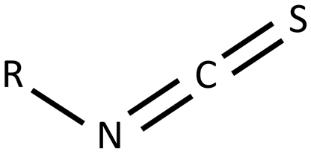
18 minute read
Antibacterial activity and degradation pathways of methallyl isothiocyanate
Ollie Bacon
Barker College
Methallyl isothiocyanate is an analogue of the well-researched antibacterial agent allyl isothiocyanate, found in plants belonging to the Brassicaceae family. Candidacy of methallyl isothiocyanate as an alternative antibacterial agent was investigated. The antibacterial activity of methallyl isothiocyanate was determined by testing multiple concentrations of the compound against K-12 E. coli achieved through serial dilutions. The compound was found to not display activity at the experimental concentrations. Multiple explanations for this are provided including an insufficient range of concentrations used for testing and structural differences between methallyl isothiocyanate and allyl isothiocyanate. Chemical degradation pathways of methallyl isothiocyanate are proposed based on literature inquiring into the decomposition of allyl isothiocyanate in water.
Literature review
Between 2001 and 2013, 107 bacterial toxin-mediated outbreaks were confirmed in Australia, affecting 2,219 people with 47 hospitalisations and 13 deaths occurring (May, Polkinghorne & Fearnley, 2016). The true number of outbreaks is likely higher due to underreporting which is common for bacterial infections. From this incident, 48% of outbreaks resulted from commercial food services inadequately controlling the temperature of environments where food was stored and handled (May, Polkinghorne & Fearnley, 2016), making conditions favourable for bacterial growth. Bacterial infections which are contracted by humans from eating or handling contaminated food are treated with the use of antibiotics. Over time, bacterial strains can develop favourable DNA mutations which render currently used antibiotic drugs ineffective, demonstrating the natural process of antibiotic resistance. The misuse of antibiotics through unnecessary or incorrect consumption is causing the rapid development of antibiotic resistance, thus reducing the ability to treat common bacterial infections (World Health Organisation, 2020). This will likelylead to a significant increase in global deaths if new antibacterial agents are not developed. Antibiotic resistance is therefore one of the largest threats to global health and as such, more effective methods of treating bacterial infections must be explored (World Health Organisation, 2020). One cause of bacterial infection is Escherichia coli (E. coli) which presents a significant danger to public health despite certain strains being used in laboratories. This is due to the wealth of knowledge surrounding this organism, the ability to be easily genetically modified and being considered as biologically safe vehicles for propagation of various genes and vectors (Kuhnert, Nicolet & Frey, 1995). In 2011, Shiga toxin-producing E. coli strains (STEC) were the cause of an outbreak in Germany in which 3,842 cases of human infections were recorded (Beutin & Martin, 2012) with person to person and foodborne transmission being the most effective mode of pathogen spread. Other unrelated STEC outbreaks highlight the ability of a wide variety of wild, domestic and captive animals to act as reservoirs for zoonotic pathogens (Kim, Lee & Kim, 2020).
Figure 1:General chemical structure of isothiocyanate functional group. R represents the glucosinolate side chain which varies between each ITCs.
As technological advances and the trend of mass production for maximised profit continue, human activities which result in the contamination of water sources and animal products will require new methods to mitigate the effects of these pathogens (Kim, Lee & Kim, 2020). One such method will include the development of novel antibacterial agents (Munita & Arias, 2016) in order to combat a future with increased antibiotic resistance.
