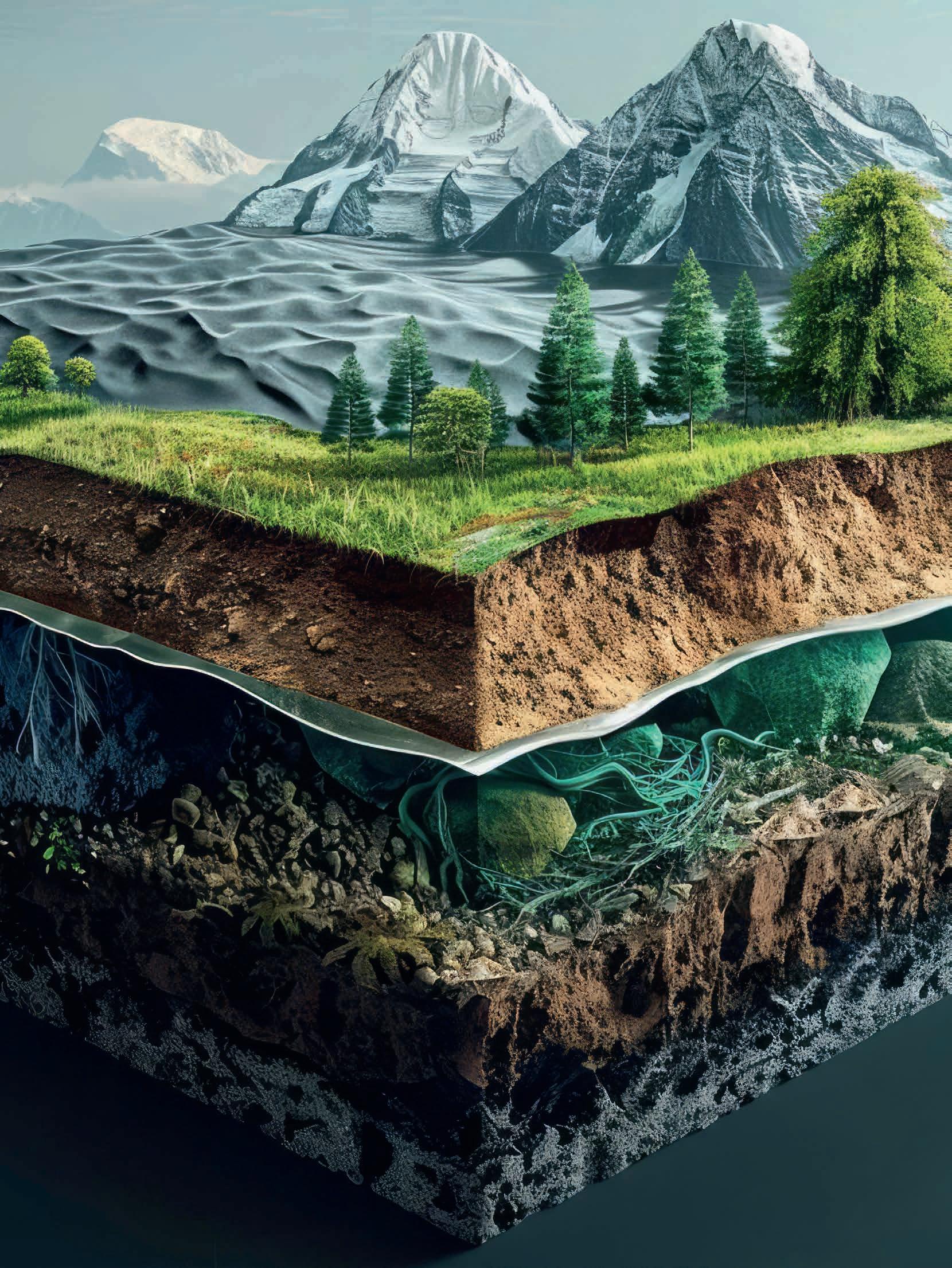
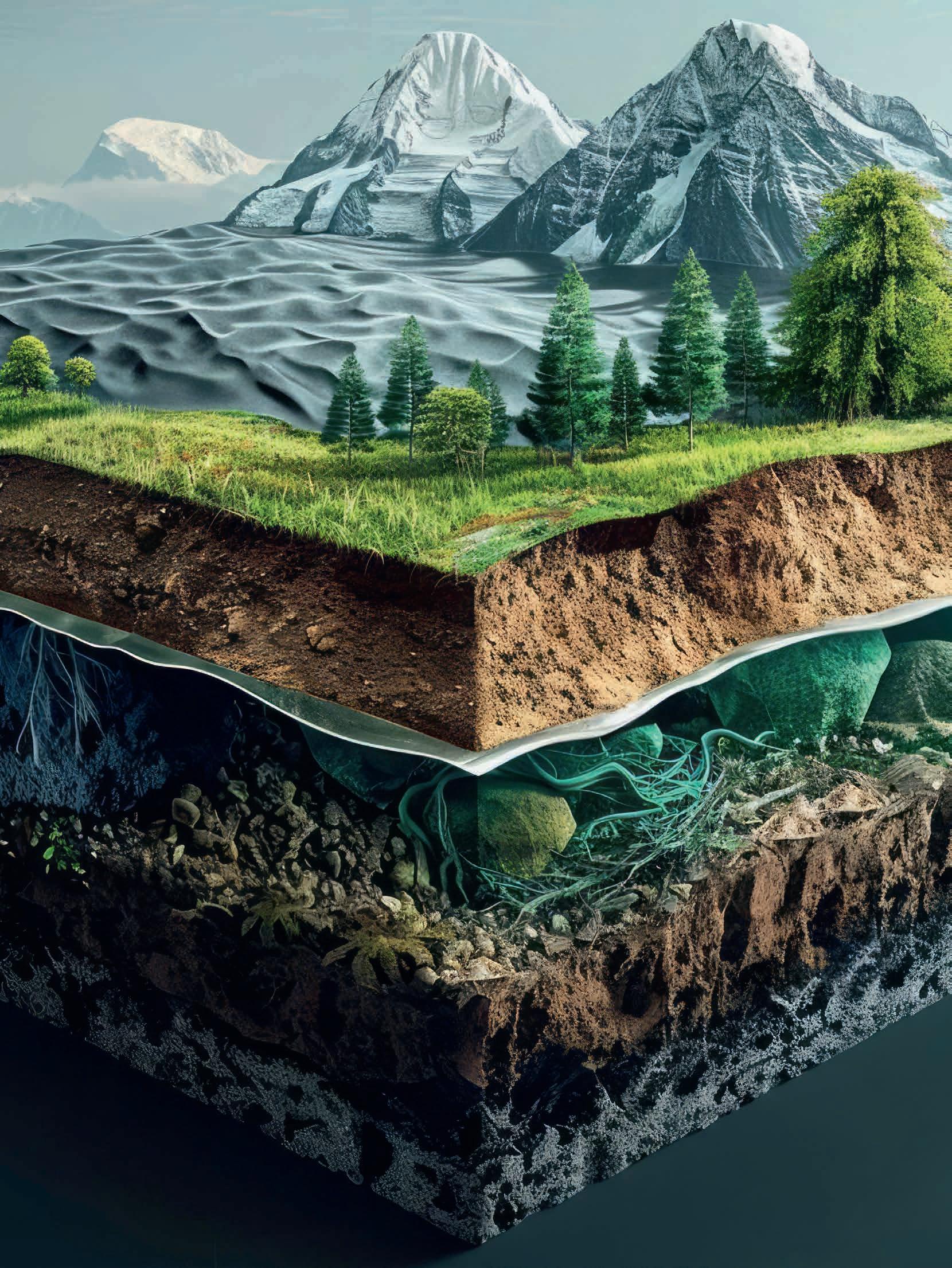
Energy Transition
EAGE NEWS B iggest energy transition event is almost here
TECHNICAL ARTICLE AVO leads identified offshore South Africa CROSSTALK Geoscience in sport
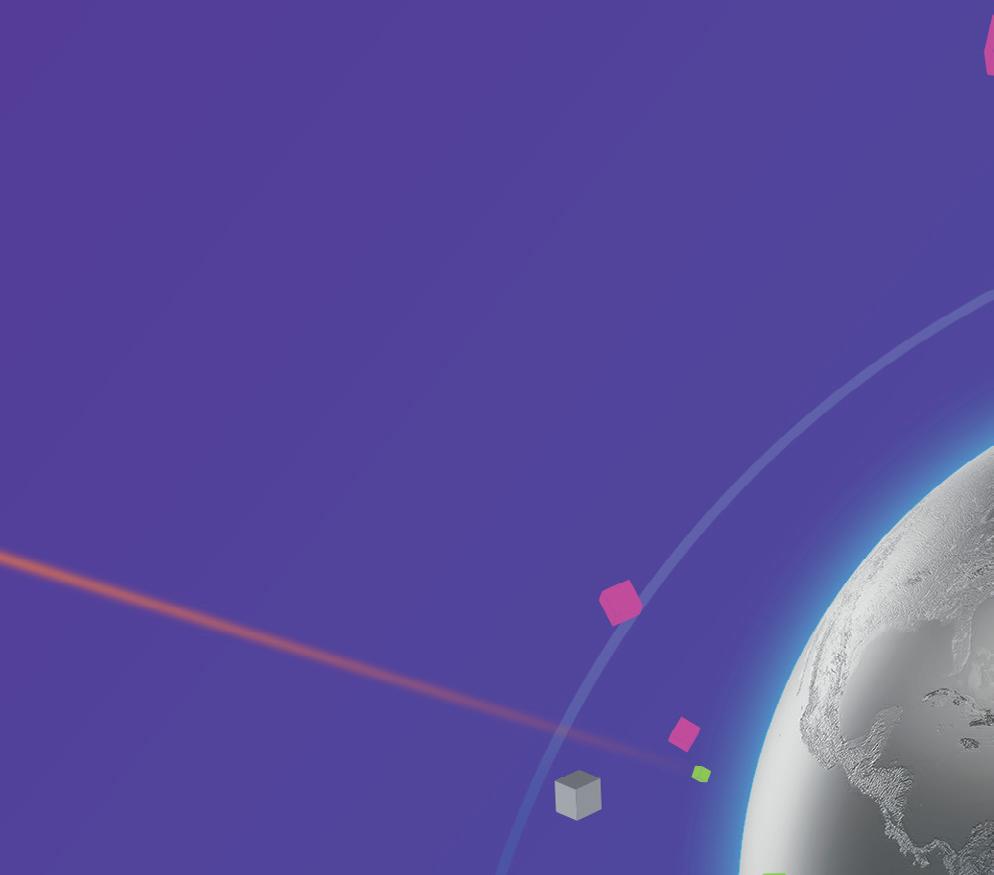
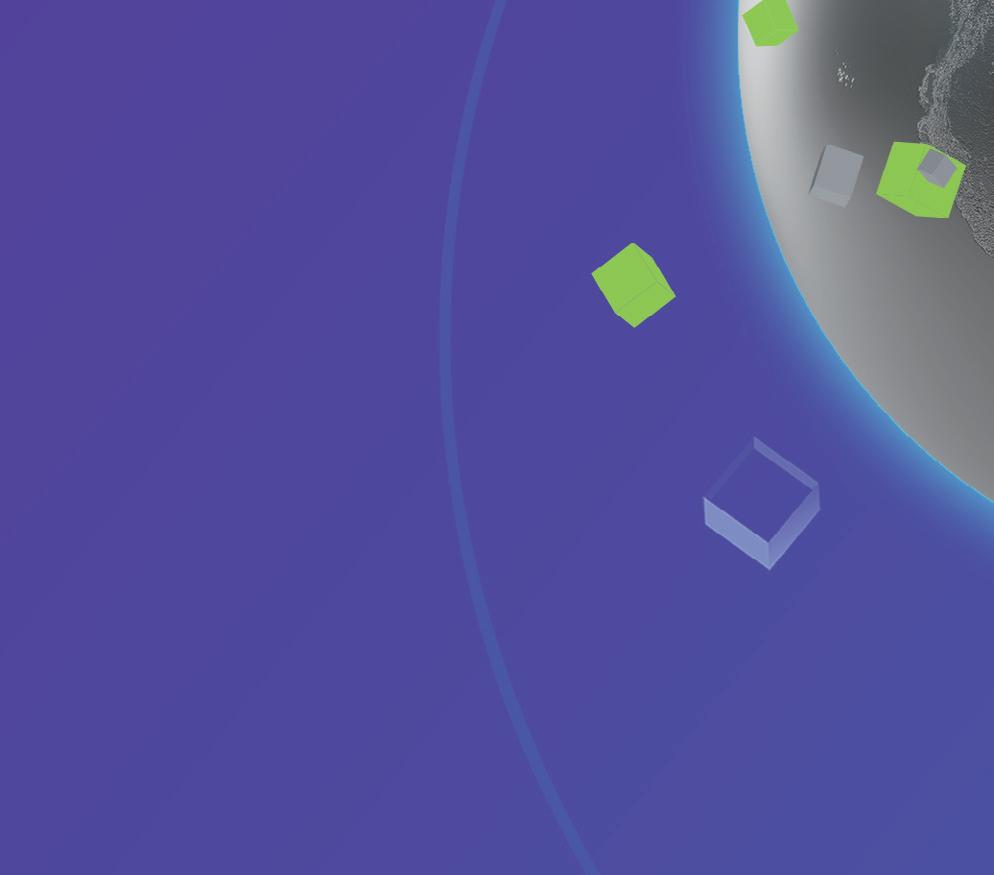
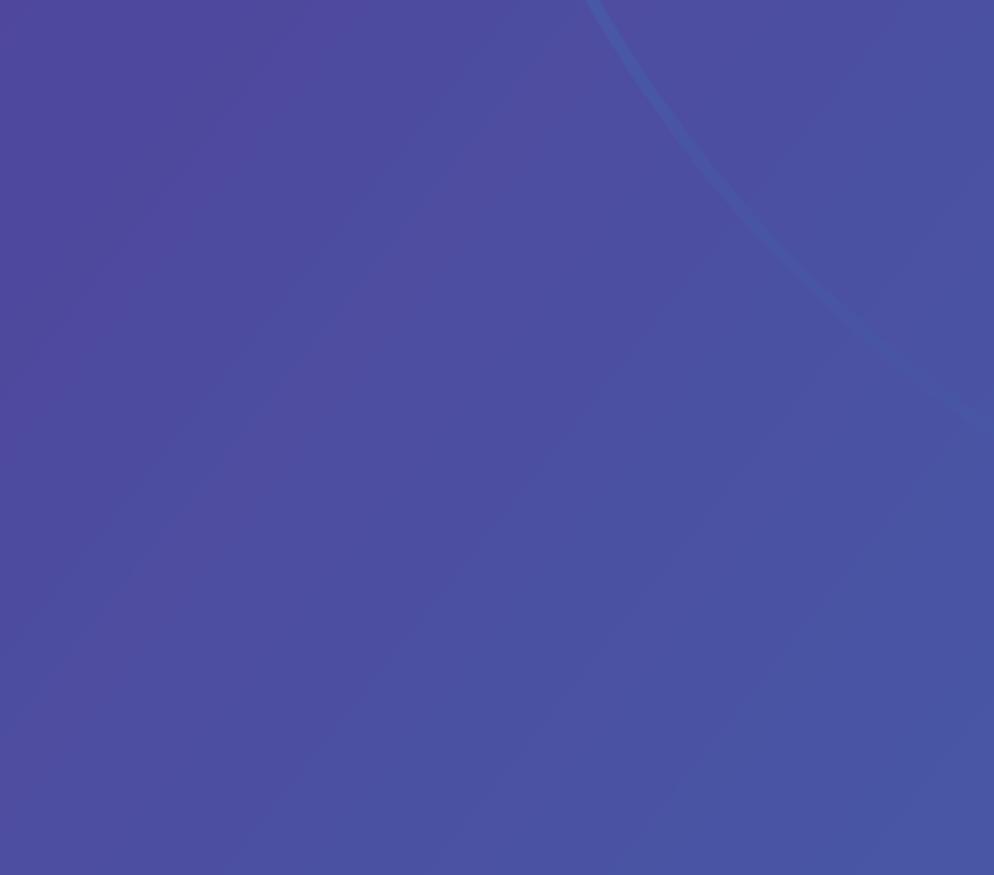
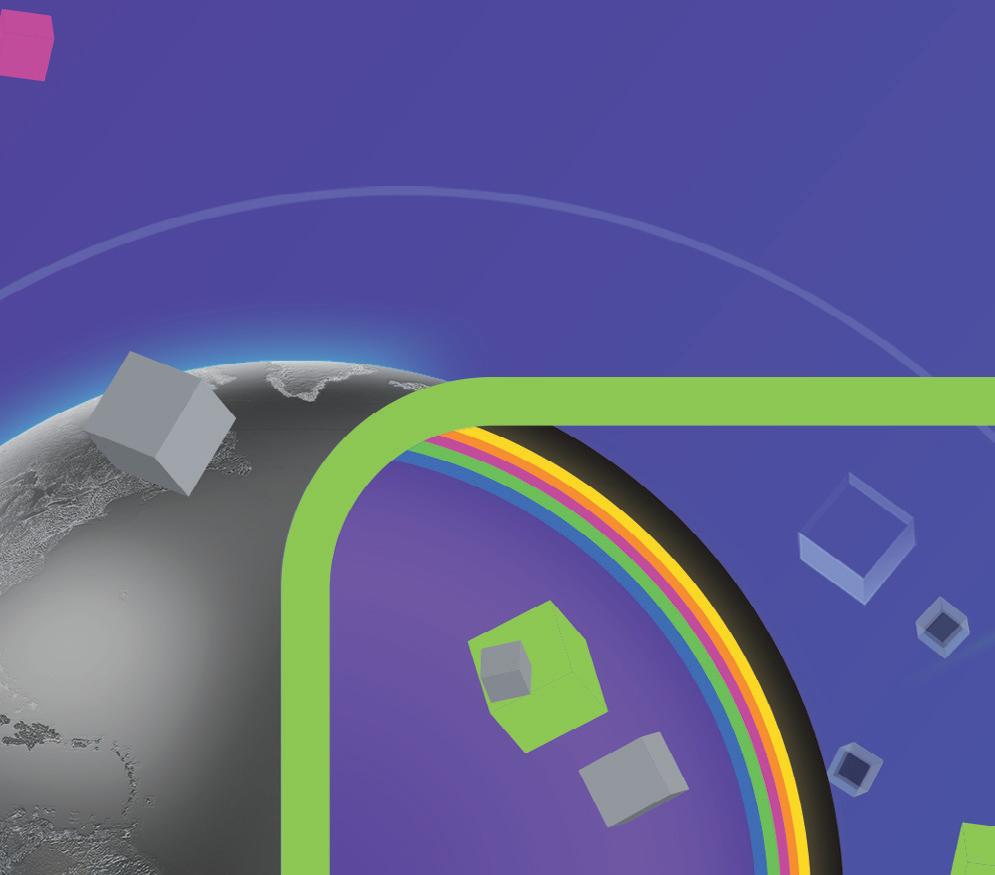
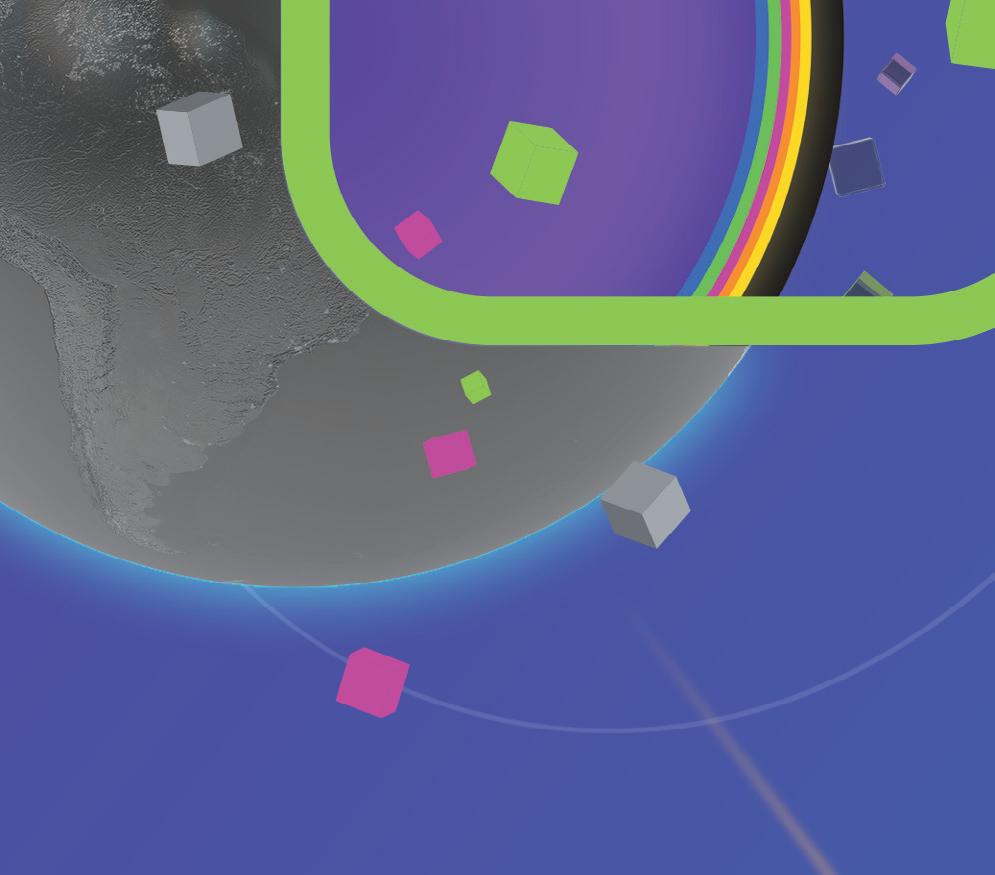
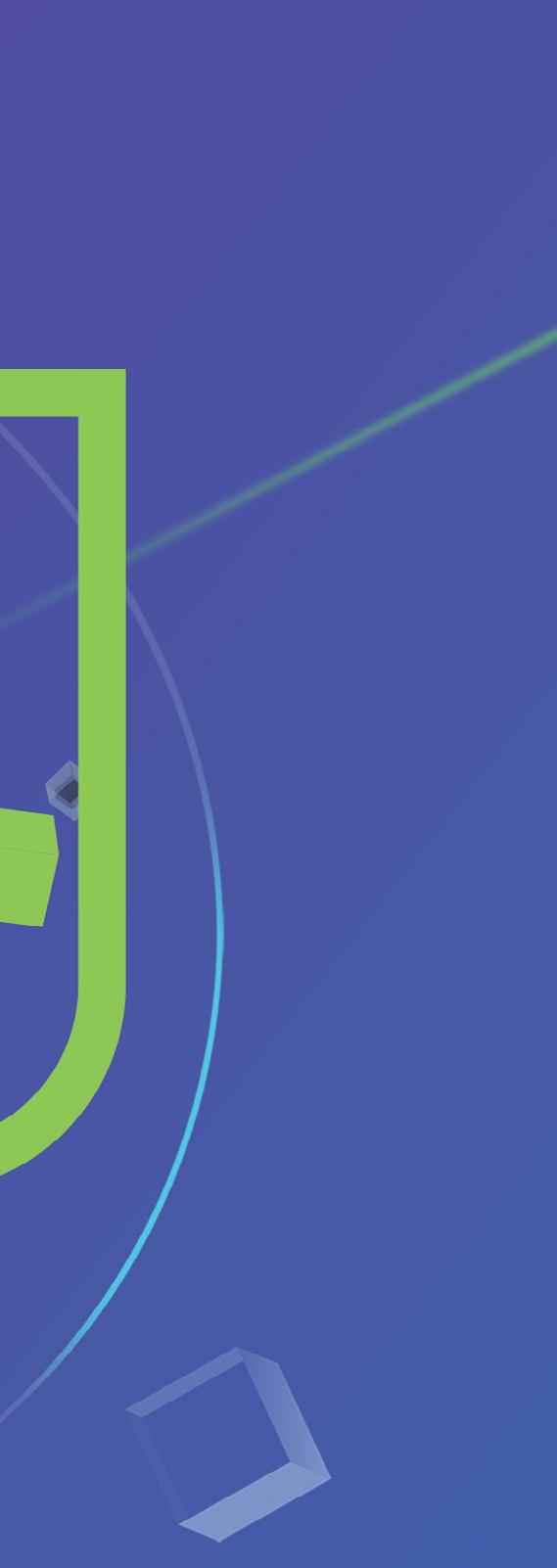
With 90+ years of experience pioneering advanced technology to meet some of the world’s most complex challenges, we’re ready to face the demands of tomorrow. We are Viridien.
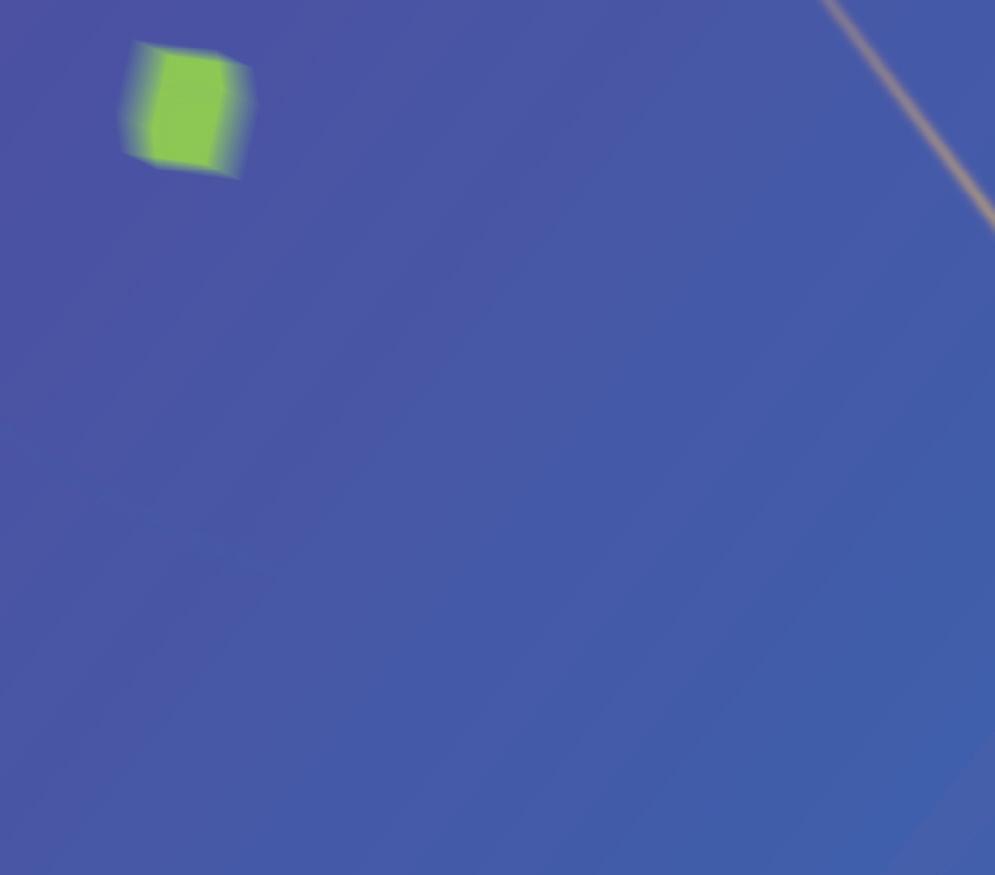
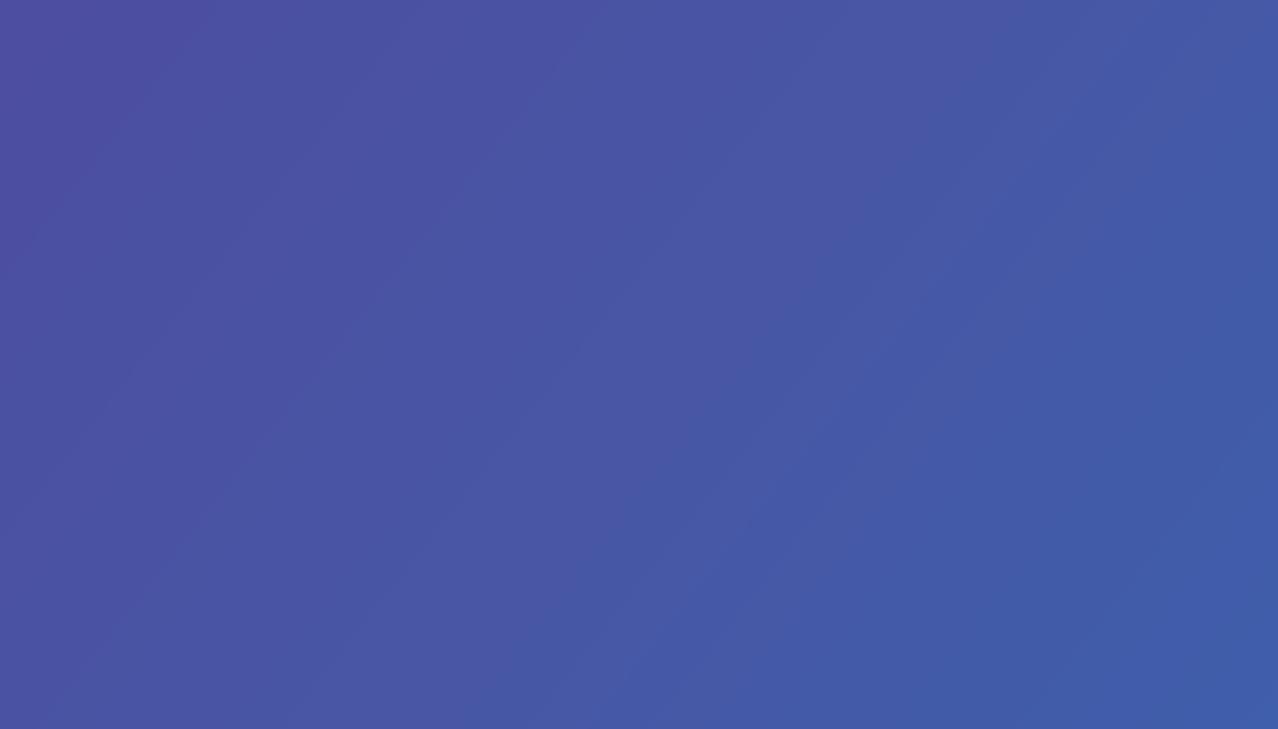
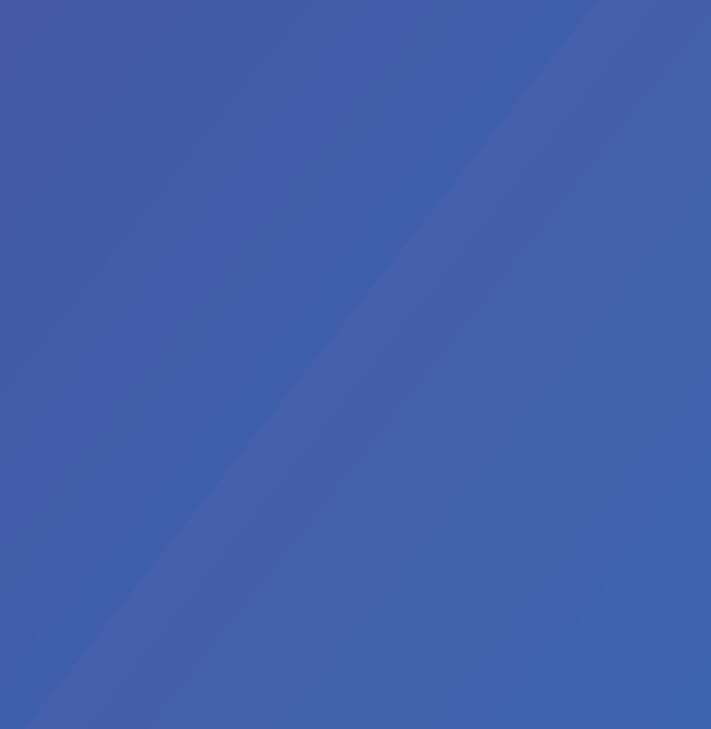
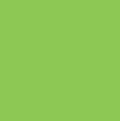





