WINTER 2022
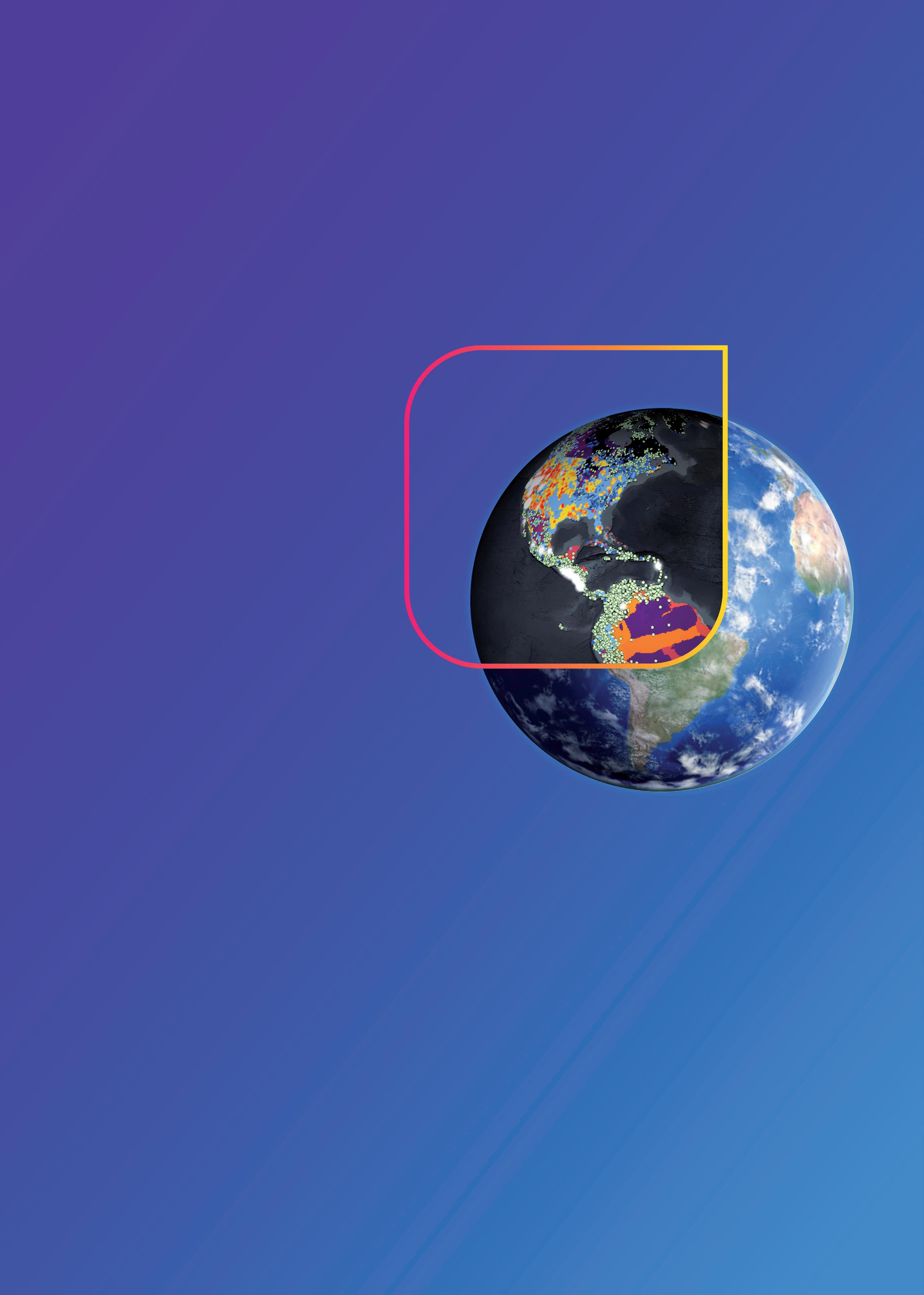
Advancing a sustainable energy future for all
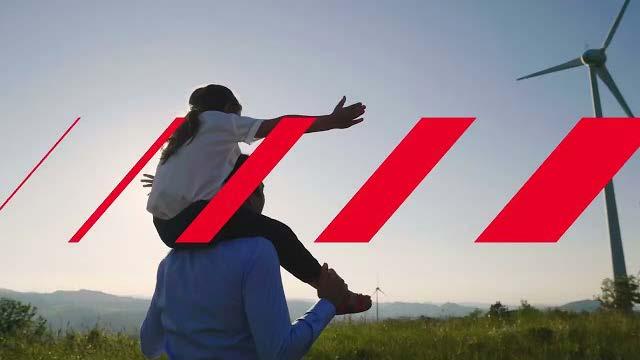
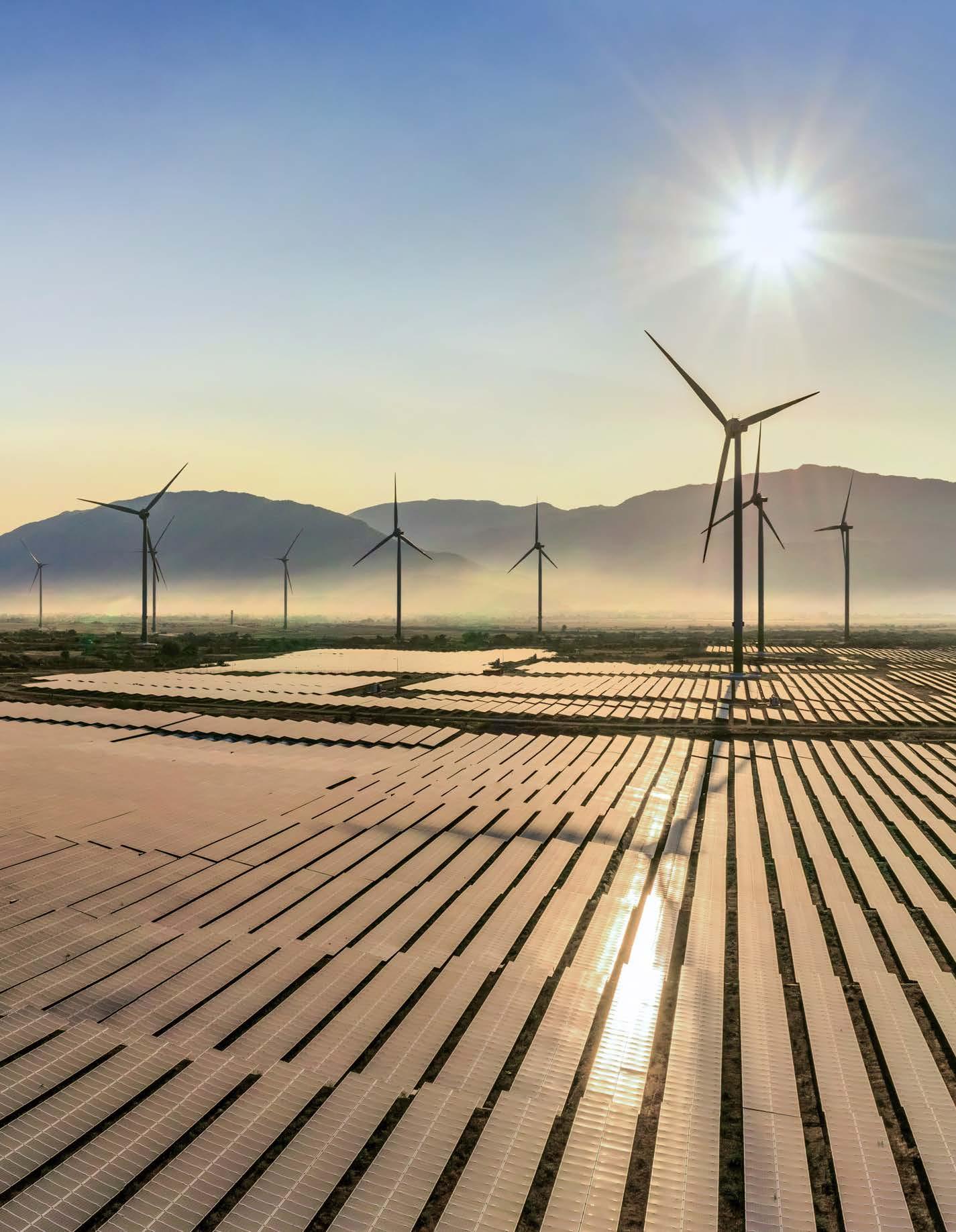
We are advancing the world’s energy system to be more sustainable, flexible and secure.
ENERGY GLOBAL
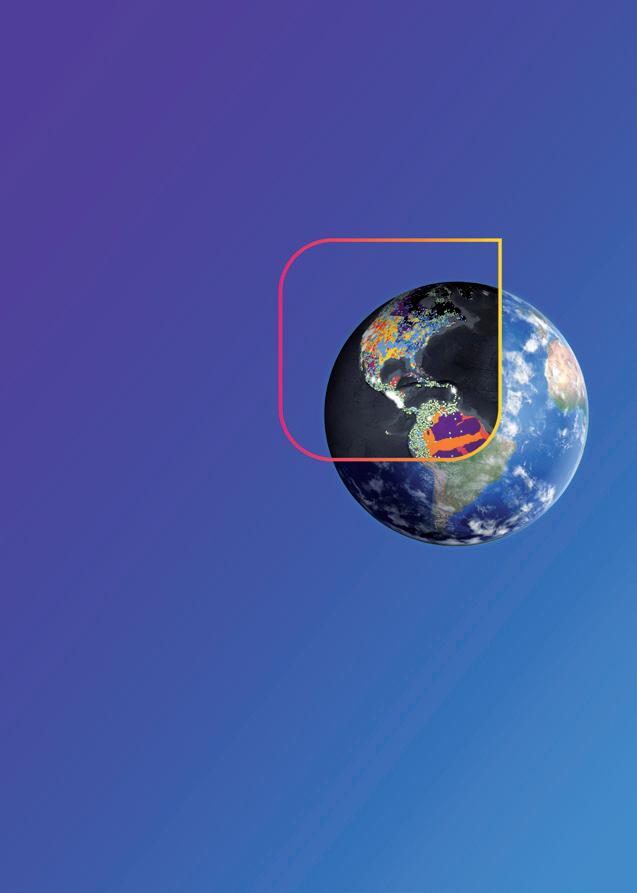
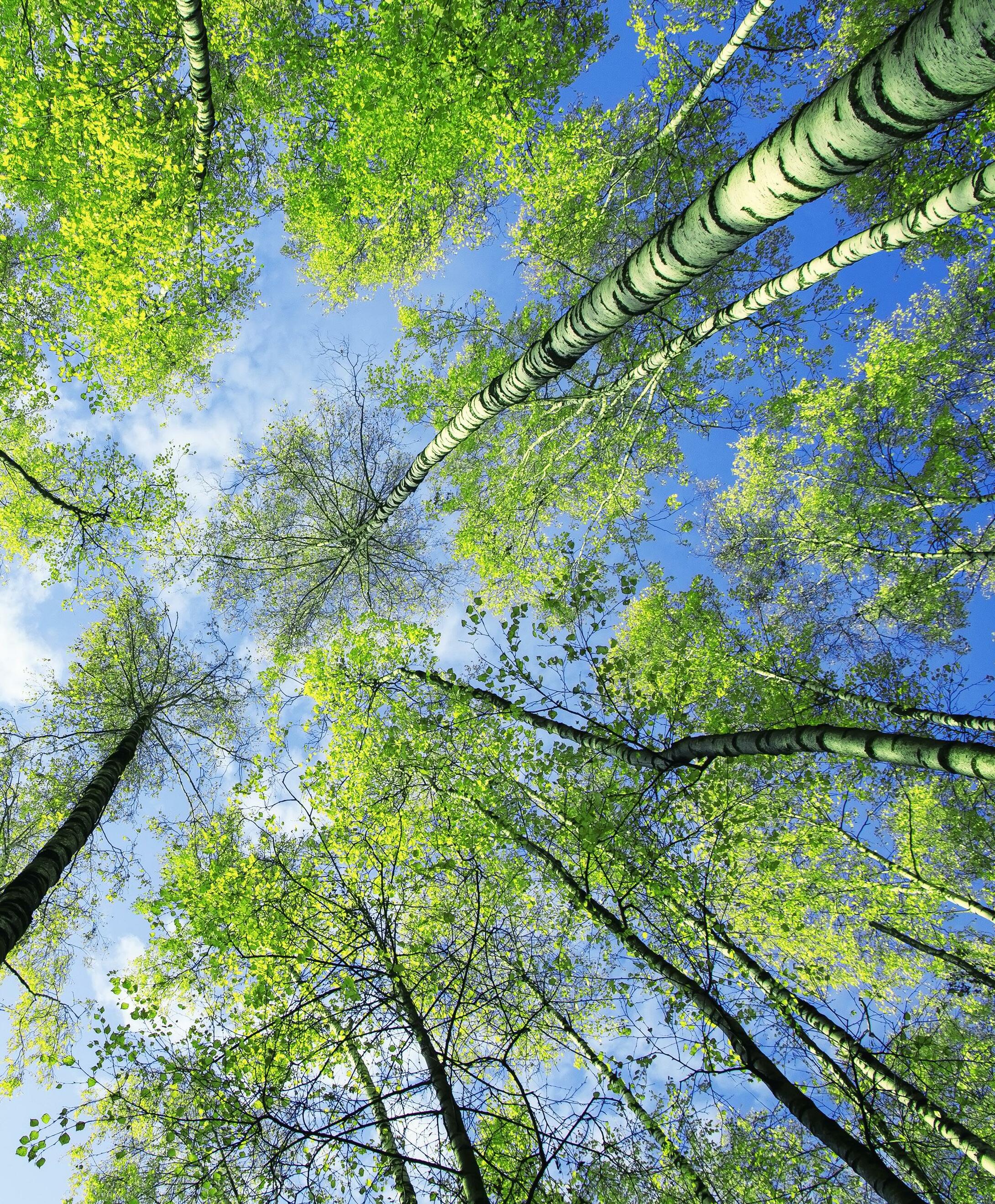
WINTER 2022
36. Staying safe whilst subsea Austin Harbison, Smart Technology Manager, CRP Subsea, UK. 42. Taking the power back Paul Cairns, Managing Director at MJR Power and Automation, UK. 48. Overcoming supply challenges with collaboration
Cerianne Cummings, Offshore Wind Market Director, Kent. 52. Reshaping Kazakhstan's energy sector
Taylor Mattie, Director of Geothermal Technologies and Innovations, Baker Hughes, USA.
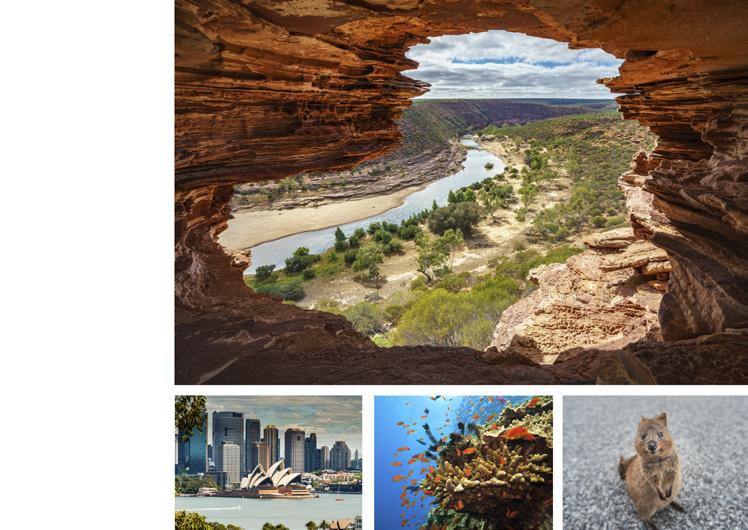
Serik Shakhazhanov, Chairman of the Management Board of Eurasian Group LLP, Kazakhstan. 56. Enhancing resilience and stability Dr Carlos de Palacio, Renewable Segment Manager Grid Integration, Hitachi Energy, Spain. 60. A strong energy storage foundation Martin Vogt, CEO of MPC Energy Solutions 64. Here comes the sun? Mark Rowcroft, Development Director, Exagen, UK. 68. Striving for sustainable solar Dongyoung Kim, Technical Assistant, Reddie & Grose. 72. Fuelling North America's Renaissance in anaerobic digestion Shawn Kreloff, Bioenergy Devco Founder and CEO, USA. 76. Global news
Rasmus Rubycz, Market Manager New Energy, Atlas Copco Gas and Process Division, Germany.