There are many chemical compounds that display antibacterial properties however the commercial potential for each compound’s application depends on a variety of factors such as toxicity to humans, cost, effectiveness and environmental impact (Nowicki et al., 2016). Plausible candidates may be found in the isothiocyanate group (Figure 1). An isothiocyanate (ITC) is a natural plant product found in vegetables belonging to the Brassicaceae family such as horseradish, cauliflower and cabbage (Dufour, Stahl & Baysse, 2015) and are known to protect plants against microbial infection (Bending & Lincoln, 2000). ITCs are produced from the enzyme, myrosinase, acting on plant glucosinolates when the plant tissues are disrupted (Dufour, Stahl & Baysse, 2015) (Figure 2). The result is a family of volatile, electrophilic substances, some of which have been shown to display an inhibitory effect on various pathogenic bacteria (Chacon, Buffo & Holley, 2006). ITCs have also been found to have an additive effect which allows them to be used in conjunction with other antibiotic agents to reduce dosages but maintain the same potency (Dufour, Stahl & Baysse, 2015). This may have the potential to slow down the rate at which a microbial strain develops antibiotic resistance (Dufour, Stahl & Baysse, 2015). ITCs can be divided into two classes – aliphatic and benzenic – with studies showing that short-chained benzenic ITCs generally display more activity against gram negative and gram positive bacteria than shortchained aliphatic ITCs (Dufour et al., 2012; Nowicki et al., 2016). Despite this, the aliphatic compound allyl isothiocyanate (AITC) (Figure 3) is of particular interest in the scientific community due to its specific side chain structure. This unique structure gives AITC its antibacterial and additive properties (Dufour, Stahl & Baysse, 2015). AITC is effective as an antibacterial agent in both liquid and vapour form (Lin, Preston & Wei, 2000), supporting its current application as a food preservative in Japan provided it comes from a natural source (Nadarajah, Han & Holley, 2005). This is due to the compound being highly volatile, toxic and carcinogenic in its pure extracted form (GHS Classification Guidance by the Japanese Government 2013). It should be noted that unlike its application as a treatment for bacterial infections mentioned previously, the aim of using AITC in food preservation is to kill pathogenic bacteria to prevent the pathogen from entering the body. Furthermore, AITC has a limited shelf life due to the compound’s decomposition at room temperature however, despite these limitations, no superior alternatives have been identified as low concentrations are effective on various pathogenic
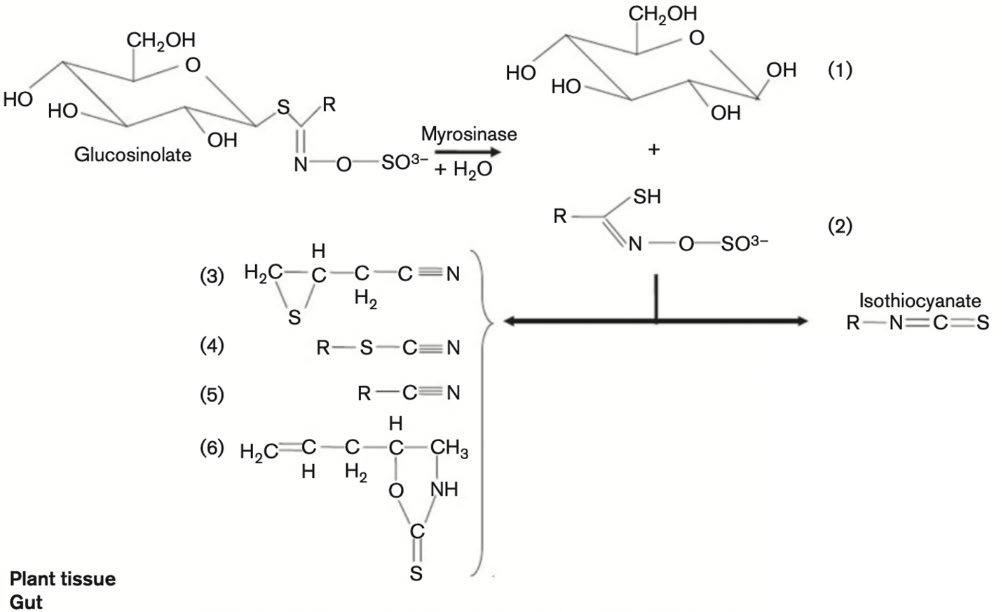
Figure 2: Degradation of glucosinolates by myrosinase. Myrosinases catalyse the hydrolysis of glucosinolates to give unstable aglucones and glucose (1). The products that are formed vary depending on the glucosinolate side chain (represented by R) and on the reaction conditions. The products are thiohydroxamate-O-sulfonate (2), epithionitrile (3), thiocyanate (4), nitrile (5), oxazolidine-2-thione (6), and ITC.
microorganisms and do not detract from the sensory quality of the food (Nadarajah, Han & Holley, 2005).
Consequently, this report has selected to investigate the antibacterial potential of the synthetic compound methallyl isothiocyanate (MAITC) as an alternative. MAITC (Figure 4) is an analogue of AITC – differing structurally only by a CH₃ group (Fizer 2013) – and as such may possess similar antibacterial properties to AITC with potentially more favourable chemical properties. It is well known that an allyl side chain, as in AITC, is a reactive structure. Adding a methyl group as in MAITC may mitigate the reactivity of the side chain in comparison, leading to a more stable compound which may possess more favourable properties which make it easier to handle and use as a food preservative. MAITC has also been thought to possess anticarcinogenic properties and shown to have an antiinflammatory response to mast cell-mediated inflammatory reactions, similarly to other isothiocyanates (Han et al., 2012). Although numerous biological properties are associated with this compound, the antibacterial potential of MAITC remains unknown due to the lack of research in this area (Han et al., 2012).