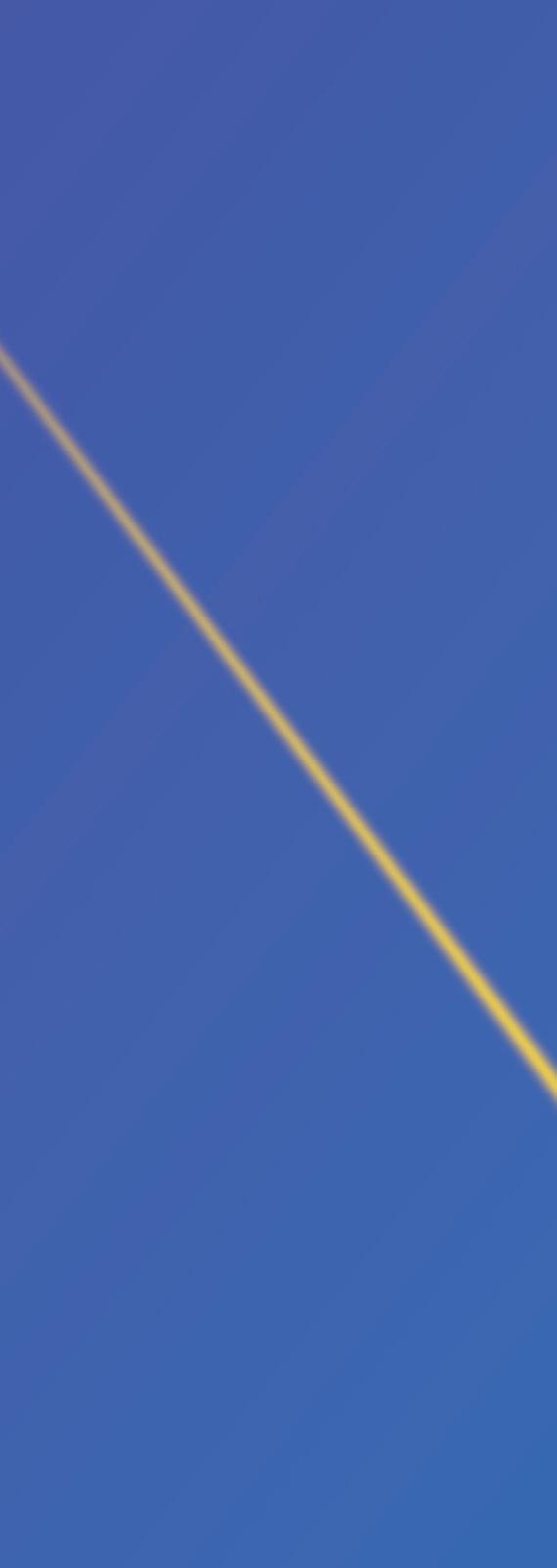
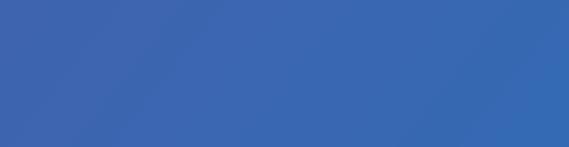
CHAIR EDITORIAL BOARD
Clément Kostov (cvkostov@icloud.com)
EDITOR
Damian Arnold (arnolddamian@googlemail.com)
MEMBERS, EDITORIAL BOARD
• Lodve Berre, Norwegian University of Science and Technology (lodve.berre@ntnu.no)
Philippe Caprioli, SLB (caprioli0@slb.com)
Satinder Chopra, SamiGeo (satinder.chopra@samigeo.com)
• Anthony Day, PGS (anthony.day@pgs.com)
• Peter Dromgoole, Retired Geophysicist (peterdromgoole@gmail.com)
• Kara English, University College Dublin (kara.english@ucd.ie)
• Stephen Hallinan, Viridien (Stephen.Hallinan@viridiengroup.com)
• Hamidreza Hamdi, University of Calgary (hhamdi@ucalgary.ca)
Gwenola Michaud, GM Consulting (gmichaud@gm-consult.it)
Fabio Marco Miotti, Baker Hughes (fabiomarco.miotti@bakerhughes.com)
• Martin Riviere, Retired Geophysicist (martinriviere@btinternet.com)
• Angelika-Maria Wulff, Consultant (gp.awulff@gmail.com)
EAGE EDITOR EMERITUS Andrew McBarnet (andrew@andrewmcbarnet.com)
PUBLICATIONS MANAGER Hang Pham (publications@eage.org)
MEDIA PRODUCTION
Saskia Nota (firstbreakproduction@eage.org) Ivana Geurts (firstbreakproduction@eage.org)
ADVERTISING INQUIRIES corporaterelations@eage.org
EAGE EUROPE OFFICE
Kosterijland 48
3981 AJ Bunnik
The Netherlands
• +31 88 995 5055
• eage@eage.org
• www.eage.org
EAGE MIDDLE EAST OFFICE
EAGE Middle East FZ-LLC Dubai Knowledge Village PO Box 501711 Dubai, United Arab Emirates
• +971 4 369 3897
• middle_east@eage.org
• www.eage.org
EAGE ASIA PACIFIC OFFICE
EAGE Asia Pacific Sdn. Bhd. UOA Centre Office Suite 19-15-3A No. 19, Jalan Pinang 50450 Kuala Lumpur
Malaysia +60 3 272 201 40
• asiapacific@eage.org
• www.eage.org
EAGE LATIN AMERICA OFFICE
EAGE Americas SAS Av. 19 #114-65 - Office 205 Bogotá, Colombia
• +57 310 8610709 +57 (601) 4232948
• americas@eage.org
• www.eage.org
EAGE MEMBERS’ CHANGE OF ADDRESS Update via your MyEAGE account, or contact the EAGE Membership Dept at membership@eage.org
FIRST BREAK ON THE WEB www.firstbreak.org
ISSN 0263-5046 (print) / ISSN 1365-2397 (online)
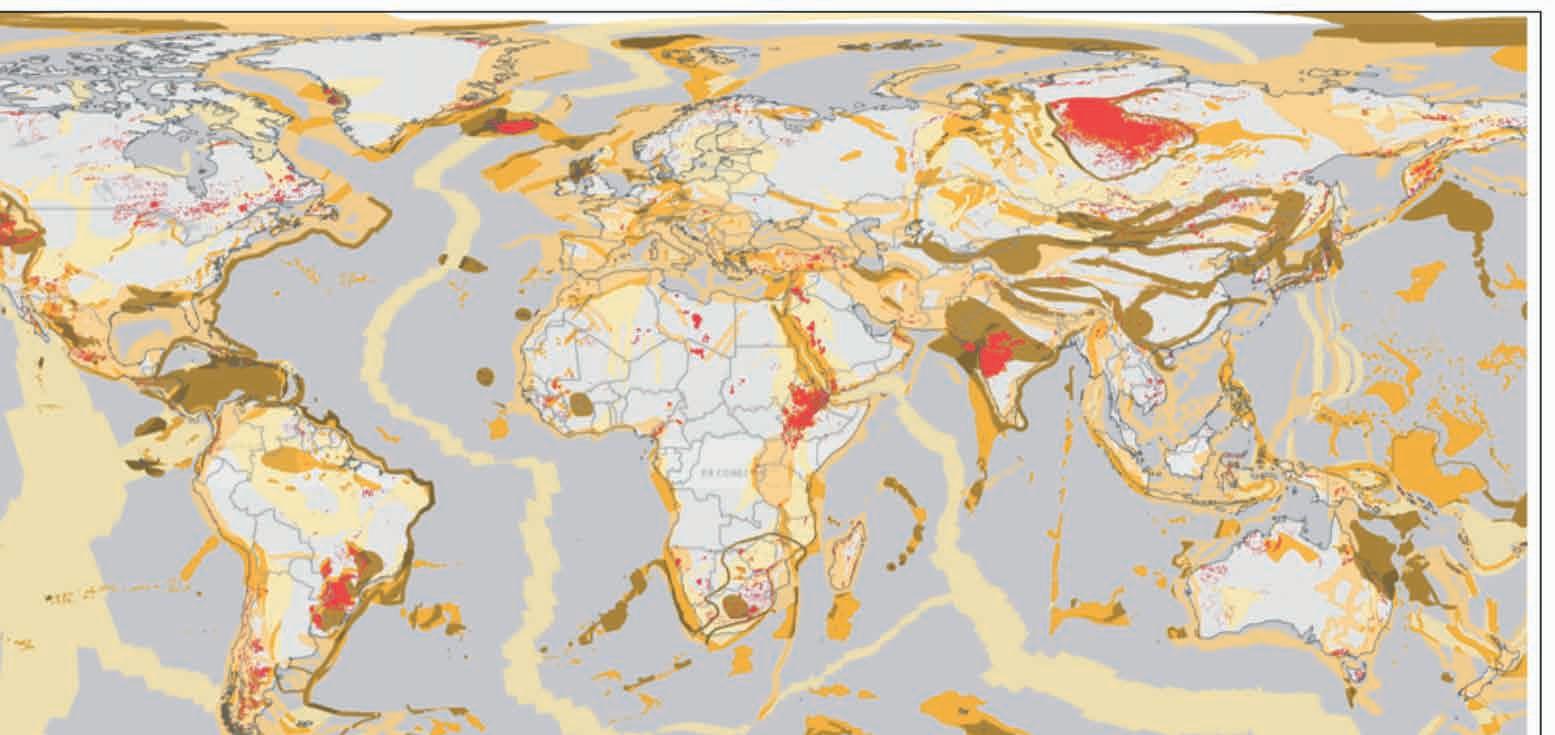
67 Mapping the potential for carbon storage in mafic and ultramafic rocks.
35 A consistent and integrated high-resolution stratigraphic framework for the Sokor Alternances in the R3 East Area, Agadem Basin, Niger Temistocles Rojas, Raul Bastante, Ed Robinson, Tim Wright and Christophe Ribeiro
43 Avo leads identified along the Natal Valley, offshore South Africa Sean Davids
Sp
ecial Topic: Energy Transition
51 The Storage Play Quality Index (SPQI): a multidisciplinary CO2 storage screening methodology
Gregor Duval, Robert Porjesz, Simon Otto, Carl Watkins, Mohammad Nassir, Alina Didenko, Pablo Cifuentes and Carolina Olivares
59 Seismic mini-streamers as a potential method for CO2 storage monitoring
Roya Dehghan-Niri, Åsmund Sjøen Pedersen, Mark Thompson, Anne-Kari Furre, Sandrine David, Harald Westerdahl and Tone Holm-Trudeng
67 Mapping the potential for carbon storage in mafic and ultramafic rocks
Paul Helps, Craig Lang and Eena Dadwal
75 Geothermal reservoir requirements for closed-loop well solutions to harvest geothermal energy
Kim Gunn Maver and Ola Michael Vestavik
81 Accelerating the energy transition using emerging geoscience skills
Philip Ringrose, Lasse Amundsen and Martin Landrø
89 A glimpse of the energy transition: Utah’s new energy corridor
Rasoul Sorkhabi, Palash Panja, John McLennan, Joseph Moore, Alan Walker, Robert Simmons and Milind Deo
95 Accelerated regional stratigraphic framework building for subsurface CO2 storage assessment
Sougata Halder, Keyla Gonzalez, Alex Fick, Vi Ly, Ben Lasscock, Zoltan Sylvester and Cameron Snow
102 Calendar
cover: Visualisation of the carbon cycle, from photosynthesis to fossil fuel combustion.
European Association of Geoscientists & Engineers Board 2024-2025
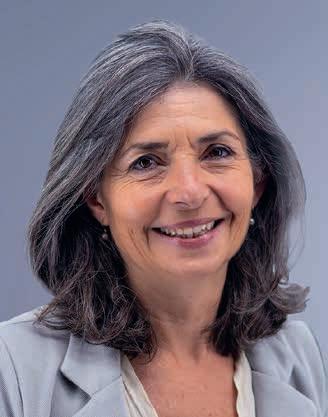
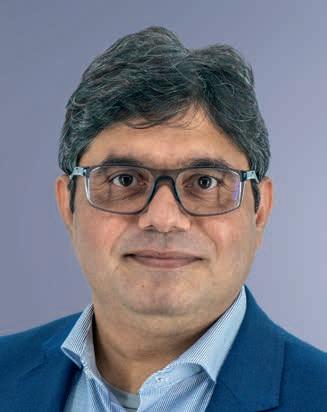
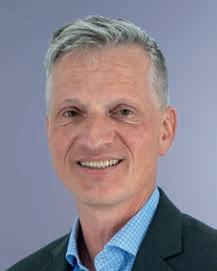
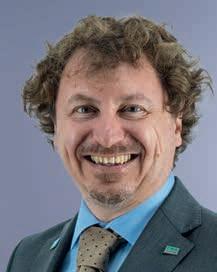
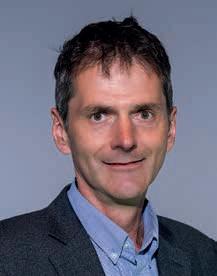
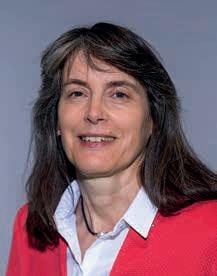
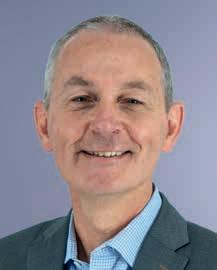
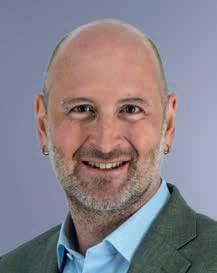
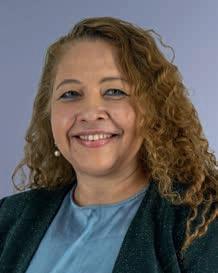
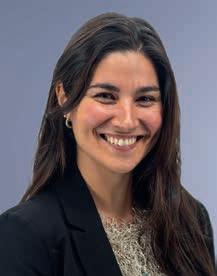
Near Surface Geoscience Circle
Andreas Aspmo Pfaffhuber Chair
Florina Tuluca Vice-Chair
Esther Bloem Immediate Past Chair
Micki Allen Contact Officer EEGS/North America
Hongzhu Cai Liaison China
Deyan Draganov Technical Programme Officer
Eduardo Rodrigues Liaison First Break
Hamdan Ali Hamdan Liaison Middle East
Vladimir Ignatev Liaison CIS / North America
Musa Manzi Liaison Africa
Myrto Papadopoulou Young Professional Liaison
Catherine Truffert Industry Liaison
Mark Vardy Editor-in-Chief Near Surface Geophysics
Oil & Gas Geoscience Circle
Yohaney Gomez Galarza Chair
Johannes Wendebourg Vice-Chair
Lucy Slater Immediate Past Chair
Wiebke Athmer Member
Alireza Malehmir Editor-in-Chief Geophysical Prospecting
Adeline Parent Member
Matteo Ravasi YP Liaison
Jonathan Redfern Editor-in-Chief Petroleum Geoscience
Robert Tugume Member
Anke Wendt Member
Martin Widmaier Technical Programme Officer
Sustainable Energy Circle
Carla Martín-Clavé Chair
Giovanni Sosio Vice-Chair
SUBSCRIPTIONS
First Break is published monthly. It is free to EAGE members. The membership fee of EAGE is € 80.00 a year including First Break, EarthDoc (EAGE’s geoscience database), Learning Geoscience (EAGE’s Education website) and online access to a scientific journal.
Companies can subscribe to First Break via an institutional subscription. Every subscription includes a monthly hard copy and online access to the full First Break archive for the requested number of online users.
Orders for current subscriptions and back issues should be sent to First Break B.V., Journal Subscriptions, Kosterijland 48, 3981 AJ Bunnik, The Netherlands. Tel: +31 (0)88 9955055, E-mail: subscriptions@eage.org, www.firstbreak.org.
First Break is published by First Break B.V., The Netherlands. However, responsibility for the opinions given and the statements made rests with the authors.
COPYRIGHT & PHOTOCOPYING © 2024 EAGE
All rights reserved. First Break or any part thereof may not be reproduced, stored in a retrieval system, or transcribed in any form or by any means, electronically or mechanically, including photocopying and recording, without the prior written permission of the publisher.
PAPER
The publisher’s policy is to use acid-free permanent paper (TCF), to the draft standard ISO/DIS/9706, made from sustainable forests using chlorine-free pulp (Nordic-Swan standard).
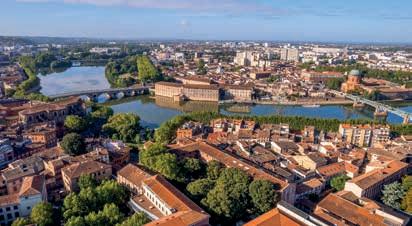
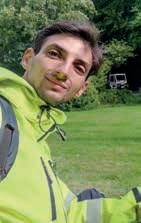
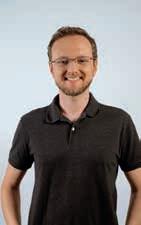
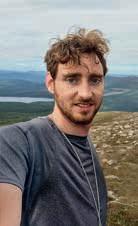
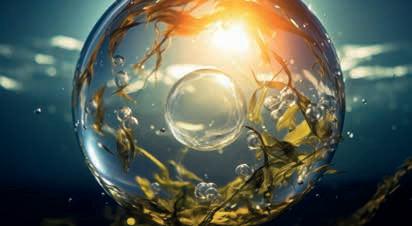
Our biggest energy transition event ever is almost here
With just a month to go, we are excited to be welcoming our global geoscience and engineering community, including professionals from the carbon capture storage, geothermal, hydrogen, energy storage, and offshore wind sectors, to the 5th Global Energy Transition Conference and Exhibition at the Convention Centre WTC in Rotterdam, on 4-7 November 2024. Here’s a glimpse of what you can expect.
Opening session
EAGE GET 2024 will kick off with the official opening session, where conference chair Yolande Verbeek, COO of EBN, along with EAGE president Valentina Socco, will deliver welcome addresses. The session will also include a presentation by the Minus CO2 Challenge winning team, the Marie Tharp Award ceremony recognising emerging talents committed to advancing energy system transformation, and key discussions with leading industry figures.
Technical Programme
The technical programme will feature nine distinct tracks, comprising over 340 presentations and more than 300 speakers.
These sessions are specifically structured to address critical topics across several domains. The Carbon Capture and Storage (CCS) programme will cover the entire value chain, including geological containment, advanced monitoring technologies, and reservoir modelling. Discussions on Geothermal Energy will put forward innovative potential assessments, well construction, and resource optimisation, with a particular focus on integrating geophysical techniques to enhance efficiency within the Dutch geothermal sector. The Hydrogen & Energy Storage track will cover a wide range of subjects, from the societal impacts of the hydrogen economy to the technical challenges associated with subsurface energy storage and
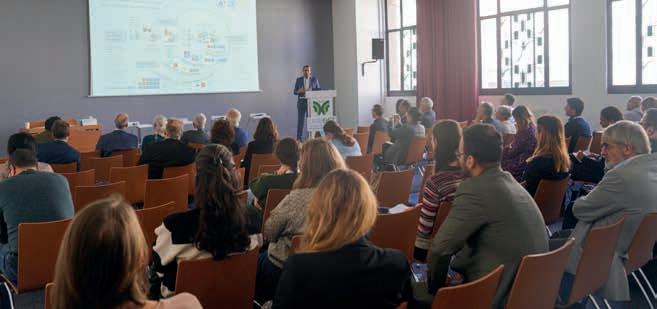
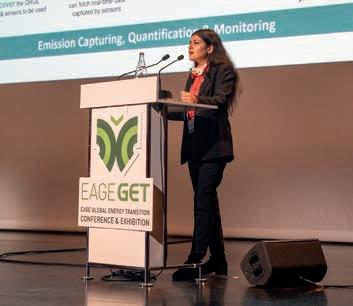
underground hydrogen storage, thereby positioning hydrogen as a vital component of future energy solutions. Offshore Wind Energy will feature sessions addressing risk management, geohazards, and the integration of geophysical and geotechnical data to optimise wind energy projects in increasingly complex environments. The dedicated sessions throughout the conference will address the technical, economic, environmental, and societal challenges associated with emerging energy technologies and practices. Key themes include the development and optimisation of energy resources, the integration of innovative technologies, and strategies for ensuring sustainability and safety across various sectors.
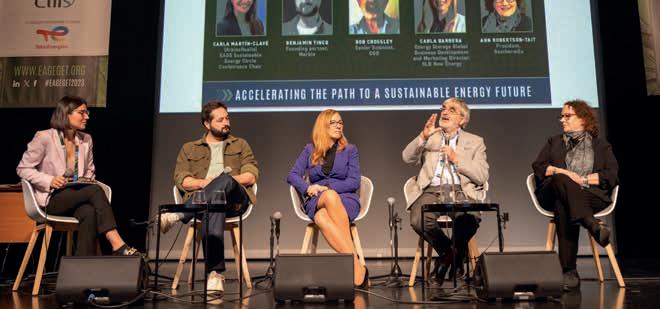
Strategic Programme
In addition to the technical sessions, the Strategic Programme will bring together top industry leaders to explore critical issues driving the global energy transition. Key topics will include building resilient value chains for the energy transition, bridging the gap between societal engagement and technological advancement, and the role of digitalisation in revolutionising technology, markets, and talent. You can look forward to joining our panellists from organisations such as the International Geothermal Association, Shell, BP, Ministry Economic Affairs & Climate, Imperial College, TGS, Viridien, Microsoft, Aker Solutions, and Utrecht University, along with many other leading companies and institutions.
Short courses, workshops, and field trips
GET 2024 participants can also look forward to an array of workshops, short courses, and field trips scheduled on the Monday before and Friday after the main conference, designed to offer a comprehensive and immersive learning experience. These side activities will explore both the technical and societal dimensions of subsurface usage and sustainable energy practices.
For example, workshops will tackle complexities like stakeholder engagement in geothermal energy, hydrogen storage, and CCS, offering a platform for discussions on innovative methods of stakeholder interaction beyond traditional expert circles. Another workshop will focus on geological risk assessment for geothermal and CCS, providing hands-on experience with core materials and specialised lab equipment at the PanTerra laboratory.
Complementing these workshops, a series of one-day short courses led by renowned instructors will cover topics such as offshore wind development, reservoir engineering for geothermal energy production, and CO2 storage project design and optimisation. For those with an interest in
communication and public engagement, a course on geoscience communication will offer expert guidance on effectively conveying complex technical issues to non-technical audiences, a skill that is increasingly vital in the energy sector.
Additionally, field trips to the HyStock hydrogen storage facility and the Porthos CO2 storage project beneath the North Sea will provide participants with an in-depth look at the practical implementation of the technologies and strategies discussed during the conference.
Exhibition
The exhibition is an essential stop for anyone looking to enhance their professional knowledge and connect with the innovators driving today’s energy transition. Here, you’ll have the opportunity to engage directly with the minds behind innovative and disruptive technologies or services across various applications in geoscience, decarbonisation, renewables, and circularity. Our diverse range of exhibitors includes major operators, universities, and industry associations. Additionally, a special zone on the exhibition floor will be dedicated exclusively to startups. EAGE is providing complimentary exhibition floor space and team registrations for selected startups to showcase innovative products and services to a global audience. It’s a chance to network, collaborate, and grow within the vibrant EAGE community. Whether you’re working on cutting-edge technology or have a new approach to sustainable energy, this platform will allow you to present your ideas to potential investors, partners, and customers.
Energy Transition Theatre
The Energy Transition Theatre in the exhibition area will host three days of dynamic discussions and interactive sessions dedicated to professionals in the energy transition sector and beyond, who play a vital role in achieving net-zero
With so much to offer, GET 2024 is shaping up to be an unmissable event for anyone involved in the energy transition. Don’t miss out on free visitor passes and early registration discount. Register by 1 October to take advantage of reduced fees. And if you want to get the full experience, opt for an all-access pass to join workshops, field trips, and short courses. For more details and to register, visit eageget.org.
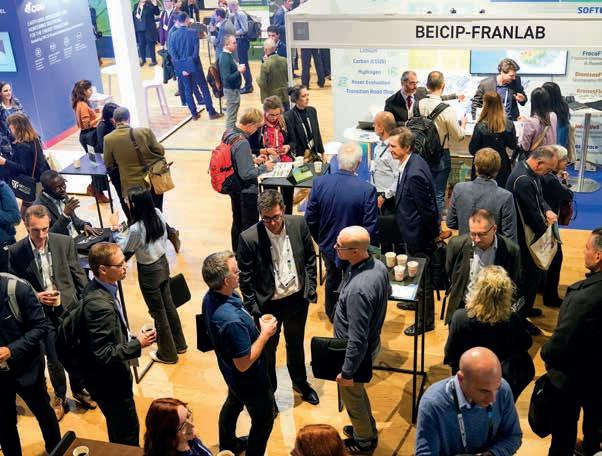
emissions. This exclusive area will focus on three key topics on the three days: Finance & Insurance, Critical Minerals & Raw Materials, and the Net Zero Journey.
Energy transition student days
For students, the conference offers a unique opportunity to engage with the energy transition through the Student Days Programme which provides free entry for students and includes four days of learning lectures, offering a comparative analysis with traditional oil field projects in terms of energy content, emissions, profitability, and stakeholder perspectives. Participants will engage in hands-on experiences, analysing underground maps, well profiles, and other essential data. Working in teams, they will be tasked with proposing development plans for underground resources, tackling both technical and non-technical challenges. This immersive experience should not only enhance their knowledge but also equip them with practical skills to assess the feasibility of these projects from multiple perspectives. Students will gain particular insights into energy transition in the Netherlands, in line with the Paris Climate goals and national targets.
Social events
Networking opportunities are a key aspect of any conference, and the planned social programme. It begins with a Speaker’s Reception at Rotterdam City Hall on 4 November, providing an exclusive setting for speakers and committee members to mingle in a historic venue. The Icebreaker Reception on 5 November in the Exhibition Hall will offer all participants a relaxed environment to meet and reconnect over light refreshments. The highlight of the social programme is the Conference Evening the historic SS Rotterdam, a former ocean liner now serving as a unique event venue.
DUG MULTI-PARAMETER
FWI IMAGING UNVEILS
A LAND OF OPPORTUNITY
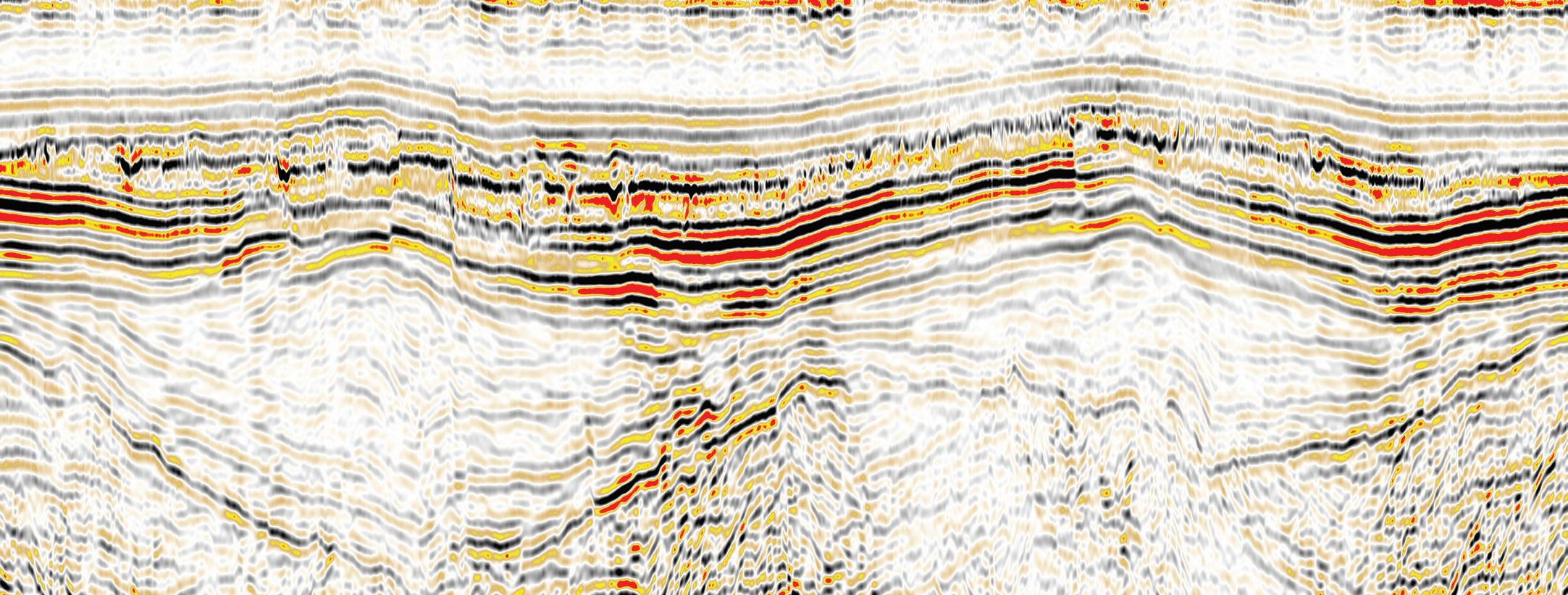
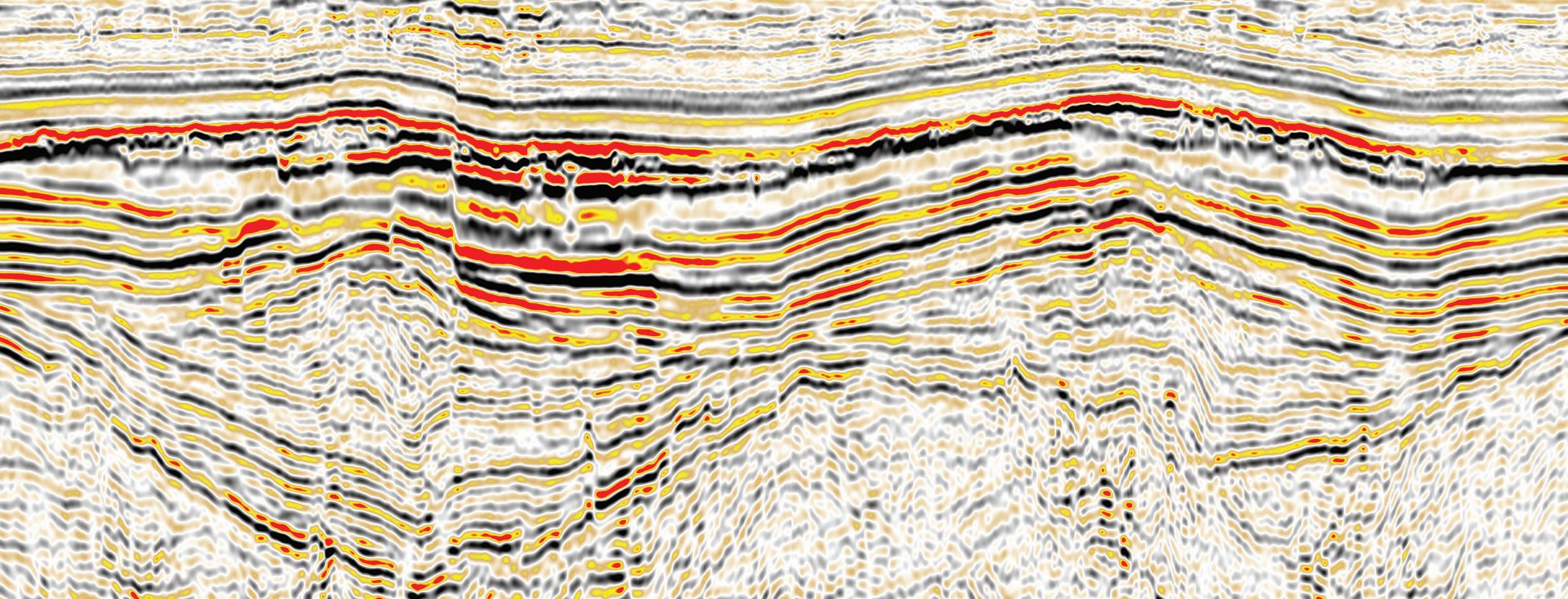
Vast opportunities await with DUG Multi-parameter FWI Imaging . Thanks to least-squares imaging of the full wavefield — including interbed multiples — DUG’s revolutionary technology achieves significantly better illumination and event continuity. It also resolves incredible near-surface detail. Superior results, in a fraction of the time — it’s an opportunity you don’t want to miss.
info@dug.com | dug.com/fwi
GET course to focus on communication skills for the transition
An exciting feature at EAGE GET 2024 conference will be the specialised course on Geoscience communication and public engagement, not least because it will be led by the high-profile geoscientist, Prof Iain Stewart, a world authority on the topic. He is currently the Jordan–UK El Hassan bin Talal research chair in sustainability, a new collaboration between the Royal Scientific Society of Jordan (RSS) and the British Academy. He is also UNESCO chair in geoscience and society, professor of geoscience communication, Sustainable Earth Institute at University of Plymouth, and an award-winning TV documentary maker on earth sciences.
The course is designed to arm participants with both the theoretical knowledge
and practical tools necessary to engage a broad audience learning how to collaborate effectively with media professionals, and discover the art of ‘storifying’ scientific data to create compelling narratives. Tailored for industry practitioners and academic researchers who are eager to enhance their communication skills, the course focuses on real-world applications. Through hands-on exercises, such as the Risk Communication Bowtie and the ABT Method (And, But, Therefore), participants will gain practical experience in crafting messages that not only inform but also engage and inspire action. This takes into account that geoscientists often work on projects that have far-reaching implications for society, from energy pro-
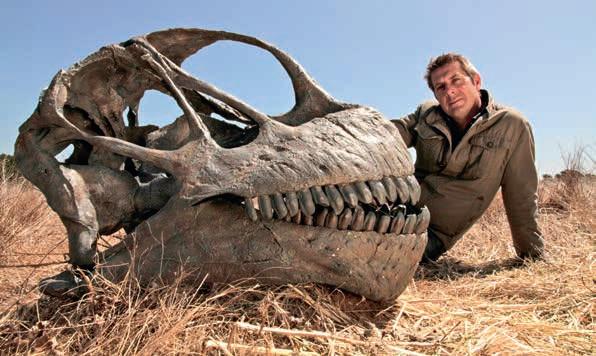
duction to environmental sustainability, which may be sources of public concern or controversy. There are no prerequisites to take part in the course, making it accessible to anyone with an interest in geoscience communication.
Prof Stewart on the communication challenge
How does this course specifically help geoscientists overcome common communication challenges?
The communication challenge that geoscience faces in connecting to the wider public is twofold. The first is simply the issue of familiarity. Most people don’t think much about geology – or the planet – much in their everyday activities and don’t encounter geoscientific issues. Of course, issues around energy are part of everyday discussions, but the association with geology, the subsurface, etc is not a topic of conversation. So, how do we make people care about our geoscientific world? For that reason, finding ways to link geoscience issues with the ‘matters of concern’ that people have is an important consideration which the course will deal with.
The second issue is that the general public has an increasingly negative view of the traditional extractive resource sector, both mining and hydrocarbons, which many see as exploitative and a major part of the climatic and ecological crisis that society is facing. By
contrast, geoscientists in those sectors see themselves as a big part of the solution to those societal issues, and so finding new narratives that highlight the important role of mining and materials is another issue that the course will address.
What role does storytelling play in shaping public perception of geoscience topics?
Storytelling is probably the single most important communication skill that geoscientists have in connecting ourselves to the outside world. The good news is that we have it. Geologists are natural storytellers, and the work we undertake in unravelling the history of the planets through the rock record is intrinsically about developing a compelling a historical narrative about humanity’s deep past. So the challenge is to remind geoscientists of this and to show how we already use storylines in communicating our technical science. We just need to develop better and more engaging storylines to draw in the non-geological audiences that we want to reach.
How important is it for geoscientists to engage with local communities and stakeholders?
Probably the most important people to reach are those local communities and stakeholders that are connected with specific energy projects and developments that we are involved with. The lazy approach is to presume a Nimby attitude amongst local people and assume that simply giving them the facts will secure the ‘social licence to operate’. In fact, social acceptance is built on multiple layers of transparent interaction and trusted relations in which the communication involves as much listening as talking. The tricky message for geoscientific specialists is that mapping the ‘community play’ is as important as mapping the ‘subsurface play’ if we want to ensure the long-term sustainability of our energy project. As a sector we need to spend a lot more time and resource on developing the communication skills to do that.
I’ll catch you on the course at GET 2024!
Toulouse is perfect setting for the EAGE Annual in 2025
There’s lots to look forward to when the EAGE Annual Conference & Exhibition convenes next year in the vibrant city of Toulouse. Renowned as the world capital of aeronautics and the European hub for the space industry, Toulouse provides an inspiring setting for a gathering focused on innovation and sustainability in geoscience and engineering. From 2-5 June 2025, the city will serve as the focal point for our global community’s efforts to explore new frontiers and drive a more sustainable future.
Our theme, ‘Navigating Change: Geosciences Shaping a Sustainable Transition’, finds a fitting stage in Toulouse. With institutions like the University of Toulouse at the forefront, the city is a leader in pioneering studies in earth sciences and environmental technologies. It is also closely connected to energy innovation in nearby regions, such as Pau. Beyond the professional landscape, Toulouse invites discovery. With its
unique blend of historical architecture and modern design, it is often referred to as ‘La Ville Rose’ or ‘The Pink City’ reflecting its distinctive pink terracotta buildings that glow warmly in the sunlight.
The Cité de l’Espace and the Aeroscopia Museum offer interactive experiences that bridge science and history, giving attendees a deeper understanding of the technological advancements shaping both the past and future of space and aviation. These attractions resonate strongly with our community, reflecting the spirit of exploration and discovery that is central to geoscience and engineering.
Walking through the city’s picturesque streets, you’ll discover a vibrant array of markets, where you can sample local delicacies like cassoulet, and Toulouse sausage. The city’s culinary scene is diverse, ranging from traditional bistros to contemporary restaurants that showcase innovative takes on French cuisine. For those interested in experiencing the
lively nightlife, Toulouse offers a host of bars, music venues, and cafes where networking can continue in a relaxed and informal atmosphere.
Toulouse’s location in southwestern France also means easy access to some of the country’s most beautiful natural landscapes. The nearby Pyrenees Mountains offer stunning vistas and diverse geological formations.
Join us for the Toulouse experience while attending the EAGE Annual Conference & Exhibition from 2-5 June 2025.
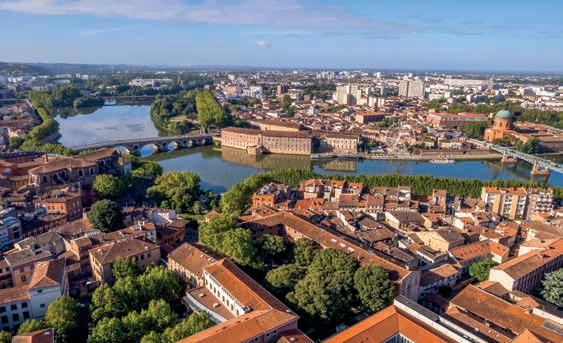
Renew your commitment to innovation in 2025
Just so you don’t miss out on all the services, events and benefits that EAGE has to offer, we encourage everyone to renew their membership early and ensure that 2025 will be a rewarding experience in the geoscience and engineering community.
And don’t forget our loyal members Recognition Programme, which acknowledges your continuous commitment by rewarding you with additional perks the longer you are a member. These include more discounted rates for events and courses, expanded access to the EarthDoc archive, and eligibility for various support programmes.
One of the most remarkable aspects of EAGE is its diverse network of communities, including technical and non-technical groups, local and student chapters. Next to these, an active global calendar of conferences, workshops and training courses,
both multi-disciplinary and specialized, empower dialogue and new ideas. This rich tapestry of opportunities allows members to connect on various levels and across many different interests.
So whether you are a seasoned professional or just beginning your journey in geoscience and engineering, the EAGE network offers you a space where you can connect, learn, and grow. As one of our members, Pedram Masoudi (senior geostatistician and geophysicist at Geovariances, and secretary for Local Chapter Paris) puts it: ‘Insightful, professional and friendly discussions. It is the magic of healthy professional associations.’ This magic is what makes EAGE a unique and valuable community for all its members.
We also take pride in supporting emerging talent and younger members.
As Tiziana Vanorio, professor at Stanford University and former chair of the EAGE Awards Committee, says, ‘Our commitment to education and research is at the heart of our efforts to advance sustainable energy solutions and responsible environmental practices. This work is crucial for nurturing the next generation of geoscientists and engineers, providing them with the tools and confidence they need to succeed’.
Hopefully early renewal of your membership will not just be a routine task but a commitment to your own continued professional development. By renewing now, you can secure uninterrupted access to the wealth of benefits that EAGE membership offers throughout 2025. We are excited about the opportunities that lie before us and look forward to the new achievements we can accomplish together.
Popular geothermal energy course now available online
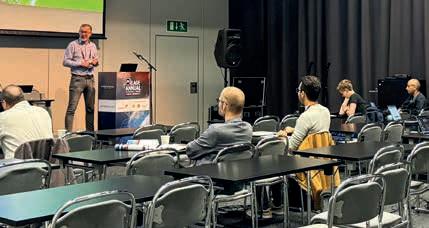
With the aim of further disseminating knowledge among our members, especially regarding energy transition demands and challenges, our in-person course Reservoir Engineering of Geothermal Energy Production has been made available in an extensive online format. Starting on 12 November 2024, this new version combines self-paced learning material with interactive sessions with the instructor, providing a deep-dive learning experience that adapts to your time availability.
Guided by Dr Denis Voskov, associate professor at TU Delft with 20 years of experience in reservoir modelling, participants will deepen their knowledge on geothermal energy production and engineering by emphasising direct-use geothermal resources. He says, ‘Global energy demand is rising, accompanied by the urgent need to
address climate change. Geothermal energy is a renewable energy source that has the potential to produce electricity and heat. However, geothermal resources for electricity generation are limited and unevenly distributed across the globe. Direct-use geothermal systems are capable of covering this demand with the resources wildly available worldwide. That explains why direct heat geothermal systems are rapidly developing and can significantly reduce the application of fossil fuels for heating purposes’.
Topics being covered in the course include the physical phenomena involved in geothermal energy production, the types of geothermal resources available, and the key principles of reservoir simulation and their application to geothermal energy modelling. The learning experience is complemented with several build-forpurpose simulation exercises in Jupyter Notebooks using the DARTS (open-source Delft Advanced Research Terra Simulator) framework. The first hands-on exercise explains the development of a basic geothermal model with all important gradients, and evaluates sensitivities to numerical and physical parameters; the second, explores
the effect of overburden and realistic heterogeneity, as well as their impact on energy production, and the third, introduces a fractured reservoir and explains how different parameters of fractured systems affect geothermal production.
Upon completion of the course, you’ll be able to understand basic physical concepts of geothermal energy production; operate with the main concepts of reservoir simulation for geothermal applications; create a basic geothermal model for realistic fluvial or fractured reservoirs, and understand the importance of different numerical and physical properties to the prediction of geothermal energy production.
For geophysics or engineering (petroleum, civil, or environmental) students or professionals, with experience of basic Python programming and interested in pursuing a career in energy transition-related fields, this is an opportunity not to be missed. EAGE members benefit from discounted registration fees, so if you are not a member yet, join or renew your membership at eage.org.
LANDRØ
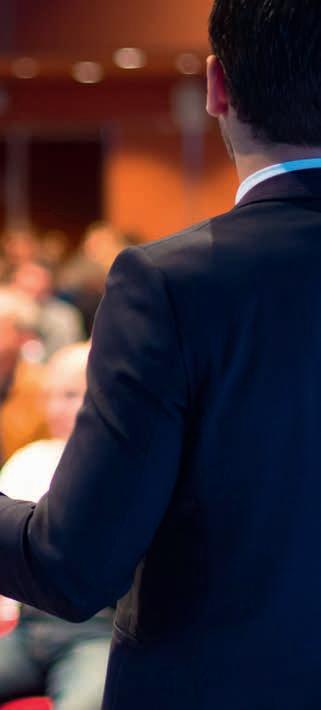
CCS Technical Community gets down to work in Oslo
Community chairs, Audrey Ougier-Simonin (BGS) and Matthias Imhof (ExxonMobil), present some highlights from the dedicated session held at the 2024 EAGE Annual on Offshore CCS.
Our dedicated session entitled ‘Offshore CCS: A North Sea Perspective’ was a celebration for the kickoff of the new EAGE Technical Community on CCS. Operators, service providers, researchers, and regulators joined in to share an aspect of their work, giving the audience a broad vision of the progress in the region deemed to be a major CCS hub.
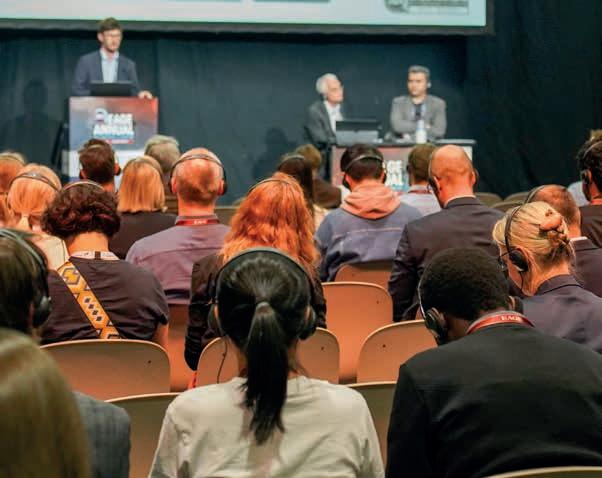
Looking at the Southern North Sea, MacBeth et al modelled fluid-related seismic timelapse signals for storage scenarios in saline aquifers and depleted gas reservoirs, which are the two main CO2 storage concepts in the North Sea. For saline aquifers, they concluded that the seismic timelapse response should be large and observable even with low-cost acquisition, though intra-reservoir complexity may require more attention. For depleted gas reservoirs, the pressure response will be readily visible but swamping the saturation change. The base reservoir response may be ‘just’ detectable with dedicated towed streamer data.
Sorbier presented an overview of the Aramis project in the Dutch offshore sector. As a successor to the Porthos project, the Aramis project can use learnings from the Porthos application process. The concept is to reuse depleted gas fields for carbon storage and leverage existing infrastructure. In the presentation, Sorbier shared
aspects of the field screening process, the value chain, the impacts of the installation design and store development plan, and the MMV design. Two key learnings were the need for adoption of a learner mindset and risk perception of different stakeholders.
Using vintage data from the Sleipner storage site, Martinez et al. presented learnings from timelapse full-waveform inversions (FWI). Prior studies of timelapse FWI at Sleipner relied on the reflected wavefield only and struggled with reconstruction of low-frequency velocity trends. Here instead they combined reflected and transmitted wavefields to obtain velocities and images that help understand the multilayer CO2 system with feeders and conduits routing CO2 between layers.
Meneguolo et al reported on the influence of clay mineralogy on the reservoir pressurization capacity at Northern Lights. They presented a multi-disciplinary, multi-scale approach that combined seismic interpretation and well-based rock measurements including wireline log interpretation, extended leak-off tests and sample-based microfracture testing, and analysis of rock composition. They found that thickness and compositional variations of the top seal occur outside the critical project area.
Inspired by Greensand, Al Khatib et al proposed the concept of predictive maintenance for CCS monitoring where focused seismic data is integrated with dynamic model predictions. Dynamic models draw on geological data, well information, seismic interpretations, and legacy production parameters. These models serve as essential tools in planning and monitoring underground CO2 injection projects. Permit applications, risk assessments, and the economics of CCS projects are developed using these models. Focused seismic surveillance tests the model predictions in time and space, and either triggering no action, a model tweak, or a more detailed follow-up survey.
Archibald et al presented an overview of the Smeaheia storage site and how
the operator (Equinor) is maturing the subsurface concept toward commercial operation. Despite the abundance of data in the adjacent Troll field, the large licence area is data poor. As is typical in most saline aquifer storage projects, there is a lack of well coverage, core data, fluid samples and dynamic data. Appraisal and de-risking of key subsurface uncertainties (injectivity, permeability, time-depth conversion, formation pressure, and trap seal) is limited when compared to oil and gas projects where observed fluid contacts prove such elements as hydrocarbon migration, trap and seal integrity. The operator will drill two exploration wells to reduce the uncertainty range in current storage volume estimates and to test upside potential in reservoir intervals not currently included in the storage concept.
Also focusing on Smeaheia, Butar and Carballares showed a workflow for enhanced fault interpretation and structural framework building to reduce uncertainty and cycle time. Their workflow is based on machine learning (ML) and requires labelling a few seismic inlines and crosslines as input. The result is a fault prediction cube that improves the understanding of the extensional fault development in the storage complex. They also observed the resulting fault sticks to be more consistent than manual picks which reduces uncertainty in the structural model.
The enthusiastic discussions clearly highlighted the strong desire and need for opportunities to share experiences and learn from one another. We hope that our new community on CCS will serve as an effective platform to fulfill this need. Eager to know more? Check out the session’s proceedings in EarthDoc.
Connect with the EAGE Technical Community on CCS
Natural hydrogen: hope or hype was the question at the Annual
‘Natural hydrogen potential assessment and modelling’ was topic of a Dedicated Session at the EAGE Annual Meeting in Oslo. Session convenors Bjorn Wygrala (SLB, retired), Johannes Wendebourg (TotalEnergies), and Thomas Hantschel (Terranta) report.
Our understanding of natural hydrogen resources is at a very early stage, but there is agreement that the geological systems and modelling approach developed for petroleum systems in hydrocarbon exploration is transferable to the geologic properties and processes controlling the generation, migration and accumulation of natural hydrogen. The hydrogen systems concept provides a geoscientific framework for structured exploration risk analysis and, accordingly, the talks in the Dedicated Session followed a similar logic covering: hydrogen sources, hydrogen exploration, and hydrogen systems modelling.
Hydrogen sources
There is currently a general agreement that the dominant source of natural hydrogen in the subsurface is serpentinisation, a high-temperature (250-300°C) water/rock interaction process that occurs in iron-rich rocks. The generation process is rapid at geological time scales and recent discoveries of active hydrogen seepage in the subsurface, as found for example in coal mines, confirm our understanding of this process. Two other generation processes are radiolysis and very late maturation of organic matter such as coals.
Moretti et al from University of Pau presented pyrolysis results from coal samples in Colombia that show that hydrogen generation from organic matter starts after methane generation at about 210°C and can continue until 400°C. The conclusion was that ‘the potential of organic matter to generate H2 must be taken into account’, and ‘the resource potential of H2 is substantial’.
Horsfield et al from GeoS4 noted that the ability of organic matter to generate H2 is independent of the type of kerogen, and that the main generation occurs in the 200-300°C range. The potential H2 yields are high, and assessments have been made in many basins. ‘Organic matter is a proven source of H2, however due to the high reactivity and mobility of H2, the extent
to which H2 can be preserved remains unclear’.
Hydrogen exploration
The proof-of-concept for the existence of accumulations of producible natural hydrogen is the Bourakébougou field in Mali. Key points are documented in two recently published papers (Maiga et al, 2023 and 2024). Since 2012, production from a stacked Neoproterozoic reservoir system without any pressure decline shows that the hydrogen reservoir is a dynamic system that is being progressively recharged at the production timescale. This observation already indicates some key characteristics of H2 accumulations to guide global exploration efforts.
Brouwers et al from Getech presented technical exploration strategies using a hydrogen systems approach comparable to petroleum systems analysis used in oil and gas exploration. Initial exploration is basin-wide and includes traditional basin modelling to determine temperatures, pressures and regional and local subsurface water flow patterns. Results can then be utilised for hydrogen play-based exploration and play-to-prospect risk analysis. These must then be integrated with commercial factors to address H2-specific challenges. Brouwers concluded that while natural hydrogen resources could evolve to be a very attractive target, significant technical and commercial challenges still need to be overcome.
Lefeuvre et al from Grenoble University presented a method to access and screen legacy geoscience data bases for the occurrence of H2, most of which are non-digital. This is especially important in oil and gas bearing basins with long production histories and thousands of wells (millions in the US!). Here, hydrogen is usually neglected as oil and gas exploration is not targeting hydrogen. However, it is occasionally recorded, and this information can have significant value for hydrogen
exploration campaigns. The authors developed a practical IT solution to screen the entire French national database of the Office of Exploration and Production of Hydrocarbons (BEPH). Two basins of interest have been identified: the Aquitaine Basin where H2 occurrences align with the geological context, and the Paris Basin where H2 occurrences were unexpected. These findings have prompted further geological investigations to characterise the key elements that control the hydrogen systems.
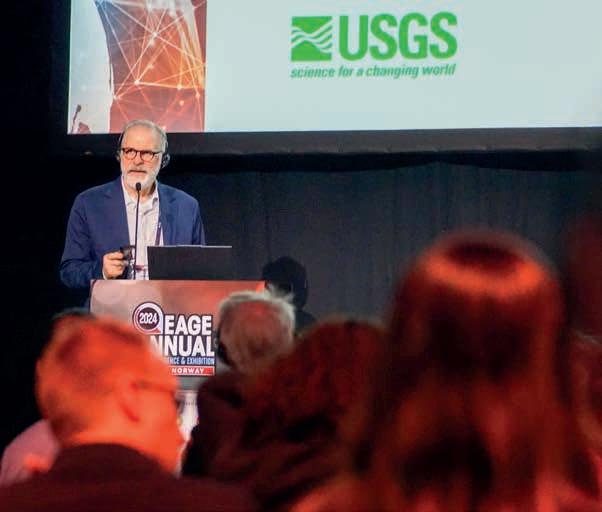
Hydrogen systems modelling
While hydrogen systems might initially appear to have much in common with petroleum systems, there are also critical differences in the controlling properties and processes. Key topics include subsurface water flow systems, their scales and controls including faults, and the time scales on which H2 generation and migration occur. New developments in reactive transport modelling have become important tools to investigate the differences between petroleum and hydrogen systems.
Ellis et al of the USGS summarised current global activities in natural hydrogen exploration and production, including exploration in Mali, USA, France, Spain and Australia, and stimulation efforts in Oman. A central component of these activi-
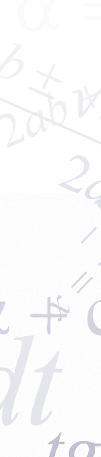
ties is the development of a conceptual geological model of hydrogen systems, using elements from petroleum, mineral and geothermal systems analyses. An example was given from the Mauléon Basin in France where a hydrogen system sourced from the serpentinised mantle is modelled where migration is controlled by meteoric water infiltration and advective flow along faults to charge potential shallower reservoirs. Fault properties and water flow systems are calibrated with hydrogen seeps at the surface. For Ellis et al, the ability to analyse the overall cause and effects of properties and processes in the system demonstrates the value of reactive transport modelling to guide natural hydrogen exploration.