Copyright © Palladian Publications Ltd 2022. All rights reserved. No part of this publication may be reproduced, stored in a retrieval system, or transmitted in any form or by any means, electronic, mechanical, photocopying, recording or otherwise, without the prior permission of the copyright owner. All views expressed in this journal are those of the respective contributors and are not necessarily the opinions of the publisher, neither do the publishers endorse any of the claims made in the articles or the advertisements.
One coating company protecting the future
With a growing portfolio of high performance products, Seal For Life offers innovative corrosion prevention and thermal insulation technologies for infrastructure protection.
A track record of more than 60 years, always pushing the boundaries to develop new solutions for the challenges of tomorrow.
sealforlife.com
COMMENT
Simone AccorneroCo-Founder & CEO, FlexiDAO
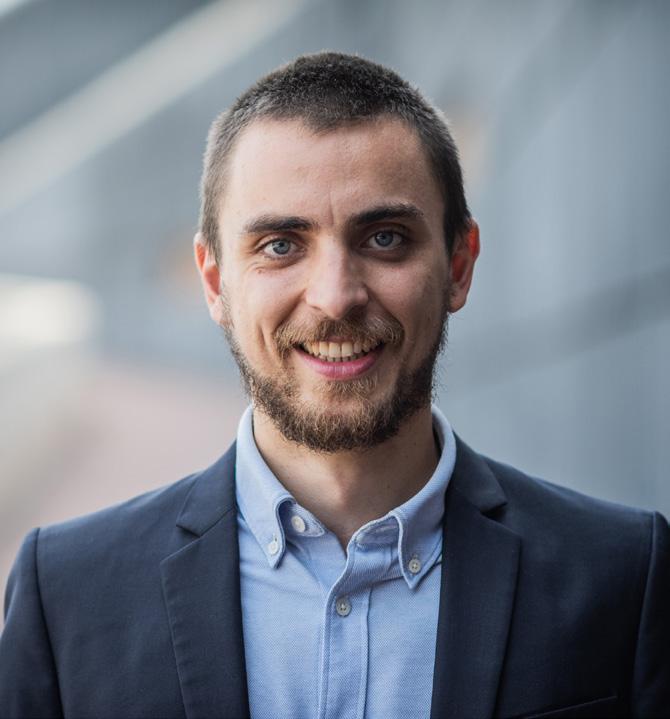
The energy sector is responsible for over three-quarters of human-caused emissions,1 making decarbonisation of our energy supplies the single biggest carbon dioxide challenge our generation faces today.
Many of the perceivable solutions involve transitioning to an electricity-based economy; for instance, updating to electric heat and transport, or replacing natural gas with hydrogen produced in electrolysers. Yet, pursuing these solutions will increase demand for electricity and put more pressure on our power grids, which are currently running on a mix of carbon-free and carbon-intensive sources. For the electrification of our society to truly reduce emissions, we need carbon-free electricity grids.
As some of the largest energy buyers, companies have a crucial role to play in generating enough demand for carbon-free electricity. Voluntary corporate procurement has been critical in expanding the deployment and installation of new renewable electricity so far. According to the current, most adopted global carbon accounting standard (The GHG Protocol), organisations can buy renewable electricity to meet their annual electricity demand and claim they are running on ‘100% renewable energy’ in their Scope 2 market-based emissions accounting.
Whereas market-based emissions accounting has provided incentives for companies to act, the challenge is that it does not consider the real locational and market constraints of our electricity grids, nor the hourly or sub-hourly fluctuations in where electricity comes from. ‘100% renewable energy’ claims do not mean that a business is running on carbon-free electricity every hour of the day.2 To give an example, a Dutch data centre consuming electricity throughout the day and during the night could claim to be ‘100% renewable’ by covering its annual consumption with electricity generated from a solar plant in Portugal. There is no chance at all that the actual electrons related to the volume of electricity (and the underlying certificates as assurance) procured from the solar plant (which will only be producing electricity when it is sunny during the day) ever actually reach the data centre (which is on an entirely different power grid to these electrons).
To remain credible and effective,3 Scope 2 market-based emissions accounting needs to be modernised and adapted to represent the current and future market reality. Market-based accounting needs to reflect the physical constraints of the power grid by imposing stricter requirements4 for quality criteria with regards to location (production should take place in the same or neighbouring power grid as consumption) and time (production should take place in the same hour as consumption).
As the rules of the energy game change to favour a more granular, accurate, and data-led accounting system, the right price incentives will be implemented to drive deeper decarbonisation of our power grids. Many of the technologies needed to fully decarbonise our energy grids – such as long-duration storage – currently suffer from a lack of demand driving down their costs and stimulating further adoption. If businesses begin accounting for their actual electricity usage on an hourly rather than annual basis, and focusing on the local grids that businesses actually operate on, corporate energy buyers will start to seek out these solutions for the times of the day when they are currently reliant on fossil fuels, using their purchasing power to much greater effect.5
References
1. ‘4 Charts Explain Greenhouse Gas Emissions by Countries and Sectors’, World Resources Institute, (2020), www.wri.org/insights/4charts-explain-greenhouse-gas-emissions-countries-and-sectors
2. BJØRN, A., LLOYD, S. M., BRANDER, M., and MATTHEWS, H. D., ‘Renewable energy certificates threaten the integrity of corporate science-based targets’, Nature Climate Change, No.12, pp.539 – 546, (2022), https://doi.org/10.1038/s41558-022-01379-5
3. ELGIN, B. and RANGARAJAN, S., ‘What Really Happens When Emissions Vanish’, Bloomberg UK, (2022), www.bloomberg.com/ news/features/2022-11-01/intel-p-g-cisco-among-major-companiesexaggerating-climate-progress
4. ‘GHG Protocol to assess the need for additional guidance building on existing corporate standards’, Greenhouse Gas Protocol, (2022), https://ghgprotocol.org/blog/ghg-protocol-assess-need-additionalguidance-building-existing-corporate-standards
5. ‘Advancing Decarbonisation through Clean Electricity Procurement’, International Energy Agency, (2022), www.iea.org/reports/advancingdecarbonisation-through-clean-electricity-procurement
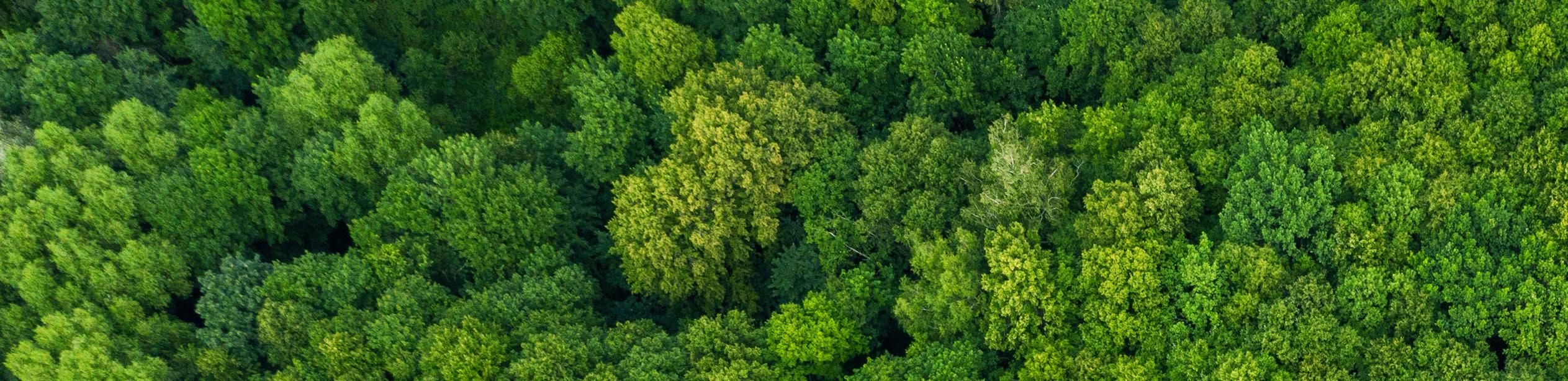
location,
Jessica Casey, Deputy Editor, and Abi Larkin, Editorial Assistant, Energy Global, UK, look at the Australian renewables sector.In September 2022, the Australian government passed legislation with the aim of reducing carbon emissions by 43% by 2030 and achieving net zero by 2050.1 The country also has a Renewable Energy Target scheme, which aims to reduce greenhouse gas emissions from the electricity sector, by encouraging investment in renewable energy power sources, including wind and solar farms, and hydroelectric power stations. 2 Australia is an island nation and generally considered to have a hot climate – factors which also contribute positively to the likelihood of green energy generation being achieved.
With concerns over climate change and global warming ever present, it is becoming increasingly important to reduce global emissions and improve the sustainability of power supplies. In addition, Russia’s recent conflict with Ukraine, and the subsequent restrictions on gas supply to Europe, has meant many countries have needed to find alternative sources of energy.
Australia did not escape the wider effects of this, with the cost of oil, gas, and electricity increasing significantly. But with Australia well positioned to take advantage of natural resources, such as wind and solar, renewables could help Australia to meet the demand of domestic energy demand.
In 2021, 29% of Australia’s total electricity generation was from renewable energy sources, including solar (12%), wind (10%), and hydro (6%) – this share of renewables was the highest on record, with the previous peak being 26% in the mid-1960s.3
Wind
Wind generation in Australia grew 19% in 2021, and by an average of 15% y/y over the last decade.3 The wind industry has also experienced significant technological advancements over the past few decades, especially in regards to the size and generation capacity of wind turbines. In the 1980s, wind turbines were approximately 17 m tall with a capacity of approximately 0.75 MW; the latest generation of offshore wind turbines in 2021 were up to 250 m tall, with a generation capacity of up to 15 MW.4
According to NOPSEMA, Australia’s offshore energy regulator, a site is considered to be suitable for an offshore wind project if:
location,
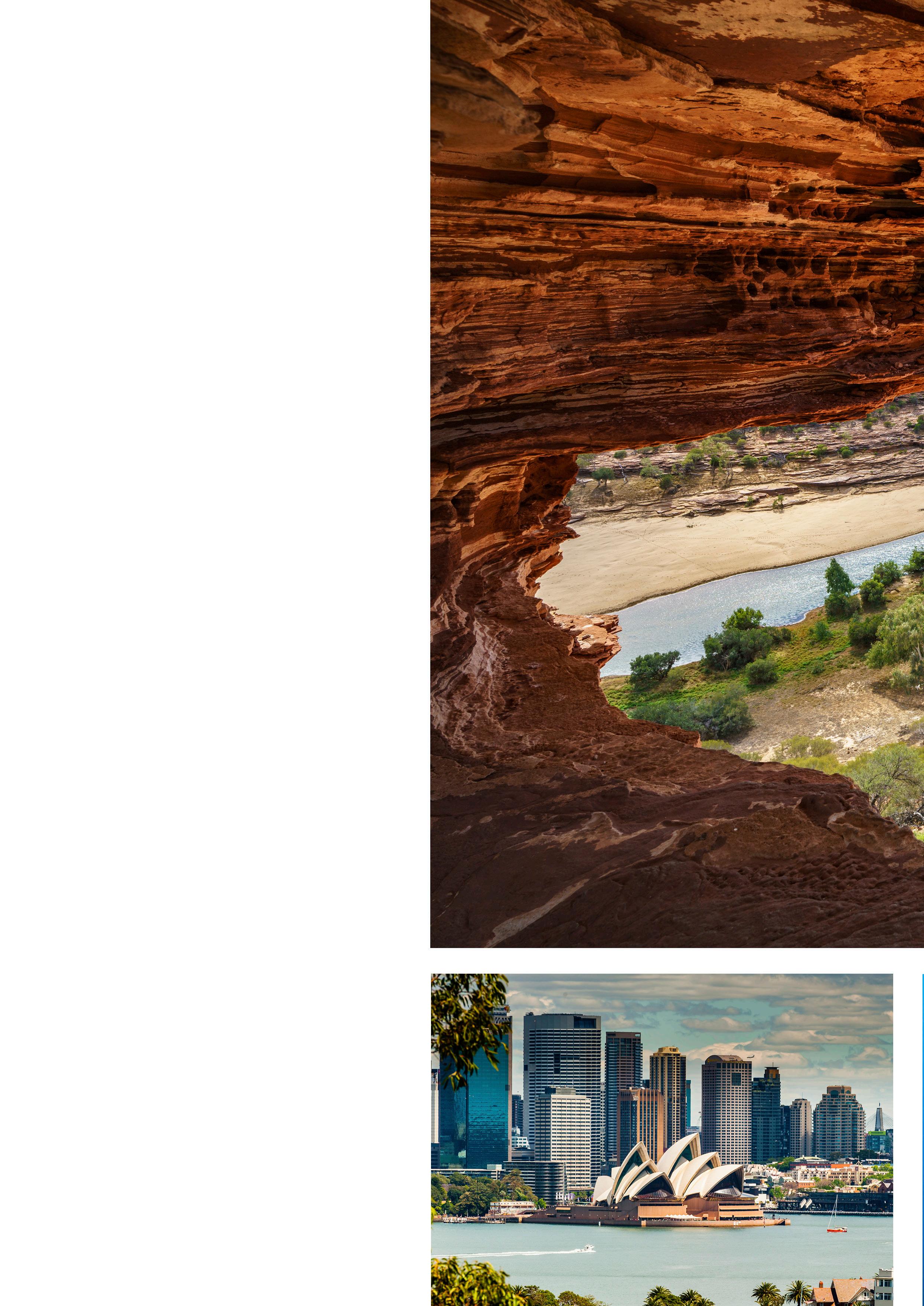
location: Australia
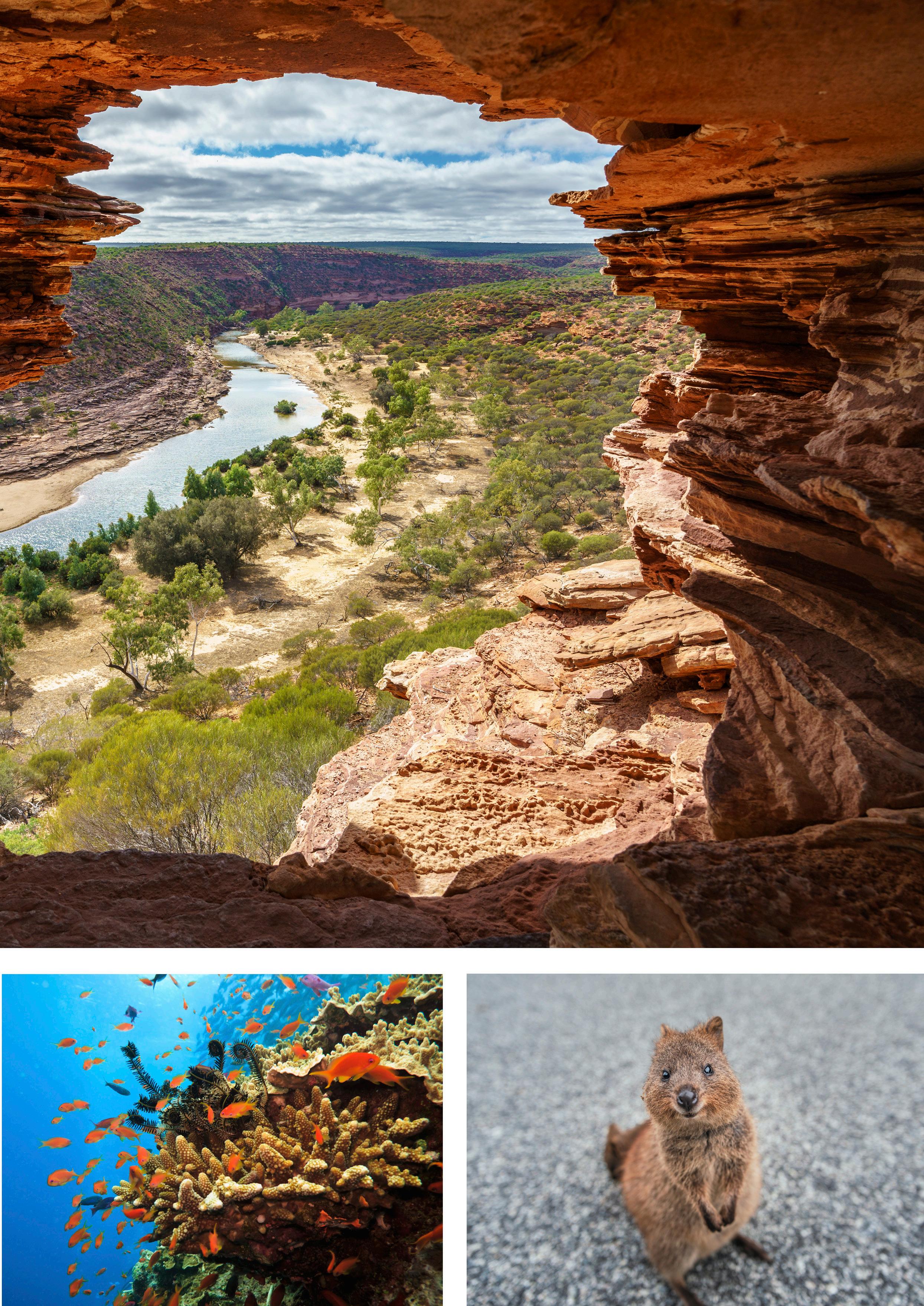
F It has high and relatively consistent wind speeds.