A similar investigation was conducted in 2019 which tested the antibacterial potential of another compound, propyl isothiocyanate (Cheng, 2019). The results found this compound – which differed from AITC by a single carbon bond in place of the carbon-carbon double bond – to display no signs of antibacterial activity in the diluted concentrations however the pure form displayed complete inhibition (Cheng, 2019). Degradation pathways were discussed to explain this phenomenon although further investigation is required. Similarly, this report will investigate whether various concentrations of MAITC display antibacterial activity against K-12 E. coli.
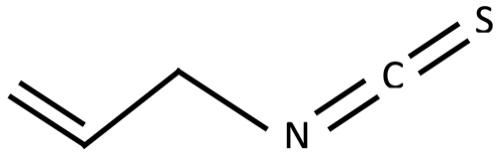
Figure 3: Chemical structure of allyl isothiocyanate Figure 4: Chemical structure of methallyl isothiocyanate
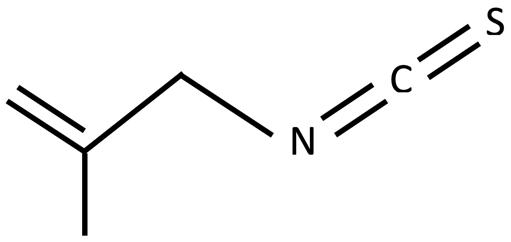
Scientific research question
Does methallyl isothiocyanate have comparable antibacterial activity to the activity reported for allyl isothiocyanate against K-12 E. coli.?
Scientific hypothesis
That methallyl isothiocyanate will display antibacterial activity against K-12 E. coli, measured by the zone of inhibition.
Methodology
Chemicals
Methallyl isothiocyanate (MAITC) was purchased from Sigma Aldrich (Sydney, Australia)
Bacteria
K-12 E. coli and chloramphenicol antibiotic was purchased from Southern Biological (Knoxfield, Victoria, Australia). 9 plates of Mueller Hinton agar were prepared, according to the manufacturer's instructions.
Sterilisation
A 70% alcohol solution was used to wipe the bench. Metal forceps and glass pipettes were dipped in the 70% alcohol solution before burning the alcohol off in a Bunsen burner. Different sterile glass pipettes were used for transferring each substance and concentration.
Three 6mm diameter wells were created pushing the large end of a glass pipette into the agar and removing the remaining circle.
Serial Dilutions
A standard solution of MAITC was created by dissolving 0.20 g of MAITC into 40 mL of DMSO and 60 mL of water in a 100 mL volumetric flask. This created a stock solution of concentration 2000 mg/L. Serial dilutions were carried out into 20 mL volumetric flasks by pipetting 1 mL of solution and 19 mL of distilled water into 20 mL volumetric flasks to create solutions with the following concentrations:
Table 1: Serial dilutions of MAITC
Solution Concentration (mg/L)
Total volume (mL)
1 2000 100 2 100 20 3 5 20
Inoculation and plating
Each agar plate was divided into 4 equal sections using a marker and labelled with the solution it would contain (Figure 5). The agar plates were relocated to a fume hood containing an ignited Bunsen burner to create an updraft of air, removing contaminants from the work site. The lid of a plate was removed. A well was created in three of the four sections of agar using the large end of a sterile glass pipette and the agar discs were removed to create the well. Using sterile disposable glass pipettes, 2 drops of K-12 E. coli were placed on each plate and spread evenly around the plate using a glass spreader. 1 drop of the 2000mg/L MAITC solution was put into the well labelled MAITC 1 and 1 drop of DMSO (acting as a negative control) was put into their respective wells. Sterile metal forceps were used to transfer the antibiotic tablet (acting as a positive control) onto the plate and the remaining well (acting as a negative control) was left blank. The lid was reapplied. This process was repeated for the remaining two plates with the label MAITC 1.