Cacas-Stentz et al from IFP Energies Nouvelles discussed how to model faults and their properties which are essential in modelling solute transport, e.g., lithium, and migration of free and dissolved natural hydrogen. Applications of regional scale hydrodynamic modelling to natural hydrogen systems were presented, demonstrating the sensitivity of potential accumulation sites to fault properties and scale issues, and highlighting risk factors related to migration and entrapment of hydrogen. It was further noted that new applications of these adapted simulators are not only related to hydrogen systems, but also to mineral systems.
Hidalgo et al from SLB discussed how traditional petroleum systems modelling software can be adapted to also model hydrogen systems. The dominant process
computational frameworks of reactive chemical processes. Other generation processes that can be modelled are radiolysis of water and decomposition of organic matter. Hydrogen transport is handled by PT-controlled aqueous transport. Hidalgo opined that hydrogen migration is best modelled using invasion percolation as it provides an optimum balance of accuracy and processing performance, whereas entrapment can be based on standard petroleum systems approaches.
Palmowski et al from Terranta presented a new-generation simulator specifically designed for hydrogen and mineral systems. Hydrogen generation via serpentinisation is a geologically very rapid process, especially at temperatures >200°C. Another generation process is radiolysis, a slow but continuous process which is based on the split of water by radiation from rocks containing traces of radioactive elements. Both generation and related transport processes can be simulated with fast processing tools on present-day geometries. Grids can be refined around fault zones, and processes that are controlled by H2 concentrations in source rocks as well as water availability and flow rates can be investigated. The assessment was that newly developed hydrogen systems modelling tools have reached a development stage that enables complex hydrogen-specific processes and controlling factors to be analysed from regional to local scales.
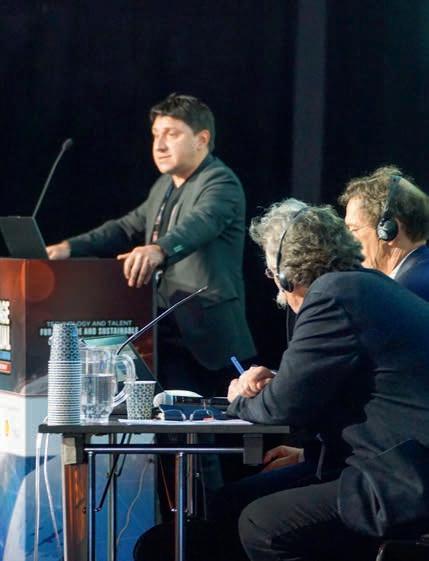
vided an excellent overview of the latest technical developments in this area and a useful range of topics and talks by speakers from research organisations and service companies. Notably absent were E&P operators. They have a wait-andsee attitude towards this new exploration paradigm as commerciality of natural hydrogen so far remains unproven. The session convenors, committee members of the EAGE Technical Community on Basin and Petroleum Systems Analysis, would like to thank all the speakers and remind readers that all extended abstracts are available on EarthDoc.
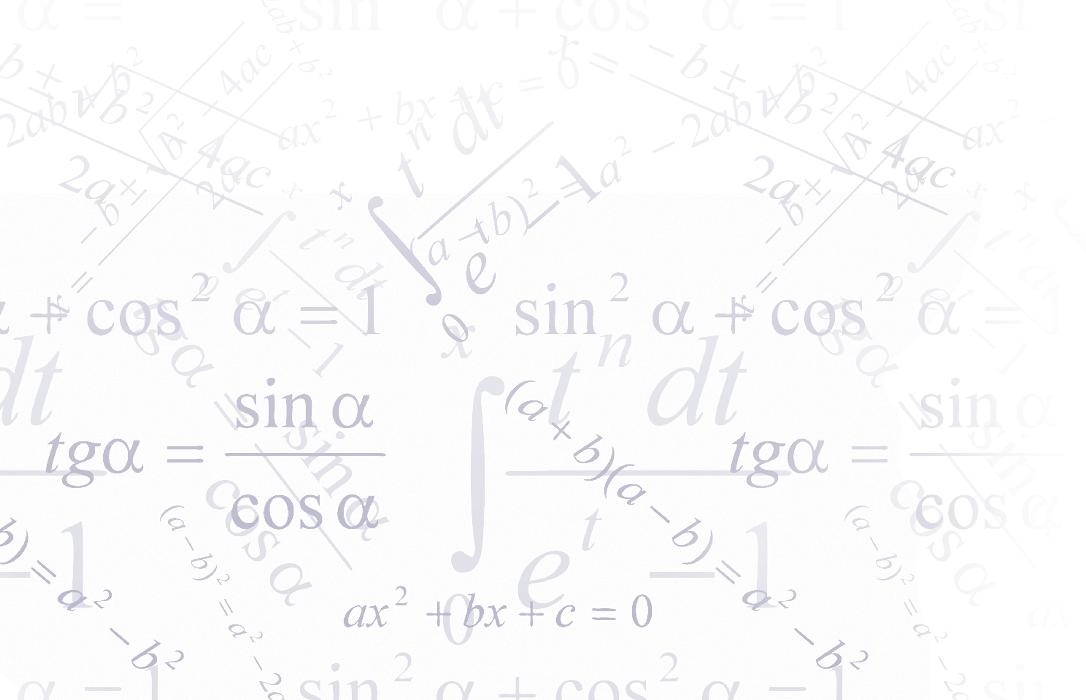
Technical Community on Basin and Petroleum Systems Analysis
EAGE Student Calendar
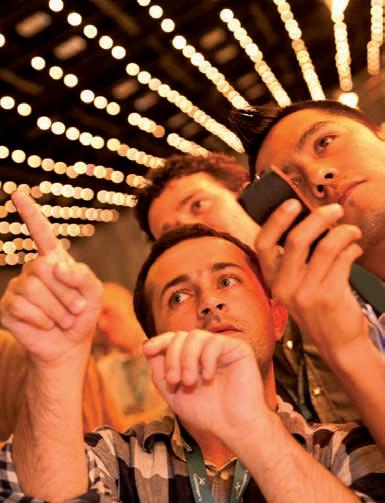
Thematic collections planned for Geoenergy and Petroleum Geoscience
Geoenergy and Petroleum Geoscience , two journals co-owned by the Geological Society of London and EAGE, are aiming to compile thematic collections focusing on the transferable knowledge and integration of various disciplines across the emerging energy hubs of Asia Pacific, Africa, and the Eastern Mediterranean.
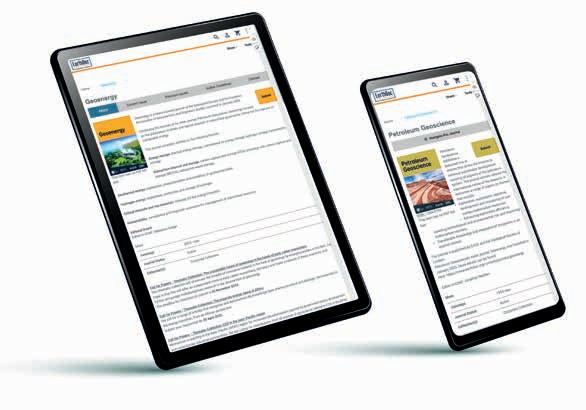
Petroleum Geoscience thematic collection:
Geoscience driving the North Africa and Eastern Mediterranean energy hub
North Africa and the Eastern Mediterranean area is poised to be a critical global energy hub in the 21st century. Unlocking oil and gas resources, developing CCS and new renewable energies such as offshore wind, hydrogen/helium, geothermal, and associated critical resources will add a cyclic economy component and cross sectorial value to the needs of the region.
For this reason, Petroleum Geoscience is launching a thematic collection to further understand the complex regional geology, the development and evolution of structural styles, depositional systems and their implications for the prospectivity, and further potential of North Africa and the Eastern Mediterranean.
Guest editors are: Jonathan Redfern (University of Manchester) and Ioannis Alexandridis (Hellenic Hydrocarbons and Energy Resources Management Company).
Geoenergy thematic collection: CCS in the Asia–Pacific region
Momentum is building in the Asia Pacific (APAC) region for increased decarbonisation spurred by government policy development and cross-border industrial partnerships. Australia, Indonesia and
Malaysia for example are progressing the carbon capture and storage (CCS) hub concept where carbon dioxide can be imported from countries with less suitable geology for CCS such as Japan, Korea and Singapore. These hubs are generally aiming at storage in depleted oil and gas fields or saline aquifers in regions where infrastructure is available and government regulations are in the main supportive. Most APAC governments have set ambitious climate-related targets, although often the current goals are not entirely supported by existing plans in place. McKinsey notes that in order to deliver on the climate pledges made, >60% of the world’s future carbon abatement (>3 GT p.a. by 2050) would have to be realised in the APAC region.
The collection is looking for contributions from all aspects of geoscience related to CCS in the APAC region. The research topics include, but are not limited to: CO2-rock interaction studies on CO2 storage effects; Field-scale studies on reservoir characterisation, injectivity, and storage capacity; Advances in modelling such as multi-phase flow, AI/ML, and uncertainty analysis; Case studies from pilot or industrial storage projects; Geological and techno-economic analysis for CCS hub sites; Developments such as hydrogen or bioenergy with CCS; Cross-border CO2 storage options; and Monitoring onshore/offshore injection reservoirs.
How to submit articles
Guest editors are: Farhana Jaafar Azuddin (Petronas, Malaysia), Lisa Chisholm (Drax, UK), David Dewhurst (CSIRO, Australia), Takeshi Tsuji (University of Tokyo, Japan), and Vikram Vishal (IIT Bombay, India).
Geoenergy thematic collection: The minerals-energy nexus in Africa
Geoenergy is calling for a range of articles that recognise and demonstrate the knowledge base relating to critical and strategic raw materials for the energy transition, from an African perspective, to be published in the thematic collection. Africa has a wealth of energy-critical resources and the nature of the resources (oil and natural gas, uranium, critical metals, and bulk transition metals), their surface or subsurface location, and international demand dictate whether raw materials are a curse or a blessing. The extraction of raw materials is exchanged for environmental damages and accelerating waste generation. This ecologically unequal exchange intersects with raw materialism, which integrates global and local natural and social processes in a competition between economies, to secure access to growing volumes of raw materials at lower costs. The designation of raw materials as ‘critical’ is an action of raw materialism in industrialised economies and an admission of dependence on resource-rich nations. The
Contributions to these thematic collections are welcome until 30 April 2025. Manuscripts should be prepared according to the author’s guidelines published on the Geoenergy/Petroleum Geoscience websites and submitted using the respective journals’ online submission webpages. When submitting manuscripts, make sure to identify the thematic collection by selecting it from the ‘Section/ Category’ drop-down list. Submission is free-of-charge. For queries, please contact the Editorial Office at geoenergy@geolsoc.org.uk (for Geoenergy) and pg@geolsoc.org.uk (for Petroleum Geoscience).
Africa Mining Vision (AMV) lays out Africa’s own response to the paradox that mineral wealth does not create local and regional benefit. The AMV has the aims of increasing knowledge-based services as well as protecting mining workers and communities, and their environment.
Research approaches that will be accepted for this collection include: Petrology and geochemistry of African
ore deposits for energy-critical commodities; Geometallurgical approaches and environmental impacts; Geological and numerical modeling of ore bodies; Extractive waste alongside their risks and opportunities; Societal, economic, and policy issues in raw material exploration; Role of knowledge-based institutions in policy development; Life cycle analysis and resource management strategies for
responsible sourcing of raw materials; and Impact of global warming on extractive operations and worker safety.
Guest editors are: Annock Chiwona (Geological Survey, Malawi), Michael Musialike (Copperbelt University, Zambia), Ishmael Quaicoe (University of Mines and Technology, Ghana), and Gabriel Ziwa (Copperbelt University, Zambia).
Young professional awards announced
EAGE is proud to recognise outstanding young professionals who have become recipients of the newly established EAGE Marie Tharp Award, and the Petroleum Geoscience and Basin Research Early Career Awards 2023.
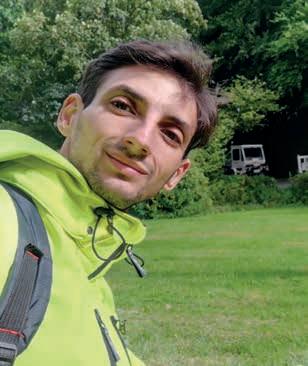
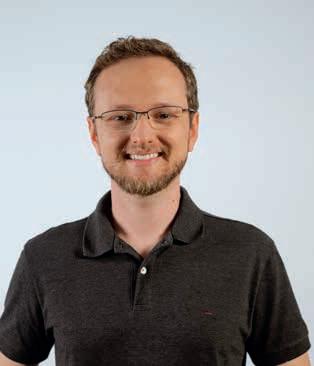
Marie Tharp Award 2024
The Marie Tharp Award is dedicated to recognising promising and creative talents among the next generation of leaders who are committed to transforming energy systems and accelerating the global energy transition. We honour Samuel Zappalà, PhD in applied geophysics at Uppsala University, as the first recipient of this prestigious award.
Valentina Socco, EAGE president, said: ‘Inspired by a talented and visionary scientist who changed our perspective of the Earth, this award is aimed at recognising a talented young professional who will contribute to make our relationship with the planet sustainable’.
Accepting the award, Samuel Zappalà said: ‘In my work with onshore reflection seismology, I focus on acquisition and processing techniques development, often optimised and applied for energy transition purposes. Two examples of this are: my master’s thesis, where advanced seismic reflection imaging methods were
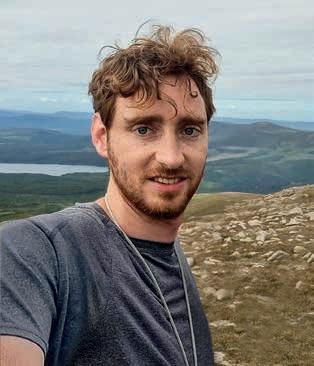
applied to increase the production of one of Europe’s main geothermal fields, and the main project of my PhD, where seismic reflection acquisition and processing techniques were developed and optimised for onshore CCS reservoir investigations. When EAGE announced the Marie Tharp Award, I immediately felt its relevance towards our community and our future. Opportunities like this help us young professionals spread our works and ideas, while encouraging more research focus towards the inevitable transition.’
Zappalà receives a grant to attend the EAGE GET 2024 Conference, taking place in Rotterdam next month, from 4 to 7 November.
Petroleum Geoscience Early Career Award
The Petroleum Geoscience Early Career Award is presented annually for the best paper published in the journal by an author in the early stages of their career. This year, we honour Dr Mateus Basso,
Congratulations to Samuel Zappalà, Mateus Basso, and Daan Beelen for their outstanding accomplishments. Their dedication, innovation, and scholarly achievements are paving the way for a more sustainable and informed approach to our industry’s challenges.
research geologist at CEPETRO-UNICAMP, for his paper ‘Characterisation of silicification and dissolution zones by integrating borehole image logs and core samples: a case study of a well from the Brazilian pre-salt’, published in Petroleum Geoscience, 29(3).
The Aptian, non-marine carbonate reservoirs of the Santos and Campos basins, offshore Brazil, are particularly known for being an unusual succession of carbonate facies affected by a complex diagenetic history. Over the past two decades, the knowledge of these pre-salt carbonates has greatly advanced, yet many scientific-frontier problems remain unsolved. Among the geological puzzles of the PreSalt, the silicification process has played a major role in creating and modifying reservoir characteristics. The study by Basso et al. (2023) focuses on the geological and petrophysical characterisation of a special well that penetrated silicified reservoir intervals in the Santos Basin. By integrating well-recovered cores with
a suite of well logs, the study provides insights into the different types of silica and their relationships with natural fractures and carbonate dissolution. The study underscores the importance of integrating borehole image logs and core samples description as well as multi-scale petrophysical approaches to properly assess the impact of silicification and carbonate dissolution.
The study contributes to our understanding of carbonate reservoirs affected by silicification and dissolution while providing support for the recognition of such processes in partially- or non-cored wells.
Mateus Basso says: ‘Being honoured with the Early Career Award is a deeply appreciated recognition for our work. The award inspires us to persist in our efforts to further advance the understanding of the remarkable carbonates in the South Atlantic provinces.’
Basin Research Early Career Award
The Basin Research Early Career Award is presented annually to recognise research published in the journal that marks a
significant step forward in our understanding of sedimentary basins and completed within three years of thesis completion. We congratulate Dr Daan Beelen (assistant professor, Utrecht University) on winning this year’s award for his paper ‘Predicting bottom current deposition and erosion on the ocean floor’, published in Basin Research, 35(5).
The deep ocean is the world’s most extensive surface type, yet it remains the least understood. In fact, most of our planet consists of the abyssal plain, yet only 15-20% of these regions have been mapped to any extent, let alone in high resolution. Although often considered relatively featureless, these areas harbour fascinating geological treasures that are largely unknown to the general public. For example, the Zapiola abyssal dunefield, located offshore of Argentina, contains giant, moving abyssal dunes that are over 120 m tall and cover an area ten times the size of England.
Daan Beelen explains: ‘These regions are not only of scientific interest but also hold significant potential for natural resources. In my paper, I aim to predict the
distribution of thermohaline bottom current deposits and zones of erosion across the entire ocean floor. Understanding these patterns could provide insights into where potential resources and scientifically important sedimentary deposits are located. For instance, zones of persistent bottom erosion can deflate surrounding sediments, isolating and locally enriching the ocean floor with mineral-rich nodules. These minerals are not only potentially profitable but also critical for technological advancement, especially as the global stock of rare earth minerals is declining faster than it is being replenished.’
Beelen adds: ‘With this award, I hope to give a small impetus to deep ocean geological research, particularly in the abyssal plain, which I believe is extremely understudied. Fortunately, the available data on deep ocean geomorphology and sedimentology is rapidly increasing, with projects like HYCOM providing state-ofthe-art model predictions and deep-sea data publication initiatives like GEBCO. I hope that future studies will build upon my work and lead to even more fascinating research.’
Fifth carbonate well injection and productivity workshop returns to Doha
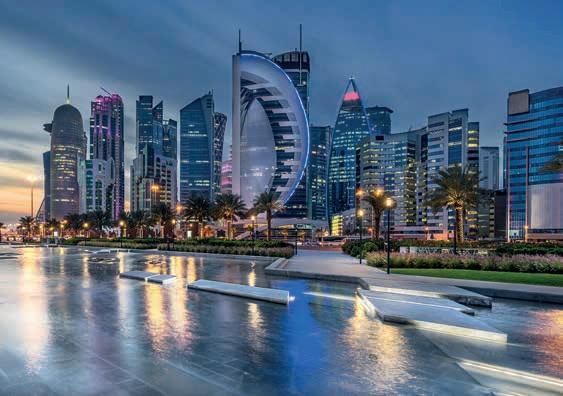
The fifth edition of the EAGE Workshop on Well Injectivity and Productivity in Carbonates (WIPIC) will be held from 14 to 16 April 2025 in Doha, Qatar. It is a follow-up to the very successful fourth edition which was held from 21 to 23 March 2022 also in Doha and attracted more than 100 participants.
For optimal field development, injectivity and productivity of wells are essential, especially in heterogeneous reservoirs such as carbonates. However, incorporating other elements, such as geological modelling, reservoir simulation, reservoir management, history matching, uncertainty assessment, and enhanced recovery mechanisms, is crucial as we move towards a multi-disciplinary approach to problem-solving. Feedback from the initial WIPIC workshops had already pushed us to expand the workshop scope, instead of just focusing on well injectivity and productivity. We will therefore continue to do so for this fifth edition, whose theme will be ‘Innovative Technology for Reservoir Optimization’.
For this upcoming WIPIC workshop, we are excited to introduce new topics: digitalisation, machine learning, and
artificial intelligence. These cutting-edge technologies promise to revolutionise our approach to reservoir management by enhancing data analytics, predictive modelling, and decision-making processes.
With representatives from national oil companies, international oil companies, service providers, universities, and research institutes, this workshop is designed to appeal to subsurface specialists in well stimulation and completion, log analysis, petrophysics, pressure transient analysis, reservoir geology and geophysics, applied mathematics, data science, geo-statistics, and reservoir engineering. It will also be valuable for subsurface generalists and reservoir managers.
WORKSHOP REPORT
Middle East workshop highlights key hydrocarbon seals challenges
A successful Fourth EAGE/AAPG Hydrocarbon Seals Workshop was held in Al Khobar from 13-15 May 2024, sponsored by Saudi Aramco and co-chaired by Dr Ali Al-Ghamdi and Dr Hussein Al-Hoteit. This is what transpired.
The workshop saw excellent participation, with 62 attendees from the Gulf region, including Saudi Arabia, Kuwait, UAE, Oman, and Malaysia, representing both industry (e.g., Aramco, KOC, PDO) and academic institutions (e.g., KAUST, KFUPM, Khalifa University). The diverse mix enriched the discussions and facilitated valuable knowledge sharing.
Over the three days, the workshop covered a range of topics, including qualitative and quantitative seal evaluation, seal capacity, and integrity assessments. The event included interactive presentations, core displays, panel sessions, and discussions. It began with a welcome speech by co-chair Ali Al-Ghamdi (Saudi Aramco) and inauguration remarks by Dr Mohammed Al-Duhailan on behalf of Mr Hafez Al Shammery, vice president
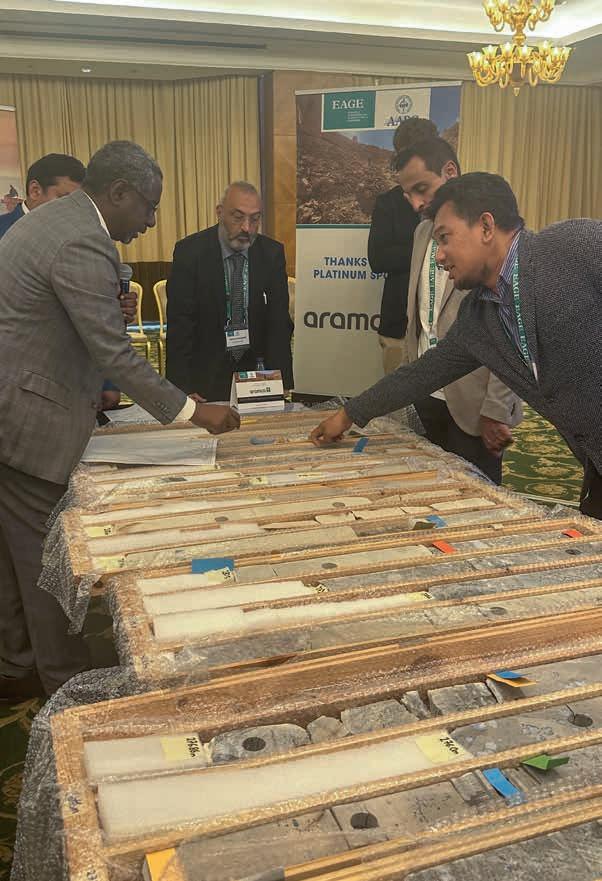
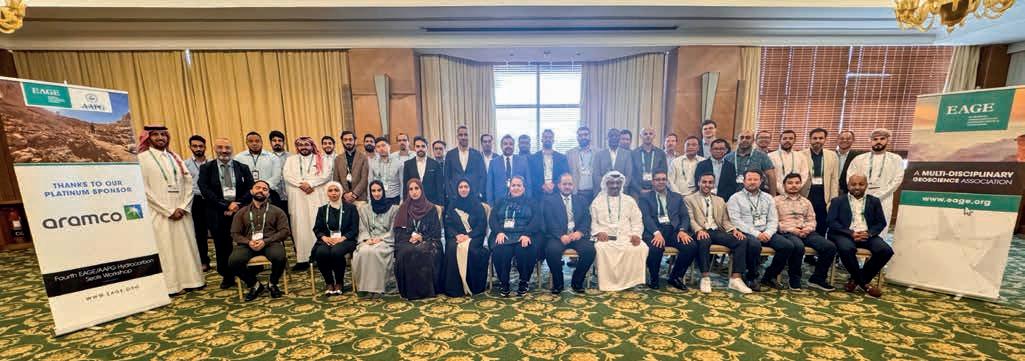
of prospect portfolio development in Saudi Aramco Exploration.
On the first day, core sections from the Jurassic and Triassic periods were displayed, with participants divided into groups to discuss and log the cores. The hands-on experience was well received, allowing participants to directly interact with the geological samples.
The second day featured a panel session focused on the critical role of seal integrity in emerging applications like CO2 sequestration and hydrogen storage. Experts shared insights from industry experiences in the North Sea and Australia, highlighting the limited knowledge in hydrogen storage. The session sparked interesting discussions on the challenges and opportunities in these areas.
The workshop also included a session on ‘Regional and intraformational seal stratigraphy’, where various presentations covered seal stratigraphy in carbonate and clastic depositions. For carbonates, discussions centred on the Hith and Arab anhydrites, key seals in the Middle East, and their mineralogical composition and rock strength, particularly in the context of CO2 storage. Challenges in mapping boundaries between porous and tight facies in carbonates were also addressed, emphasizing the importance of integrating geophysical and geological methods.
For clastic deposits, the workshop highlighted the challenges of early Silurian seal rocks, including sub-seismic resolution, poor seismic data quality, and limited penetration. The session emphasized the need to understand the lateral extent and thickness of these seals, using seismic forward modelling and sequence stratigraphy to address uncertainty issues.
A subsequent talk focused on identifying and characterising muddy fluvial elements, which are crucial for effective stratigraphic sealing. The recognition of their extent and connectivity in the subsurface was highlighted as a key tool for de-risking heterogeneous subsurface targets.
Traps and the risk of cap rock failure in CO2 storage projects were also discussed. A study examined lithological layers to assess their sealing integrity, emphasising the role of tight carbonate facies as top and lateral seals for candidate CO2 storage reservoirs.
The ‘Seal capacity evaluation’ session began with a discussion on evaluating the seal potential of the Hith formation. This research is part of Saudi Arabia’s efforts to assess the risks of carbon capture and storage (CCS) developments, aiming to mitigate global warming by preventing greenhouse gas emissions. The study explored the rock’s behaviour and reaction to CO2 exposure,
providing valuable insights for future research and applications.
Another presentation examined the influence of CO2 on carbonate rock structure, essential for well stimulation and flow assurance. The presence of CO2 was shown to trigger calcite dissolution, enhancing pore spaces and weakening rock strength, while its absence led to calcite precipitation, reducing permeability. The session also mentioned the use of mercury injection capillary pressure (MICP) analysis and artificial intelligence-powered multi-resolution graph-based clustering (MRGC) techniques to predict petrophysical rock properties and electrofacies in uncored wells.
The ‘Seal integrity evaluation via structural and fluid assessment’ session featured talks on evaluating top and fault seals using structural and fluid analysis methods. One talk focused on tracking
leakage and entrapment across faulted gas fields, using fluid data, PVT data, fault interpretation, and migration models to assess top seal integrity. Another discussed the evolution of LWD deep and ultra-deep resistivity techniques for geo-mapping highly resistive seals, emphasising the use of resistivity logs for detecting and mapping top seals. The final talk in this session explored enhancing fault seal analysis through realistic uncertainty assessment, investigating the impact of uncertainties from seismic horizon and fault picking on fault seal analysis, and proposing a more probabilistic approach.
The session on ‘Technology and sustainability of seal assessment techniques’ covered topics related to technology and sustainability in seal assessment. The first talk analysed the wettability, sealing efficiency, and storage capacity of a proxy caprock for hydrogen storage,
finding that sealing efficiency decreases with increased pressure and organic acid concentration but increases with temperature. Another presentation discussed adsorption isotherm models for CO2 and CH4 gases on tight sandstone, coal, and shale formations, identifying the BET model as the best fit for experimental data. The final talk examined the wettability of the CO2/brine/kaolinite system, showing that increased pressure and salinity raise the contact angle, reducing capillary entry pressure and making CO2 less likely to be effectively sealed beneath the shale caprock.
The workshop concluded with discussions on assessing seals for emerging applications, including CO2 sequestration, hydrogen storage, and geothermal energy. Participants identified key issues such as environmental impact, automation, simulation, and project costs.
Time to get on board 2025 EAGE Mentoring Programme
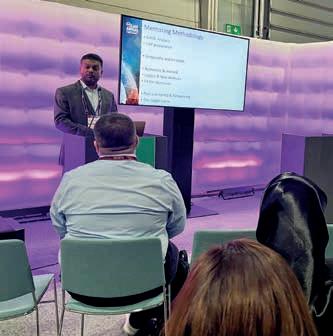
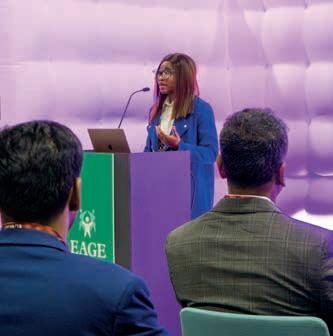
EAGE’s highly successful Mentoring Programme, open to EAGE members in all career stages, is due to begin soon. If you think you can benefit either as a mentor or mentee, sign up before 15 November to participate in the 2025 Programme.
The idea is to connect seasoned professionals with those in their early careers to share knowledge and experience and to provide advice.
For Ellie MacInnes (Viridien), the chance to participate ‘has been a wonderful opportunity to share what I’ve encountered and learned over a diverse and sometimes challenging career in the oil and renewables industry. Conversations with my mentee have also given me fresh perspectives on career outlooks and on the issues faced by mid-career professionals.’
Johan Alejandro Ibarra, undergraduate student from Universidad de Los Andes, says this from the mentee perspective. ‘The mentoring programme has significantly clarified the real
industry landscape for me. My mentor has greatly assisted me in establishing a stronger professional profile for better international exposure’.
During the Mentoring Meet-up held last June at the EAGE Annual, the mentors and mentees agreed that mentoring is a mutually enriching experience in which they could refine their leadership skills, explore new perspectives, and join forces towards contributing to the future of the field. Chandramani Shrivastava (SLB), one of the speakers, went even further: ‘Engaging with students from diverse backgrounds has been incredibly enriching for me as a mentor. It has given me unique insight into the human aspect of mentoring the next generation of leaders.’
If you are considering the next step in your career, but still not sure which would be the best way forward or you are interested in learning about a different field of expertise, taking part in the Mentoring Programme could be for you.
Fiona Dewey (Wintershall Dea), who has been a mentor for several years now, has witnessed her mentees’ growth. ‘It gives me great pleasure and pride when they finally land that job or reach their goal, knowing that I played a small part in helping someone on their career journey.’
Setting the priorities for EAGE Digital 2025
Glyn Edwards, interim subsurface transformation manager at BP and chair of the EAGE Digital 2025 conference explains the significance of the event being held on 24-26 March in Edinburgh, Scotland.
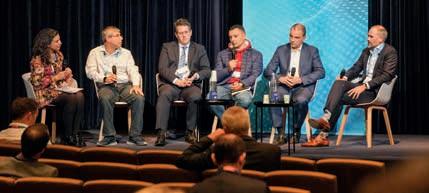
The future demand for oil and gas is highly uncertain, with forecasts differing drastically depending on whether global society transitions to a net-zero trajectory or remains on the current path. This uncertainty creates a short-term investment horizon, making it increasingly important to invest with a high likelihood of achieving expected returns.
Given the vast amounts of data we can now access, along with the volume
of historical data and learnings available, digital technologies are essential in helping us make sense of it all. These technologies not only provide access to all this information but also help us filter it down to the most relevant data, ensuring we don’t overlook any errors or biases.
To guide the discussions at EAGE Digital 2025 we will focus on three key sub-themes. First, with an ever-growing amount of data per person, we must explore how to enable geoscientists and engineers to automatically prioritise the most relevant data and distinguish the signal from the noise. Second, we will consider how our digital tools can combine this data to support timely decisions that are reliable when viewed from a portfolio perspective. Lastly, we will look at how we can leverage
knowledge capture and sharing to prevent the repetition of past mistakes, particularly in the context of the workforce changes discussed at EAGE Digital 2024.
As we explore these themes together, I encourage you to actively engage in the discussions and share your insights. The future of our industry depends on our ability to harness digital technologies to their full potential: let’s make sure we’re prepared to invest wisely and predict accurately.
The call for abstracts is open! We invite submissions on a wide range of topics that explore the advanced applications of digital technologies in our industry. For detailed information visit eagedigital.org.
Join the seismic inversion discussion this month in Naples
The 3rd EAGE Conference on Seismic Inversion on 14-16 October in Naples, Italy will mark the first-ever in-person gathering for the event series, following two successful online editions in 2020 and 2022.
The theme ‘Revealing the subsurface’ alludes to the primary objective of the conference to discuss recent advancements, breakthroughs, and future trends in seismic inversion methodologies and their applications. The aim is to foster dialogue and explore the technological advances and case studies on a diverse range of topics, such as rock physics, data conditioning and fitness, layer properties prediction, azimuthal anisotropy, thin layer property estimation, detuning, hydrocarbon saturation, direct hydrocarbon interpretation (DHI) and risk analysis, modification of prospects, and EOR drainage strategies, FWI and other methods.
This year’s conference will provide a platform for knowledge exchange and networking opportunities with top specialists in the geoscience community, bringing together experiences in conventional and unconventional resources, carbonates and clastic regions, fostering grounds for an exchange of knowledge.
The three-day programme will feature technical presentations showcasing various methodologies and applications utilised in the industry, presented through both oral and poster formats. The event will conclude with a field trip to the active Campi Flegrei caldera, offering participants a unique opportunity to observe Bradyseism in Pozzuoli’s historic centre. This volcanic field, characterised by a 12 km circular structure encompassing various volcanic features, is currently exhibiting renewed activity marked by hydrothermal anom-
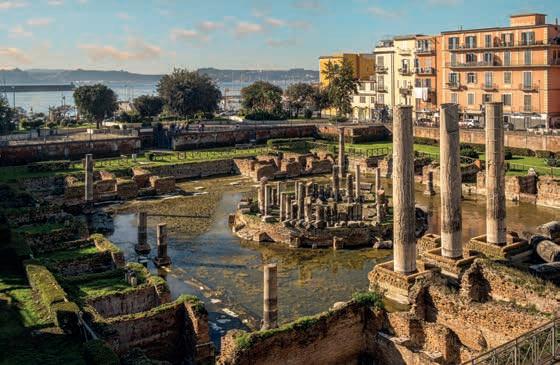
alies, increased seismicity, and ground uplift centred in Pozzuoli. During the walking tour, participants will have the unmissable opportunity to visit the Roman buildings that have recorded volcano-tectonic activity over the past two millennia.
For more information on the conference programme and registration, visit www.SeismicInversion.org.
International CCUS event planned for Bergen next year to focus on engineering solutions
An inaugural World CCUS Conference is being planned for 1-4 September 2025 in Bergen, Norway, aiming to explore the latest advancements, strategies, and best practices in CCUS, and to forge partnerships that will shape the future of the field. The aim is to establish a premier, cross-organisational, and inter-disciplinary technical event, focusing on efficient solutions to address global technological and industry challenges in CCUS.
The event is a collaborative effort between the Norwegian Academy of Technological Sciences (NTVA) and the Danish Academy of Technological Sciences (ATV), with support from individual members of the National Academy of Engineering (NAE), the Royal Academy of Engineering, and the Chinese Academy of Engineering (CAE). EAGE will be acting as organiser and promoter of the event on behalf of the institutions involved.
The World CCUS Conference takes as its context the International Energy Agency (IEA)’s Sustainable Development Scenario that puts the needed CCUS capacity at around seven gigatonnes by 2050. This growth in CCUS is only one of the multiple actions needed to achieve a 50% reduction in emissions by mid-century. Expansion of renewables, significant energy efficiency measures and sustainable bioenergy will also be vital.
Guiding the conference’s strategic vision is a distinguished Board of Directors featuring seven leading representatives from both industry and academia across Europe (Equinor, Norwegian University of Science and Technology, Carbongeo, and Technical University of Denmark),
the US (Stanford University, University of Houston), and China (China University of Petroleum - Beijing). Notably, Professor Rui Zhenhua from China University of Petroleum, Beijing, who also holds the UNESCO Chair in Green Transition for Carbon Neutrality and Climate Change, serves on this Board. The Board’s expertise guarantees high-quality discussions and actionable insights, further enhancing the conference’s global impact.
Tao Yang, chief professional and senior specialist at Equinor and co-chair of the World CCUS Conference, says: ‘While geological CO2 storage currently sees the most activity, we aim to launch a new CCUS event that emphasises engineering and explores a broader spectrum of solutions. Our goal is to accelerate the implementation of CCUS technologies across all areas.’
Philip Ringrose, professor at NTNU and founding member of this initiative, goes on to explain: ‘This event is focused on speeding up decarbonisation activities globally. The world urgently needs more projects capturing, utilising and storing CO2 across all sectors - industrial emissions, energy-related emissions and in support of Carbon Dioxide Removal (CDR) projects.’
The conference programme intends to cover a spectrum of topics:
Geological CO2 storage and utilisation: Latest research on safely storing captured CO2, pioneering techniques for its utilisation in various industrial applications, geological storage capacity quantification, trapping mechanisms, and CO2 monitoring technologies.
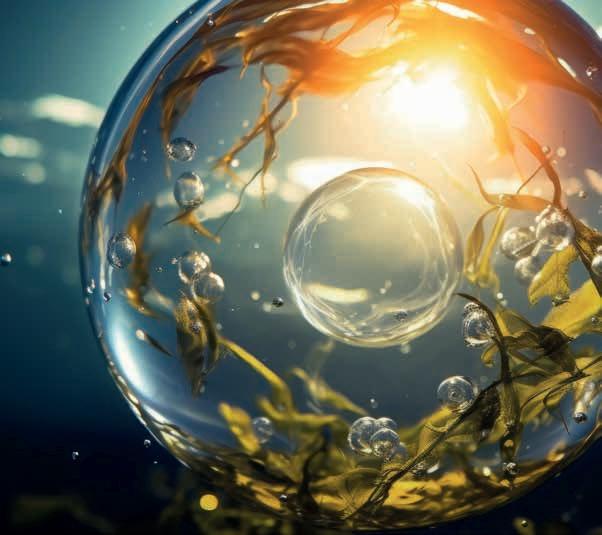
CO2 capture and transportation : State-of-the-art technologies and logistics for capturing CO2 from emission sources and transporting it to storage facilities. Development of CCS hubs and transport networks and novel ways of reducing costs.
Emerging technologies: Updates on emerging technologies such as negative emissions, chemical and biological utilisation, AI/ML applications, and net-zero/ climate-positive systems analysis.
Policy and socioeconomics: Comprehensive discussions on public perception, business environments, regulatory frameworks, and the economic implications of CCUS strategies, as well as the necessary skillsets, communication strategies, and training opportunities to tackle global CCUS challenges.
Updates on this innovative, worldclass conference initiative can be found at www.wccus.org.
The EAGE Student Fund supports student activities that help students bridge the gap between university and professional environments. This is only possible with the support from the EAGE community. If you want to support the next generation of geoscientists and engineers, go to donate.eagestudentfund.org or simply scan the QR code. Many thanks for your donation in advance!
Personal Record Interview
From journeyman geo to multi-client vocation
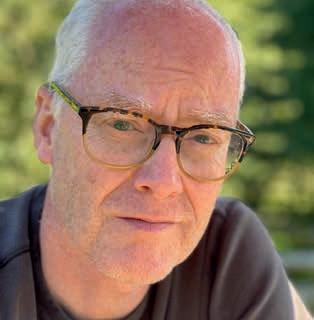
Geologist Neil Hodgson only realised his true métier was multi-client survey analysis when he began work at Spectrum and then Searcher Seismic. It has been his preoccupation ever since and subject of many articles (including First Break ). He describes himself as a journeyman geo in his previous roles at BP, Premier, and a start-up struggling to make a profit in Russia.
Dad’s unusual job
I grew up in Sandhurst, a military town 50 km from London where my dad based himself for his frequent adventures in Africa selling British radio equipment, and then new-fangled ‘computers’ the size and temperament of HAL 9000 in the 2001: Space Odyssey movie. I played a lot of sport as a kid but my world changed when I was introduced to caving. Although free diving flooded passages isn’t everyone’s gig, I found the knack for climbing in the dark, a metaphor for an explorer’s life if ever there was one. Subsequently, a bored careers advisor told me to be a geologist – and I’m still trying.
Highlights of student years
At Manchester University in the 1980s, I was frankly most interested in climbing rocks. Then at Leicester University, volcanoes, trace elements and running around ocean islands of the world looking for impossible rocks (carbonatites) filled my doctorate years, hard rock study was not ideal as a potential career path yet luckily BP was looking for an ‘eclectic’ intake, and clearly curiosity was prized more than any actual knowledge.
First job
BP changed my life. I got to work offshore at a wellsite, exposed to a colourful new language and I started to learn about critical thinking and depositional systems. Operations suited me because I was interested in improving the way drillers and geos inter-
act, especially for planning those scary, high temperature and pressure wells in the UK’s Central Graben. I was among the first BP people to be sent to Glasgow when the company got involved with Britoil. I had some great mentors who guided me through some weird geology, unravelling the salt tectonics story of the Central North Sea. Working with the great structural geologist Frank Peel was a revelation. After a few decent oil discoveries in the Diapir play fairway in the North Sea, I left to work for British Gas, becoming exploration manager in Cairo, Egypt. I loved the country and the people, although it was perhaps an adventure that I was probably not ready for. Fortunately, a bright flat-spotted anomaly jumped out of our 3D survey in the Nile Delta covering a migration shadow on the Rosetta fault. It became the Rosetta Field. Similarly, we acquired 3D over the West Delta deep marine and unexpectedly made the Scarab, Saurus, Sapphire and Saffron discoveries, all named after British pop bands (you guess which?).
Working in Russia
After BG I worked at Premier Oil on global exploration getting a chance to ‘see everything’ and then helped found a start-up oil company where the main difficulties were raising money to work in Russia. What were we thinking? Finding oil wasn’t exactly hard as the Volga Urals Basin has an astonishingly ubiquitous source rock, but making money from what you discovered was almost impossible.
Multi-client is special
Working in multi-client seismic – first in Spectrum and now very happily at Searcher – changed me from a journeyman geo. It has massive appeal compared with ‘normal’ oil and gas. I was suddenly invited to be first finding the hydrocarbon stories hidden in the exploration data and then being able to share those stories. I had found where I was supposed to be.
Whimsy in your writing
There is no point writing a detailed tightly argued case if no one reads it. So our articles are stuffed full of so much whimsy they are likely to sprout ears and sing a song. By wrapping the story up in some cultural reference, I hope the reader understands that we are not saying we are smarter or better explorers – we are just normal people, and we have the data.
Good time for exploration
There has been no better time to be an explorationist than right now. What I really love is that the industry is targeting the amazing passive margin basin floor play in deepwater that hold the world’s biggest plays. This has come together so well in Namibia/South Africa and Senegal/Mauritania, and has told us a lot of what we thought we knew was just not so. New ideas about source rock, heat flow, trapping and crustal structure are appearing on a weekly basis and the ground is shifting quickly.
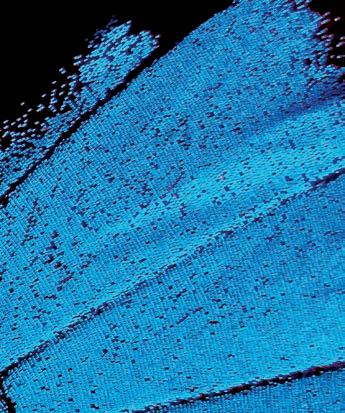
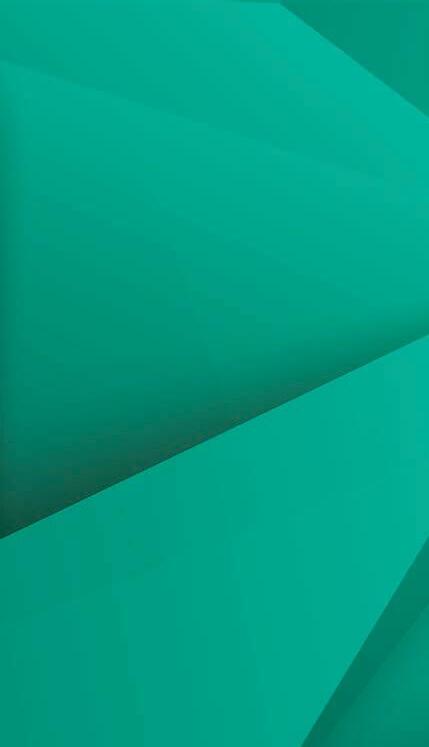
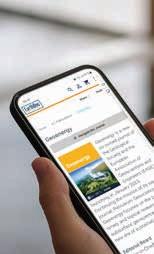
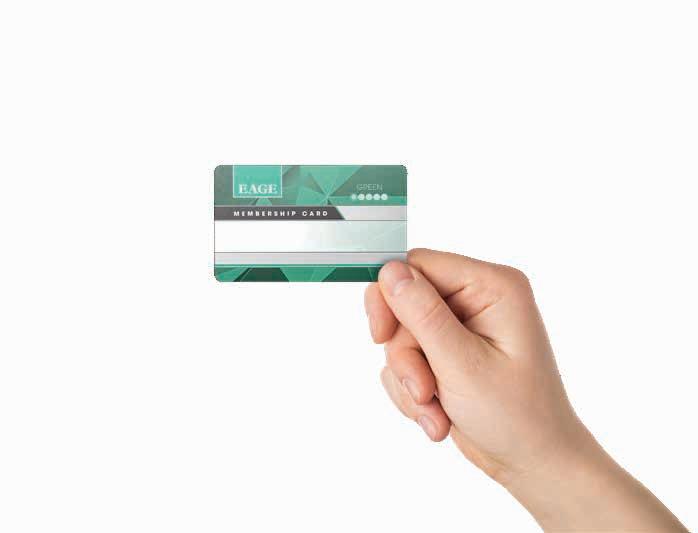
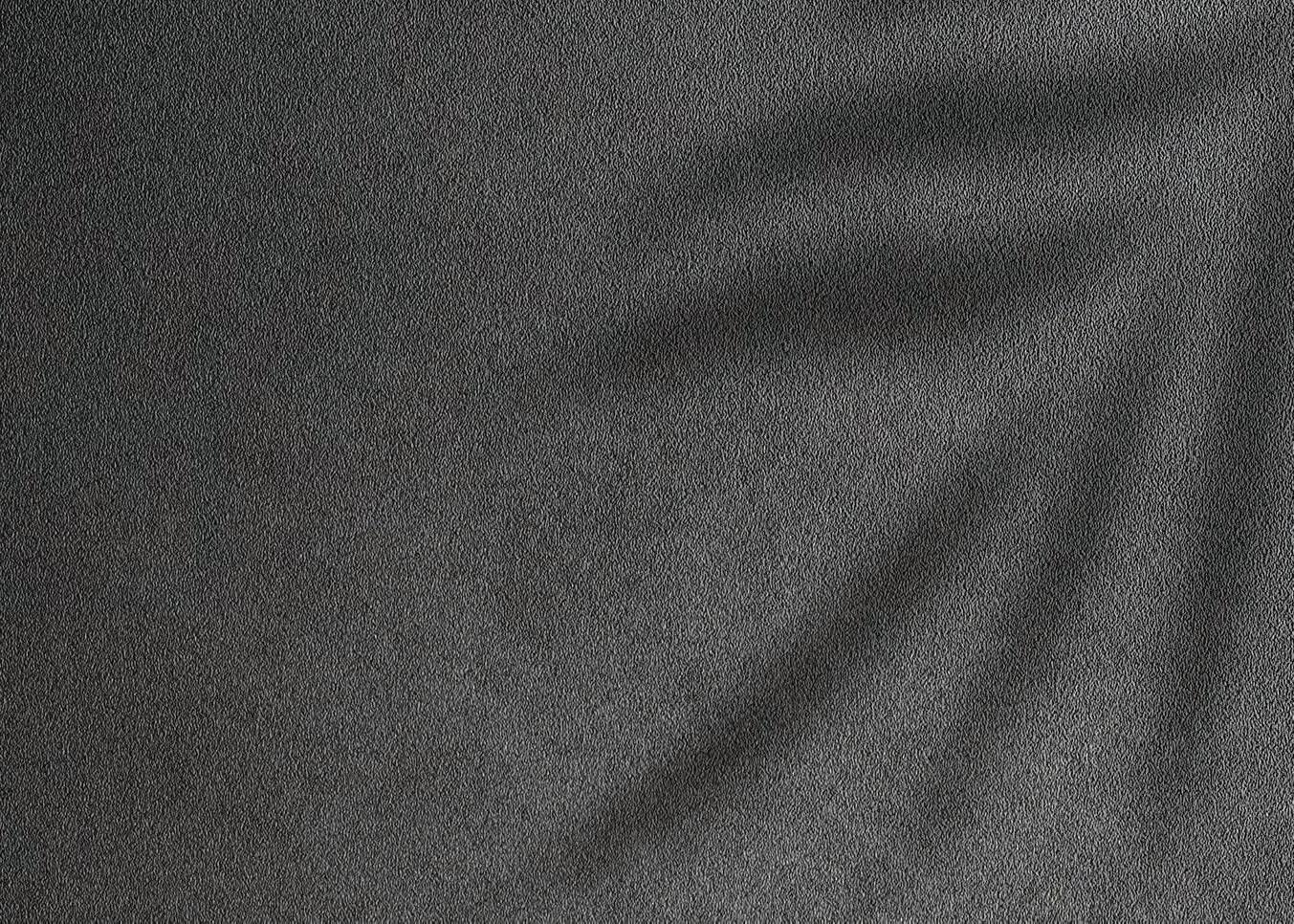
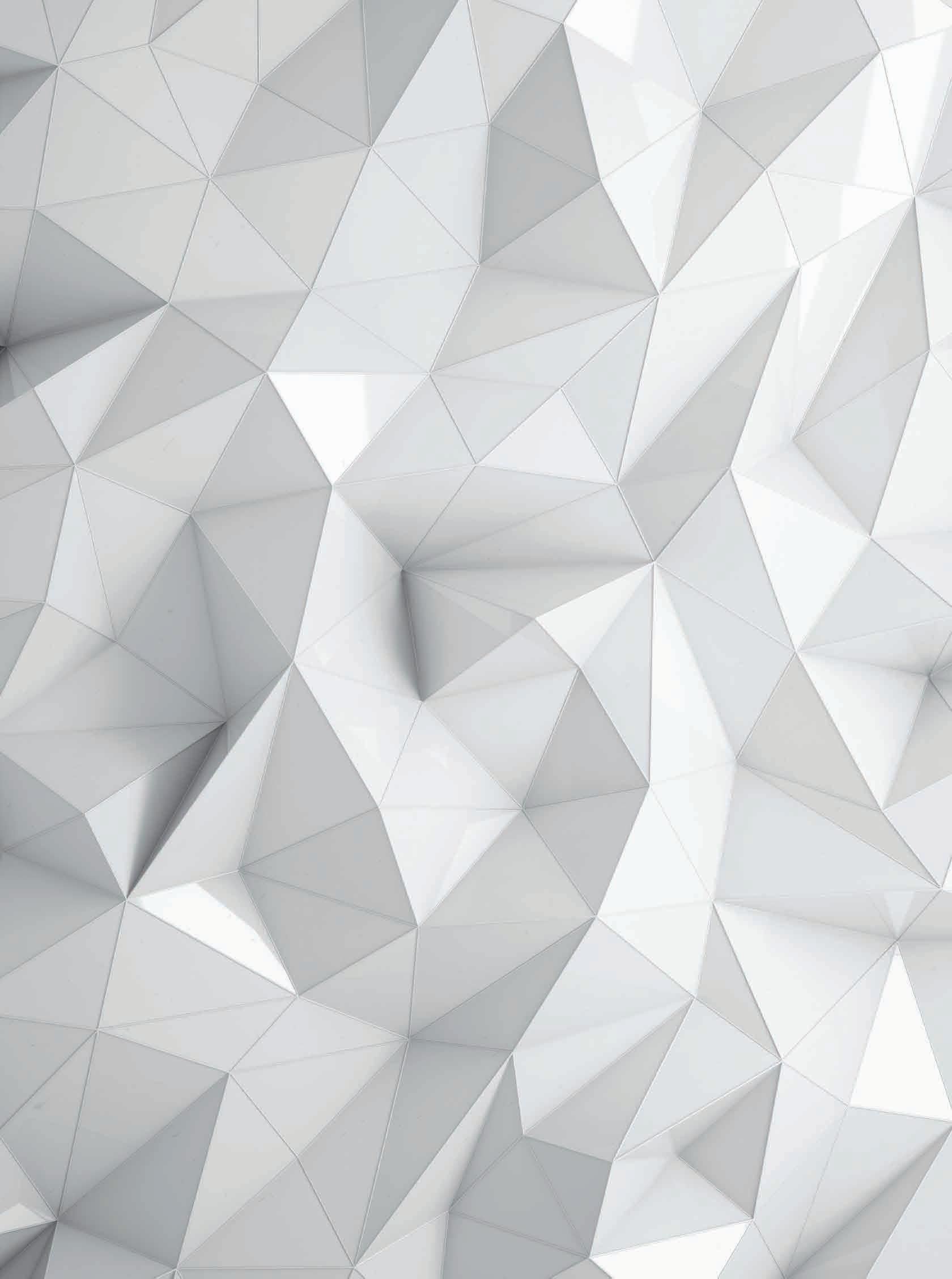
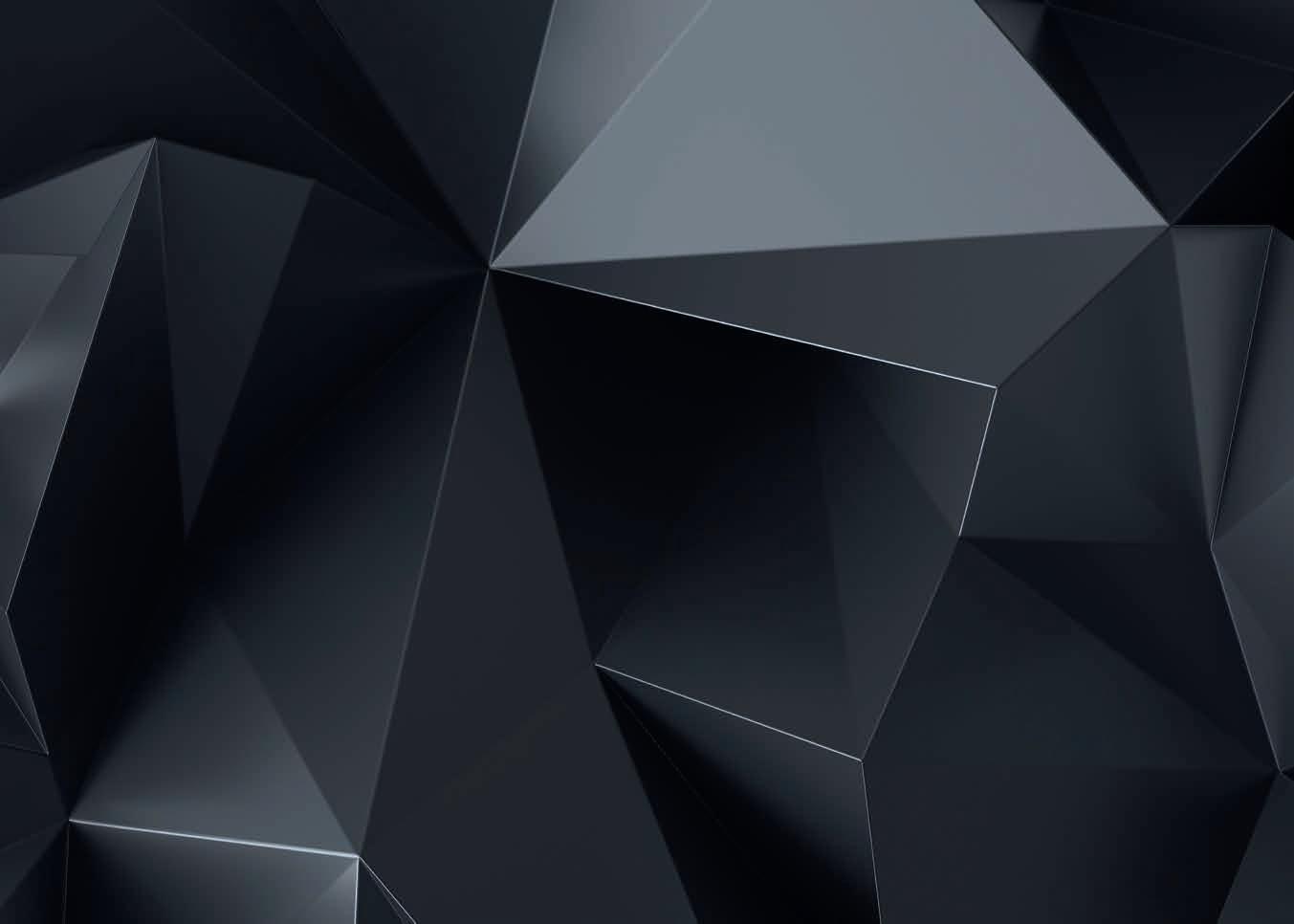
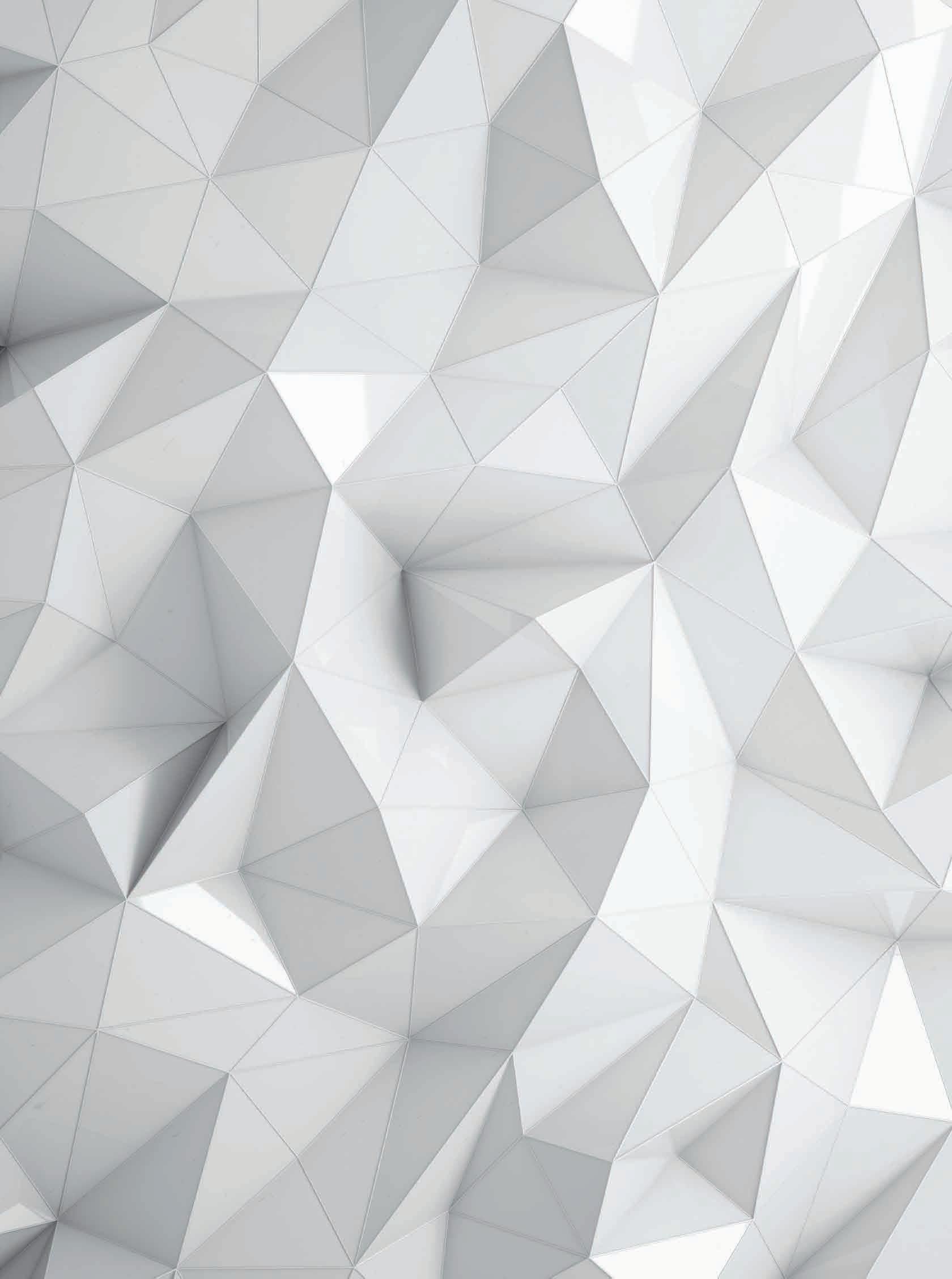
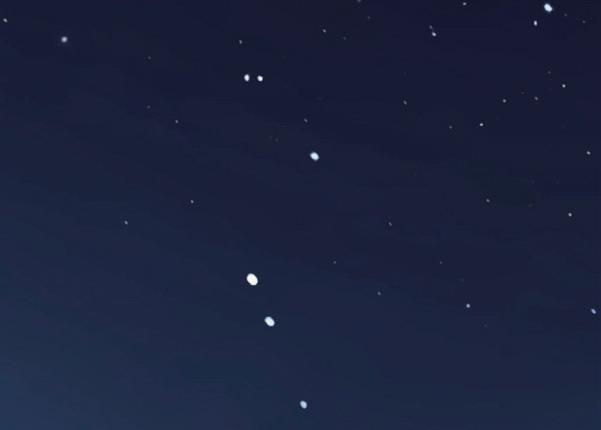
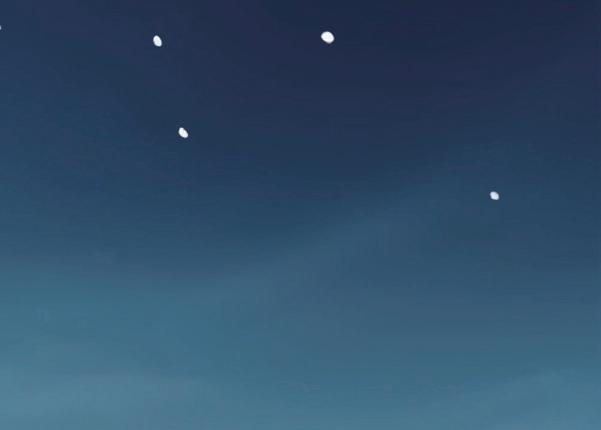
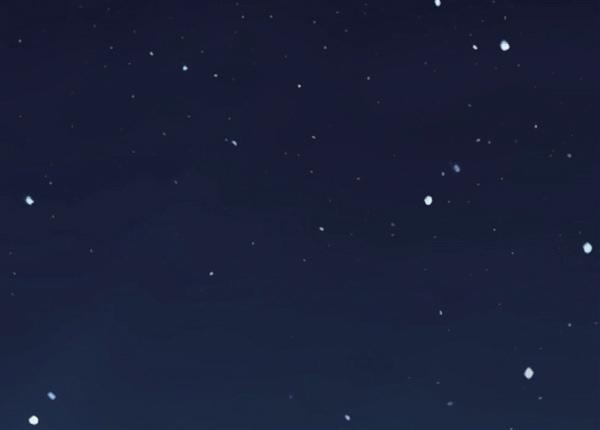