F It has an appropriate water depth.
F The site is either able to be connected to an electricity grid, or is in a suitable location for the generation of energy export products such as hydrogen and ammonia.5
As an island, Australia has some of the best wind resources in the world. These are mainly located in southern parts of the country, which is situated in the ‘roaring forties’ – areas between latitudes of 40 – 50˚ south in the Southern Hemisphere with persistent winds from the west. The southwest of Western Australia, southern South Australia, western Victoria, northern Tasmania, and elevated areas of New South Wales (NSW) and Queensland have good wind resources.5
Many projects are being developed in these areas. One such example is BlueFloat Energy and Energy Estate’s proposed capacity expansion from 1.275 GW to 2.085 GW of the Greater Gippsland offshore wind project. The project is located in the Bass Strait off the coast of Gippsland in Victoria, which was declared as the first area to be assessed for suitability for offshore wind developments by the Australian government in August 2022.6
In addition, BHP has signed a renewable power purchase agreement (PPA) with Neoen that is expected to supply 70 MW of electricity to Olympic Dam and will support Neoen in constructing the 203 MW Goyder South Stage 1b wind farm. This wind farm will form part of the larger Goyder Renewables Zone in South Australia – a hybrid wind, solar, and storage project – and will introduce new renewable generation into the South Australian electricity grid. Neoen will also construct a large scale battery energy storage system in Blyth to support the PPA and assist in improving the stability of the South Australian electricity grid.7
These projects are just two examples of how Australia is utilising its abundant natural resources; along with the Australian government’s commitment to the Global Offshore Wind Alliance (signed on Energy Day at COP27 in Sharm-El-Sheikh, Egypt,)8 this implies that Australia intends to harness its offshore wind potential as a key part of its future energy mix.
Solar
When people think of Australia, the sun generally comes to mind, and is one of the main attractions for tourists visiting the country. In fact, the Australian continent has the highest solar radiation per square metre of any continent, and consequently some of the best solar energy resources in the world, receiving an average of 58 million PJ of solar radiation per year – this is approximately 10 000 times larger than its total energy consumption.9 However, Australia appears to be endeavouring to make the most of what is available: large scale solar farms are on the rise, with almost 7 GW of generation connected to the electricity grid,10 and an increase in Australia’s large scale solar energy capacity to a total of 5.8 GW across 80 projects, as of February 2022.11
Solar and battery storage
Several of the solar projects have been combined with a battery energy storage system (BESS). BESS’s can help renewable energy supplies effectively respond to energy demands, even when the sun is not shining. For example, Energy Vault Holdings, Inc. has recently announced a notice of award from Meadow Creek Solar for the deployment of a 250 MW/500 MWh BESS at the Meadow Creek solar farm in Victoria. The BESS, being co-located with solar photovoltaics (PV), will provide the resiliency and flexibility of charge and discharge, essential to stabilising renewable energy supply across the network as Australia adopts the Australian Energy Market Operator’s Integrated System Plan.12
Also on the horizon is the Sun Cable project in the Northern Territory, which, at 10 GW, will be the world’s largest solar farm and will feature a battery 150 times larger than the 150 MW Hornsdale Power Reserve in South Australia.13,14 The project is not expected to generate any electricity until 2026; nevertheless, its continued development is a positive sign of the important role large scale solar will play in Australia’s transition to a renewable-fuelled future.
Solar and wind
Many companies are also utilising Australia’s solar and wind in combination with each other. For example, Iberdrola has chosen Australia for the company’s first wind-solar hybrid plant in the world, Port Augusta. The renewable facility, located in South Australia, combines 210 MW of wind power with 107 MW of PV power. Comprising 50 wind turbines and 250 000 solar panels, the complex is the largest wind-solar hybrid farm in the Southern Hemisphere and will prevent 400 000 tpy of carbon dioxide emissions.15 The fact that a Spanish company has chosen Australia for its first solar-wind hybrid plant is testimony to the resources available. Another example of a solar-wind hybrid project is bp’s Asian Renewable Energy Hub (AREH) in the Pilbara region of Western Australia. The company will develop the project in multiple phases, creating up to 26 GW of combined solar and wind power generating capacity. At full scale, the AREH will produce approximately 1.6 million t of green hydrogen. From 1 July 2022, bp took a 40.5% stake and operatorship of the AREH project and will develop the project in collaboration with its project partners: InterContinental Energy, CWP Global, and Macquarie Capital, and Macquarie’s Green Investment Group.16
In addition, Fortescue Future Industries and Windlab are to partner on a Super Hub which could generate more than 10 GW of wind and solar power and underpin the industrial scale production of green hydrogen from purpose-built facilities within Queensland.17 Green hydrogen is considered by many as a possible solution for the decarbonisation of many industries, including transport and energy storage. This ‘Super Hub’ could be incremental in improving the attainability of industrial scale green hydrogen, which has been constrained by the lack of renewables available to power the electrification process. The first stage includes the 800 MW Prairie wind farm and 1000 MW Wongalee project with construction, subject to approvals, expected to
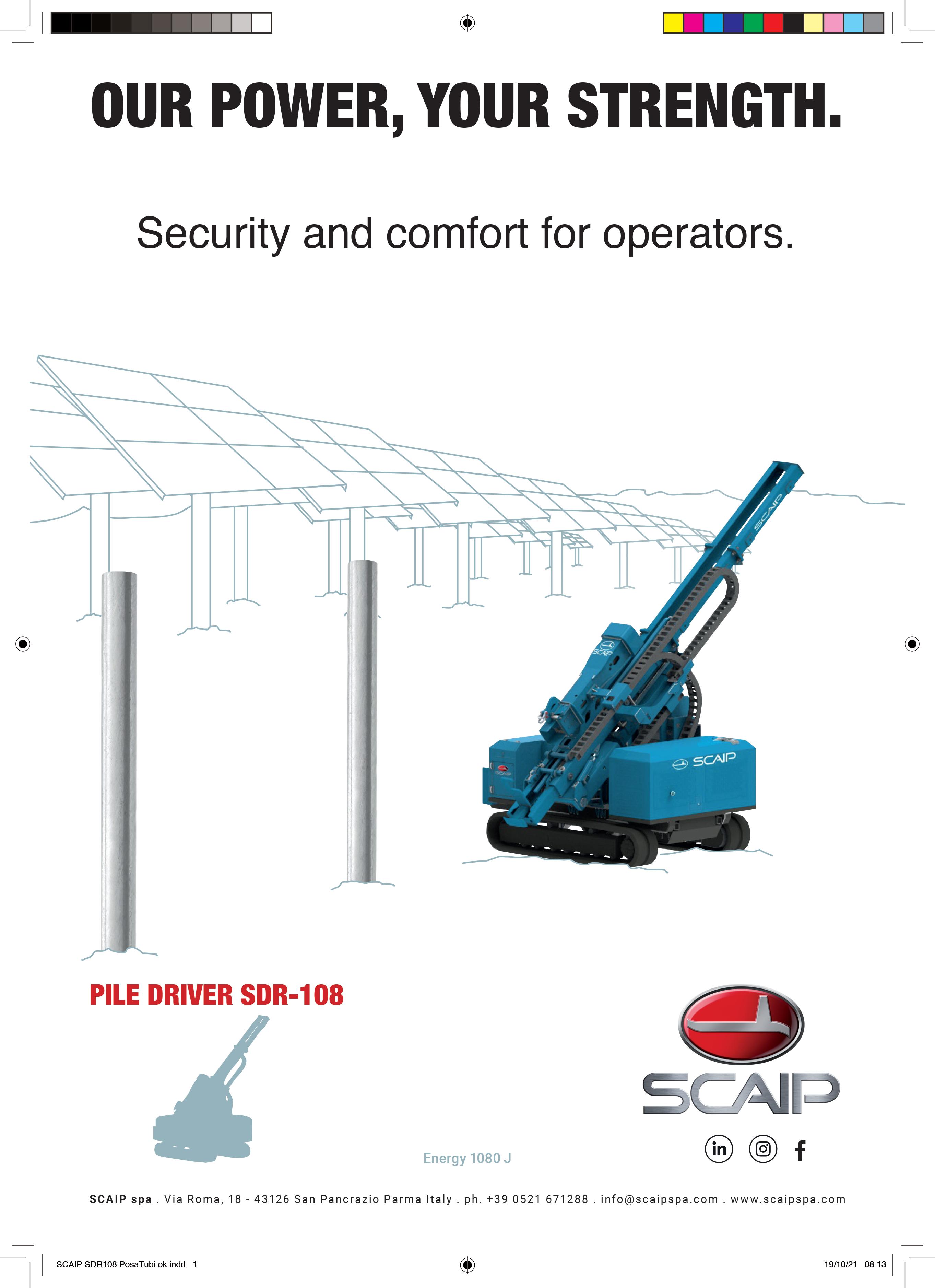
commence in 2025, with power anticipated to begin to be produced by 2027.18
Hydropower
Australia has more than 100 operating hydroelectric power stations, with a total installed capacity of approximately 7800 MW. They are located mostly in NSW and Tasmania, where there is the highest rainfall and elevation,19 with most of the hydroelectricity generated by Hydro Tasmania’s network of power plants and the Snowy Mountains Hydro Scheme in NSW.20
Particularly in Tasmania, hydropower provides much of the state’s electricity. In fact, the abundance of hydropower assets in Tasmania means that it is one of the few jurisdictions in the world that gets all its power from renewable energy – in 2021, approximately 99.9% of Tasmania’s electricity generation came from renewables, according to the Clean Energy Council’s Clean Energy Australia Report 2022.21
The importance of hydroelectricity to Australia’s net zero goals is being demonstrated through the schemes and investments that are being put in place. The Tasmanian integrated hydropower scheme utilises hydro energy from six major water catchments and involves 50 major dams, numerous lakes, and 29 power stations with a total capacity of 2600 MW.19 The Australian government is also making targeted investments to help realise the significant potential that pumped hydro can deliver to the market. The government is supporting pumped hydro through:22
F Partnering with the Tasmanian government to expedite the 1500 MW second Tasmanian interconnect, known as Marinus Link, through to a final investment decision by 2024.
F Making up to AUS$1 billion of low-cost loans available from ‘Rewiring the Nation’ to eligible ‘Battery of the Nation’ projects.
F Providing additional equity to Snowy Hydro Ltd to construct Snowy 2.0.
The biggest new hydro project is Snowy 2.0, a 2000 MW/350 000 MWh project currently under construction in NSW. In 2020, the project received state and federal environmental approval, both of which were fast-tracked as part of the government’s COVID-19 recovery efforts, a AUS$125 million transmission investment by Clean Energy Finance Corp. and approval of a segment factory, which will manufacture 130 000 concrete tunnel segments for use during construction.19 Once complete, Snowy Hydro 2.0 will provide more energy storage capacity than all of the utility scale batteries in the world combined.23
Queensland Premier, Annastacia Palaszczuk, has also recently announced a plan to build the world’s largest capacity pumped storage hydropower project in the north of Queensland. The Pioneer-Burdekin project would provide 5 GW of installed capacity and 24-hour storage, enabling flexibility and security of the state grid. The project is part of the state government’s wider energy plan to reach 70% renewable energy by 2032 and 80% by 2035.17
Conclusion
Australia is not alone in adopting multiple sources of renewable energy in order to cut greenhouse gases. It may not be a solution that will happen in the immediate future due to the time it will take for projects to be approved and constructed; however, the projects that have been highlighted are just some examples that indicate Australia is actively working towards the Paris Agreement’s goals of limiting global warming to 1.5˚C. With the increasing number of proposed renewables project, it is no surprise renewables are expected to supply 69% of Australia’s main electricity grid by 2030,20 and with investment from both international and Australia-based companies, it puts the country in good stead to achieve their net zero goals.