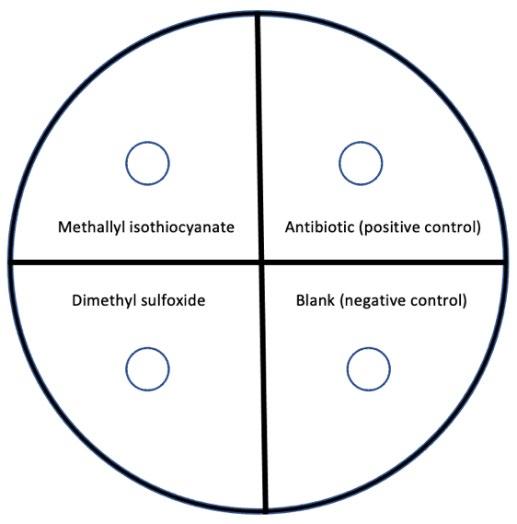
Figure 5: Diagram of agar plate setup (divided into 4 sections).
The same process was used to prepare three plates containing the 100 mg/L solution of MAITC (labelled MAITC 2), and similarly to prepare the 5 mg/L solution labelled MAITC 3. Each plate was sealed using tape and placed in an incubator at 37℃ for 36 hours.
Determination of inhibitory concentration
The annular radius was measured using vernier callipers. If a zone of inhibition was detected, the substance was deemed to be antibacterial (Figure 6). If no zone of inhibition observed, the substance specific concentration of the substance was deemed to be ineffective at inhibiting bacterial growth.
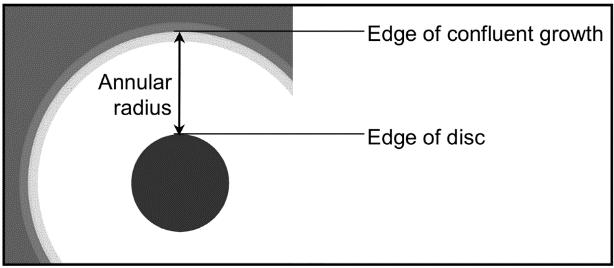
Figure 6: Measurement of annular radius (Source: Bell et al. 2018, pp. 19).
Results
A test for each concentration was conducted 3 times (Figure 7). In every case, the chloramphenicol positive control displayed antibacterial activity measured by the zone of inhibition (Table 2). The blank section of agar and DMSO (acting as negative controls) showed no inhibition (Figure 8) on every plate. All plates for each concentration of MAITC tested displayed no antibacterial activity (Table 2) determined by the absence of a zone of inhibition.
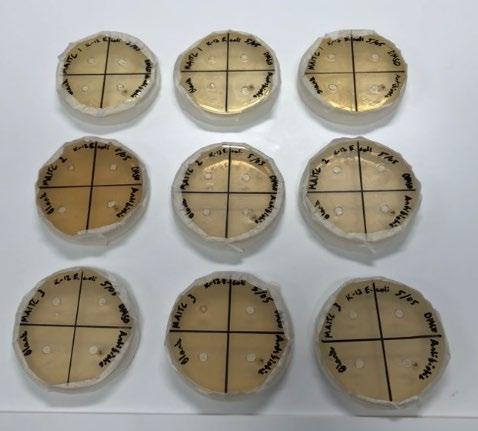
Figure 7: All 9 plates containing 3 repetitions of each concentration
Table 2: Zone of inhibition of chloramphenicol and different concentrations of MAITC against K-12 E. coli
Concentration of MAITC (mg/L) Average zone of inhibition (mm)
MAITC Chloramphenicol 2000 No inhibition 8.7 100 No inhibition 9.0 5 No inhibition 8.3
Figure 8: Agar plate of each concentration of MAITC
Discussion
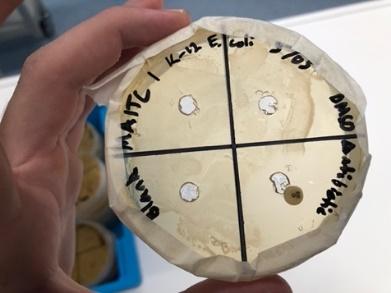
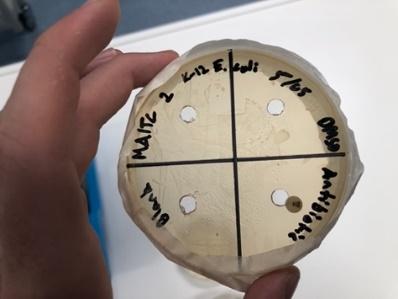
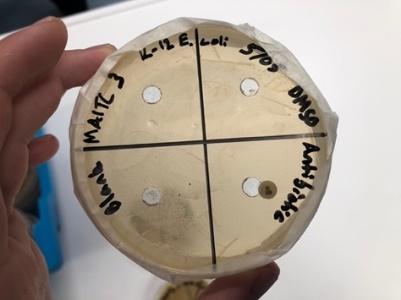
A lawn of K-12 E. coli was successfully grown on each agar plate. The inhibitory activity demonstrated by the positive control (chloramphenicol) and lack of activity in both the negative controls (blank well and well filled with DMSO) show the results of the different concentrations of MAITC to be valid and reliable. Despite the structural similarities to AITC, the experimental concentrations of MAITC were found to not possess antibacterial activity.