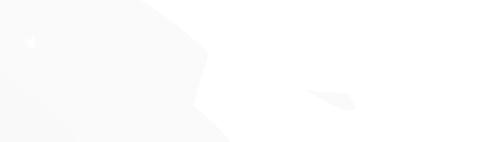
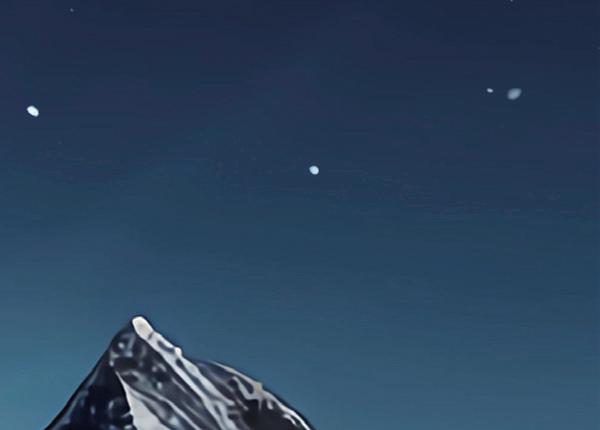
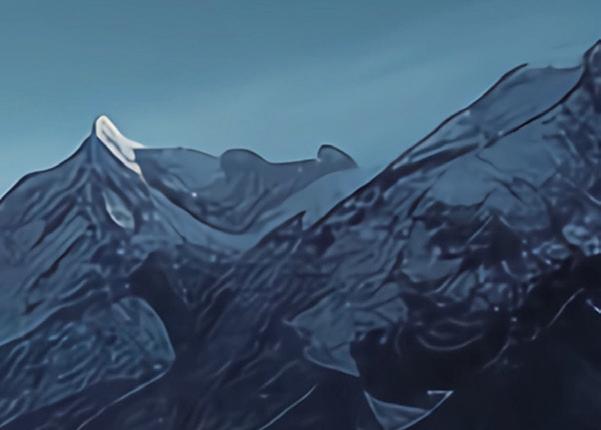
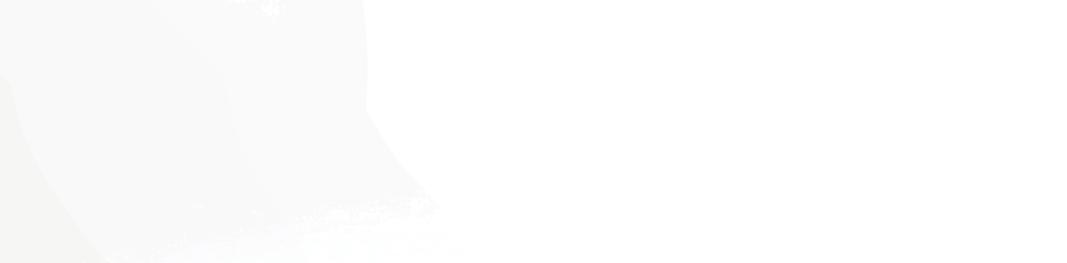
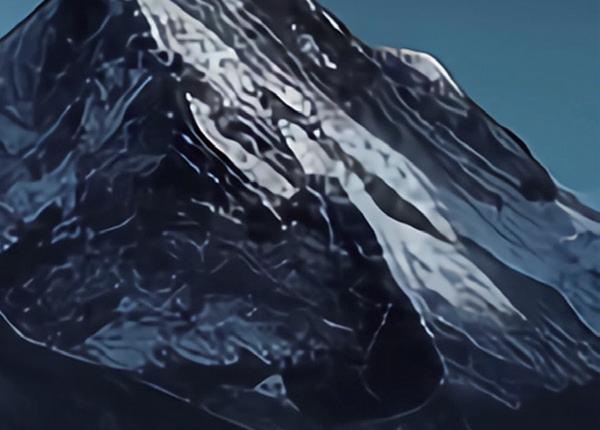
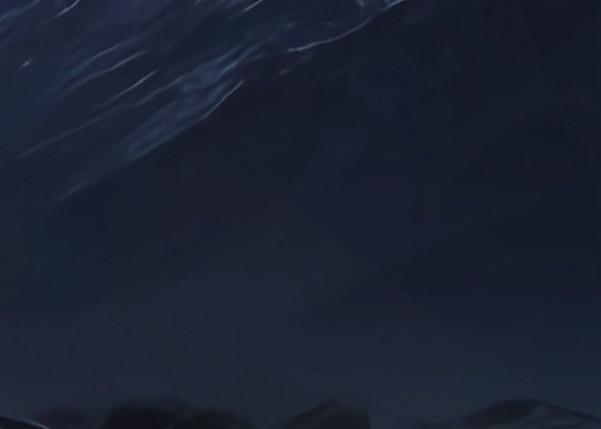
New generation of Seismic instruments
Nodal Seismic data acquisition system
550,000 Seismic nodes to be delivered.
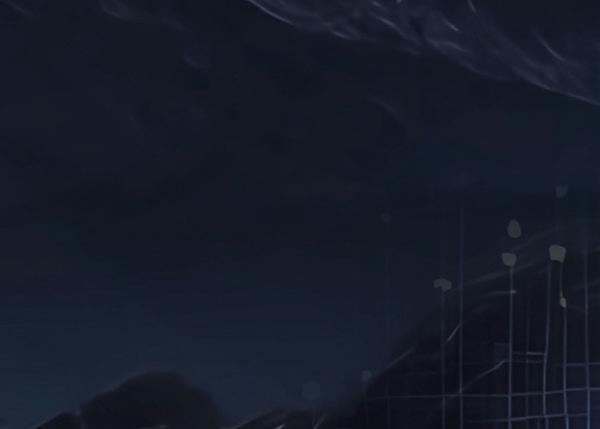
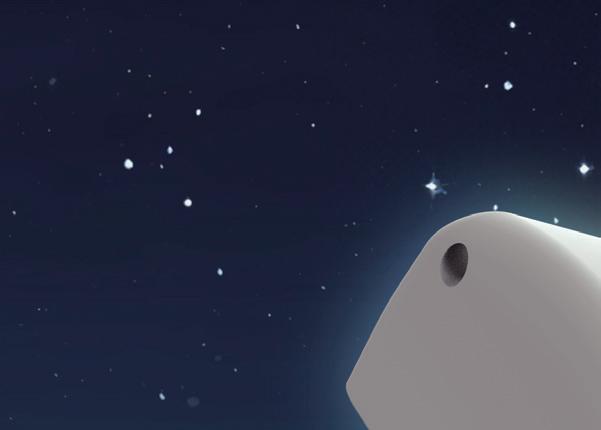
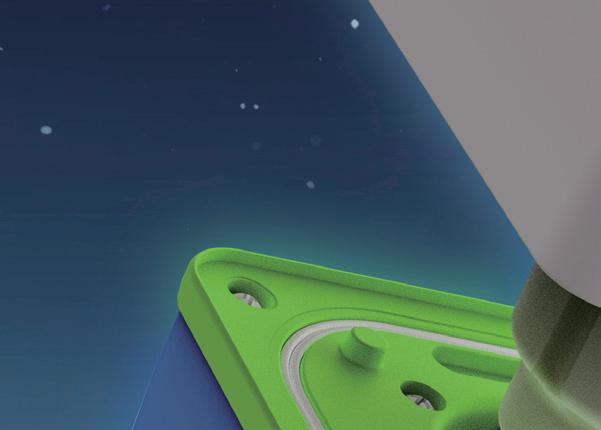
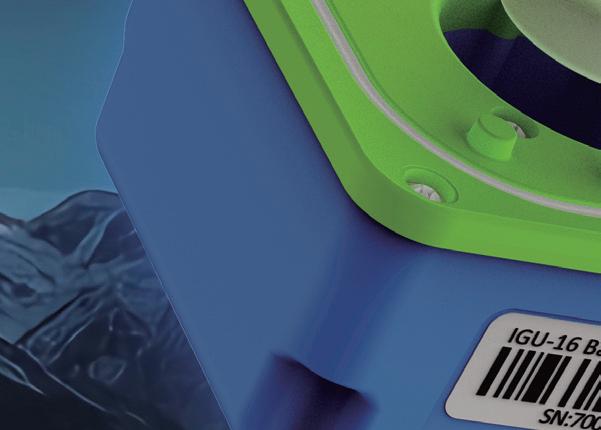
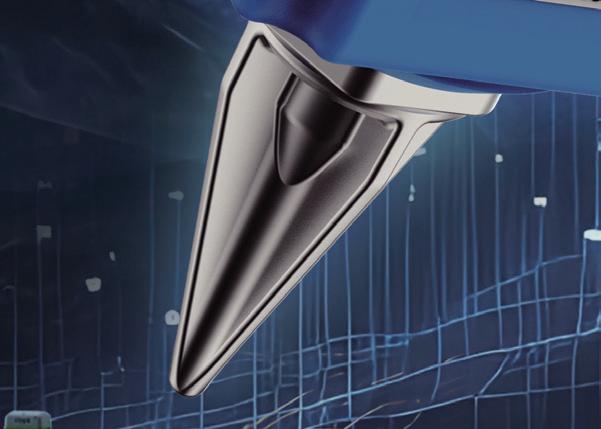
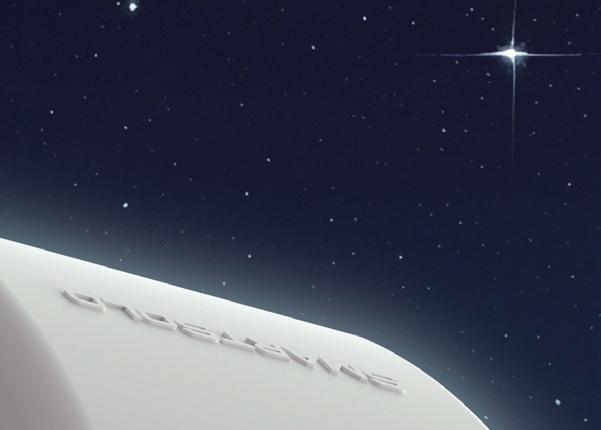
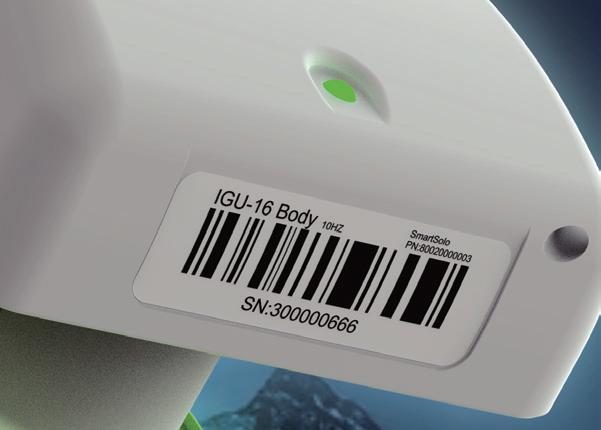
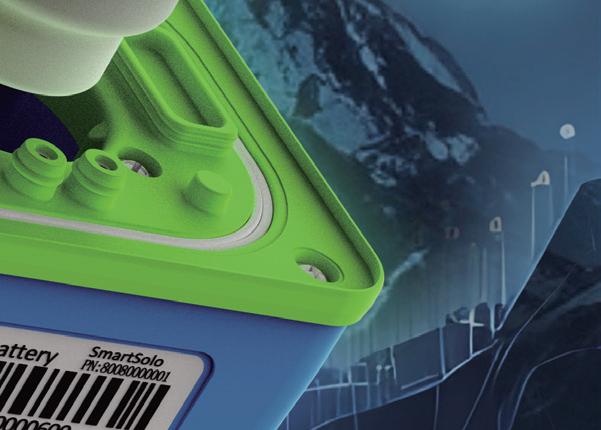
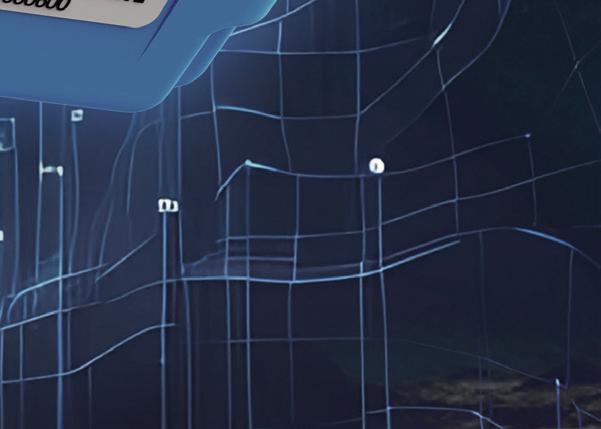
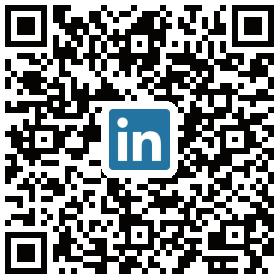
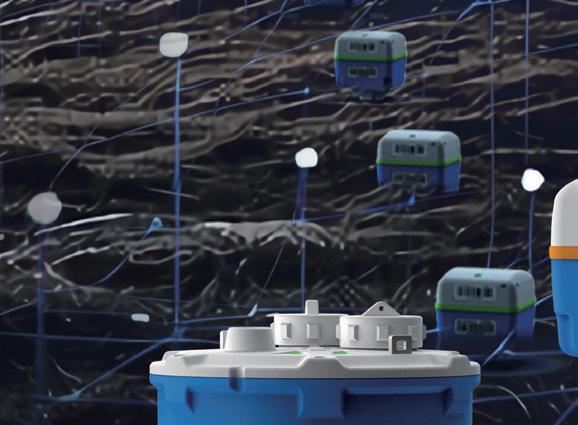
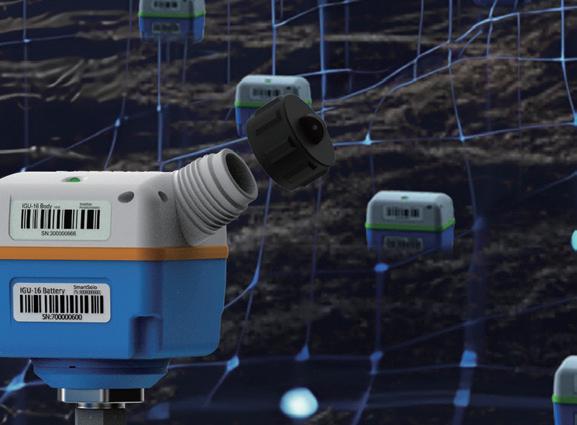