References
1. ‘Australia passes a law for net zero emissions by 2050’, Reuters, (2022), www.reuters.com/ world/asia-pacific/australia-passes-law-net-zero-emissions-by-2050-2022-09-08/
2. ‘Renewable Energy Target Scheme’, Australian Government: Department of Climate Change, Energy, the Environment and Water, www.dcceew.gov.au/energy/renewable/target-scheme
3. ‘Renewables’, Australian Government: Department of Climate Change, Energy, the Environment and Water, www.energy.gov.au/data/renewables
4. ‘Offshore wind energy’, NOPSEMA, (2021), www.nopsema.gov.au/sites/default/files/2021-11/ Offshore%20wind%20energy%20brochure_0.pdf
5. ‘Australia’, Asia Wind Energy Association, www.asiawind.org/research-data/market-overview/ australia/
6. ‘BlueFloat Energy and Energy Estate announce expansion of Greater Gippsland Offshore Wind Project to 2.085 GW’, BlueFloat Energy, (2022), www.bluefloat.com/bluefloat-energyand-energy-estate-announce-expansion-of-greater-gippsland-offshore-wind-project-to-2085-gw/
7. ‘New wind and battery project in South Australia’, BHP, (2022), www.bhp.com/news/mediacentre/releases/2022/11/new-wind-and-battery-project-in-south-australia
8. ‘Australia signs with the Global Offshore Wind Alliance’, Clean Energy Council, (2022), www.cleanenergycouncil.org.au/news/cop27-energy-day
9. ‘Solar energy’, Geoscience Australia, www.ga.gov.au/scientific-topics/energy/resources/otherrenewable-energy-resources/solar-energy
10. ‘Solar energy’, Australian Renewable Energy Agency, https://arena.gov.au/renewableenergy/solar/
11. ‘Solar Report Quarter 1, 2022’, Australian Energy Council, (2022), www.energycouncil.com.au/ media/nb0fjq2z/australian-energy-council-solar-report_q1-2022.pdf
12. ‘Energy Vault Issued Notice of Award From Meadow Creek Solar Farm for a 250MW/500MWh Grid-connected Battery in Victoria, Australia’, BusinessWire, (2022), www.businesswire.com/ news/home/20221026005391/en/Energy-Vault-Issued-Notice-of-Award-From-Meadow-CreekSolar-Farm-for-a-250MW500MWh-Grid-connected-Battery-in-Victoria-Australia
13. ‘Large-scale solar’, Clean Energy Council, www.cleanenergycouncil.org.au/resources/ technologies/large-scale-solar
14. Hornsdale Power Reserve, https://hornsdalepowerreserve.com.au/
15. ‘Iberdrola starts up the world’s first wind-solar hybrid plant in Australia’, Iberdrola, (2022), www.iberdrola.com/press-room/news/detail/iberdrola-starts-up-the-worlds-first-wind-solarhybrid-plant-in-australia
16. ‘Renewable energy hub in Australia’, bp, www.bp.com/en_au/australia/home/who-we-are/ reimagining-energy/decarbonizing-australias-energy-system/renewable-energy-hub-inaustralia.html
17. ‘World’s largest capacity pumped storage hydropower project to be built in Queensland, Australia’, International Hydropower Association, (2022), www.hydropower.org/news/largestcapacity-pumped-storage-project-queensland
18. ‘Game changing North Queensland Super Hub to power green hydrogen with wind, solar’, Fortescue Metals Group, (2022), www.fmgl.com.au/in-the-news/media-releases/2022/11/14/ game-changing-north-queenland-super-hub-to-power-green-hydrogen-with-wind-solar
19. ‘Hydro Energy’, Australian Government: Geoscience Australia, www.ga.gov.au/scientifictopics/energy/resources/other-renewable-energy-resources/hydro-energy
20. ‘Hydro’, Clean Energy Council, www.cleanenergycouncil.org.au/resources/technologies/ hydroelectricity
21. ‘Clean Energy Australia Report 2022’, Clean Energy Council, https://assets.cleanenergycouncil. org.au/documents/resources/reports/clean-energy-australia/clean-energy-australiareport-2022.pdf
22. ‘Pumped hydro’, Australian Government: Department of Climate Change, Energy, the Environment and Water, www.energy.gov.au/government-priorities/energy-supply/pumpedhydro-and-snowy-20
23. ‘Here and now: The state of low emissions technology in Australia’, Australian Academy of Technological Sciences & Engineering, (2022), www.atse.org.au/wp-content/uploads/2022/07/220711-ATSE-Explainer-energy-tech.pdf
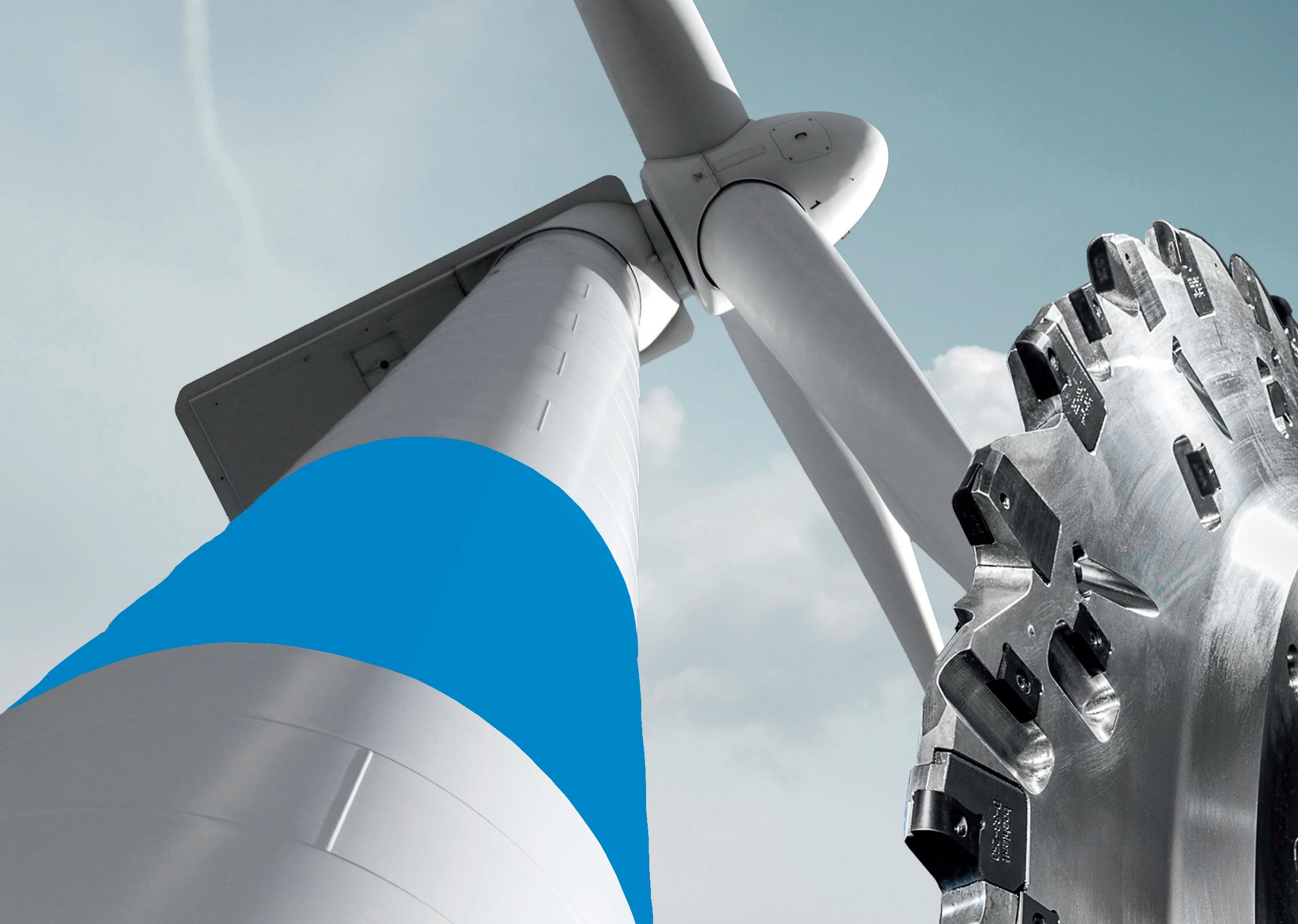
t is generally accepted that a wide range of sustainable and integrated energy sources are going to be needed to reach the greenhouse gas (GHG) targets set by the world community at COP meetings, in order to prevent global temperatures from rising to 2˚C above pre-industrial levels. To this end, the use of solar and wind power has increased dramatically over the last few years, but what happens at night time, when it is cloudy or the wind drops?
Step forward energy from within the Earth itself. Originating in the heat generated when the planet was first formed and constantly regenerating through radioactive decay, geothermal energy is always there and fully sustainable. It has been used as a local heat source for millennia, as well as more recently to generate electricity in places where there is a high heat flow close to the surface of the Earth (Figure 1).
But accessing geothermal energy need not be confined to these regions – in fact, there is potential for utilising it throughout the globe.
Heat from within the Earth
The temperature of the Earth’s subsurface rises with distance from the surface. This gradual change, known as the temperature gradient, is usually approximately 25˚C for each kilometre of depth, increasing up to temperatures in excess of 900˚C where rock may be in a molten state – magma. Near plate boundaries and volcanic centres, such as the Pacific ‘Ring of Fire’, magma rises towards the surface where it heats underground aquifers to temperatures of 350˚C or more and pressurised water escapes in the form of geysers, hot springs, and steam vents. These surface emanations have been utilised for decades in places such as Iceland, Italy, New Zealand, and California, the US (Figure 2). Naturally occurring hydrothermal fluids such as these can be used directly to heat buildings, greenhouses, and swimming pools, or, where hot enough, they can be used to produce steam for electrical power generation.
However, rather than rising to the surface, most of the heat remains locked in the Earth, and this is where its potential as a global sustainable energy source lies. Geothermal energy is now being developed in a variety of different geological settings throughout the world; all it requires is a system by which fluids heated within the subsurface can be accessed by drilling. These hot fluids may be naturally occurring water or brine, in pores and fractures in permeable rocks. If the hot rock does not contain enough natural cracks for the fluid to flow easily, it can be artificially fractured and a fluid circulation system developed. Sometimes the rock does not contain sufficient water to give commercially useful flow rates, in which case additional water can be pumped from the surface into the hot dry, fractured rocks, where it is heated by conduction. Once the hot fluid comes to the surface, whether by pumping or under natural convection, it can be used to produce steam for power generation, and in many cases the water cooled after use is pumped back into the aquifer to create a circular system (Figure 3).
Historically, geothermal developments were located close to surface expressions of natural hydrothermal geofluid circulation systems, often in igneous or metamorphic rock areas. However, recent advances in technology give increasing
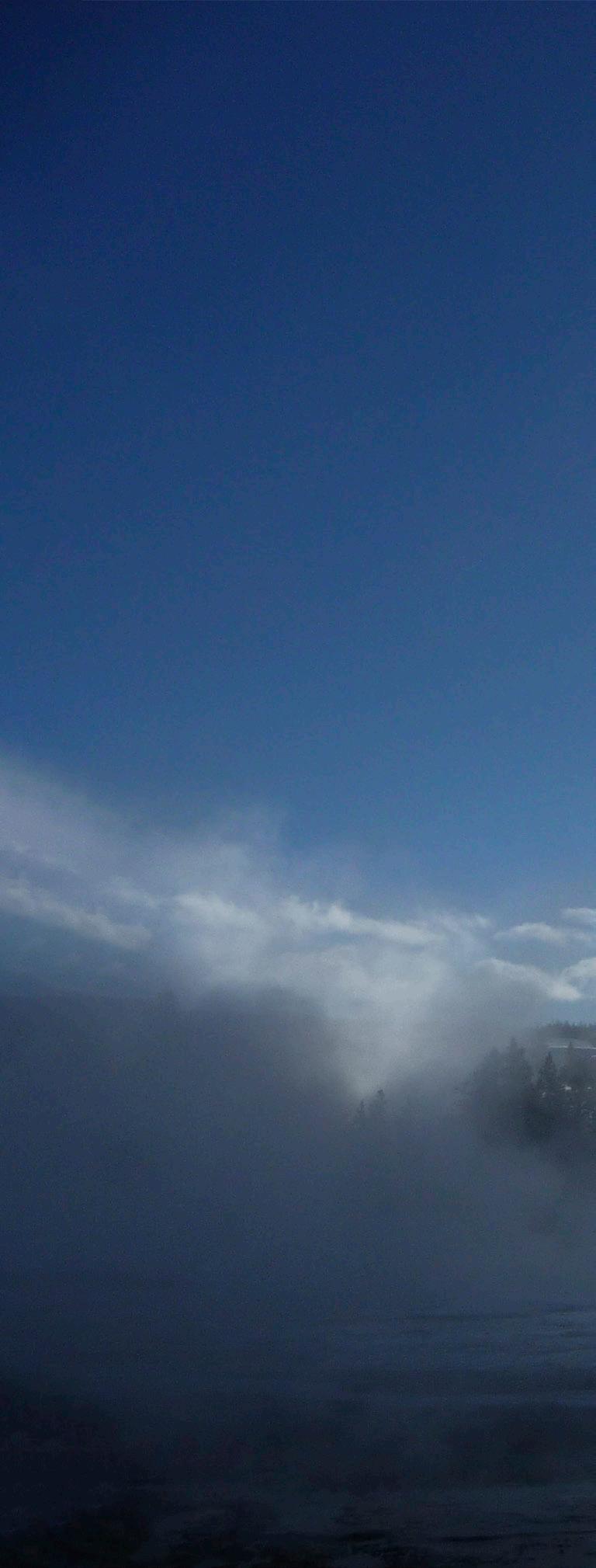
Dr
CGG, UK
looks at how geothermal energy, with the help of geoscience, can be accessed throughout the world – and how the add-on value chain is crucial to economically exploiting the resource.
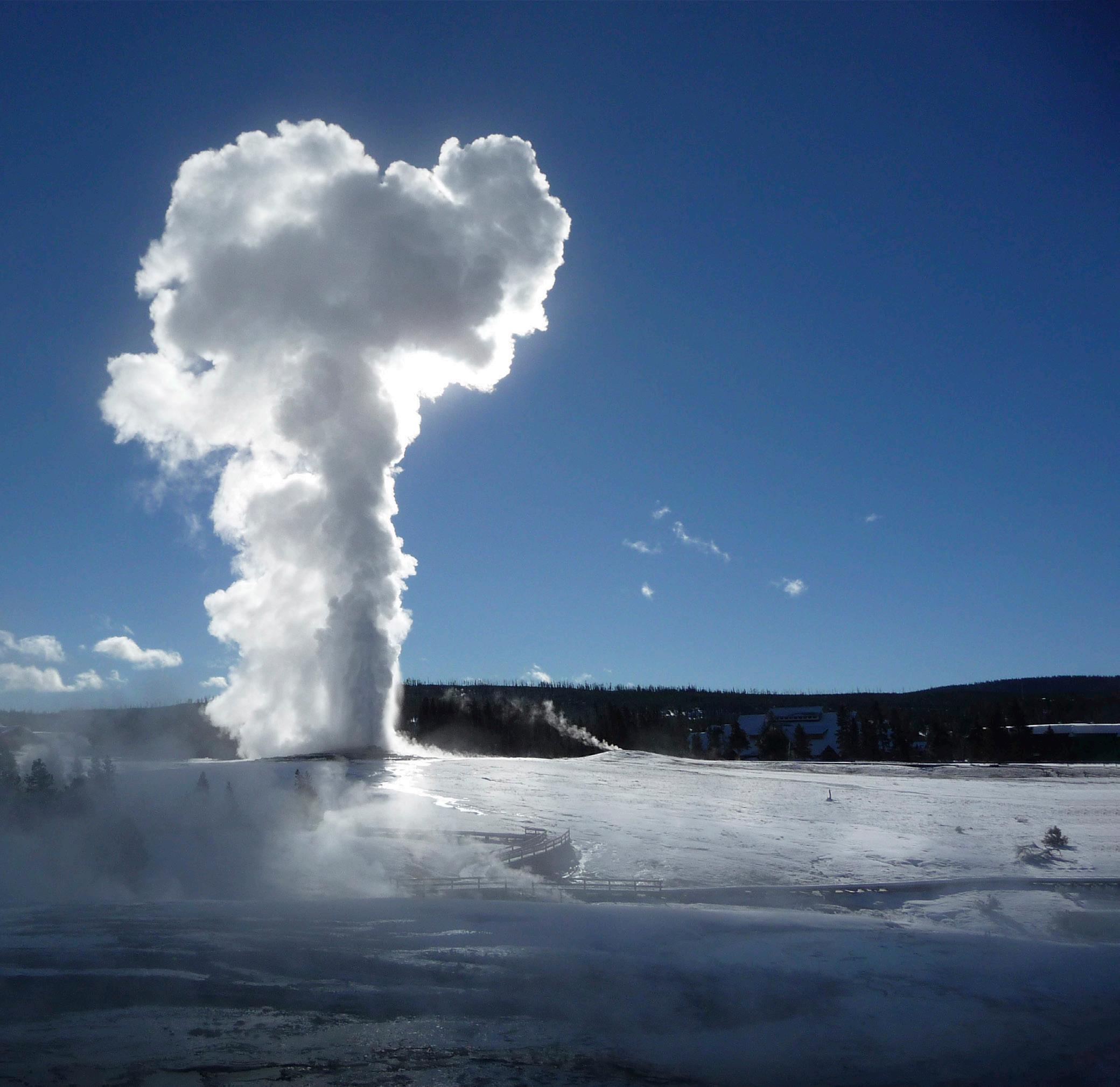
capability for finding hidden geothermal systems and for accessing geothermal energy from sedimentary basins.
Geoscience has the answers
Since this energy is based within the Earth, geoscience is the route to finding the optimum ways to access it. In order to do this, it is important to understand not just areas of high heat flow in the subsurface, but also how permeable and porous the rocks are and whether fluids will flow through them easily or if fracturing will be needed. Being able to recognise how rocks respond chemically and physically to heat and pressure, and how they change when fluids pass through them, makes it possible to assess how easy it will be to drill through them to access geothermal fluids.
Companies that have worked in the oil and gas industry for many decades have built up a valuable and detailed understanding of the Earth and its subsurface. One such company is CGG, a global geoscience technology and HPC leader that has been collecting and interpreting geoscientific data for over 90 years. Its geoscientists can draw on their knowledge, skills, and technologies to bring valuable intelligence and capabilities to help better understand and de-risk the development of geothermal energy throughout the world. Over the last 20 years, for example, CGG has undertaken more than 150 geothermal projects, mostly applying geophysical technologies such as analysis of magnetotellurics, gravity, and microseismicity in traditional areas such as the ‘Ring of Fire’, but latterly also helping companies explore and develop ‘hidden’ geothermal resources using these techniques (Figure 4).