It is possible that the concentrations selected did not provide a wide enough range for MAITC to display activity. A highly relevant research paper investigating the potential candidacy for the analogue propyl isothiocyanate as an antibacterial agent displayed identical results in a similar range of concentrations however when tested in its pure, undiluted form, the compound displayed antibacterial properties (Cheng 2019). It could therefore be speculated that using undiluted MAITC would yield a similar outcome. Further research would need to be conducted to determine whether MAITC is antibacterial at higher concentrations, which would be valuable to the scientific literature, however the application of an efficacious dose of MAITC as an antibacterial agent would require a clinically unacceptable quantity compared to existing, more potent antibacterial agents. If a zone of inhibition was observed, an ANOVA test with post-hoc tukey would have been conducted to analyse the difference in the means between concentrations of the compound against K-12 E. coli however, as no zone of inhibition was detected this was not conducted.
Previous studies have suggested AITC’s antibacterial activity results from either its ability to alter the structures of essential proteins, attack the active site of enzymes or interfere with bacterial enzymic activity, respiration, metabolism and the transcription of genes (Kawakishi & Kaneko, 1985; 1987; Dufour, Stahl & Baysse, 2015, p.20; Luciano & Holley, 2009). As conflicting proposals for the mechanism of AITC’s antibacterial activity exist, the true mode of action remains unknown. Despite this, one potential explanation for MAITC’s inactivity at the tested concentrations may be the presence of an additional CH₃ group (Fizer, 2013). As previously mentioned, ITCs consist of a functional group (Figure 1) and a side chain which differs between each ITC, giving it specific properties. Chemical reactions can be easily initiated at either the C or N centre of the –N=C=S group (Figure 1) without losing reactive possibilities of the other centre (Fizer, 2013). As this functional group is common to all
ITCs, it would suggest that different side chains (i.e. between AITC and MAITC) are likely the cause of difference between the activity of the two compounds. In other words, the allyl side chain, as in AITC, holds partial responsibility for the antibacterial activity of the compound compared to the additional methyl group present in MAITC side chain which may alter the properties of the compound, making this activity is no longer possible.
A phenomenon which may provide another explanation for MAITC’s inactivity is the degradation of AITC in water. Research conducted by Olaimat and Holley in 2013 proposed that the bactericidal activity of AITC is strongest at room temperatures as the compound is more stable than at higher temperatures with a 2009 study finding that AITC decomposes rapidly in water at 37℃ (Luciano & Holley 2009). Similarly, degradation pathways may also occur with MAITC when dissolved in water, although further exploration of the decomposition products has yet to be carried out. MAITC is insoluble in water (as is AITC) and was therefore mixed first with DMSO prior to carrying out the serial dilutions. After introducing water to the MAITC-DMSO solution both heat and gas were produced, which may indicate the occurrence of chemical degradation when mixing MAITC with either water (as supported by the literature) or even possibly the solvent DMSO. It is unlikely that the reaction resulted from the mixing of DMSO and water as there is no evidence in the literature to support this.
Multiple papers propose different decomposition products of AITC in water (Figure 9) however the majority of them agree the products are not responsible for antibacterial activity. If similar products occur from the degradation of MAITC in water then it could be speculated that the pure, undiluted form of the compound may display antibacterial activity and the serial dilutions carried out in this investigation inhibited this result.