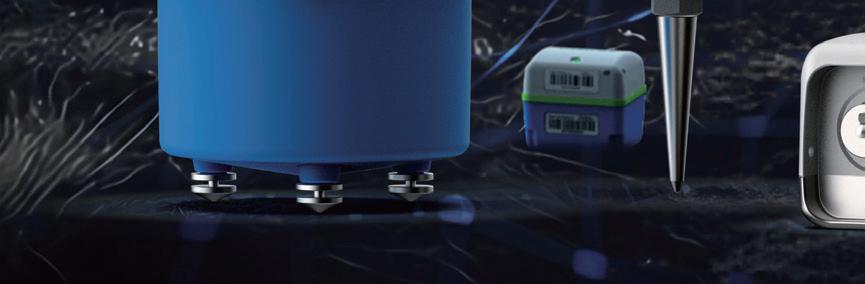
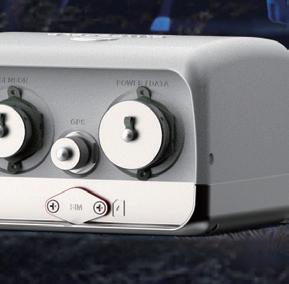
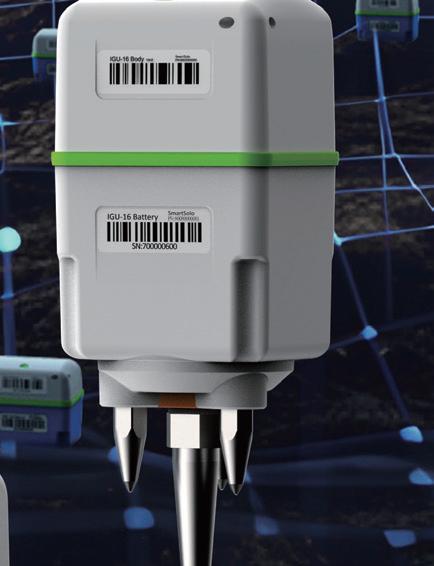
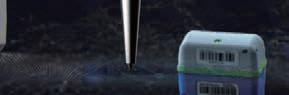
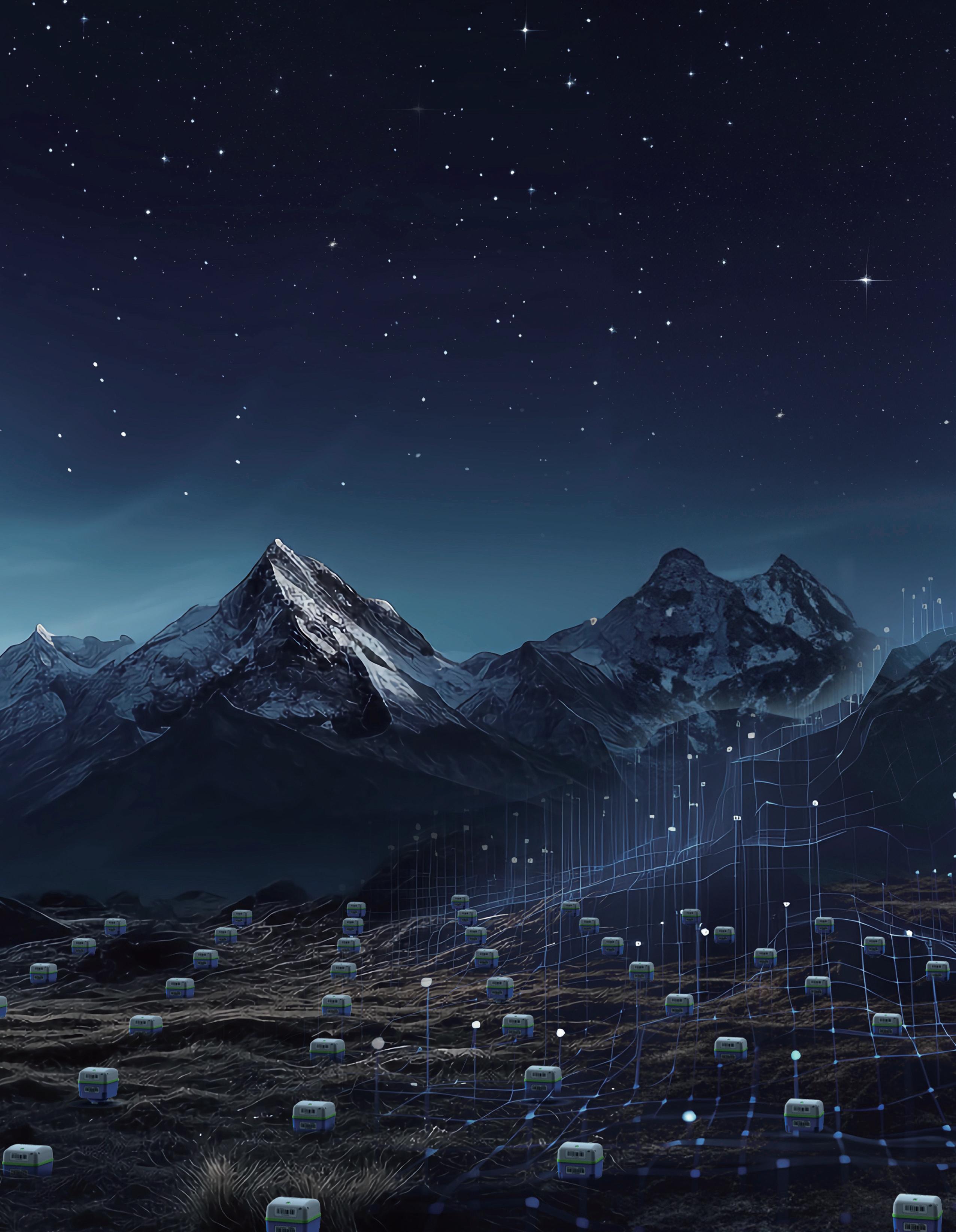
CROSSTALK
BY ANDREW M c BARNET
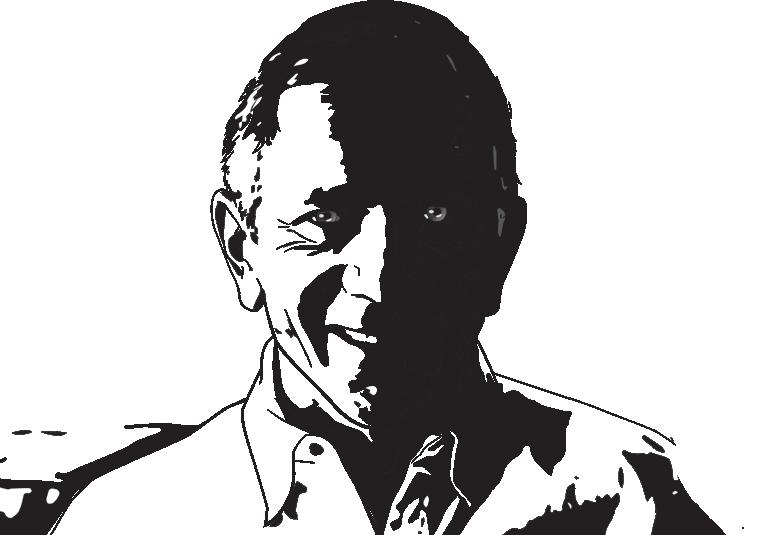
Spot the geoscience
in sport
Reflecting on this year’s memorable Paris Olympics and Paralympics, geoscience has not had much of a look in, not that you would expect it. Reference to studies of geology and geophysics that have much relevance to the world of sports are few and far between. Nor among the world’s active sporting stars, for example, in tennis and golf, do you find among their extensive support teams of trainers, psychologists, PRs, etc a geo of any description, no surprise there.
Yet, if you think about it, the Earth as in undulating terrain plays a huge role in numerous sports be it climbing, cycling, mountain biking, or ski-ing. The underlying geology may not concern participants but nonetheless should offer potential scientific interest. In similar vein, near-surface geophysical methods such as GPR can potentially tell the story of the subsurfaces for soccer and rugby pitches, and help golf course design and maintenance. We can safely assume any analysis of this kind never preoccupies the players in these sports.
Even in the rock climbing and bouldering fraternity, no deep-seated knowledge of geology is required. There are of course plenty of geologists who do participate but for the climber it is the nature of the challenge, not how the rocks came to be formed. They may well understand the basic difference between those walls of sandstone, limestone, granite and slate that are most regularly faced by climbers. But the devil is in the detail, i.e., the nature of the rock surface, whether it is smooth or, in the mountaineering vernacular, does it feature tiny crimper edges to grip on, big buckets, knobs, pockets, slopers, or jugs. Other considerations might include the angle of the rock face and its height, what kind of fractures may be involved (narrow vs. wide, undulating vs. parallel) all of which determine potential hand and foot holds and gear placement. Crucially, will the hand holds or rock bolts support body weight?
so when serious racers in a Grand Fondo or professional tour event review what’s ahead. Yet hills and their geology define any bike ride and in races are often the determining factor in the outcome. They can’t be avoided, so a kind of inspirational resignation is required to meet the challenge. Eddie Merckx, the greatest of them all, remarked ‘Don’t buy upgrades, ride up grades’. The philosphy of Lance Armstrong, who won the Tour de France seven times before his disgrace for having been found to have taken performance enhancing drugs, was brutal but on point observing something along the lines ‘Pain is temporary, quitting lasts forever.’ During a race or a tough ride, the nature of the view is not what is on the cyclist’s mind. It’s all about getting to the top, conserving energy, etc.
‘Underlying geology may not concern participants’
However, for some followers of the major cycling tour events, contours of the routes have become a geological opportunity. Since 2021 you can catch a brief segment during Tour de France TV coverage presented by cycling enthusiasts Douwe van Hinsbergen, professor of global tectonics and paleogeography at Utrecht University, and colleague Marjolein Naudé in which they explain the geology of the often spectacular landscapes crossed by Le Peloton. Their notfor-profit organisation Geo-Sports, supported among others by their university, has now extended its scope to covering the geo-background stories on all Tour de France and Tour de France Femmes stages, the Monument races in Italy, Belgium, France, and the Netherlands’ own Amstel Gold event. Non-cycling competitions such as the Dakar Rally have also been added.
The British Geological Survey website has got into the spirit. It includes a survey of the rocks encountered in the professional Tour of Britain race, and even delves into the natural material that goes into the construction of a bike.
Less surprising, geology doesn’t come up often or ever when a cycling group discuss the route of a day’s outing and even less
The Tour de France is the second most viewed sporting event on TV in the world, unsurprisingly topped by the FIFA World Cup of football which also attracts huge crowds of spectators at
football stadiums. Geophysicists from time to time have taken an interest in the seismic impact of these mass gatherings and similar, e.g., American football and baseball. Currently the stage belongs to Taylor Swift and the seismic activity generated by her sell-out Era Tour concerts. At her Seattle venue the commotion of fans and the recording system triggered the equivalent of a 2.3 magnitude earthquake, according to a seismology team at the city’s Lumen Field.
Such research is not as frivolous as it might seem. The impact of noise and reverbration has implications for urban infrastrucutre, according to Jordi Diaz, associate professor at the Institute of Earth Sciences Jaume Almera in Barcelona. In a presentation to the European Geophysical Union meeting a few years ago, he described the apparently inadvertent monitoring of the Nou Camp stadium, home of the famous Barcelona football team and venue for big musical concerts proving that a Lionel Messi goal does make the earth tremble.
Recordings from a seismometer that had been placed half a kilometre from the stadium ostensibly to monitor traffic and subway activity. In a match against Chelsea in which Messi scored after three minutes, the seismograph spiked like the ‘lie detector answer when the murder swears he didn’t do it’. Later when the game was largely won, Messi scored again with a more muted seismic response. By contrast big name musical concerts produced what the researchers dubbed ‘harmonic structures’, energy localised in precise amplitudes because people are dancing not jumping, so for example at a well attended Bruce Springsteen concert ‘every single song had a particular pattern.’
An Australian team of researchers in a CSIRO paper ‘The application of geophysics to the sport of cricket’ claims that the game of cricket is relatively straightforward, summarised as a batsman using a wooden bat to defend a set of three wooden stumps at one end of a pitch (ideally made from heavily compacted grass) while a bowler attempts to knock them over by bowling a hard leather ball from the other end of the pitch. The batsman aims to hit the ball in order to acquire runs without the ball being caught in-flight by a fielder. If only it was that simple!
The research focuses on the majority of balls that bounce on the pitch before they reach the batsman. The combined width of the three stumps is only 22.9 cm and the pitch is over 20 m long, so accurate bowling is very important. Because a fast bowler can bowl the ball at between 135 and 150 km/h, it is extremly difficult to judge with the naked eye where the ball has pitched. Televised cricket uses a ‘hawk-eye system’ of six or seven cameras to track the ball, and provide a pitch map, said to be prohibitively expensive. The research team found that a 48-channel seismic recording system around the pitch, coupled with basic processing, proved a cost-effective, i.e., much cheaper, method for locating where a cricket ball impacted the pitch, with an accuracy of ±10 cm.
‘A Lionel Messi goal makes the earth tremble’
No one would expect that a sport such as cricket would merit geoscientific interest. But one recent study proves the contrary. This is timely because in 2028 cricket is returning as a demonstration sport at the 2028 Olympics after an absence of 128 years. This may seem surprising when for huge swaths of the globe cricket is virtually unknown, the sport basically being a legacy of the old British Empire. However, inclusion in the Olympics does make sense when you realise that cricket is the second most viewed sport in the world after football, a statistic heavily influenced by the fanatical popularity of the sport in India.
Cricket probably didn’t catch on more internationally because of its Britishness enshrined in extraordinarily complicated rules, and old-fashioned etiquette, all exemplified by terms such as googly, silly mid-off, long-on, yorker, stumped, LBW (leg before wicket) not to mention the ritual tea interval. To be fair, the game at international level has evolved beyond recognition these days from matches lasting a stately five days to the T20 fast, furious and colourful format chosen for the Olympics that makes the game much more appealing to spectators and lasts only three hours or so.
Some of the same research team has considered how geophysics can help cricket umpires decide whether a batsman ‘nicked’ (made contact contact with) the ball with the bat on the way from the bowler to the wicket keeper behind the stumps. Any contact and the batsman is ‘out’. Their solution is to add a three-component sensor to the bat which is much more accurate than the naked eye, TV camera or audio recording used in international matches.
Something scientists are getting round to is the potential impact of climate change on sport and sport’s effect on the environment. For example, an article in the American Geophysical Union publication Eos by a research team led by climate scientist and baseball fan Christopher Callahan found a discernible increase in home runs from 1962 to 2019 in Major League Baseball attributable to increasing temperature trends.
Numerous sports organisations worldwide have signed on to the UN Sport for Climate Action framework linked to achieving Net Zero targets.
The case for more focused environmental research can be found in an article in the Journal of Sport Management entitled ‘Sport Ecology: Conceptualising an emerging subdiscipline within sport management’ by Brian P. McCullough, Madeleine Orr and Timothy Kellison. Meantime, the University of Toronto recently created what must be one of the first academic posts to further the cause of sports ecology focused on the impacts of sport on climate and the role of athletes as climate activists.
Views expressed in Crosstalk are solely those of the author, who can be contacted at andrew@andrewmcbarnet.com.
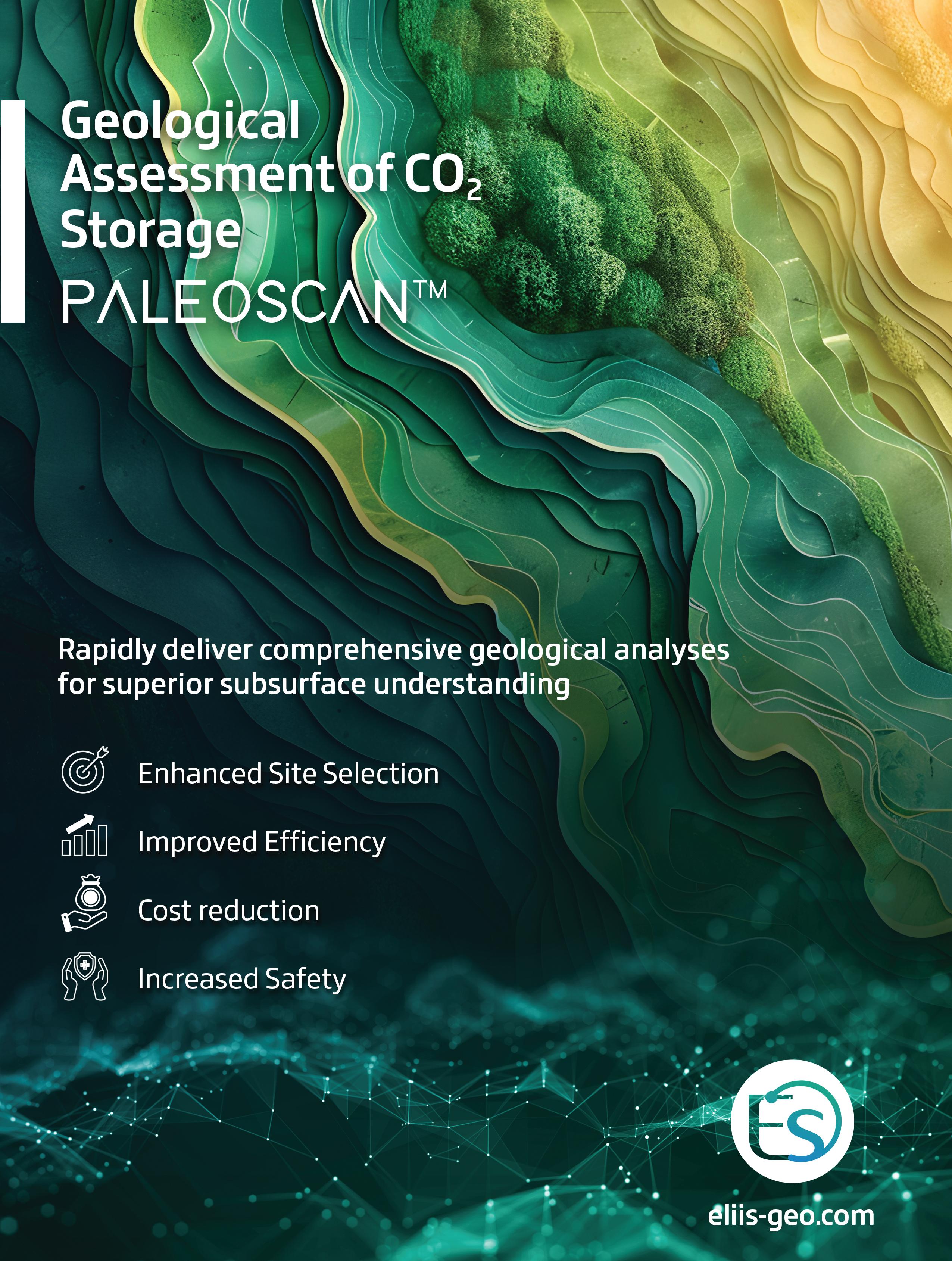
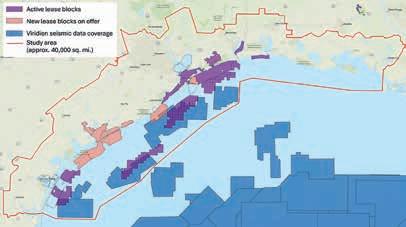
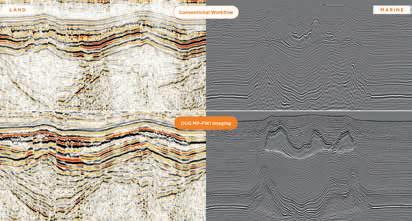
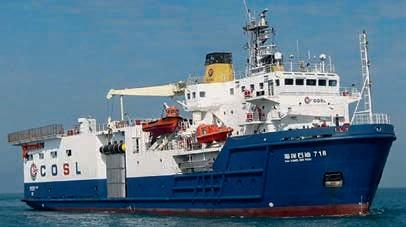
Oil and gas will still provide 75% of global energy demand by 2030, says Rystad
Oil and gas will remain central to the global energy mix for the foreseeable future with global energy demand for hydrocarbons projected to exceed 650 exajoules (EJ) in the coming years, according to research from Rystad Energy.
Rystad estimates that by 2030 more than 75% of total demand will be met by fossil fuels, with emissions climbing as a result. A significant portion of these emissions will originate from upstream activities, particularly hydrocarbon extraction (75%) and gas flaring (25%). This is expected to contribute around 1.1 billion tonnes of carbon dioxide equivalent (CO2e) annually over the next few years.
As investors and governments intensify their focus on carbon-reduction goals, identifying basins that can help to lower the overall emissions impact is becoming increasingly important, said Rystad. Premium energy basins (PEB) – a term coined by Rystad Energy – are particularly valuable because they are rich in hydrocarbon reserves and offer potential for integrating low-carbon energy sources and solutions to reduce emissions, said Rystad.
Having analysed PEBs based on their availability of remaining hydrocarbon resources, development cost, emissions and the availability of new energy sources such as wind and solar, together with their suitability for carbon storage, the Central Arabian and Rub Al Khali basins stand out as carbon-efficient, resourcerich basins with significant potential, said Rystad. ‘These Middle Eastern basins are at the forefront of PEBs and play a pivotal role in global conventional discovered volumes, especially as global discoveries decline and exploration activity peaks,’ it said in a statement. These basins also score highly in terms of renewable potential, with both offering more than 6.2 gigawatts (GW) combined of installed and upcoming solar capacity.
Since 2015, the Central Arabian and Rub Al Khali basins have contributed approximately 40 billion barrels of oil equivalent (boe) in newly discovered volumes, evenly divided between liquids and gas. Egypt’s Nile Delta, driven by Eni’s giant Zohr gas discovery in the Mediterranean Sea, ranks third with about
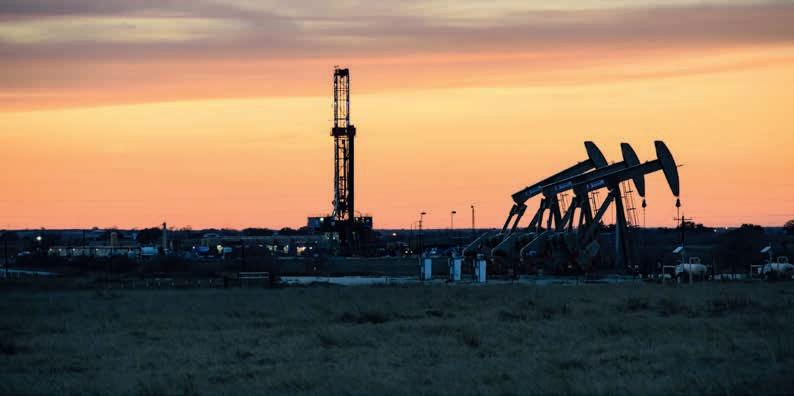
5 billion boe discovered during this period, followed by the US Gulf Deepwater (3.7 billion boe) and the Central Asian Amu-Darya (3.6 billion boe) basins.
With combined capital expenditure of $638 billion, the Rub Al Khali, US Gulf Deepwater and Central Arabian basins have had the highest greenfield investments since 2000. Due to the vast volumes discovered, the unit cost of development in the two Middle Eastern basins has been under $2 per boe. In contrast, the smaller average resource size in the exclusively offshore US Gulf Deepwater Basin has driven development costs to over $9 per boe, with only the Viking Graben Basin ($11 per boe) in northwest Europe having a higher development cost. Significant investments have also been made in resource development in Brazil’s Santos Basin ($153 billion) and Australia’s North Carnarvon Basin ($140 billion).
‘Several PEBs offer significant potential for carbon storage, particularly in late-life or abandoned oil and gas fields, which are suitable for enhanced oil recovery or permanent storage,’ said Rystad. ‘These basins are increasingly being utilised for carbon capture and storage due to their geological properties. Deep-seated saline aquifers are especially promising, with the US Gulf Deepwater Basin leading the way among PEBs in CO2 storage potential, boasting 750 gigatonnes of saline aquifer capacity.’
Shearwater wins contracts offshore India and Ghana
Shearwater Geoservices has launched a three-month extension to its large deepwater ocean bottom node (OBN) survey off the coast of India at depths of 100 to 2900 m.
The extension builds on the execution of the initial six-month scope using the vessel SW Tasman, Sheawater’s purpose-built seismic source and dual ROV operations vessel, and the in-house developed Pearl node.
Irene Basili, CEO of Shearwater, said: ‘Our operation in India has delivered
an impressive performance, confirming the significant operational efficiencies enabled by Shearwater’s unique vessel design and the state-of-the-art Pearl node technology.
‘We look forward to extending this important project with our client and further adding to their successful data acquisition programme this season.’
Meanwhile, Shearwater Geoservices has won a 4D seismic monitoring contract for the Jubilee field in Ghana, operated by Tullow Ghana.
The two-month survey will be conducted in early 2025, utilising capacity from Shearwater’s fleet. This will be the first contract conducted by Shearwater Ghana, in conjunction with local partner Destra Energy; and will include considerable local content participation, according to the company.
Basili said: ‘Our towed-streamer technology is an ideal fit for the Jubilee field, enabling repeatable surveys to provide Tullow and partners with high-quality data in support of better-informed reservoir optimisation.’
Twenty one companies submit APA applications in Norway
Norway has received applications from 21 companies in its Awards in Predefined Areas (APA) 2024.
By the application deadline of September 3, applications had been received from Shell, Aker BP, Concedo, ConocoPhillips, DNO, Equinor, INPEX Idemitsu, Lime Petroleum, M Vest Energy, OKEA, OMV, Pandion Energy, Petrolia NOCO, PGNiG Upstream, Repsol, Source Energy, Sval Energi, TotalEnergies EP, Vår Energi, Wellesley Petroleum, and Wintershall Dea.
‘It’s gratifying to see the continued significant interest in exploring new acreage in mature areas on the Norwegian continental shelf (NCS), even in light of the many awards in
recent APA rounds,’ said Kalmar Ildstad, director of licence management in the Norwegian Offshore Directorate.
The Norwegian Offshore Directorate is evaluating the applications, with emphasis on geological comprehension and plans for exploration of the areas. When production licences are awarded, emphasis is also placed on the companies’ technical expertise and experience, as well as financial strength.
Thirty seven additional blocks were added in May, including three in the north west Norwegian Sea and 34 in the Barents Sea.
The authorities aim to award new production licences in the announced areas in early 2025.
TGS completes CO2 storage assessment of Illinois Basin
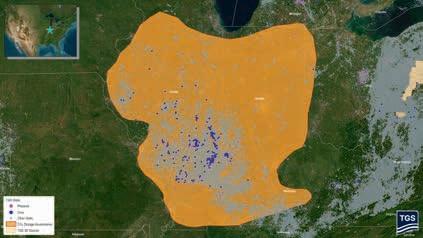
TGS has released the Illinois Basin CO2 Storage Assessment, which identifies prime reservoirs for carbon dioxide (CO2) sequestration across a 66-million-acre region within the Illinois Basin in the US.
With data from 2500 wells and a thorough analysis of key geologic formations,
this assessment provides essential insights into reservoir quality, capacity and sealing integrity – factors crucial for advancing carbon capture and storage (CCS) initiatives.
TGS is offering an integrated evaluation of formation tops and petrophysical characteristics. Insights are then easily accessible within industry-standard workstation environments, enabling informed, data-driven decisions, said TGS.
The Illinois CO2 Storage Assessment provides attribute maps for a whole suite of key reservoir properties and petrophysical curves for thousands of wells across the basin.
This assessment features a stratigraphic framework, petrophysical anal-
ysis and log curve interpretations. It includes regional mapping of storage properties and volumetric visualisations, offering an evaluation of the area’s storage potential.
Coverage of the basin extends across contiguous areas in Illinois, Indiana and Western Kentucky.
Carel Hooijkaas, executive vice president at TGS, said: ‘The Illinois Basin Carbon Storage Assessment sets a new industry standard with its unmatched data coverage and expert analysis, pinpointing the most effective reservoir and seal formations for CO2 sequestration.’
Visit www.tgs.com/carbon-captureand-storage/storage-
Viridien completes carbon storage study in Gulf of Mexico
Viridien has released phase 2 of its GeoVerse Carbon Storage Screening Study of the Gulf of Mexico.
The final product complements Viridien’s multi-client seismic data to provide comprehensive subsurface data coverage over the US Gulf of Mexico shallow waters and coastal areas. Its delivery will accelerate the screening process to identify the high-potential areas on offer in the upcoming Texas General Land Office and School Land Board Request for Proposals for several carbon sequestration leases.
The full product provides a unique integrated package of interactive ArcGIS-compatible screening maps, digitised well data and merged legacy seismic data across the attractive shallow water shelf of the Gulf of Mexico basin where several large-scale aquifer stores are available throughout the stratigraphic section.
Dechun Lin, EVP, Earth Data, Viridien, said: ‘This new Gulf of Mexico study is located over a key area of interest for the CCUS industry with a number of licences already offered for carbon storage and more licensing rounds to come. With our growing footprint of screening studies in the world’s most promising regions, we are helping to address the global industry challenge of accelerating CCUS with our subsurface data, data
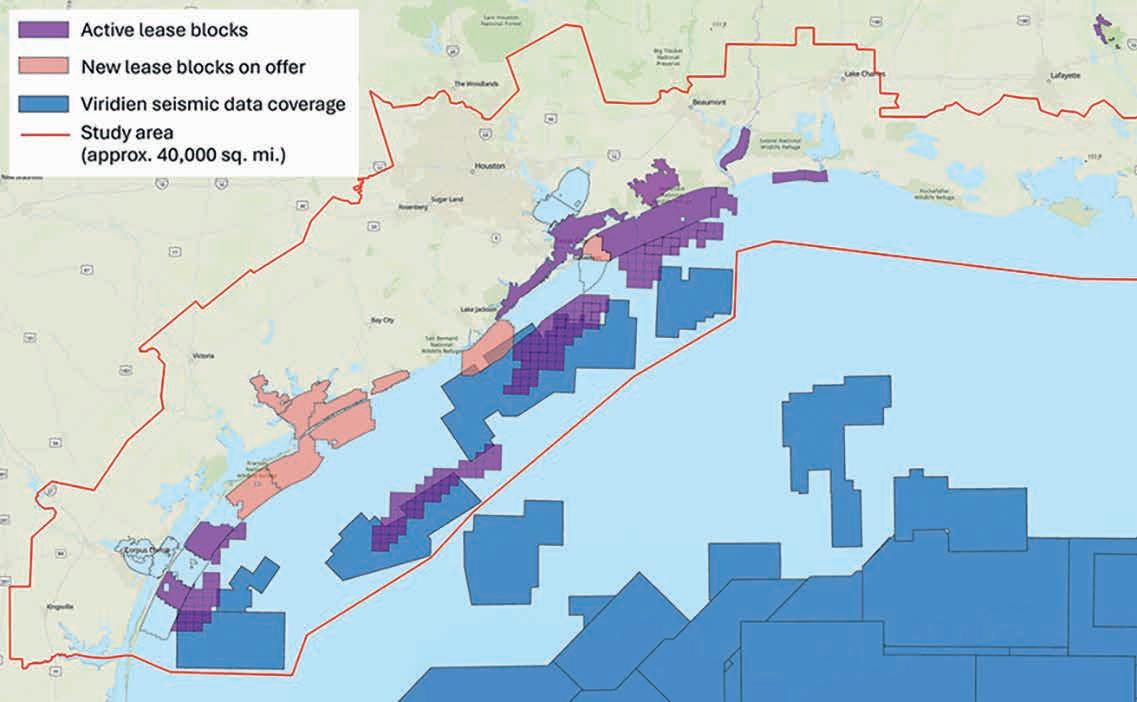
science and geoscience expertise. Viridien is committed to helping operators to identify the areas with the most potential by providing a robust and globally consistent approach to carbon storage screening.’
Both phases of the screening study are available for licensing.
STRYDE wins landmark contracts in Mexico
STRYDE has signed its first contracts in Mexico with Servicios Sísmicos de Exploración (SSE) and SeisGlobe Geoservices.
Under the first contract, STRYDE has supplied 19,080 nodes and the company’s Nimble Receiver System to SSE to enable 2D and 3D seismic surveys, across Southern Mexico.
Javier Nuñez Carbajal, managing director at SSE said: ‘For this project, our customer required high-trace density seismic data across 85 km² of challenging terrain, including tropical rainforests, lakes, and wetlands.
‘Having used other nodal equipment for previous surveys, we are impressed with how STRYDE’s nodes are enabling denser and faster data collection, at the same cost as traditional sparser surveys. In a competitive market where the speed and accuracy of acquisition are critical to the success of exploration projects, this advancement is invaluable.’
Initially, the 3D survey was planned with a 60-m spacing between receivers. However, STRYDE said that its technolo-
gy has enabled SSE to densify the survey design by reducing the receiver spacing to 30 m. The doubling of receiver density will yield significantly higher-definition seismic data, enhancing customers’ understanding of the subsurface and leading to better decision-making, the company added.
Victor Villamizar, head of business development for Latin America at STRYDE, said: ‘By eliminating the complex management of cumbersome cables and unnecessary features, we not only reduce the common logistical challenges and equipment downtime, but also lower overall project costs.’
Under the second contract, STRYDE has supplied SeisGlobe Geoservices with a 3600-node seismic system, to enable the first land 4D3C seismic survey over a field in southeast Mexico, aimed at identifying reservoir optimisation opportunities—crucial for addressing Mexico’s declining oil and gas reservoirs.
Miguel Gomez, managing director at SeisGlobe Geoservices, said: ‘We are
using the latest seismic technology to gather seismic data across a 4 km² area surrounding existing wells and oilfield infrastructure. In this challenging environment, deploying traditional cabled geophone arrays or analogic bulky nodal devices would have been extremely difficult, and expensive.
‘The high-density spatial sampling enabled by STRYDE’s small, lightweight, and cable-free nodes was crucial to the success of this one of a kind project in Mexico.’
Cam Grant, chief commercial officer at STRYDE, added: ‘Having successfully deployed our technology and data processing services across Latin America, including Brazil, Chile, Colombia, and Bolivia, we are excited to see our seismic technology now making an impact in Mexico’s energy sector.’
In addition to supplying the seismic technology for these projects, STRYDE will also provide in-field training and support at the startup of both projects, as well as remote support as needed.
US launches wind energy sales offshore Oregan
The US will hold an offshore wind energy lease sale offshore southern Oregon. The two areas to be auctioned on 15 October by the Bureau of Ocean Energy Management could generate more than 3.1 gigawatts of renewable energy.
The final sale of notice (FSN) includes details regarding certain provisions and conditions of the leases, auction details, the lease form, criteria for evaluating competing bids, award procedures, appeal procedures, and lease execution.
The recently published FSN, which includes two areas offshore Oregon. Lease Area P-OCS 0566 (Coos Bay) consists
of 61,203 acres and is approximately 32 miles from shore. Lease Area P-OCS 0567 (Brookings) consists of 133,792 acres and is around 18 miles from shore.
BOEM also recently published its final environmental assessment (EA) of the possible impacts from issuing leases for potential offshore wind energy development off the Oregon coast, including site assessment and site characterisation activities such as geophysical, geological, and archaeological surveys. The EA concluded that lease issuance would have no significant impacts to people or the environment.
TGS launches 3D survey in Brazil
TGS has launched the PAMA 3D Phase 1 Survey in the Equatorial Margin of Brazil. This survey covers an extensive area of 19,343 km2 and more than 25 future exploration blocks within the Pará-Maranhão Basin, one of the world’s largest major unexplored and highly prospective basins.
The PAMA 3D Phase 1 Survey expands TGS’ data coverage in this promising region, building on previous 3D surveys covering more than 60,000 km2. The Equatorial Margin has drawn industry attention due to prolific successes in neighbouring Guyana and Suriname. These efforts target reservoir quality sands within deepwater fan systems, sourced from Amazon River-related drainage, in a diverse range of plays, from stratigraphic pinch-outs at the paleo-slope edge to anticline structures within the fold-and-thrust belt extending along the margin.
Kristian Johansen, TGS CEO, said: ‘The Equatorial Margin represents one of the most exciting exploration frontiers today. In addition to developing and owning the survey, we will use our own high-end streamer vessels to acquire the data, as well
Meanwhile, BOEM has published a Call for Information and Nominations for a second regional offshore wind energy sale in the Central Atlantic, inviting public feedback on wind energy development 9ff the coasts of New Jersey, Delaware, Maryland, Virginia, and North Carolina.
The Central Atlantic 2 Call Area consists of 13,476,805 acres. BOEM will collaborate with the National Oceanic and Atmospheric Administration’s National Centres for Coastal Ocean Science to help identify where conflicts may exist and inform decisions regarding the most appropriate locations for WEAs.
as our own imaging capacity. Finally, the data will be delivered to customers through the TGS data management solution.’
Meanwhile, TGS has won a fourmonth OBN contract extension in the Gulf of Mexico.
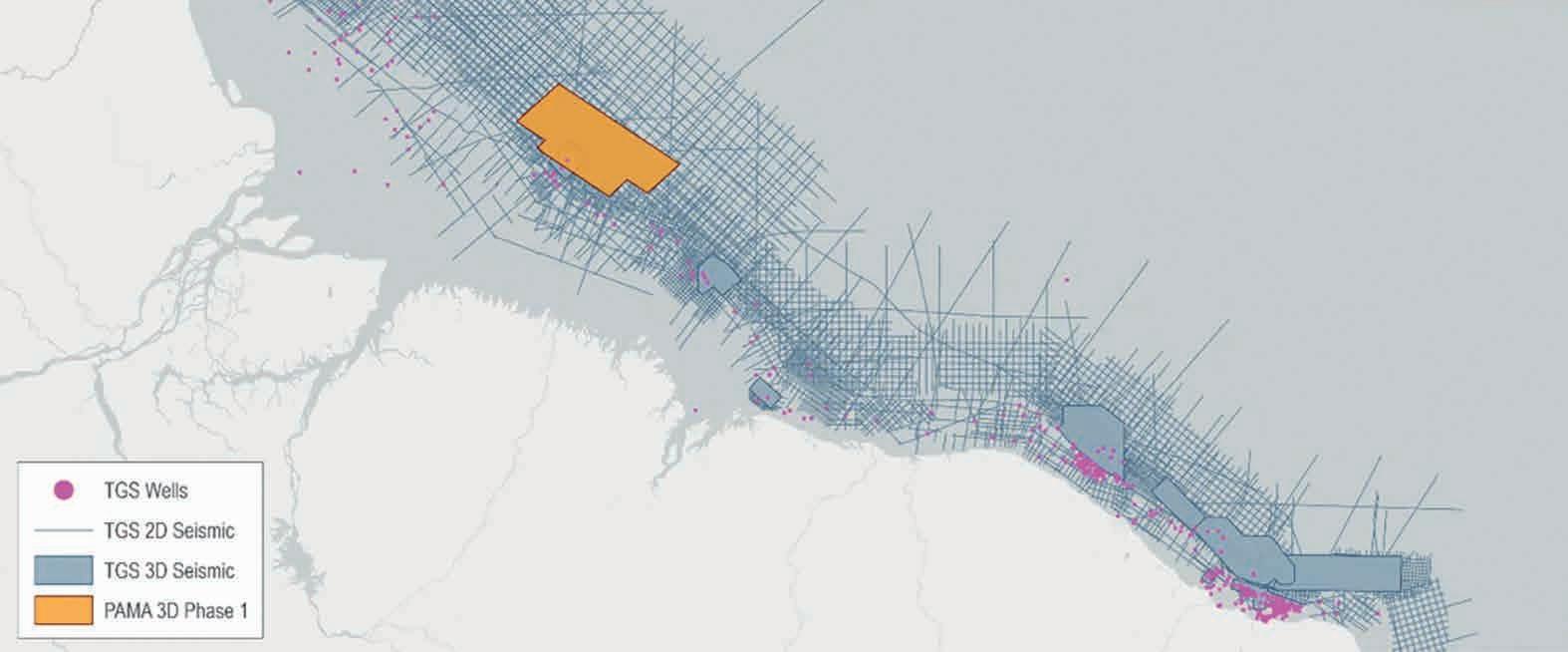
Sercel sells land seismic nodes to DMT
Sercel has sold 30,000 Sercel WiNG land seismic nodes to DMT. The engineering services and consultancy headquartered in Essen, Germany. DMT will deploy the WiNG nodes on a campaign of large-scale seismic surveys planned in urban areas to target energy resources, including geothermal.
Featuring the ultra-sensitive broadband digital MEMS (microelectromechanical systems) QuietSeis sensor, the Sercel
WiNG node delivers optimal data quality for outstanding subsurface imaging, Sercel claimed. With its field-proven Pathfinder transmission management technology, the the company’s crew can view and monitor the entire acquisition spread in real time, ensuring the most comprehensive and efficient quality control of operations, said Sercel..
Shearwater reports operating profit of $46 million
Shearwater GeoServices has reported second quarter operating profit of $46 million on revenues of $214 million, compared to operating profits of $36 million on revenues $238 million in Q2 2024.
Second quarter EBITA of $80 million was up from $66 million in Q2 2023.
Vessel utilisation of 83% from 10 active vessels compared to 83% in Q2 2024, when the company was operating 14 active vessels.
Highlights in the quarter include completion of an OBN survey in India, start up of operations in the North Sea and Canada, and a second carbon capture survey this year off the coast of the UK.
Irene Basili, CEO of Shearwater Geoservices, said: ‘Activity increased during the second quarter in line with seasonal demand. Although, so far in 2024, the marine seismic acquisition market has been slower than anticipated, we still see a positive development on EBITDA from the previous quarter and the 2nd quarter last year. A healthy pipeline of potential projects for the upcoming winter indicates increased
demand globally. However, uncertainty around timing of projects, related to permits and internal processes with our clients, could lead to projects which we earlier assumed would come in Q4 to slide into 2025.
‘Operationally, we have launched a project in a prosperous area in offshore Brazil, as well as our recently announced technology agreement with Petrobras. This month we also completed the first major OBN survey combining Shearwater’s in-house developed Pearl node and purpose-built ROV vessel SW Tasman. The survey was recently extended by the client in India continuing through Q3 and into Q4.
‘Our long-term view of the market remains positive on increased demand for our services. Recent projections by super major ExxonMobil in their ExxonMobil Global Outlook: our view to 2050, supports the strong need for more investments, in order to increase oil supply to fend off a projected natural decline of a staggering 15% annually in the period covered by the report.’
DUG launches elastic MP-FWI imaging solution
DUG Technology has launched DUG Elastic Multi-parameter Full Waveform Inversion (MP-FWI) Imaging, which it claims will offer a step change in imaging quality with its elastic rock properties for quantitative interpretation and pre-stack amplitude analysis — directly from field-data input.
Strong impedance contrasts (in particular those with high impedance
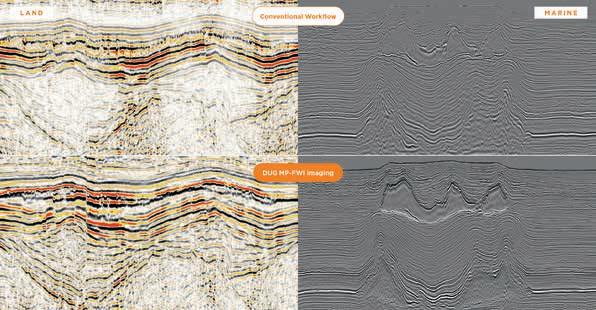
contracts produced by salt or chalk, for example) produce significant elastic effects that must be accounted for to correctly image the seismic wavefield and deliver true-amplitudes for quantitative interpretation, said DUG. Its latest elastic imaging technology solves for three-component reflectivity, Vp, Vs, P-impedance, S-impedance and density.
DUG’s managing director Matt Lamont said: ‘DUG’s foundations were built on both seismic data imaging and quantitative interpretation. Our new elastic MP-FWI imaging combines these disciplines into a single, elegant solution. The results are spectacular and we have no doubt this solution will be transformative for our clients.’
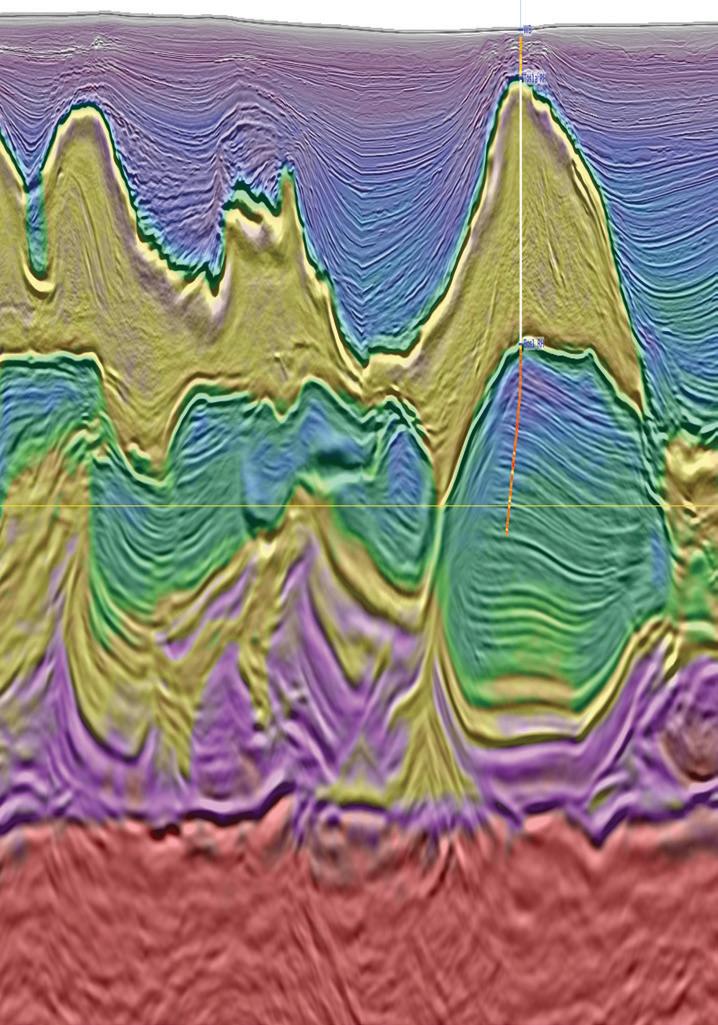
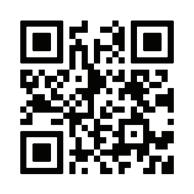
ENERGY TRANSITION BRIEFS
Norway has received applications from six companies for potential storage of CO2 in the North Sea. The Ministry of Energy is aiming to award exploration licences in the second half of 2024. By the deadline of 29 August, applications were received from Aker BP; Equinor; Harbour Energy; Horisont Energi; Storegga and TotalEnergies.
Petronas, Adnoc and Storegga have signed a joint study and development agreement to evaluate the carbon dioxide (CO2) emissions storage capabilities of saline aquifers and the construction of carbon capture and storage facilities in the Penyu basin, offshore Peninsular Malaysia. The agreement is targeting at least 5 million tonnes per annum (mtpa) of CO2 capture and storage capacity by 2030 and its scope includes a CO2 shipping and logistics study, geophysical and geomechanical modelling, reservoir simulation and containment research.
Equinor has won a lease in the US’ offshore wind energy lease auction in the US Central Atlantic region. The ~2 GW lease will have the capacity to produce enough energy to power approximately 900,000 US homes. With a bid of $75,001,001 for 101,443 acres in the Atlantic Ocean, Equinor secured one of two fixed-bottom lease areas on offer, located 26 nautical miles from the mouth of the Delaware Bay.
After the recent Nagoya CCS feasibility study, BP and Chubu Electric Power Co have expanded their collaboration to explore a CCS value chain from Port of Nagoya, Japan, to the Tangguh field in Teluk Bintuni, Papua Barat, Indonesia. Bp, as the operator of Tangguh production sharing contract, and Chubu Electric have signed an updated agreement to include evaluation on cost optimisation across the CCS value chain.
GeoMark Research, a geochemistry and PVT service provider, and Petricore, a global oil services company, have teamed up to offer an integrated suite of carbon capture and sequestration (CCS) services.
Crown Estate sets out vision for the UK’s seabed to accelerate energy transition
A vision for how the UK’s seabed can continue to support the accelerated delivery of transition to clean energy has been set out by the UK’s Crown Estate, which manages the seabed around England, Wales and Northern Ireland.
Amid increasing demand on the seabed from sectors critical to the UK economy, the Crown Estate’s Marine Delivery Routemap hopes to provide visibility and certainty for developers of offshore renewables, helping to address current pinch-points such as consenting and grid connections. The plan will also set out how to protect biodiversity and marine environments.
A Routemap was one of the key recommendations published in last year’s independent report from Electricity Networks Commissioner Nick Winser. It builds on the Crown Estate’s expertise in spatial mapping and digital capabilities, which received a global Esri award for geospatial innovation in July.
The Crown Estate has published an offshore wind and consultation alongside the Routemap. It is expected to be followed with reports on nature, carbon capture and other sectors.
The UK is a world-leader in offshore wind said the Crown Estate, with the current pipeline standing at approximately 95GW, including almost 15GW already operational. The newly formed UK government energy company Great British Energy and the Crown Estate recently announced a new partnership to bring to market an additional 20-30GW of offshore wind leasing opportunities by 2030.
The Crown Estate report Future of Offshore Wind sets out its approach to leasing this additional capacity for delivery by 2040. It is seeking input and feedback from industry and wider stakeholders to help shape its approach to seabed leasing.
Much of the new offshore wind capacity is expected to be in areas of the Celtic Sea, which lies off the coasts of South Wales and South West England, and North
East England. A number of smaller projects are also likely off the coasts of North Wales, North West England, Lincolnshire and Yorkshire.
The report sets out further details on how the Crown Estate could play a greater role in investing to support enabling infrastructure to allow the accelerated delivery of offshore wind projects. This follows the introduction of legislation in the British parliament in the past few months to modernise the Crown Estate’s borrowing and investment powers.
Gus Jaspert, managing director, marine at the Crown Estate, said: ‘Our evolving approach to offshore wind development is designed to help remove some of the barriers to deployment of important new renewable energy and provide more certainty to investors ’
RenewableUK’s chief executive Dan McGrail said: ‘Providing long-term visibility on the details of future offshore wind leasing rounds as early as possible will further increase confidence in the long-term stability of the UK’s world-leading offshore wind market, potentially leading to billions of pounds of additional private investment in the decades ahead.’
Fintan Slye, executive director of the Electricity Systems Operator (ESO), said: ‘We are already working closely with the Crown Estate, as demonstrated by our recent collaboration on the leasing round for floating offshore wind currently underway in the Celtic Sea.’
Dr Gemma Harper OBE, chief executive at Joint Nature Conservation Committee (JNCC), said: ‘We welcome the clear focus being placed on nature by the Crown Estate in the development of its Marine Delivery Routemap. The Routemap advances the critical discussion of how we value and integrate the marine environment with the many sectors with an interest in the seabed, ensuring its use is sustainable now and for future generations.’
TGS shoots 2D survey offshore Indonesia
TGS has started a 2D seismic survey in the Sumatra basin, Indonesia. The survey acquired by COSL’s HYSY 718 vessel, is expected to shoot between 5500- and 6500-line km, covering two regions.
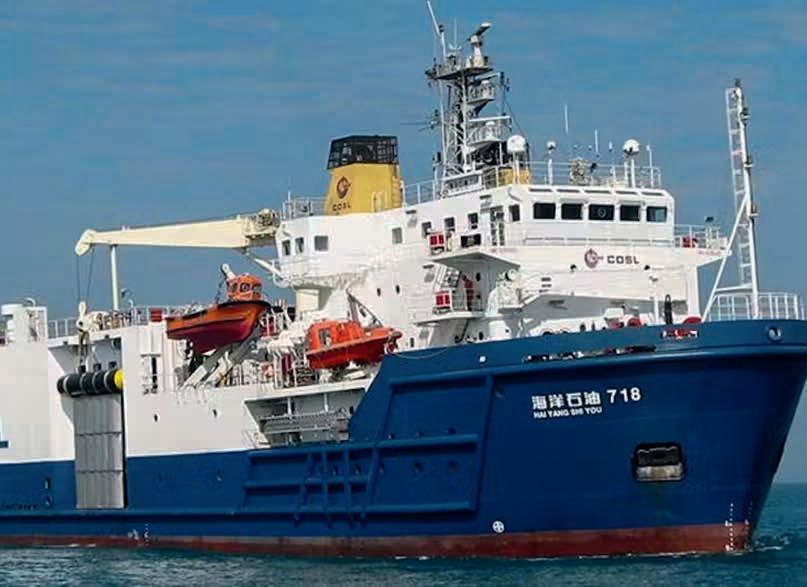
Earlier this year, TGS completed a multi-client 2D reprocessing project in the same basin which aims to integrate
key discoveries with available open acreage.
Kristian Johansen, TGS CEO, said, ‘North Sumatra has been the site of major discoveries in the past few years. With this being our sixth consecutive acquisition project offshore Indonesia, TGS remains dedicated to advancing exploration in the region. Our high-quality seismic data continues to shed light on crucial play concepts, extending exploration potential into open acreage and unlocking exciting opportunities within the Sumatra basin.’
The seismic acquisition is expected to be completed by the end of Q4 2024.
Meanwhile, TGS has signed a Memorandum of Understanding (MoU) with Petrobras to collaborate on scientific research and technological development activities in Brazil.
The collaboration will focus on developing new technologies to increase
efficiency and sustainability in oil and gas exploration and production. Additionally, it will drive innovation in renewable energy technologies and carbon capture solutions.
Finally, TGS has announced a strategic partnership with ComboCurve to enable seamless access to TGS’ licensed production, completions, and cost data via the ComboCurve platform, with fully automated data integration that requires minimal technical infrastructure.
‘The partnership streamlines access to TGS production data by integrating it directly into the ComboCurve platform through ComboCurve’s ComboSync product, eliminating the need for time-consuming downloads and formatting,’ said TGS.
‘By leveraging ComboCurve’s advanced forecasting and economic models, and TGS’s data quality, this partnership significantly enhances endto-end deal evaluations,’ the company added.