Over the years, CGG geoscientists have collected extensive databases of the important parameters that need to be understood in oil and gas exploration, and many of these, such as temperature gradients, porosity, permeability, fluid chemistry, and flow rate, are also essential for geothermal projects. All this data has been merged with the company’s extensive seismic
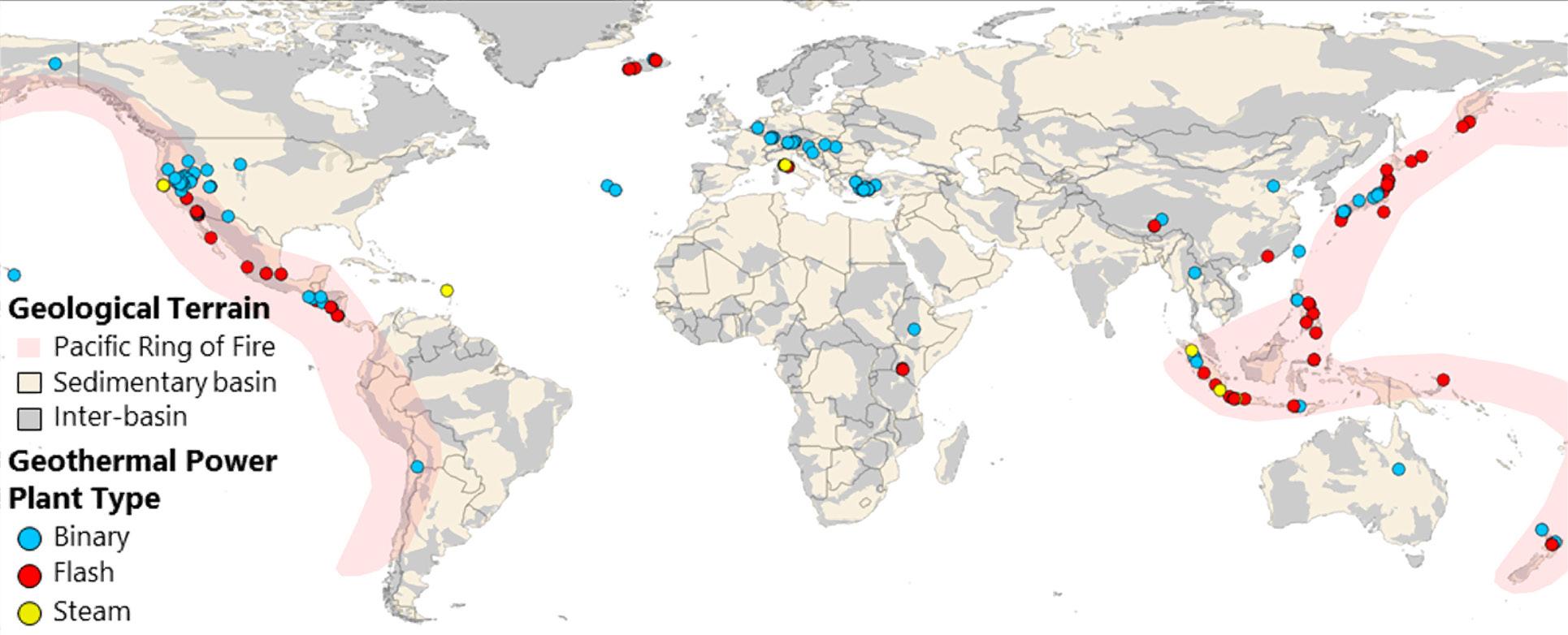
library to develop a geoscientific understanding of the Earth which can help identify new potential areas for the development of geothermal energy projects. Using this information, CGG recently completed a global Geothermal Resource Assessment study that can be used not only to identify and assess potential promising geothermal energy sites, but also to analyse the nature of the geothermal reservoir rock and provide information on production and monitoring solutions (Figure 5).
Sedimentary basins have been a major focus for the oil and gas industry, but they are of interest to the geothermal industry, not only because over the years a great deal of geological knowledge has been gathered about them, but because, unlike volcanic areas, they are often close to population centres and therefore any geothermal resource developed in them will have a ready market. The aquifers of the Paris Basin, for example, have been providing district heating for over 700 000 people for more than 30 years. However, key properties of the producing layers, such as distribution of porosity and permeability, which will identify the rocks with high rates of fluid flow that will make future development of this resource more effective, remain poorly understood. To reduce this uncertainty, a recent study by CGG, using established oil and gas techniques such as seismic inversion and recently developed rock physics-guided deep neural networks, was able to characterise the reservoirs and guide the location and design of future geothermal wells.
In oil and gas field areas, the percentage of water co-produced from the subsurface reservoir increases as the hydrocarbons are extracted. This water, which is usually discarded or pumped back into the reservoir, can be at temperatures high enough to be used directly for heating or in some cases to generate electricity. There are some technical challenges – the higher flow rates needed for useful geothermal energy production may need a wider
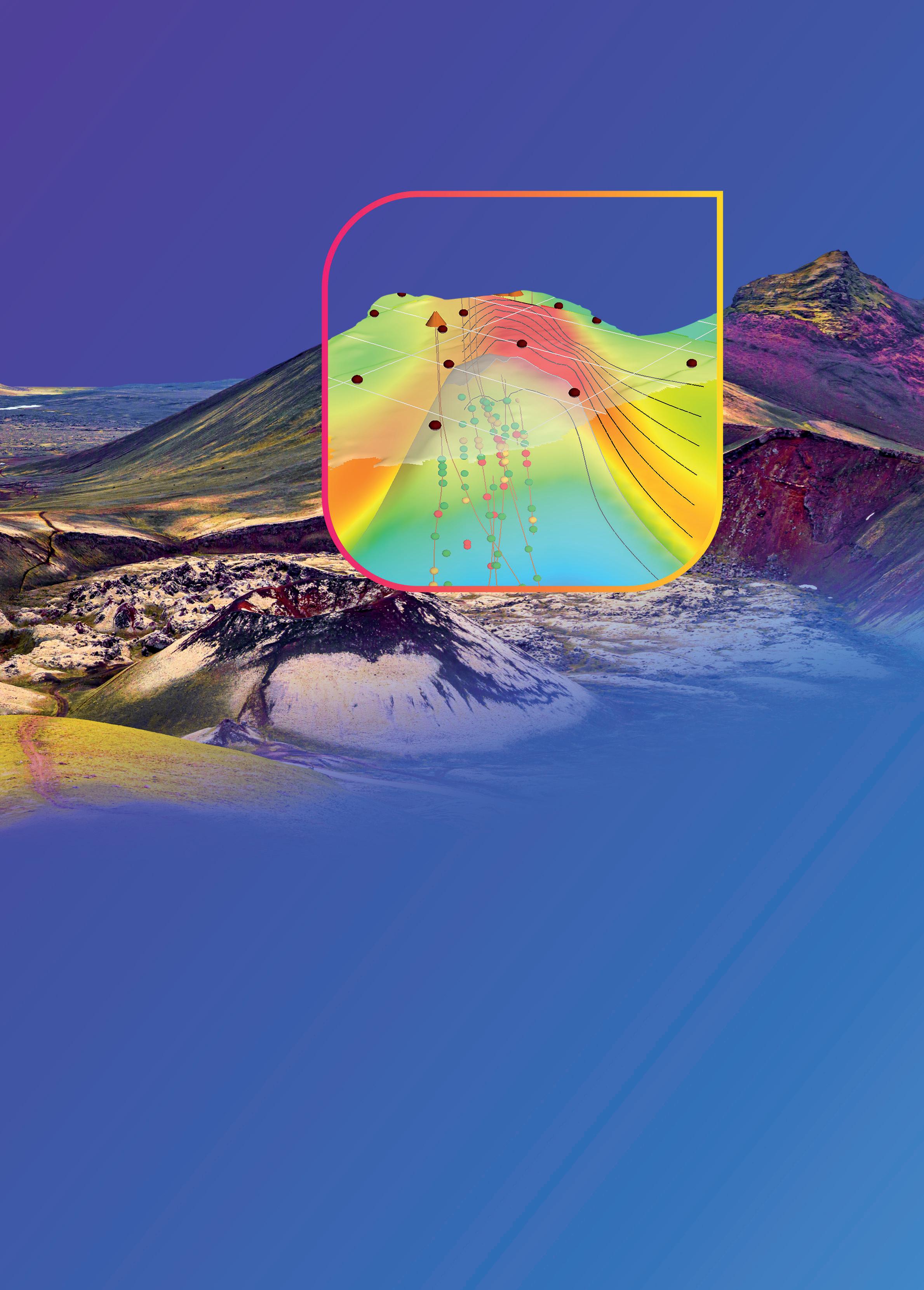
diameter borehole, for example – but it is a promising and potentially cost-effective development.
The geothermal value chain
However, accessing the natural heat of the Earth is not just about producing heat to generate electricity; there is abundant thermal energy in the fluid remaining after driving the turbines. The value of this remaining thermal energy varies with climate: 40˚C water would, for example,
provide much more additional heating in the Arctic than in the Tropics.
If the geothermal resource is hotter than approximately 120˚C, it can be converted directly to electricity using flash, steam, or binary turbine systems and supplied to the grid. As the water cools, it can be recycled, with cascading uses as the temperature drops. Geothermal fluids of less than 120˚C can be used directly to heat or cool residential and industrial premises, for some manufacturing processes, and in agriculture and fish farming, all without access to a power grid or without imposing extra demand on an existing power grid. Other uses for thermal energy include drying industrial cement and aggregate and for pulp, paper, and food processing.
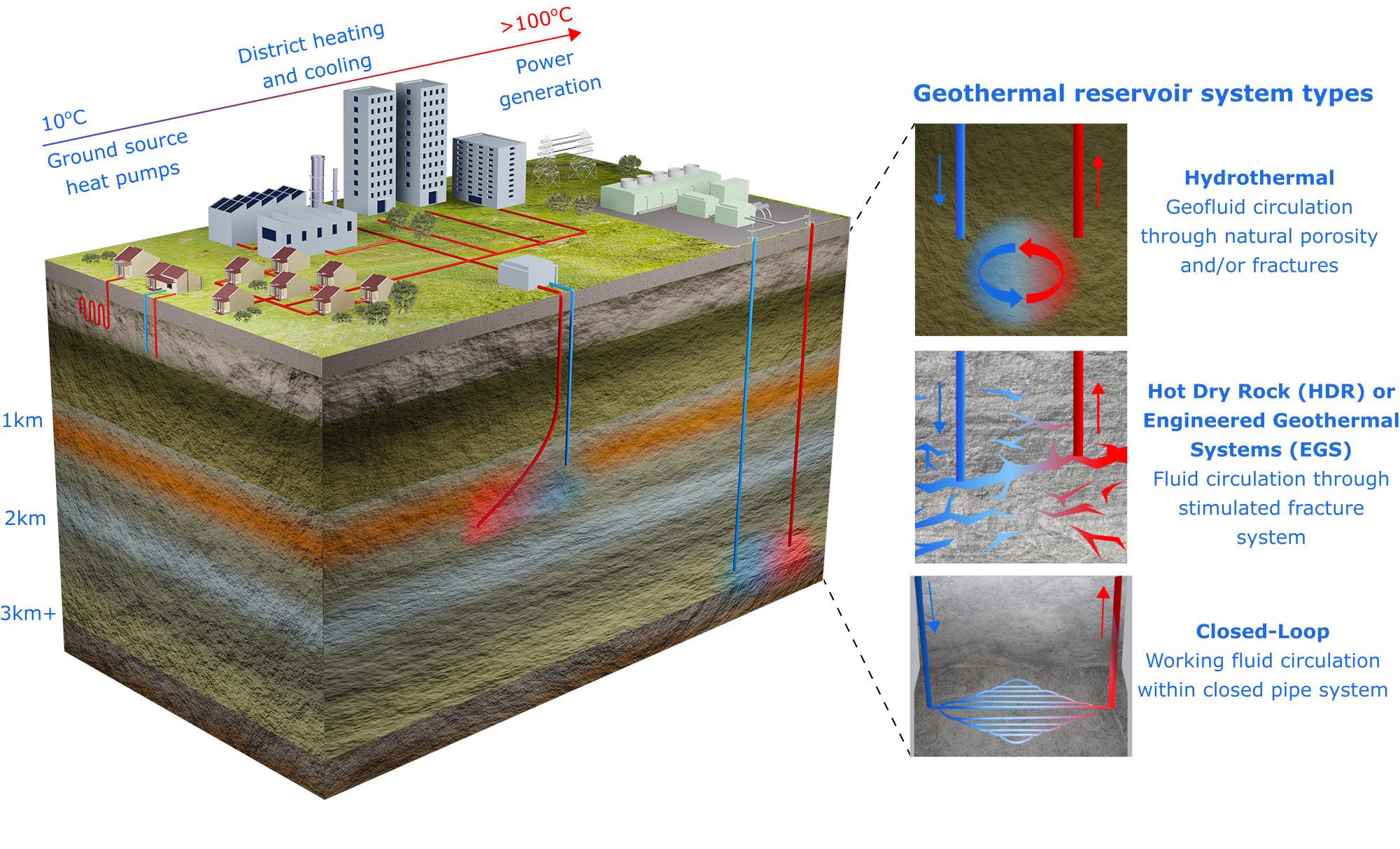
Since approximately 40% of carbon released into the atmosphere currently comes from domestic and industrial heating and cooling, switching as much as possible to geothermally sourced heat makes sense, through individual ground source heat pumps and on an industrial scale. China is a leader in the field of geothermal direct heat; Sinopec Green Energy has built 719 heat centres in China and drilled over 700 wells. It annually produces 15 MWe, and estimates it has saved 16 million t of CO 2 emissions since it started in 2006.1
After passing through flash or steam turbines, the steam is normally condensed to water for re-injection into the reservoir to maintain geothermal fluid pressures and flow rates. However, if alternative sources of injection water are available, the condensate can be used directly with minimal further treatment as potable water. In coastal arid environments, geothermal energy can be used in desalination plants, to preheat the saline water prior to final desalination using gas, for example.
As with wind, solar, and nuclear, geothermal electrical energy can be used to generate green hydrogen through hydrolysis of fresh water. The freshwater condensate from flash or steam geothermal turbines provides a self-contained hydrogen-generation system, whereas the other energy sources would need an external source of fresh water – a potential problem in water-stressed areas.
A potentially very important economic benefit from geothermal projects is in critical mineral production. When brines are trapped at high pressure deep in the Earth, they often have high concentrations of minerals, including critical elements such as lithium and manganese, as well as rare earths, platinum group metals, and arsenic, all of which are in high demand for the production of batteries
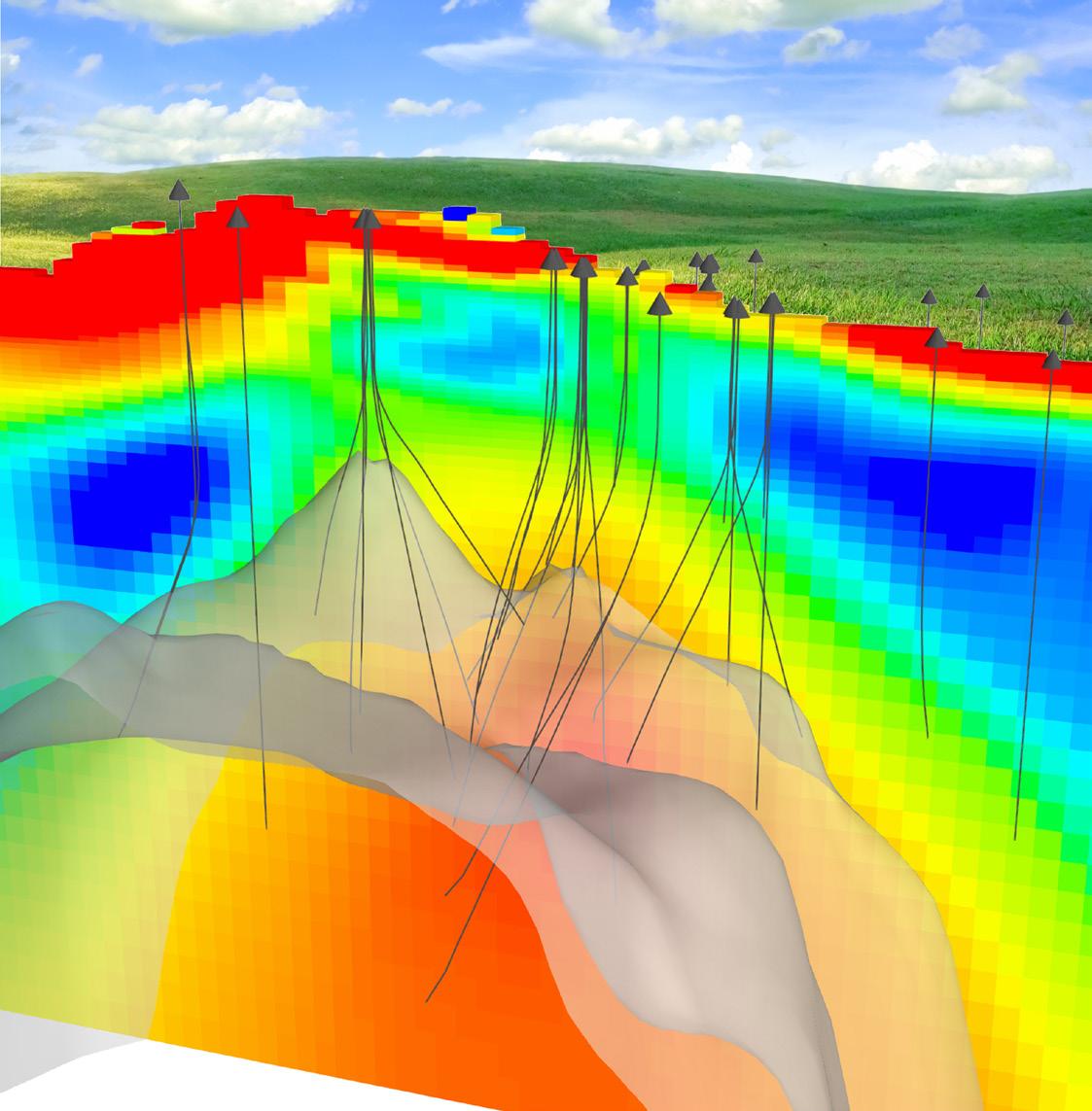
and electronics and the construction of wind and solar farms. If these critical minerals can be economically extracted and purified from the brines, geothermal projects would become more cost-effective at a wider range of locations.