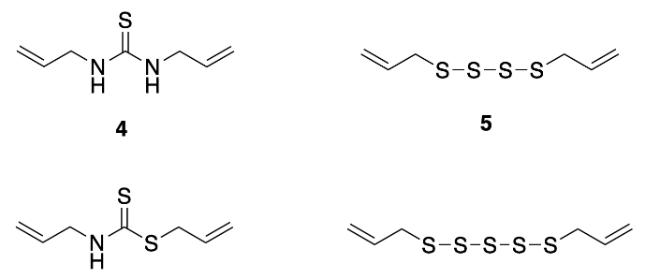
Figure 9: Proposed decomposition products of AITC (Source: Cheng, 2019)
A proposed degradation pathway of AITC to allyl thiocyanate (Figure 10) includes an allylic shift via a circular six membered ring transition state to achieve degradation (Pecháček, Velíšek & Hrabcová, 1997). The importance of the carbon-carbon double bond on the allyl side chain for this could potentially allow MAITC to degrade by following this pathway as this bond is present in both compounds.
An alternative degradation pathway can be seen in Figure 11 which involves the formation of dimethallylthiourea from MAITC. This pathway does not rely on the importance of the carbon-carbon double bond and instead involves the addition of an ion to the functional group of the compound. There is a strong case for this pathway as the hydroxide ions would have been present during the serial dilations in which water was combined with the compound.
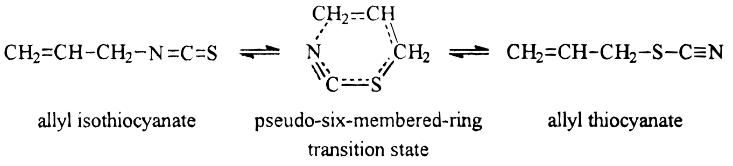
Figure 10: Rearrangement pathway from AITC to allyl
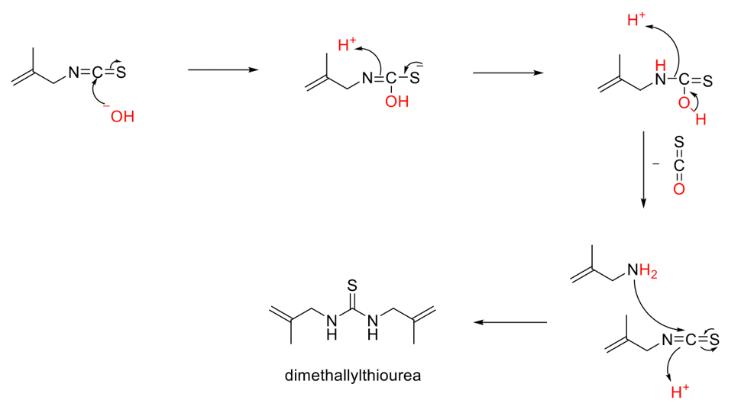
Figure 9: Proposed degradation pathway for formation of dimethallylthiourea from MAITC
Conclusion
MAITC was found to not display antibacterial activity at the tested concentrations against K-12 E. coli as determined by a lack of an observable zone of inhibition. These results are supported by all positive and negative controls working as intended on each plate for all concentrations. The findings may be a result of the experimental concentrations being too low and as such further investigation is advised. Another explanation may be the additional CH₃ group to the AITC side chain to form MAITC, which could potentially inhibit the mechanism for AITC’s antibacterial activity. It may also be due to chemical degradation through similar pathways to those proposed for AITC or the proposed pathway for the degradation of MAITC with hydroxide ions to produce dimethallylthiourea. This potential pathway suggests that MAITC may display antibacterial when in its undiluted, pure form however subsequent research is required.
Acknowledgements
I wish to show my appreciation to Dr Katie Terrett for her supervisory assistance in generating this research report and Dr Alison Gates for her expertise and assistance in carrying out the practical experiment.
References
Bending, G.D. & Lincoln, S.D. 2000, ‘Inhibition of soil nitrifying bacteria communities and their activities by glucosinolate hydrolysis products’, Soil Biology and Biochemistry, vol. 32, no. 8–9, pp. 1261–9. Beutin, L. & Martin, A. 2012, ‘Outbreak of Shiga Toxin–Producing Escherichia coli (STEC) O104:H4 Infection in Germany Causes a Paradigm Shift with Regard to Human Pathogenicity of STEC Strains’, Journal of Food Protection, vol. 75, no. 2, pp. 408–18. Chacon, P., Buffo, R. & Holley, R. 2006, ‘Inhibitory effects of microencapsulated allyl isothiocyanate (AIT) against Escherichia coli O157:H7 in refrigerated, nitrogen packed, finely chopped beef’, International Journal of Food Microbiology, vol. 107, no. 3, pp. 231–7. Cheng, S. (2019). Antibacterial activity and chemical degradation pathways of propyl isothiocyanate. Barker Science Extension Journal, 1(1). Dufour, V., Alazzam, B., Thepaut, M., Ermel, G. & Baysse, C. 2012, ‘Antimicrobial Activities of Isothiocyanates Against Campylobacter jejuni Isolates’, Frontiers in Cellular and Infection Microbiology, vol. 2, viewed 14 June 2021, <https://www.frontiersin.org/articles/10.3389/fcimb.2012.00 053/full>.