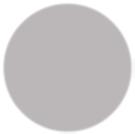
BRIEFS
Egypt has launched the country’s Egyptian International Bid Round 2024, which includes 12 open blocks for exploration in the Mediterranean and Nile Delta. EGAS said that companies can review and purchase datasets online through the EUG portal. For bid round enquiries, contact bidround@eug.petroleum.gov.eg.
TGS has entered the software solutions market with Imaging AnyWare – a proprietary enterprise processing system designed to enhance subsurface imaging and integration projects. Its modern architecture is complemented by geophysical algorithms, ensuring users experience seamless cloud transitions and enhanced operational efficiency, the company added. Wadii El Karkouri, EVP of Imaging and Technology at TGS. ‘This expansion addresses the evolving needs of our clients, offering a versatile tool.’
Hartshead has won 10 blocks, across six licences in the UK’s 33rd Licensing Round. Five licences, consisting of nine blocks, are situated in the Southern gas basin. Two licences, consisting of three blocks, are adjacent to the P2607 Licence. The gas field redevelopments and undeveloped gas fields amount to 1187 Bcf. Most of the blocks come with commitments to carry out seismic reprocessing.
A consortium of Brazil’s state-owned Petrobras and Shell have acquired 26 concession contracts in the deepwater Pelotas basin under the fourth Permanent Concession Offer Cycle. Petrobras will hold a 70% stake in the blocks as the operator while Shell will own a 30% stake. Petrobras has secured three other blocks in partnership with CNOOC and Shell. In this partnership Petrobras will own a 50% stake, CNOOC 20%, and Shell 30%. Forty four bllocks in the Pelotas Basin were awarded. Chevron secured 15 blocks.
APA Corporation has agreed to sell noncore producing properties in the Permian Basin to an undisclosed buyer for $950 million, The properties are located in the Central Basin Platform, Texas and New Mexico Shelf, and Northwest Shelf.
UK government plans to revise environmental guidance for oil and gas projects
The UK government is planing to revise environmental guidance for oil and gas companies ‘to provide stability for industry, support investment, protect jobs, deliver economic growth, and meet its climate obligations’.
The release stated that the guidance is necessary in light of a UK Supreme Court ruling that has implications for the assessment of new development consents. It highlighted that the ‘landmark Finch ruling requires regulators to consider the impact of burning oil and gas, scope 3 emissions, in the Environmental Impact Assessment for new projects’.
The release noted that the government is acting swiftly so that decisions on oil and gas development consents can be made. ‘Crucially, oil and gas production in the North Sea will be a key component of the UK energy landscape for decades to come as it transitions to our clean energy future,’ it added.
UK Minister for Energy Michael Shanks said, ‘We will consult at pace on new guidance that takes into account the Supreme Court’s ruling on environmental impact assessments, to enable the industry to plan.’
The release stated that the government will not challenge judicial reviews brought against development consent for the Jackdaw and Rosebank offshore oil and gas fields in the North Sea, noting that this decision ‘will save the taxpayer money’. It highlighted that this litigation does not mean the licences for Jackdaw and Rosebank have been withdrawn.
The release also noted that the government will consult later this year on the implementation of its manifesto position not to issue new oil and gas licences to explore new fields. It said the government will aim to conclude its consultation by spring 2025.
Industry body Offshore Energies UK (OEUK) said: ‘Regulatory uncertainty further impacts investor confidence, and we urge the government to proceed at pace with updates to the relevant guidelines in light of the Finch ruling.’
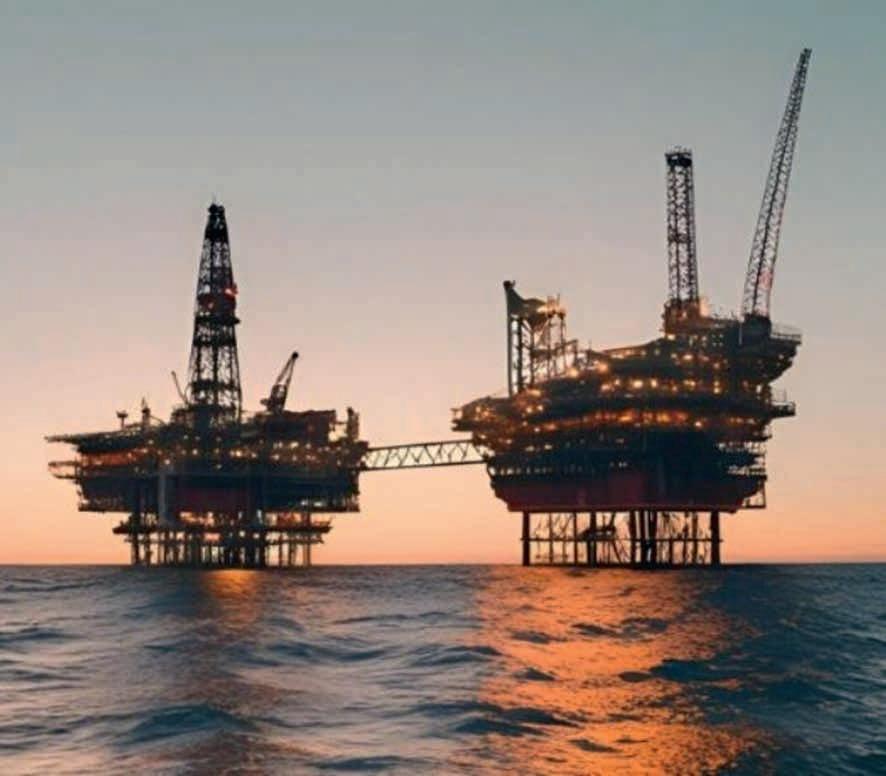
OEUK also noted that the Labour government ‘made significant manifesto commitments not to revoke existing licences and to manage existing fields for the entirety of their lifespan’, adding that the UK uses oil and gas for 75% of its energy needs including electricity, heating, and fuel.
‘Our members are the same UK companies which will produce the energy we need in future and we are committed to a homegrown energy transition which delivers the wind, hydrogen, and carbon capture, as well as the oil and gas, the UK will need during this time.’
Meanwhile, OEUK has published a fiscal assessment showing that the UK government’s proposals to toughen the fiscal regime on oil and gas companies is impacting investment. Data revealed the removal of capital allowances will ultimately result in a £12 billion loss in tax receipts due to a rapid decline in production due to under-investment over this decade.
OEUK chief executive David Whitehouse said: ‘I fully support the build out of renewable energy at pace as vital to net zero. But today 24 million homes are heated with gas. We ignore the need for domestic gas production at our peril – you do not protect consumers or tackle climate change by importing energy at the expense of homegrown production.’
Offshore wind sector expanded in 2022 despite challenges, says Rystad Energy
Global offshore wind projects have increased by 7% in 2023 despite inflationary pressures and supply chain disruptions, exemplified by postponed permitting processes and delayed auctions, according to Rystad research.
The sector is expected to grow by 9% in 2024 to more than 11 gigawatts (GW) by the end of the year. Rystad estimates that global installations, excluding mainland China, will exceed 520 GW by 2040.
Europe will play a crucial role in this growth, relying heavily on floating wind to meet ambitious national targets. By 2040, the continent is expected to account for more than 70% of global floating wind installations. Although some project delays beyond 2030 are anticipated, there will likely be a strong push to accelerate deployment. As a result, floating wind capacity is projected to approach 90 GW by 2040, with the UK, France and Portugal at the forefront of development. Asia will also be key in advancing floating wind as a mature technology, and the region – excluding mainland China – is expected to capture a share of 20% of global installations by 2040.
While the floating wind sector has been boosted by a recent rise in project announcements, it currently grapples with supply chain constraints similar to the bottom-fixed segment, where wind turbines are installed on fixed foundations in shallow waters. These challenges could hinder the advancement of floating wind technology in the short term, with capaci-
ty estimates of less than 7GW by 2030. To overcome these hurdles, increased government support is crucial, said Rystad.
‘The global offshore wind sector is experiencing robust growth, fuelled by increased investment and auction activity. However, supply chain bottlenecks present significant challenges to the industry’s further expansion. While ambitious targets boost investor confidence, it is crucial to address logistical issues to ensure that offshore wind can successfully take a key role in the energy transition,’ said Petra Manuel, senior offshore wind analyst, Rystad Energy.
In the bottom-fixed market, the UK, Germany and the Netherlands are expected to emerge as the three dominant players. The three countries are projected to account for 150GW of installed capacity by 2040, followed by the US with less than 40GW.
Between 2025 and 2030, the Americas, led by the US, will experience significant growth, with close to 2GW of installed capacity in 2025. Asia, excluding mainland China, will follow, with 7GW in 2025 and reaching nearly 28GW by 2030, with Taiwan (China), South Korea and Vietnam emerging as big markets in the region. Europe is projected to have 41GW of installed capacity by 2025 and more than 112GW by 2030.
Looking ahead to between 2030 and 2035, an increase in growth is anticipated in Asia, excluding mainland China, followed by the Americas and Europe.
Liberia launches licensing round
Liberia has launched a direct negotiation licensing for 29 offshore blocks in the Liberia and Harper Basins The Liberian offshore sector presents diverse geological plays, ranging from the synrift Lower Cretaceous to the deepwater Upper Cretaceous, with multiple source rock intervals throughout the stratigraphy.
TGS is offering multi-client subsurface data for the available blocks, including more than 24,000 km of 2D seismic data and more than 26,000 km2 of 3D seismic data. This data set includes
During this period, Latin America, particularly Brazil and Colombia, is also expected to begin contributing to offshore wind capacity in the Americas.
From 2025 to 2030, Rystad anticipates that only Asia and Europe will be actively installing floating wind capacity. By 2030, it expects Europe to have installed almost 5 GW of floating wind, while Asia, excluding mainland China, is projected to add 2GW. From 2030 to 2035, it foresees a substantial ramp-up in installations. Europe is expected to add 20GW of floating wind capacity, and Asia, excluding mainland China, up to 5GW. It does not expect floating wind projects to be installed in other regions until the period of 2035 to 2040. By 2040, Rystad predicts that Europe will have installed more than 65GW of floating wind capacity, while installations in Asia, excluding mainland China, will have reached 17GW.
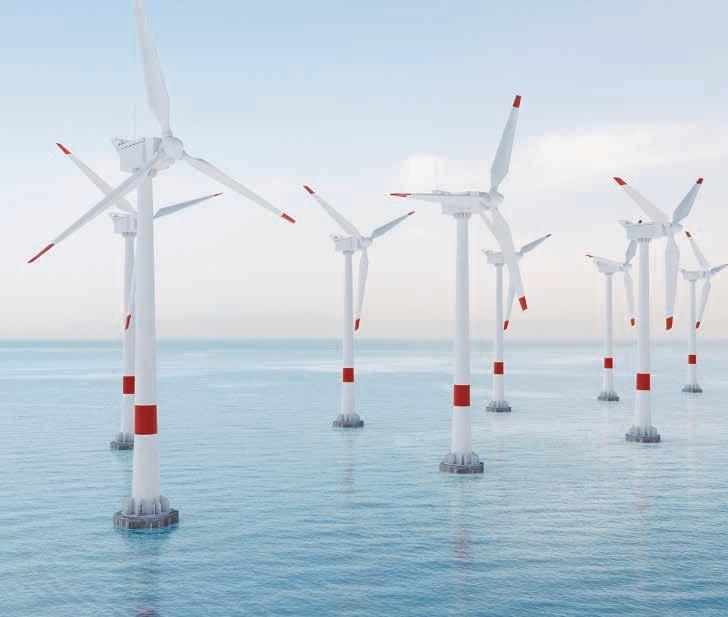
5100 km2 of newly reprocessed 3D seismic data and 12,000 km2 of 2D seismic data, both enhanced using advanced Pre-Stack Depth Migration (PSDM) technology. Gravity, magnetic data, and well data is also offered to enhance understanding of numerous prospects and identifiable leads.
This reprocessing method delivers enhanced imaging of key targets in the Cretaceous reservoirs, providing the latest insights into the region’s potential.
Denmark’s first carbon storage project completes pilot phase
Denmark has begun storage for CO2 in the North Sea subsoil after the 23 partners behind Project Greensand submitted the final report from the pilot project.
Independent technical verification carried out by DNV shows that the stored CO2 remains safely and permanently in the closed Nini West reservoir 1800 m below the North Sea seabed, as expected.
‘We now have documentation that we have a well-functioning storage for CO2 in the North Sea subsoil. We can see that the stored CO2 behaves as expected in the reservoir 1800 m below the seabed. That
confidence gives us a solid foundation to take the next steps that will be crucial for CCS in Denmark’, said Mads Gade, country manager at INEOS Denmark, the leading partner behind Project Greensand.
Project Greensand has also demonstrated that captured CO2 can be transported across borders and stored offshore to mitigate climate change. ‘We are the first in the world to succeed in developing, testing and demonstrating a well-functioning value chain for safe and efficient capture, transport and storage of CO2 across national borders,’ added Gade.
Oil and gas round-up
Equinor has proven gas/condensate in development well 6406/2-L-2 H, 260 km southwest of Brønnøysund in the Norwegian Sea. The well is close to the Lavrans discovery. The discovery is estimated to be in the range of 2-4 million Sm3 of recoverable oil equivalent. The licensees will consider tying the discovery back to infrastructure being developed for Lavrans – which is part of the Kristin field. The objective of the well was to prove petroleum in Lower Jurassic sandstones in the lower part of the Tilje Formation. Well 6406/2-L-2 H encountered a gas/condensate column of about 30 m in the lower parts of the Tilje Formation, with moderate-to-good reservoir properties. Gas/ condensate was also proven in the upper parts of the Tilje Formation in a sandstone reservoir with moderate-to-poor reservoir properties. The well was drilled to vertical depths of 6075 and 5045 m below sea level, and was terminated in the Åre Formation in the Lower Jurassic.
CNOOC has drilled a natural gas well in the ultra-deepwater Liwan 4-1 structure in the Pearl River Mouth Basin in the South China Sea. The well was tested to produce 430,000 m3 per day of absolute open flow natural gas, marking the first major exploration breakthrough in ultra-deepwater carbonate rocks offshore China. The well is located in Baiyun Sag, the largest
hydrocarbon-rich sag in the Pearl River Mouth Basin, about 300 km southeast of Shenzhen with a water depth of nearly 1640 m. The well was drilled to a vertical depth of nearly 3000 m, completed at a depth of nearly 4400 m, and encountered gas pay zone of approx. 650 m in the horizontal section. The well has revealed promising exploration prospects in the ultra-deepwater Globigerinid limestone in China.
The Joint Venture of Sinjhoro Block –comprising Oil & Gas Development Company Limited (OGDCL) as operator (76%), Orient Petroleum (19%) and GHPL (5%) – has made a gas condensate discovery at the Baloch-2 well in the exploratory zone of Sembar Formation, in Baloch Development and Production Lease (D&PL) located in District Sanghar, Sindh Province, Pakistan. Baloch-2 well was drilled to total depth of 3920 m TVD in the Sembar Formation. Based on the results of wireline logs interpretation, there is estimated to be 6.8 Million Standard Cubic Feet per Day of gas and 388 Barrels of Condensate per Day.
Eni has won approval for its plan of development (POD) of the Geng North (North Ganal PSC) and Gehem (Rapak PSC) gas fields. The integrated development will create a new production hub, called Northern Hub, in the Kutei Basin. Eni has also
INEOS and licence partners Wintershall Dea (now Harbour Energy) and Nordsøfonden have applied for final approval for Denmark’s first permanent large-scale CO2 storage facility in the North Sea by the end of 2025 or the beginning of 2026. Up to 400,000 tonnes of CO2 would be stored per year, while the plan is to store up to 8 million tonnes of CO2 per year in the area under the North Sea’s seabed from 2030.
Work is also underway to investigate whether it is possible and safe to store CO2 underground on land in Denmark.
won approval for the POD for Gendalo and Gandang fields (Ganal PSC). Additionally, Eni has been awarded a 20-year extension of the IDD licences named Ganal and Rapak. The Northern Hub POD envisages the development of the 5 TCF gas and 400 million barrels of condensates of the Geng North discovery announced by Eni in October 2023, along with the 1.6 TCF of the nearby Gehem discovery. Eni is also planning to conduct a drilling campaign in the next 4-5 years to assess the significant near-field exploration potential within the Eni-operated blocks in the Kutei Basin, amounting to more than 30 TCF of gas.
Gran Tierra Energy has discovered oil at the Charapa Block in Ecuador, just west of the recently discovered Arawana-J1 and Bocachico Norte-J1 wells. The Charapa-86 well is Gran Tierra’s third oil discovery in 2024 and fifth in Ecuador.
Melbana Energy has approved development of Unit 1B onshore Cuba, which includes two additional production wells using existing 2D seismic control targeting the highest confidence 1C resource of 16 million barrels. A 3D seismic acquisition planned for next year will allow subsequent development wells. The entire recoverable resource is estimated at 129 million boe. Melbana Energy is the operator of Block 9 PSC with a 30% interest.
Special Topic
ENERGY TRANSITION
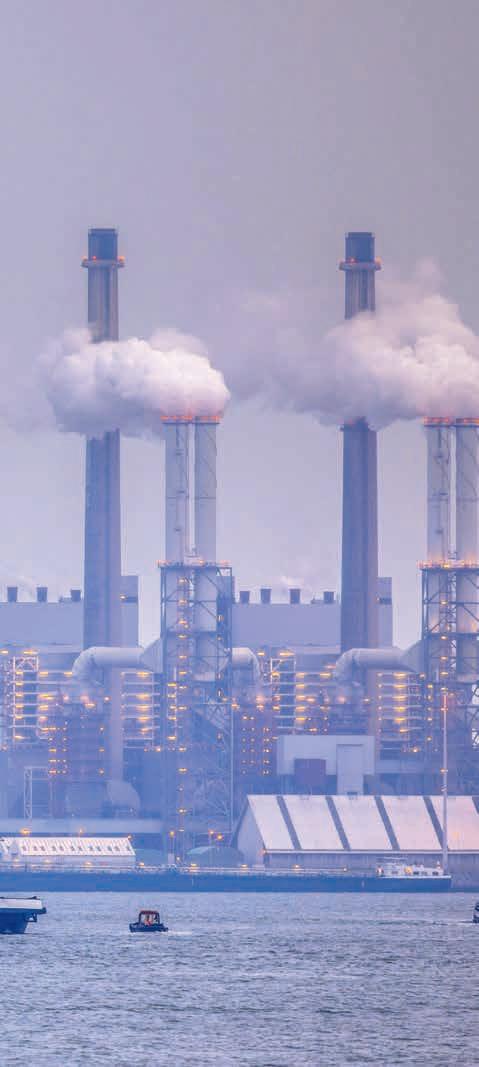
Submit an article
This month we showcase the critical role that geoscientists are playing in developing innovations to ease the energy transition.
Gregor Duval et al present a method for the quantitative assessment of the subsurface suitability of saline aquifer CO2 storage sites using a case study from the Northern North Sea.
Roya Dehghan-Niri et al present the results from a series of 2D and 3D ministreamer operations across the Sleipner CO2 storage site, which are assessed and compared with conventional streamer seismic.
Paul Helps et al discuss CO2 mineralisation within mafic and ultramafic rocks, where carbon is incorporated into the structure of the rock through the crystallisation of new, stable carbonate minerals, potentially offering the means to store CO2 safely, rapidly and permanently in large quantities.
Kim Gunn Maver et al demonstrate that with closed-loop solutions it is possible to significantly impact the decarbonisation of district heating, district cooling, industrial heating requirements, and electricity production moving towards ‘zero’ CO2 emissions with a nearly unlimited resource of heat from the earth’s interior that is both reliable and cost effective.
Philip Ringrose et al outline the critical role that geoscientists can play in advancing projects and communicating the risks and benefits of emerging projects to society.
Rasoul Sorkhabi et al highlight recent advancements and key features within Utah’s new energy corridor, showcasing the state’s progress toward sustainable energy resources.
Sougata Halder et al present a novel workflow for developing a basin-scale stratigraphic architecture for defining the major saline reservoirs and sealing units within a basin.
First Break Special Topics are covered by a mix of original articles dealing with case studies and the latest technology. Contributions to a Special Topic in First Break can be sent directly to the editorial office (firstbreak@eage.org). Submissions will be considered for publication by the editor.
It is also possible to submit a Technical Article to First Break. Technical Articles are subject to a peer review process and should be submitted via EAGE’s ScholarOne website: http://mc.manuscriptcentral.com/fb
You can find the First Break author guidelines online at www.firstbreak.org/guidelines.
Special Topic overview
January Land Seismic
February Digitalization / Machine Learning
March Reservoir Monitoring
April Underground Storage and Passive Seismic
May Global Exploration
June Technology and Talent for a Secure and Sustainable Energy Future
July Modelling / Interpretation
August Near Surface Geo & Mining
September Reservoir Engineering & Geoscience
October Energy Transition
November Marine Acquisition
December Data Management and Processing
More Special Topics may be added during the course of the year.
CALENDAR OF EVENTS
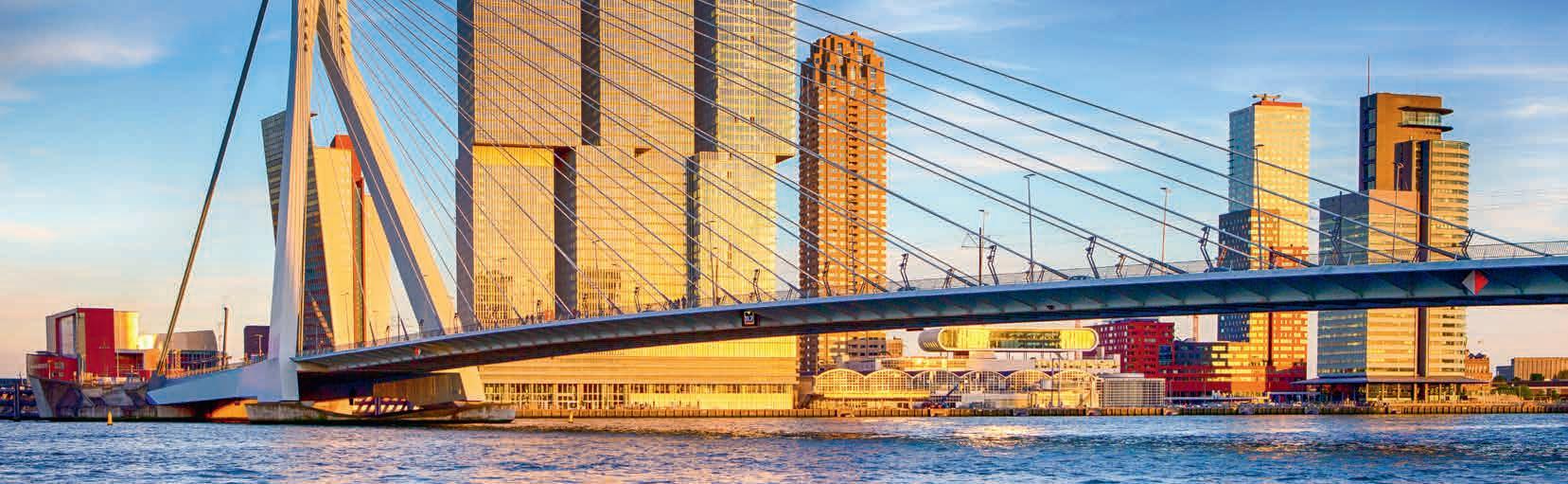
1-4 Oct 18 th SAGA Biennial Conference & Exhibition www.sagaconference.co.za
3-4 Oct Third EAGE Workshop on EOR www.eage.org
3-5 Oct 59 th CEEC Event www.ceecsg.org
6-8 Oct EAGE Workshop on Naturally Fractured Rocks (NFR) www.eage.org
7-9 Oct GeoTerrace 2024 - International Conference of Young Professionals www.eage.org
14-16 Oct Third EAGE Conference on Seismic Inversion www.seismicinversion.org
15-16 Oct EAGE Conference on Energy Excellence: Digital Twins and Predictive Analytics www.eage.org
16-17 Oct Third EAGE Workshop on Advanced Seismic Solutions in the Gulf of Mexico www.eage.org
21-24 Oct GEO 4.0: Digitalization in Geoscience Symposium www.geo4event.com
29-30 Oct EAGE Workshop on Borehole Technologies - Pioneering Sustainable Solutions in Energy www.eage.org
November 2024
3-7 Nov 15 th General Assembly of Asian Seismological Commission www.asc2024.org
4-7 Nov Fifth EAGE Global Energy Transition Conference and Exhibition www.eageget.org
EAGE Carbon Capture and Storage Conference
Part of GET 2024 (Fifth EAGE Global Energy Transition Conference and Exhibition)
EAGE Geothermal Energy Conference
Part of GET 2024 (Fifth EAGE Global Energy Transition Conference and Exhibition)
EAGE Hydrogen and Energy Storage Conference
Part of GET 2024 (Fifth EAGE Global Energy Transition Conference and Exhibition)
EAGE Offshore Wind Energy Conference
Part of GET 2024 (Fifth EAGE Global Energy Transition Conference and Exhibition)
6-8 Nov First EAGE Conference on Energy Opportunities in the Caribbean www.eage.org Port of Spain Trinidad & Tobago
12-13 Nov 2 nd EAGE Workshop on Integrated Subsurface Characterization and Modeling www.eage.org
Kuala Lumpur Malaysia
14-16 Nov GEOSCIENCE International Symposium www.geosymposium.org Bucharest Romania
20-21 Nov Asia Petroleum Geoscience Conference and Exhibition (APGCE) icep.com.my/apgce
25-27 Nov First EAGE/SBGf Conference on The Roadmap to Low Carbon Emissions in Brazil www.eage.org
December 2024
3-5 Dec First EAGE Symposium on Geosciences for New Energies in America www.eagenewenergies.org
EAGE Workshop on Near Surface Geoscience & Mineral Exploration in Latin America Part of First EAGE Symposium on Geosciences for New Energies in America
EAGE Workshop on Geothermal Energy in Latin America
Part of First EAGE Symposium on Geosciences for New Energies in America
EAGE Workshop on Water Footprint
Part of First EAGE Symposium on Geosciences for New Energies in America
3-5 Dec 3 rd SEG/EAGE Workshop on Geophysical Aspects of Smart Cities www.eage.org
February 2025
3-4 Feb EAGE Workshop on Carbon Capture and Storage (CCS) in Basalts www.eage.org
Kuala Lumpur Malaysia
17-19 Feb EGYPES 2025 www.egypes.com Cairo Egypt
18-20 Feb International Petroleum Technology Conference (IPTC) 2025 www.iptcnet.org Kuala Lumpur Malaysia
20-21 Feb GeoTHERM Expo & Congress 2025 www.geotherm-offenburg.de/en Offenburg Germany
27-28 Feb First EAGE Workshop on the Triassic and Jurassic Plays in Northwest Europe www.eage.org Sunbury United Kingdom
March 2025
19-21 Mar 2 nd AAPG/EAGE Papua New Guinea Petroleum Geoscience Conference & Exhibition
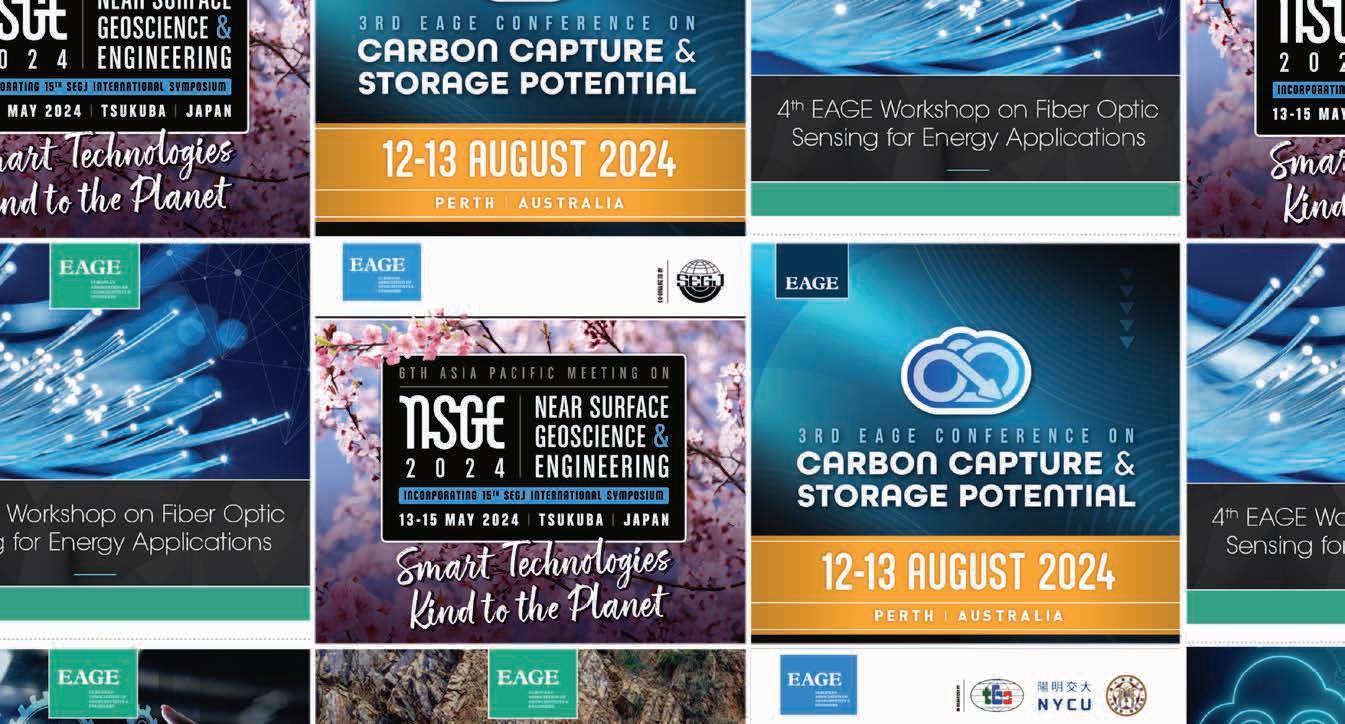
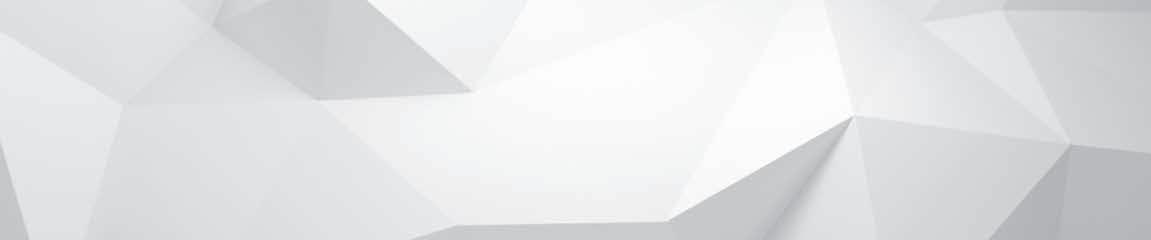
CALENDAR OF EVENTS
The Middle East Oil, Gas and Geosciences Show (MEOS GEO) www.meos-geo.com
22-24 Sep Sixth EAGE Borehole Geology Workshop www.eage.org
23-24 Sep EAGE Workshop on Optimizing Upstream Excellence for Oil and Gas Success www.eage.org
23-24 Sep First EAGE Workshop on Geophysical Techniques for Monitoring CO2 Storage www.eage.org
29 Sep1 Oct Second AAPG/ EAGE Mediterranean and North African Conference (MEDiNA) medinace.aapg.org
29 Sep1 Oct Eighth EAGE Borehole Geophysics Workshop www.eage.org
October 2025 Oct Ninth EAGE High Performance Computing Workshop www.eage.org
8-9 Oct First EAGE Workshop on Fiber Optics Sensing www.eage.org
21-23 Oct Second EAGE Conference on Offshore Energy Resources in the South Atlantic www.eage.org
November 2025
3-5 Nov EAGE/AAPG Workshop on Tectonostratigraphy of the Arabian Plate: Structural Evolution of the Arabian Basins www.eage.org
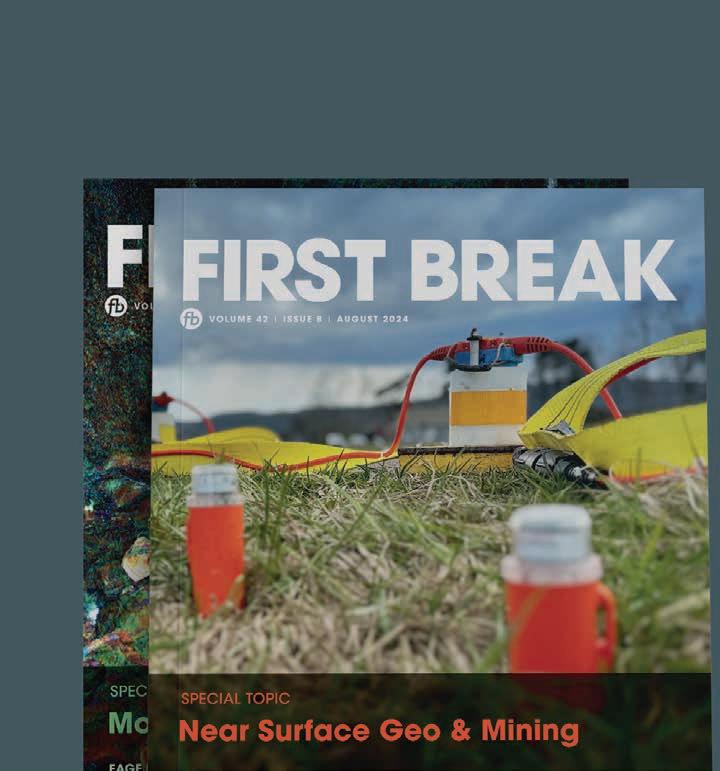
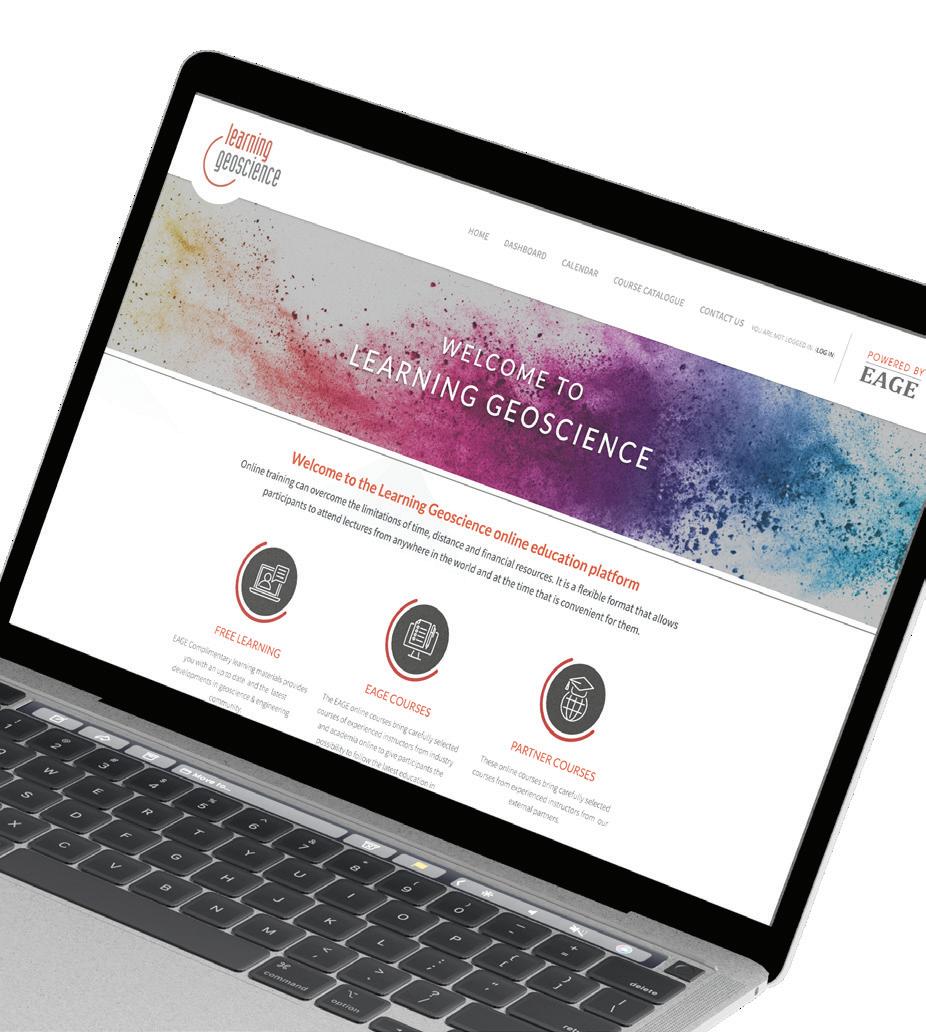
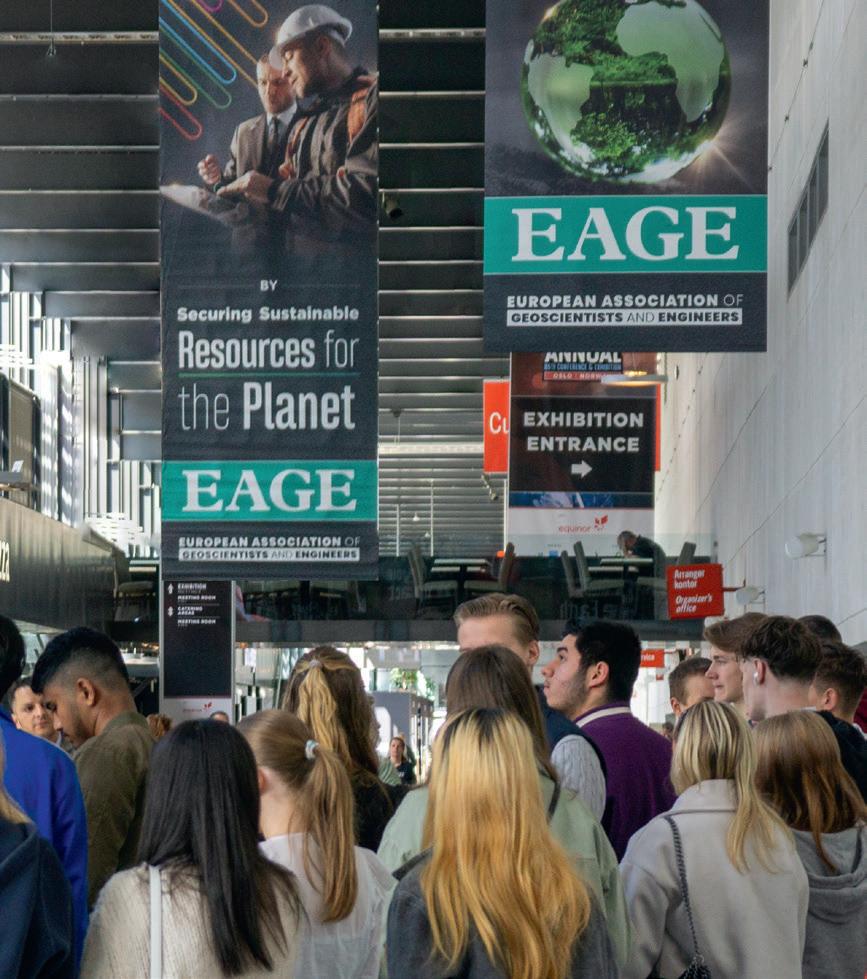
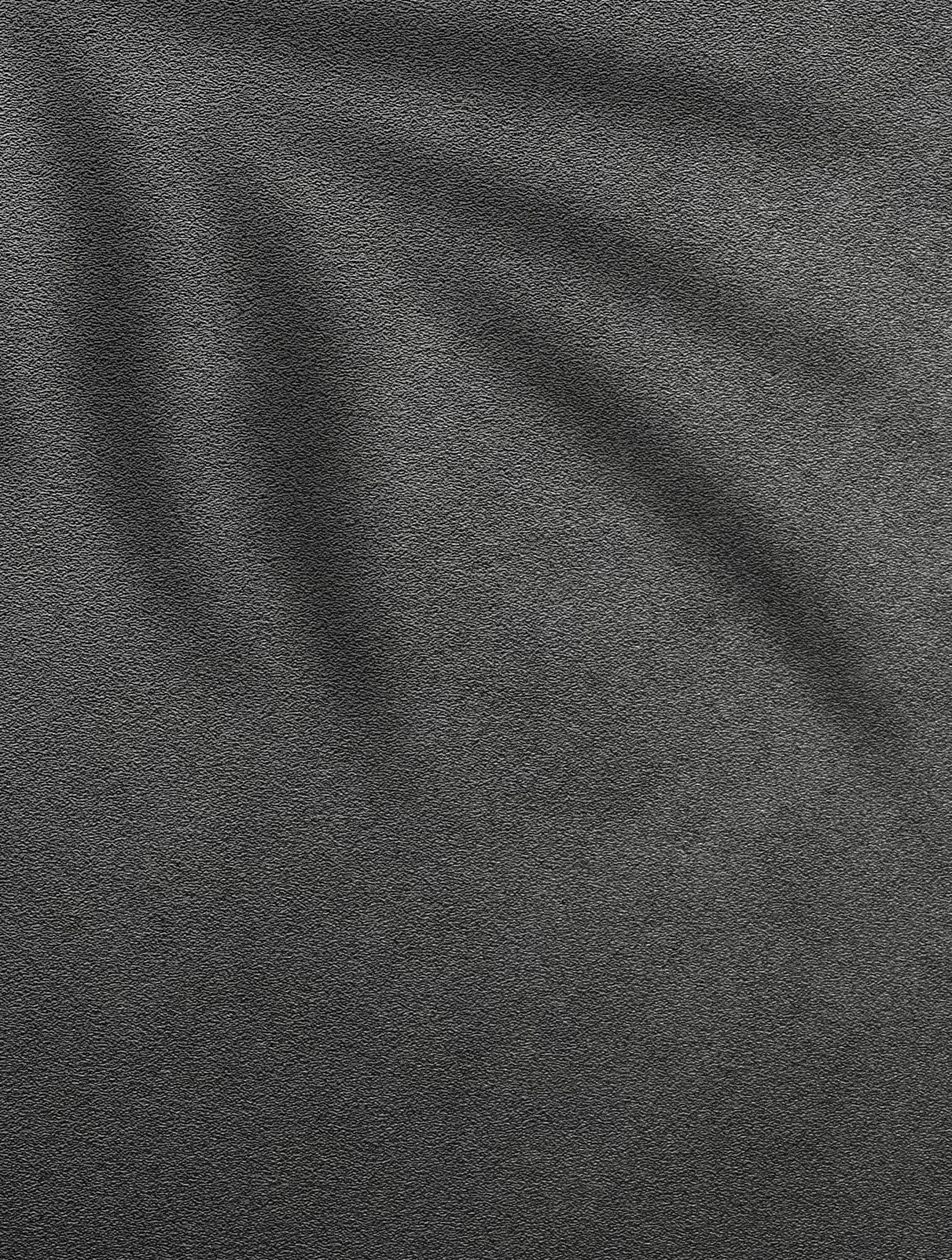
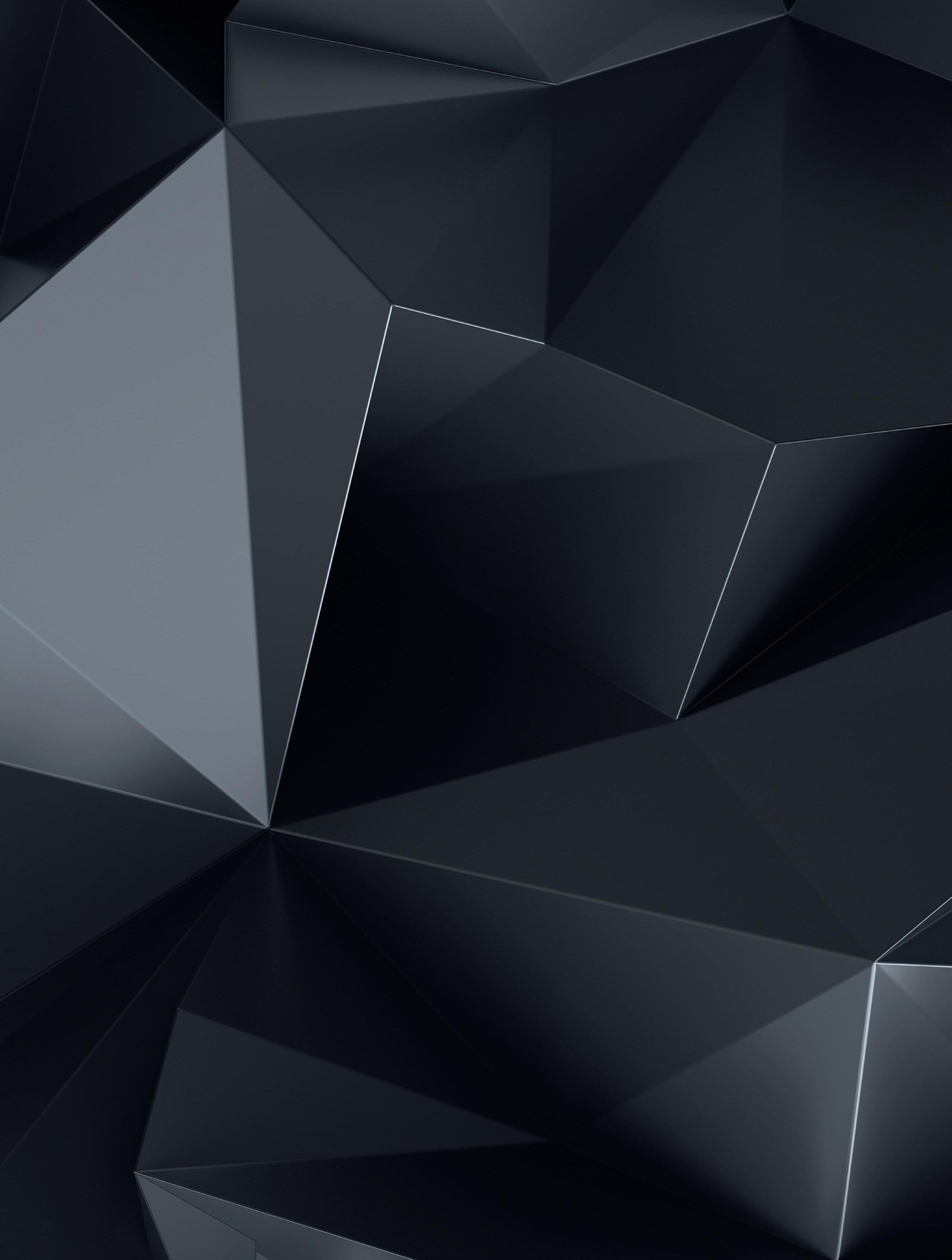
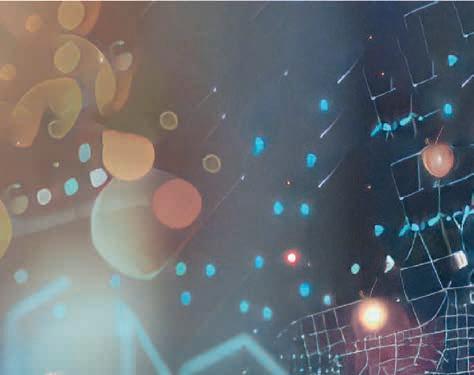
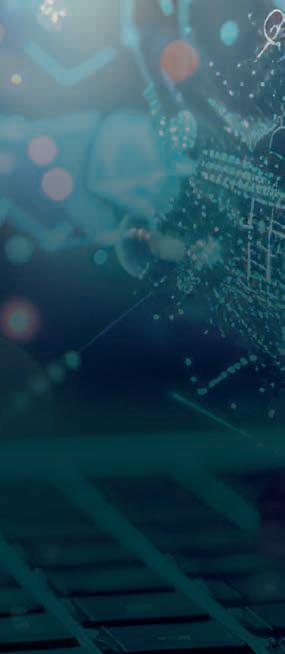