Considerable research is being undertaken on this idea in, among other places, the Salton Sea, a shallow, saline lake in southern California, the US, that lies on the San Andreas fault and is an area of high geothermal activity, where there are already a number of producing geothermal power stations. Several of these are working on direct lithium extraction from the hot brine residuals from the power plants, and it has been estimated that the 11 existing geothermal plants along the Salton Sea alone could have the potential to produce enough lithium metal to provide approximately 10 times the current US demand. 2
Since at the moment the value of lithium is 6 – 8 times greater than that of geothermal power, mineral extraction from brines could be the key to making geothermal projects more economic. A knowledge of the subsurface and constituents of geothermal waters may be key to unlocking this promise, and this can be provided through CGG’s Lithium Brine Screening study, which evaluated more than 250 000 data points and 27 000 lithium measurements to create a comprehensive and consistent water chemistry database, supplemented by key engineering and geochemical characteristics (Figure 6).
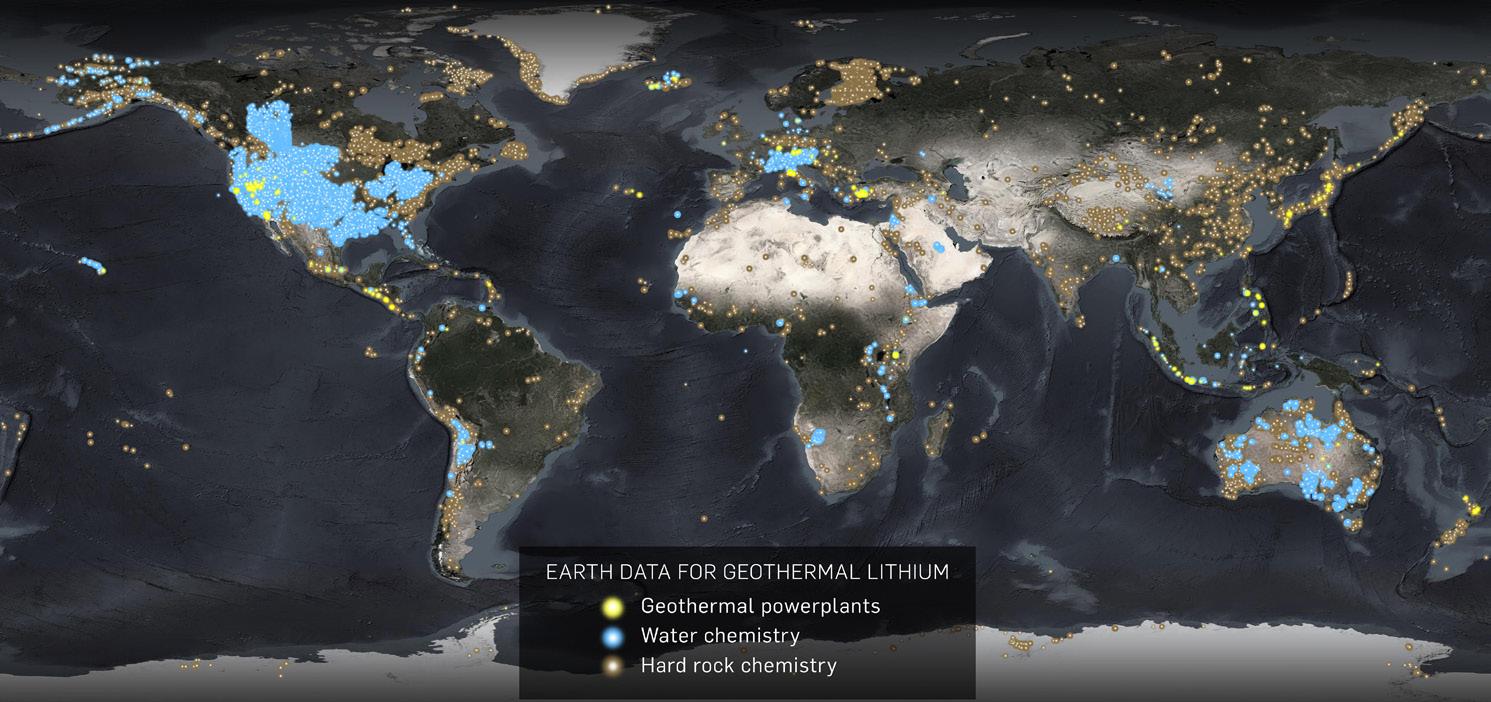
This cascading chain of additional uses and products is an important aspect of geothermal projects, as they can deliver more than electrical energy alone.
Decades of cumulative expertise
The capabilities, technologies, skills, and understanding acquired by CGG over many years is relevant to geothermal projects because the exploitation of a geothermal project follows a similar trajectory to that of an oil or gas discovery and uses similar technologies. Initially, research may be on a global scale, aided by products such as the global Geothermal Resource Assessment study and then, akin to the exploration phase of an oil and gas project, it will move to a more regional scale, where subsurface knowledge from CGG’s seismic databases
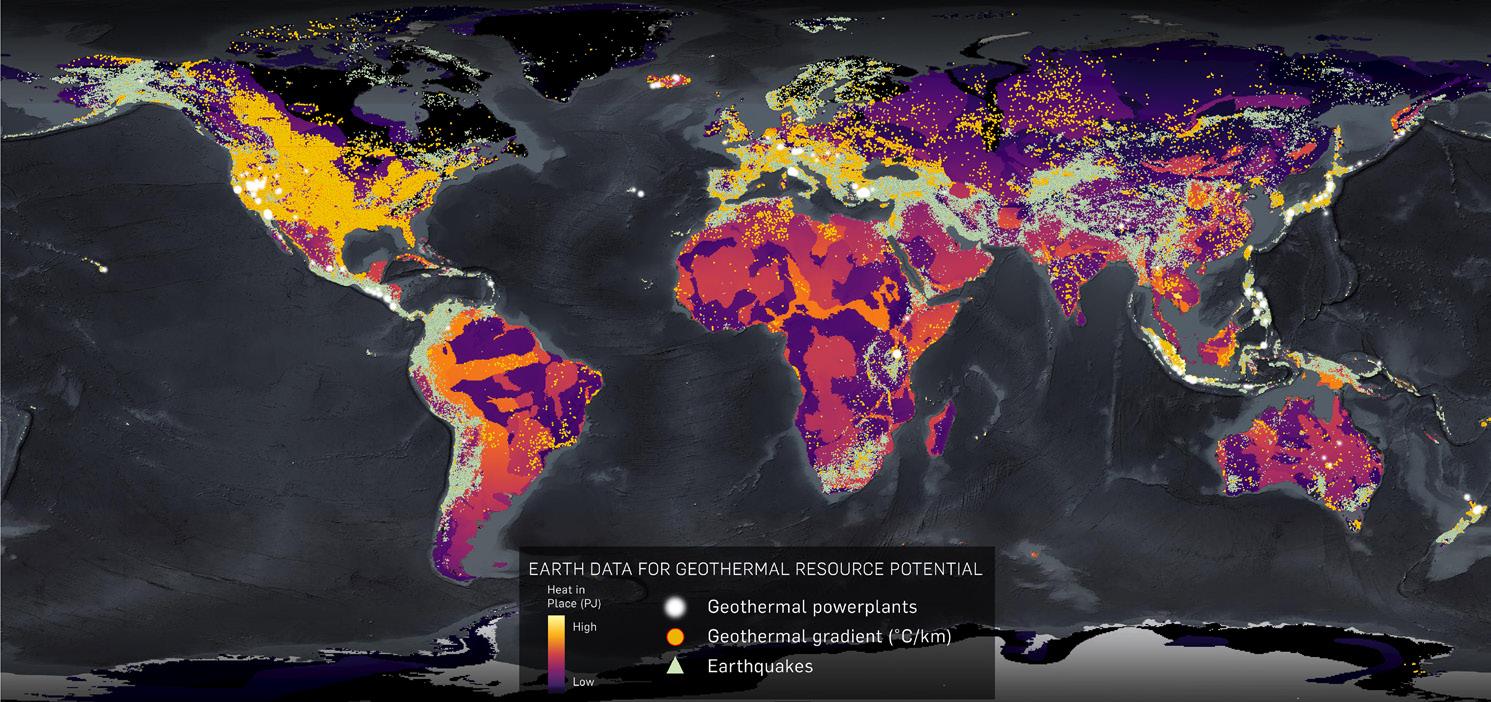
will be crucial. Having identified a promising area, the project would progress to the appraisal stage, where, to understand the reservoir and predict what the resource will be, a detailed knowledge of the rock properties is key. This is followed by the development stage, in which detailed 3D structural models based on seismic, other geophysical technologies, and well data are used to ensure optimum well placement into the aquifer. Once a geothermal project is underway, reservoir modelling tools and seismic are used to monitor what is happening underground and to help to understand the flow of the fluids in the subsurface.
Therefore, to ensure geothermal projects can take full advantage of the value chain and minimise subsurface risks, it is vital that there is a good understanding of the subsurface using geoscience knowledge, including the databases, technologies, skills, and experience developed over many decades by companies, such as CGG.
References
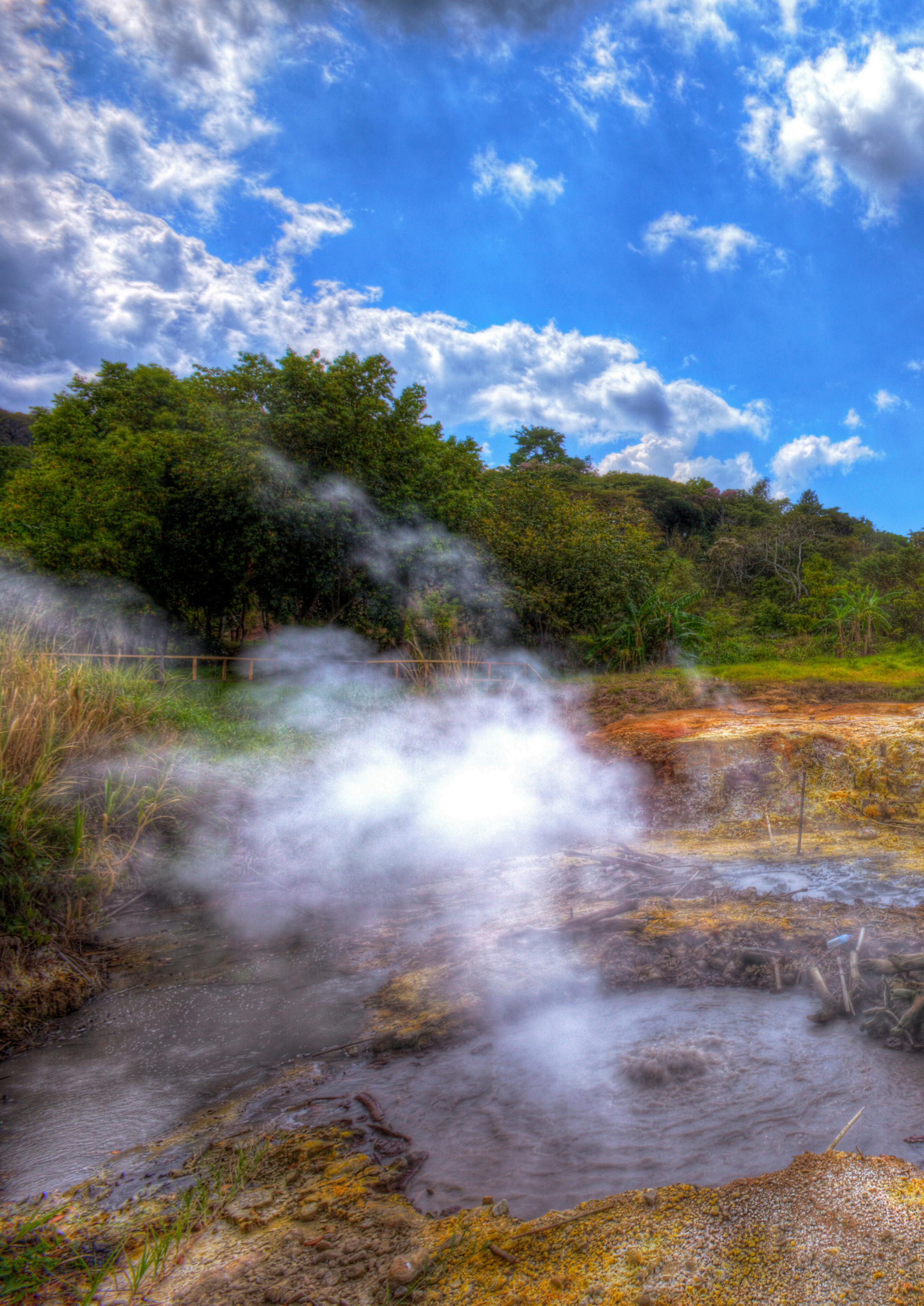
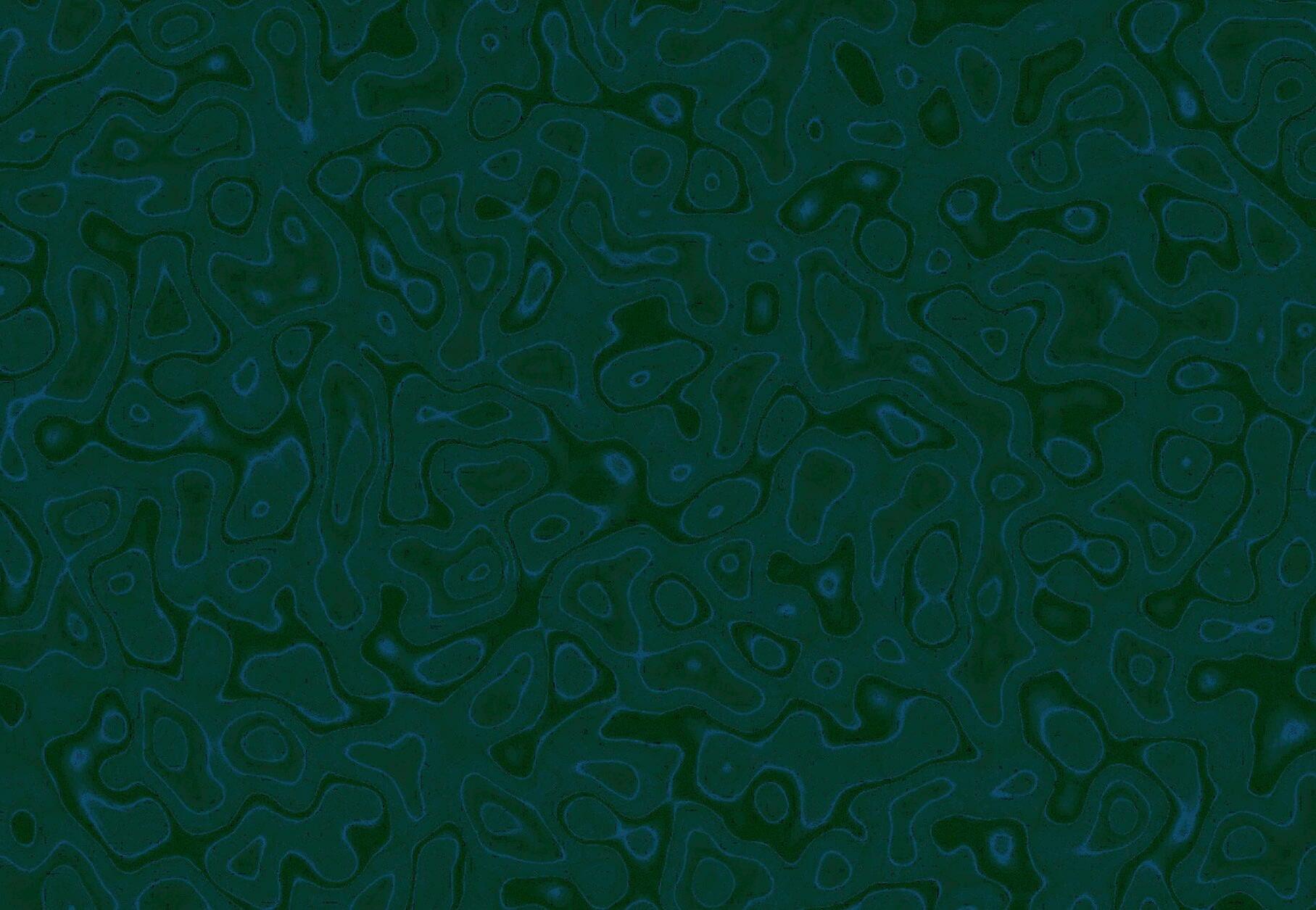
Taylor Mattie, Director of Geothermal Technologies and Innovations, Baker Hughes, USA, explores geothermal power in the context of offtakers.
