128/jvi.67.12.7659-7662.1993
Iwamoto, M., Saso, W., Sugiyama, R., Ishii, K., Ohki, M., Nagamori, S., Suzuki, R., Aizaki, H., Ryo, A., Yun, J.-H., Park, S.-Y., Ohtani, N., Muramatsu, M., Iwami, S., Tanaka, Y., Sureau, C., Wakita, T., & Watashi, K. (2019). Epidermal growth factor receptor is a host-entry cofactor triggering
hepatitis B virus internalization. ProceedingsoftheNational AcademyofSciences,116(17), 8487–8492.
https://doi.org/10.1073/pnas.1811064116
Iwamoto, M., Shibata, Y., Kawasaki, J., Kojima, S., Li, Y.-T., Iwami, S., Muramatsu, M., Wu, H.-L., Wada, K., Tomonaga, K., Watashi, K., & Horie, M. (2021). Identification of novel avian and mammalian deltaviruses provides new insights into deltavirus evolution. VirusEvolution,7(1), veab003.
https://doi.org/10.1093/ve/veab003
Lee, B. D., & Koonin, E. V. (2022). Viroids and viroid-like circular rnas: Do they descend from primordial replicators?
Life,12(1), 103. https://doi.org/10.3390/life12010103
Lee, B. D., Neri, U., Roux, S., Wolf, Y. I., Camargo, A. P., Krupovic, M., Simmonds, P., Kyrpides, N., Gophna, U., Dolja, V. V., & Koonin, E. V. (2023). Mining metatranscriptomes reveals a vast world of viroid-like circular RNAs. Cell,186(3), 646-661.e4.
https://doi.org/10.1016/j.cell.2022.12.039
Lee, C.-Z., & Sheu, J.-C. (2008). Histone H1e interacts with small hepatitis delta antigen and affects hepatitis delta virus replication. Virology,375(1), 197–204.
https://doi.org/10.1016/j.virol.2008.02.003
Lefkowitch, J. H., Goldstein, H., Yatto, R., & Gerber, M. A. (1987). Cytopathic liver injury in acute delta virus hepatitis. Gastroenterology,92(5), 1262–1266.
https://doi.org/10.1016/S0016-5085(87)91086-9
Li, J., Hsu, H.-C., Mountz, J. D., & Allen, J. G. (2018).
Unmasking fucosylation: From cell adhesion to immune
system regulation and diseases. CellChemicalBiology,25(5), 499–512. https://doi.org/10.1016/j.chembiol.2018.02.005
Macnaughton, T. B., & Lai, M. M. C. (2006). HDV RNA replication: Ancient relic or primer? CurrentTopics in MicrobiologyandImmunology,307 , 25–45.
https://doi.org/10.1007/3-540-29802-9_2
Mentha, N., Clément, S., Negro, F., & Alfaiate, D. (2019). A review on hepatitis D: From virology to new therapies. Journal ofAdvancedResearch,17 , 3–15.
https://doi.org/10.1016/j.jare.2019.03.009
Nagata, S., Kiyohara, R., & Toh, H. (2021). Constraint of base pairing on hdv genome evolution. Viruses, 13(12), 2350.
https://doi.org/10.3390/v13122350
NCI Dictionary of Cancer Terms. (2011, February 2). National Cancer Institute .
https://www.cancer.gov/publications/dictionaries/cancerterms/def/transcriptomics
Ojala, T., Kankuri, E., & Kankainen, M. (2023). Understanding human health through metatranscriptomics. Trendsin MolecularMedicine,29(5), 376–389.
https://doi.org/10.1016/j.molmed.2023.02.002
Perez-Vargas, J., Amirache, F., Boson, B., Mialon, C., Freitas, N., Sureau, C., Fusil, F., & Cosset, F.-L. (2019). Enveloped viruses distinct from HBV induce dissemination of hepatitis D virus in vivo. Nature Communications, 10(1), 2098.
https://doi.org/10.1038/s41467-019-10117-z
Poretsky, R. S., Bano, N., Buchan, A., LeCleir, G., Kleikemper, J., Pickering, M., Pate, W. M., Moran, M. A., & Hollibaugh, J. T. (2005). Analysis of microbial gene transcripts in environmental samples. AppliedandEnvironmentalMicrobiology,71(7), 4121–4126. https://doi.org/10.1128/AEM.71.7.4121-4126.2005
Rizzetto, M., Canese, M. G., Aricò, S., Crivelli, O., Trepo, C., Bonino, F., & Verme, G. (1977). Immunofluorescence detection of new antigen-antibody system (Delta/anti-delta) associated to hepatitis B virus in liver and in serum of HBsAg carriers. Gut,18(12), 997–1003.
https://doi.org/10.1136/gut.18.12.997
Rizzetto, M., Canese, M. G., Gerin, J. L., London, W. T., Sly, D. L., & Purcell, R. H. (1980). Transmission of the hepatitis b virus-associated delta antigen to chimpanzees. Journalof InfectiousDiseases, 141(5), 590–602.