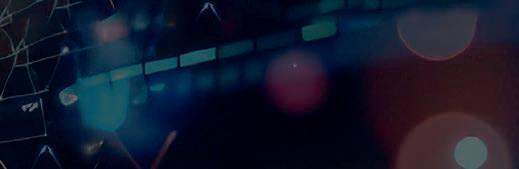
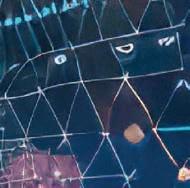
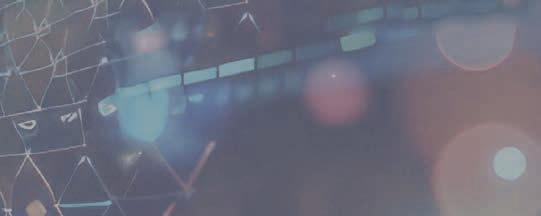


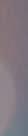
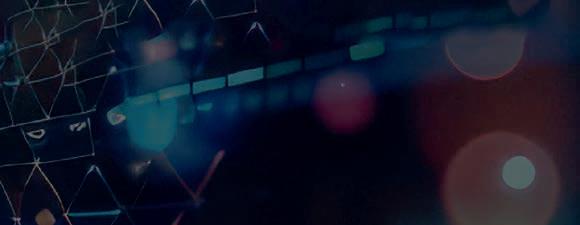
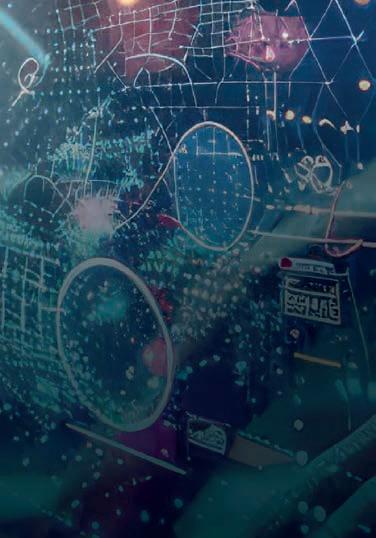