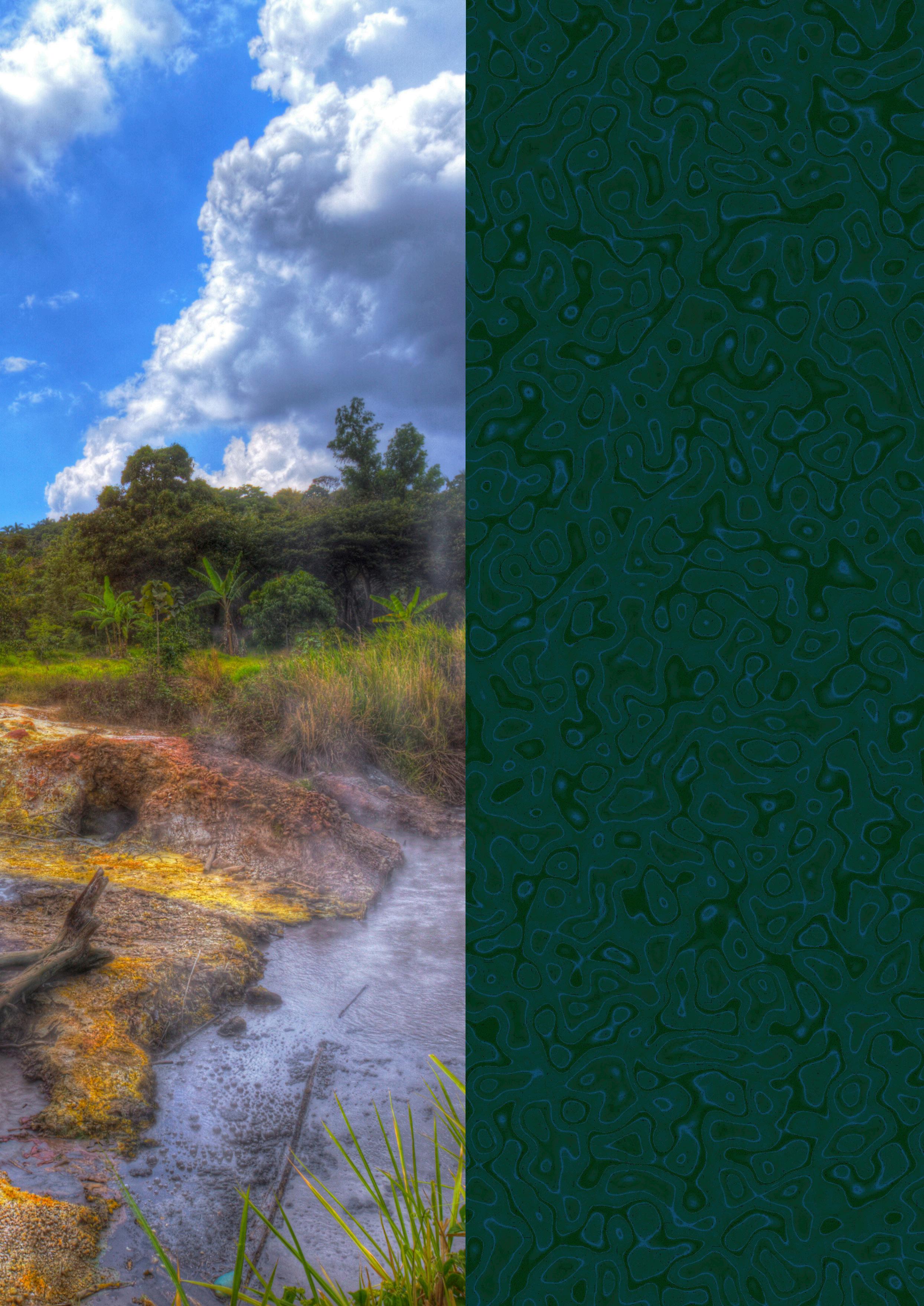
One would be hard pressed to find an industry today that is not actively working to decarbonise and participate in the global energy transition. While many may focus on wind, solar, and battery storage as
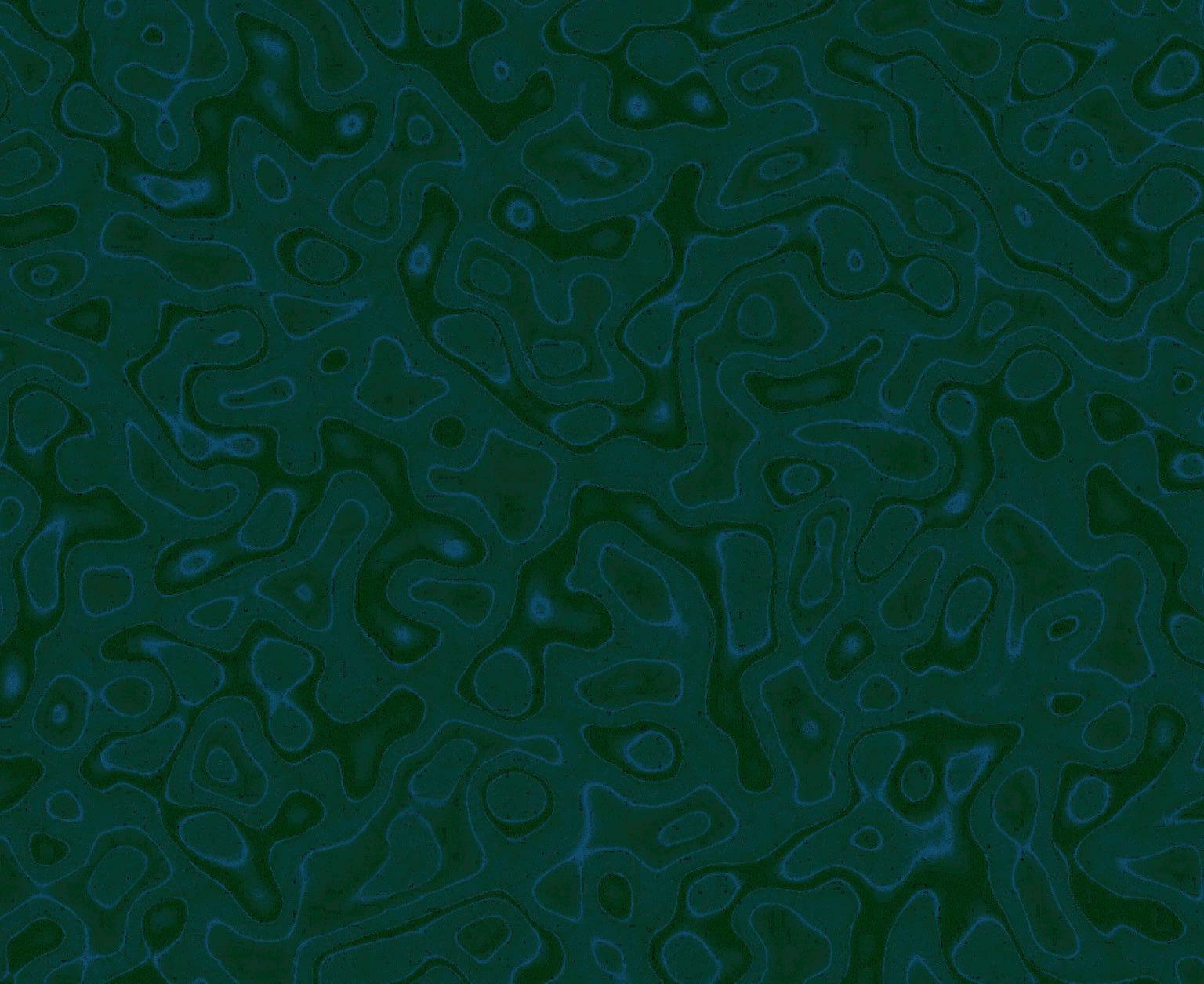
possible replacements for fossil fuels, other solutions are often overlooked.
One such example is geothermal energy, which uses the vast energy of the Earth in the form of heat for power generation or heating and cooling. It has been used to generate power for nearly 100 years and counting, and Baker Hughes has been supporting this energy source in various capacities for nearly half that period.
Using the heat generated within the Earth means geothermal is always on, making it a baseload energy source. Unlike wind and solar, it is producing energy more than 95% of the time and is indifferent to the sun shining or the wind blowing. Its continuity and reliability of supply and availability are significant advantages. Consider too,
most geothermal systems today generate zero carbon emissions and take up a fraction of the surface footprint compared to wind and solar generation sites.
There are barriers to developing geothermal, however; projects require high levels of up-front capital investment – typically between US$2 – US$7 million per well. Even though those costs can be recouped over the long term, operators and investors want a quick payout or to reduce costs and eliminate as many risks as possible. Developers and investors want assurance that the energy produced will be purchased if a geothermal plant is constructed. This is known as the offtaker.
Geothermal and the digital world
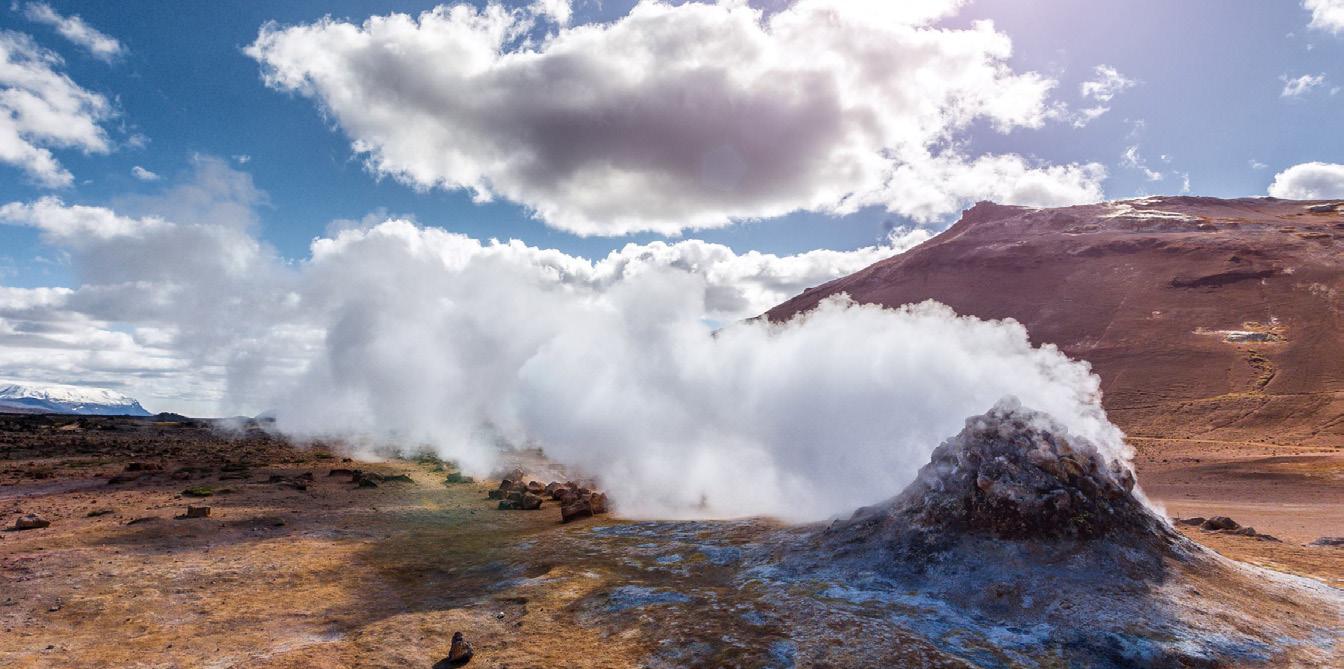
In the past few years, however, a new type of geothermal power offtaker is dawning on the horizon: datacentres and crypto farms. These differ from traditional power and heat purchasers due to both the massive volumes of energy required to run them and the nature of the facilities themselves. Here, geothermal is used as a zero-carbon fuel and enabler for the digital world. And the digital world is energy-hungry.
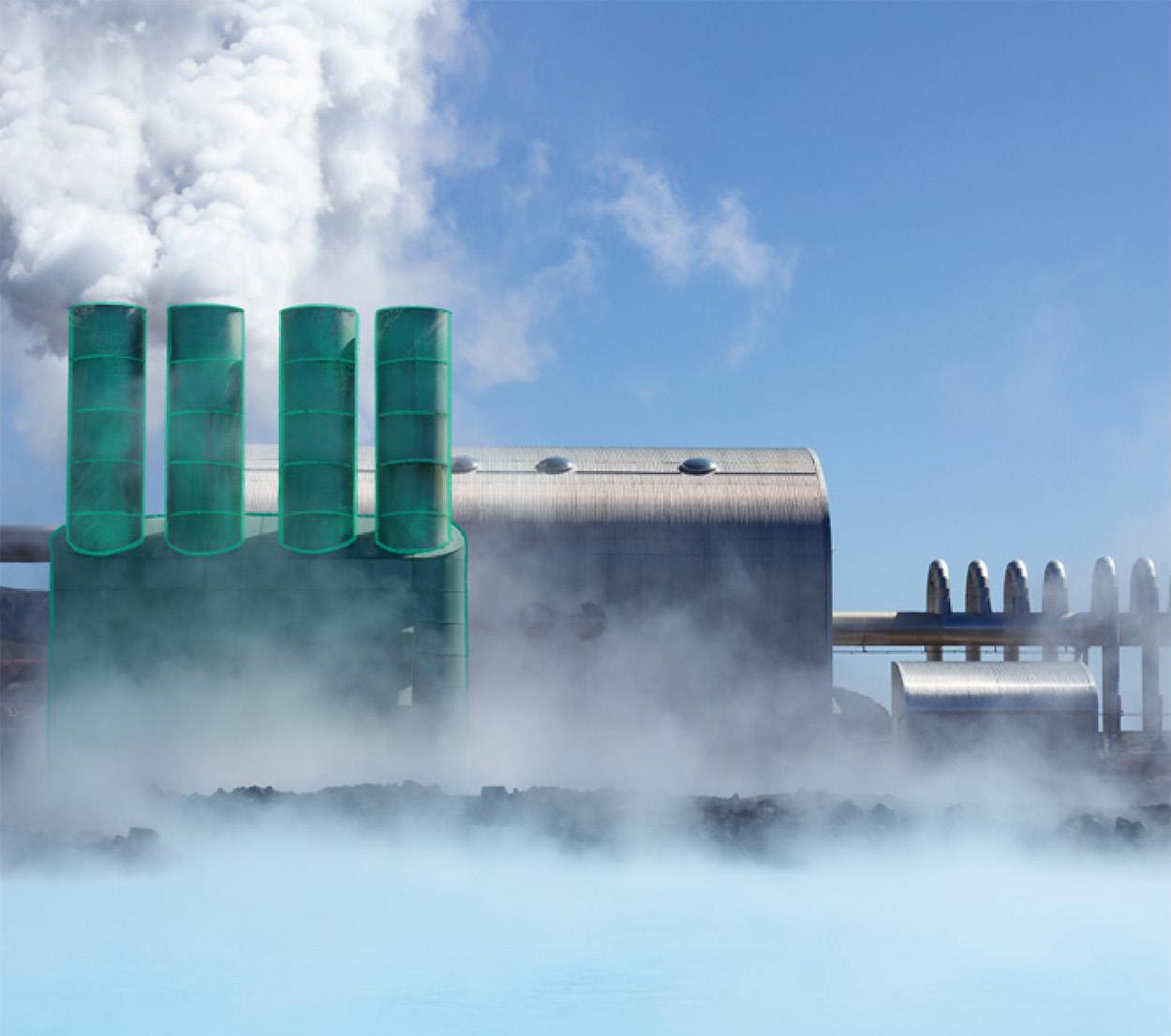
Figure 1 While many focus on wind, solar or batter storage, geothermal is often overlooked as an alternative energy source.