Dufour, V., Stahl, M. & Baysse, C. 2015, ‘The antibacterial properties of isothiocyanates’, Microbiology, vol. 161, no. 2, pp. 229–43. Fizer, M. 2013, ‘Methallyl Isothiocyanate’, Synlett, vol. 24, no. 15, pp. 2019–20. Han, N.-R., Kim, I.-K., Kim, H.-M. & Jeong, H.-J. 2012, ‘Methallyl isothiocyanate inhibits the caspase-1 activity through the inhibition of intracellular calcium levels’, Biochimie, vol. 94, no. 3, pp. 816–22. Kawakishi, S., and T. Kaneko. 1985. ‘Interaction of oxidized gluta- thione with allyl isothiocyanate’. Phytochemistry vol. 24, pp. 715–718.
Kawakishi, S., and T. Kaneko. 1987. ‘Interaction of proteins with allyl isothiocyanate. J. Agric’. Food Chem. vol. 35, pp. 85–88.
Kim, J.-S., Lee, M.-S. & Kim, J.H. 2020, ‘Recent Updates on Outbreaks of Shiga Toxin-Producing Escherichia coli and Its Potential Reservoirs’, Frontiers in Cellular and Infection Microbiology, vol. 10, viewed 14 June 2021, <https://www.frontiersin.org/articles/10.3389/fcimb.2020.00 273/full>.
Kuhnert, P., Nicolet, J. & Frey, J. 1995, ‘Rapid and accurate identification of Escherichia coli K-12 strains.’, Applied and environmental microbiology, vol. 61, no. 11, pp. 4135–9. Lin, C.-M., Preston, J.F., III & Wei, C.-I. 2000, ‘Antibacterial Mechanism of Allyl Isothiocyanate†’, Journal of Food Protection, vol. 63, no. 6, pp. 727–34. Luciano, F.B. & Holley, R.A. 2009, ‘Enzymatic inhibition by allyl isothiocyanate and factors affecting its antimicrobial action against Escherichia coli O157:H7’, International Journal of Food Microbiology, vol. 131, no. 2, pp. 240–5. May, F.J., Polkinghorne, B.G. & Fearnley, E.J. 2016, Epidemiology of bacterial toxin-mediated foodborne gastroenteritis outbreaks in Australia, 2001 to 2013, vol. 40, no. 4, p. 10. Ministry of Health, Labour and Welfare, Ministry of the Environment 2013, ‘GHS Classification Result Table’ GHS Classification Guidance Japanese Government, Japan. Munita, J.M. and Arias, C.A., 2016. ‘Mechanisms of antibiotic resistance’. Microbiology spectrum. vol 4. no. 2 Nadarajah, D., Han, J.H. & Holley, R.A. 2005, ‘Use of mustard flour to inactivate Escherichia coli O157:H7 in ground beef under nitrogen flushed packaging’, International Journal of Food Microbiology, vol. 99, no. 3, pp. 257–67. Nowicki, D., Rodzik, O., Herman-Antosiewicz, A. & Szalewska-Pałasz, A. 2016, ‘Isothiocyanates as effective agents against enterohemorrhagic Escherichia coli : insight to the mode of action’, Scientific Reports, vol. 6, no. 1, p. 22263. Olaimat, A. and Holley, R. 2013, ‘Effects of changes in pH and temperature on the inhibition of Salmonella and Listeria monocytogenes by Allyl isothiocyanate’. Food Control, vol. 34, pp. 414-419.
Pecháček, R., Velíšek, J. & Hrabcová, H. 1997, ‘Decomposition Products of Allyl Isothiocyanate in Aqueous Solutions’, Journal of Agricultural and Food Chemistry, vol. 45, no. 12, pp. 4584–8. World Health Organization (2020). Antibiotic resistance. [online] World Health Organization. Available at: https://www.who.int/news-room/fact-sheets/detail/antibioticresistance.