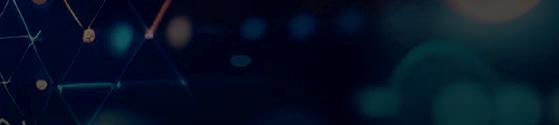
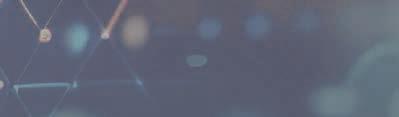
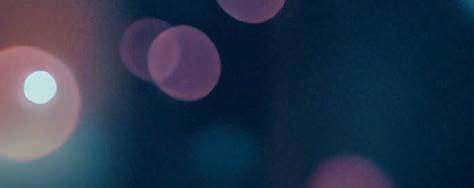
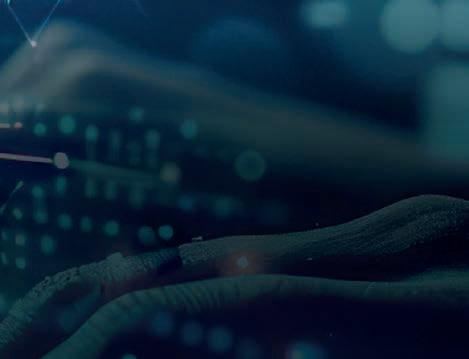
CONFERENCE AND EXHIBITION


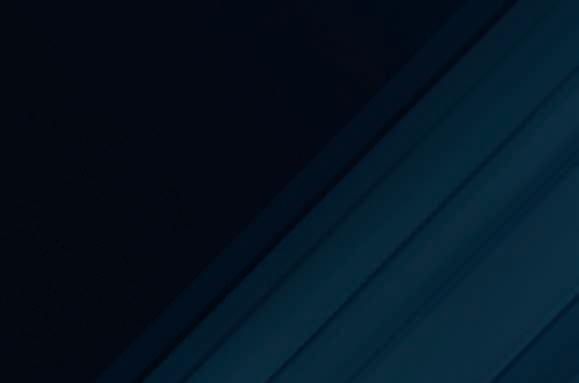
CHECK
DATA MANAGEMENT
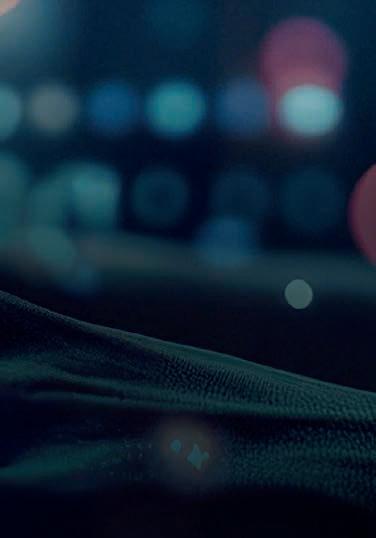
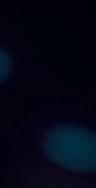
ENHANCING PREDICTIONS & INVESTMENTS WITH DIGITAL TECHNOLOGIES
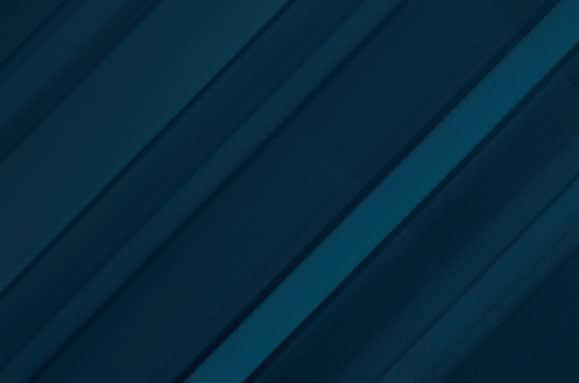


BUSINESS PROCESSES AND OBJECTIVES
MODELS, ALGORITHMS & NEW DEVELOPMENTS
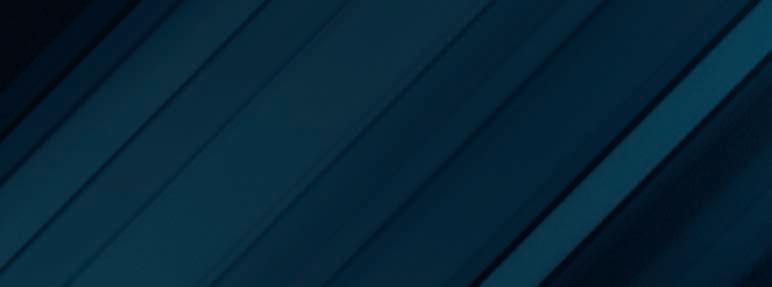
ADVANCED TECHNOLOGIES
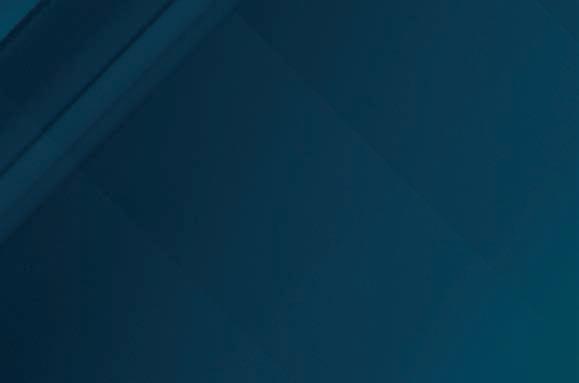
SUSTAINABILITY AND NEW ENERGY APPLICATIONS
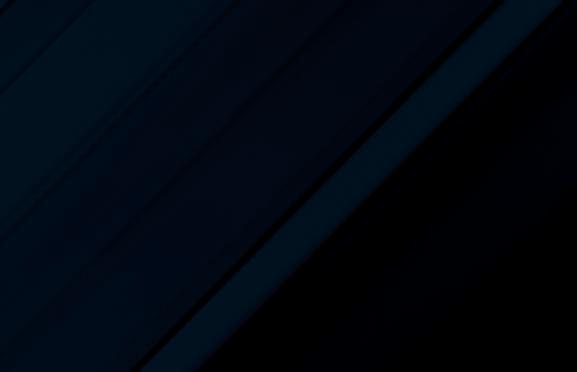
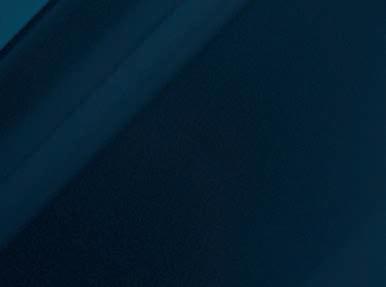
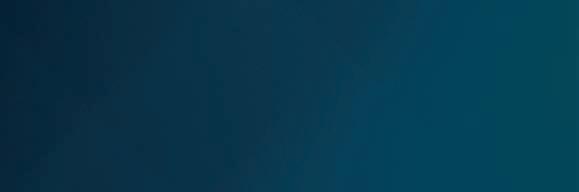
SUBMIT YOUR ABSTRACT
SUBMISSION DEADLINE: 24 DECEMBER 2024
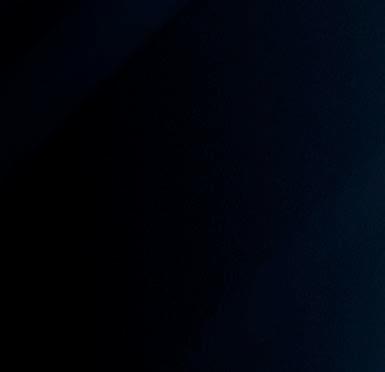
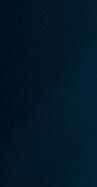


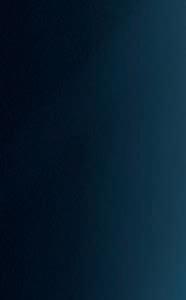


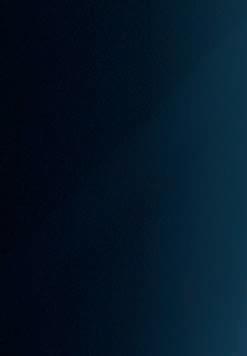




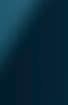



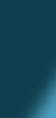
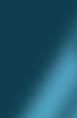
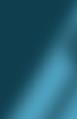


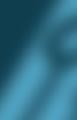

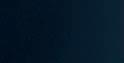
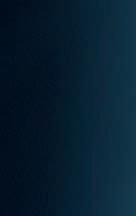

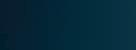
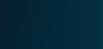






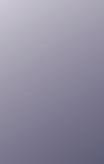
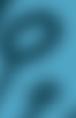
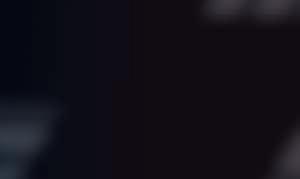



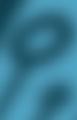


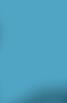





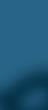



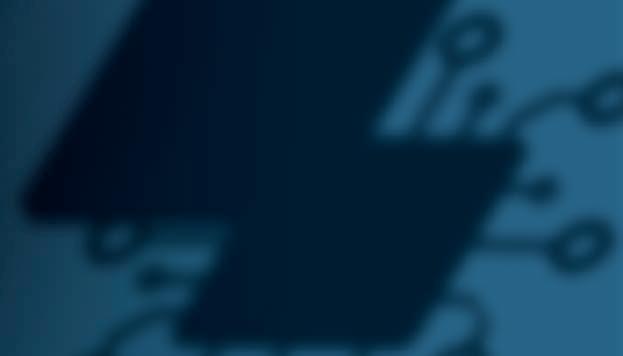
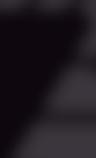














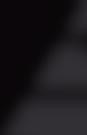
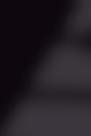







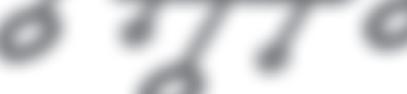
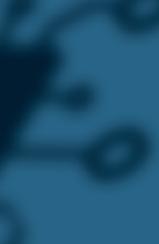
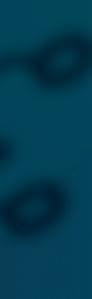
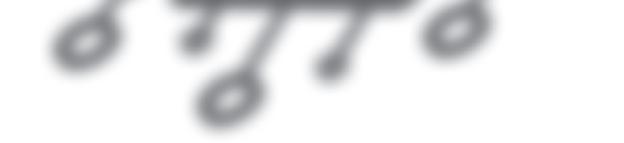