https://doi.org/10.1093/infdis/141.5.590
Rizzetto, M., Hoyer, B., Canese, M. G., Shih, J. W., Purcell, R. H., & Gerin, J. L. (1980). Delta agent: Association of delta antigen with hepatitis b surface antigen and rna in serum of delta-infected chimpanzees. ProceedingsoftheNational AcademyofSciences,77(10), 6124–6128.
https://doi.org/10.1073/pnas.77.10.6124
Spaan, M., Carey, I., Bruce, M., Shang, D., Horner, M., Dusheiko, G., & Agarwal, K. (2020). Hepatitis delta genotype 5 is associated with favourable disease outcome and better response to treatment compared to genotype 1. Journalof Hepatology,72(6), 1097–1104.
https://doi.org/10.1016/j.jhep.2019.12.028
Stockdale, A. J., Kreuels, B., Henrion, M. Y. R., Giorgi, E., Kyomuhangi, I., De Martel, C., Hutin, Y., & Geretti, A. M. (2020). The global prevalence of hepatitis D virus infection: Systematic review and meta-analysis. JournalofHepatology, 73(3), 523–532.
https://doi.org/10.1016/j.jhep.2020.04.008
Stoeckl, L., Funk, A., Kopitzki, A., Brandenburg, B., Oess, S., Will, H., Sirma, H., & Hildt, E. (2006). Identification of a structural motif crucial for infectivity of hepatitis B viruses. Proceedings of the National AcademyofSciences,103(17), 6730–6734.
https://doi.org/10.1073/pnas.0509765103
Sutherland, B. J. G., Finke, J. F., Saunders, R., Warne, S., Schulze, A. D., Strohm, J. H. T., Chan, A. M., Suttle, C. A., & Miller, K. M. (2022). Metatranscriptomics reveals a shift in microbial community composition and function during summer months in a coastal marine environment.
EnvironmentalDNA,edn3.353 .
https://doi.org/10.1002/edn3.353
Wang, K.-S., Choo, Q.-L., Weiner, A. J., Ou, J.-H., Najarian, R. C., Thayer, R. M., Mullenbach, G. T., Denniston, K. J., Gerin, J. L., & Houghton, M. (1986). Structure, sequence and expression of the hepatitis delta (Δ) viral genome. Nature, 323(6088), 508–514. https://doi.org/10.1038/323508a0
Wedemeyer, H., & Negro, F. (2019). Devil hepatitis D: An orphan disease or largely underdiagnosed? Gut,68(3), 381–382.
https://doi.org/10.1136/gutjnl-2018-317403
Whatishepatitisd Faq|cdc.(2020, December 3).
https://www.cdc.gov/hepatitis/hdv/hdvfaq.htm
White, R. J. (2013). Rna polymerase i and rna polymerase iii in eukaryotes. In EncyclopediaofBiologicalChemistry(pp. 159–161). Elsevier. https://doi.org/10.1016/B978-0-12-3786302.00263-2
Wille, M., Netter, H. J., Littlejohn, M., Yuen, L., Shi, M., Eden, J.-S., Klaassen, M., Holmes, E. C., & Hurt, A. C. (2018). A divergent hepatitis d-like agent in birds. Viruses,10(12), 720.
https://doi.org/10.3390/v10120720
Yamaguchi, Y., Filipovska, J., Yano, K., Furuya, A., Inukai, N., Narita, T., Wada, T., Sugimoto, S., Konarska, M. M., & Handa, H. (2001). Stimulation of rna polymerase ii elongation by hepatitis delta antigen. Science,293(5527), 124–127.
https://doi.org/10.1126/science.1057925
Yamaguchi, Y., Mura, T., Chanarat, S., Okamoto, S., & Handa, H. (2007). Hepatitis delta antigen binds to the clamp of RNA polymerase II and affects transcriptional fidelity. Genesto Cells,12(7), 863–875. https://doi.org/10.1111/j.13652443.2007.01094.x
Yan, H., Zhong, G., Xu, G., He, W., Jing, Z., Gao, Z., Huang, Y., Qi, Y., Peng, B., Wang, H., Fu, L., Song, M., Chen, P., Gao, W., Ren, B., Sun, Y., Cai, T., Feng, X., Sui, J., & Li, W. (2012). Sodium taurocholate cotransporting polypeptide is a functional receptor for human hepatitis B and D virus. eLife, 1 , e00049. https://doi.org/10.7554/eLife.00049
Yurdaydın, C., Idilman, R., Bozkaya, H., & Bozdayi, A. M. (2010). Natural history and treatment of chronic delta hepatitis: Chronic delta hepatitis. JournalofViralHepatitis,17(11), 749–756. https://doi.org/10.1111/j.1365-2893.2010.01353.x
Zhou, Y., Fukuda, T., Hang, Q., Hou, S., Isaji, T., Kameyama, A., & Gu, J. (2017). Inhibition of fucosylation by 2-fluorofucose suppresses human liver cancer HepG2 cell proliferation and migration as well as tumor formation. ScientificReports,7(1), 11563.
https://doi.org/10.1038/s41598-017-11911-9
Zi, J., Gao, X., Du, J., Xu, H., Niu, J., & Chi, X. (2022). Multiple regions drive hepatitis delta virus proliferation and are therapeutic targets. Frontiers inMicrobiology,13 .
https://www.frontiersin.org/articles/10.3389/fmicb.2022.838382