Large datacentres being planned today have power demands that can range up to 300 MW, or roughly the equivalent demand of 225 000 – 300 000 average households. Looking forward, the trend is to further leverage economies of scale and deploy even larger installations.
There are a number of operational datacenters and crypto mining facilities already using geothermal for their energy needs – some of which were developed with the support of Baker Hughes and its bespoke technology portfolio.
Case study: Iceland and El Salvador
As early as 2014, crypto currencies, such as Bitcoin, were being mined in Iceland, where the combination of cool, year-round temperatures and an abundance of geothermal energy create ideal conditions for low-carbon power and the need for server cooling. In fact, so many datacentres are rushing to take advantage of these conditions that some in the tech industry are calling it a ‘digital arms race’.
Figure 2 Using the Earth’s own heat means geothermal energy is ‘always on’ and not dependent on the wind blowing or the sun shining.
Baker Hughes is well-positioned to assist in this effort, with an historic presence in Iceland, in addition to a portfolio of technology for geothermal well drilling and construction. As part of this portfolio, the company has
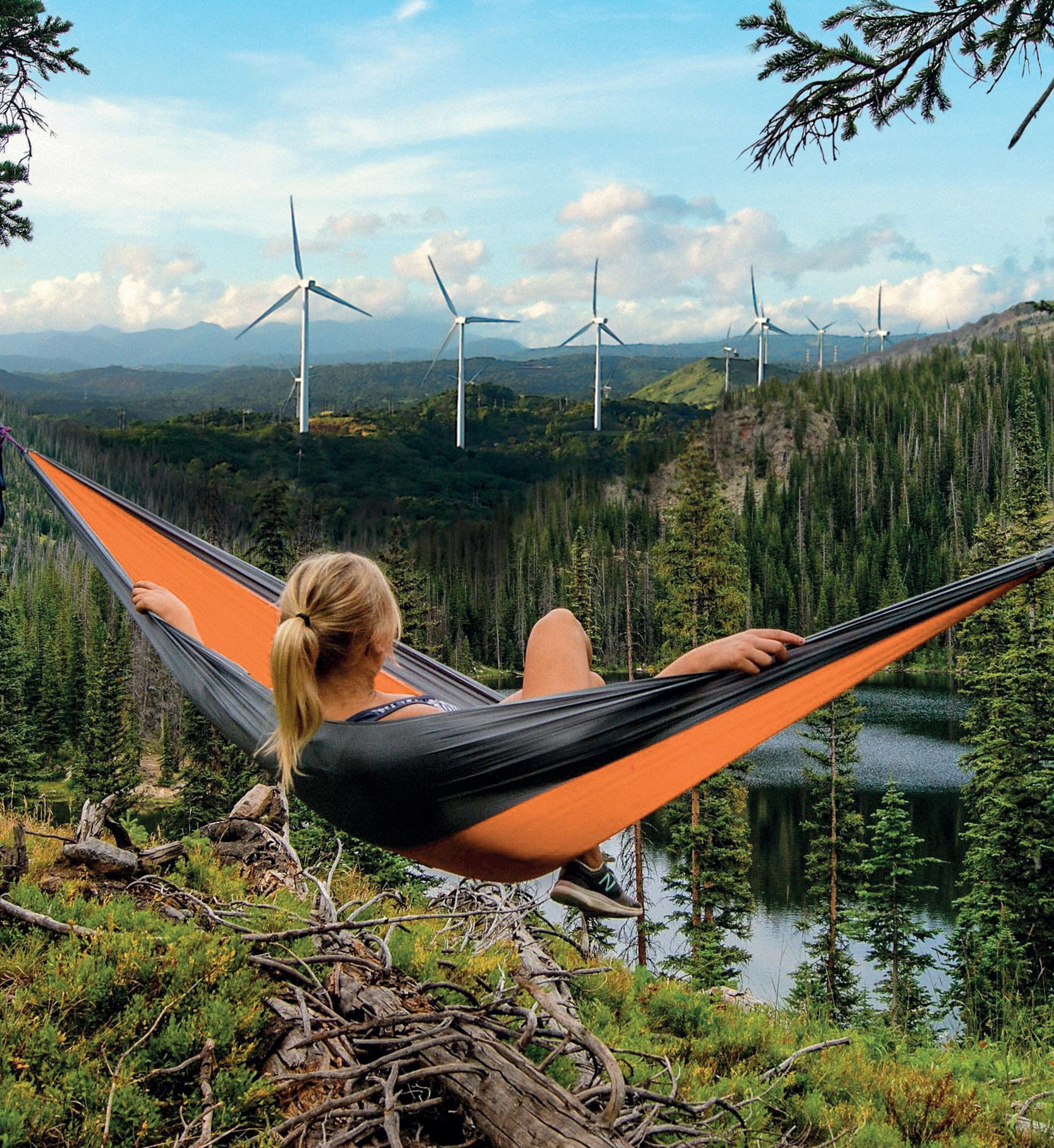
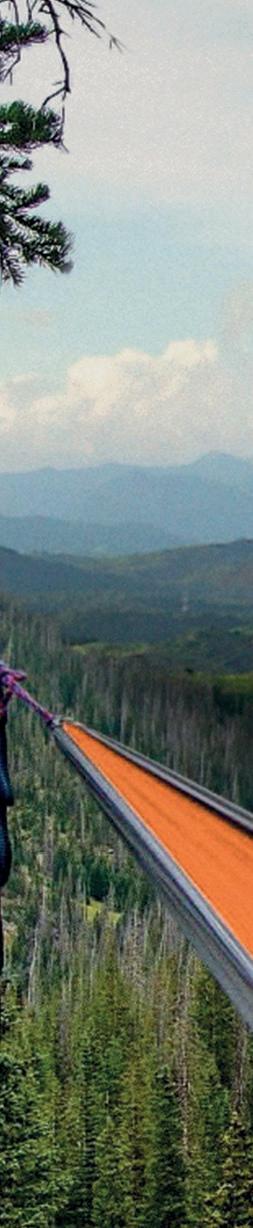
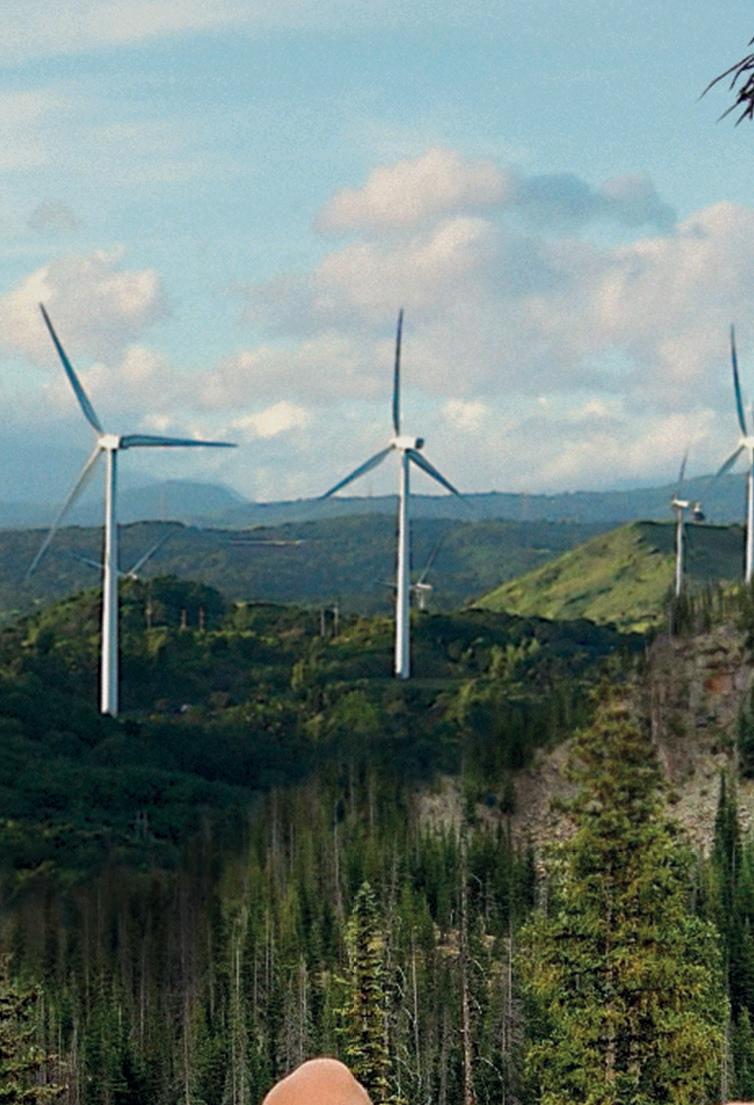
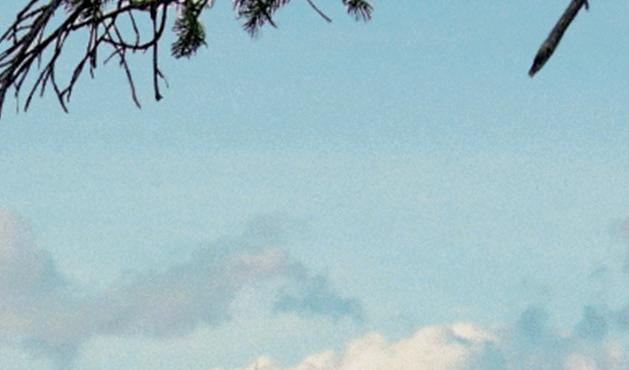

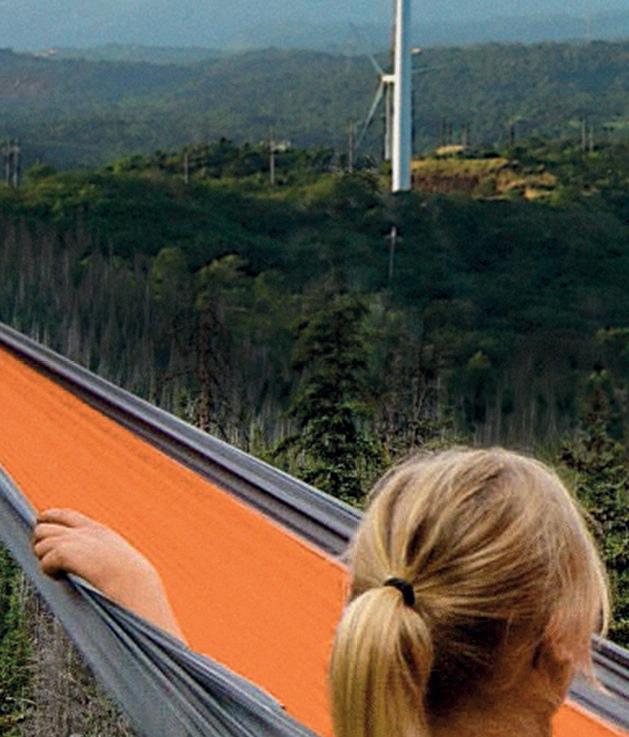
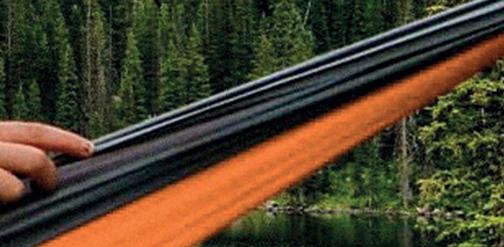

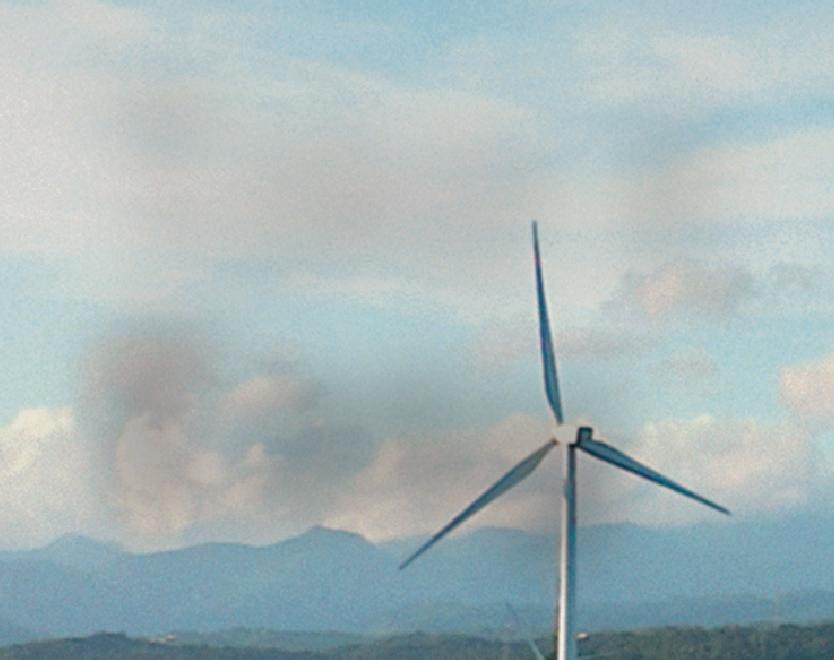
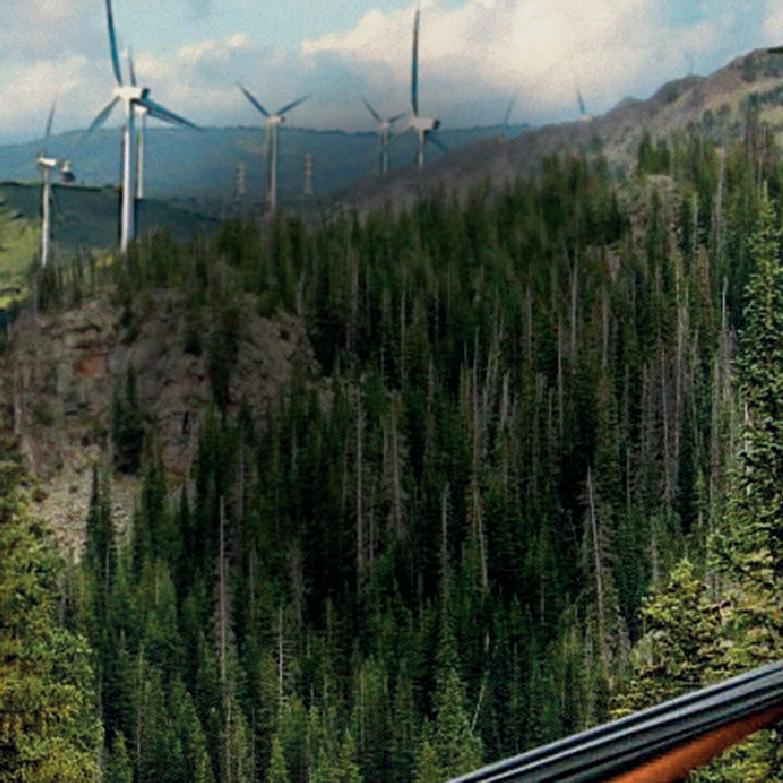
drilled a well for the Icelandic Deep Drilling Project 2, which is the hottest and deepest geothermal well ever drilled in Iceland.
While Iceland’s cool climate is highly advantageous when it comes to cooling servers, it is not a requirement. For example, on the opposite end of the spectrum, there has been significant crypto currency mining in El Salvador, powered by geothermal energy. Specifically in the region around the Tecapa volcano, a number of Bitcoin mining operations are active, using geothermal power produced by the 109 MW Berlin geothermal field. In 2021, El Salvador’s president announced a new project called ‘Bitcoin City’, which will be developed at another volcano approximately 100 km from Berlin. The Berlin geothermal power plant has been using a flash steam turbine from Baker Hughes since 2004.
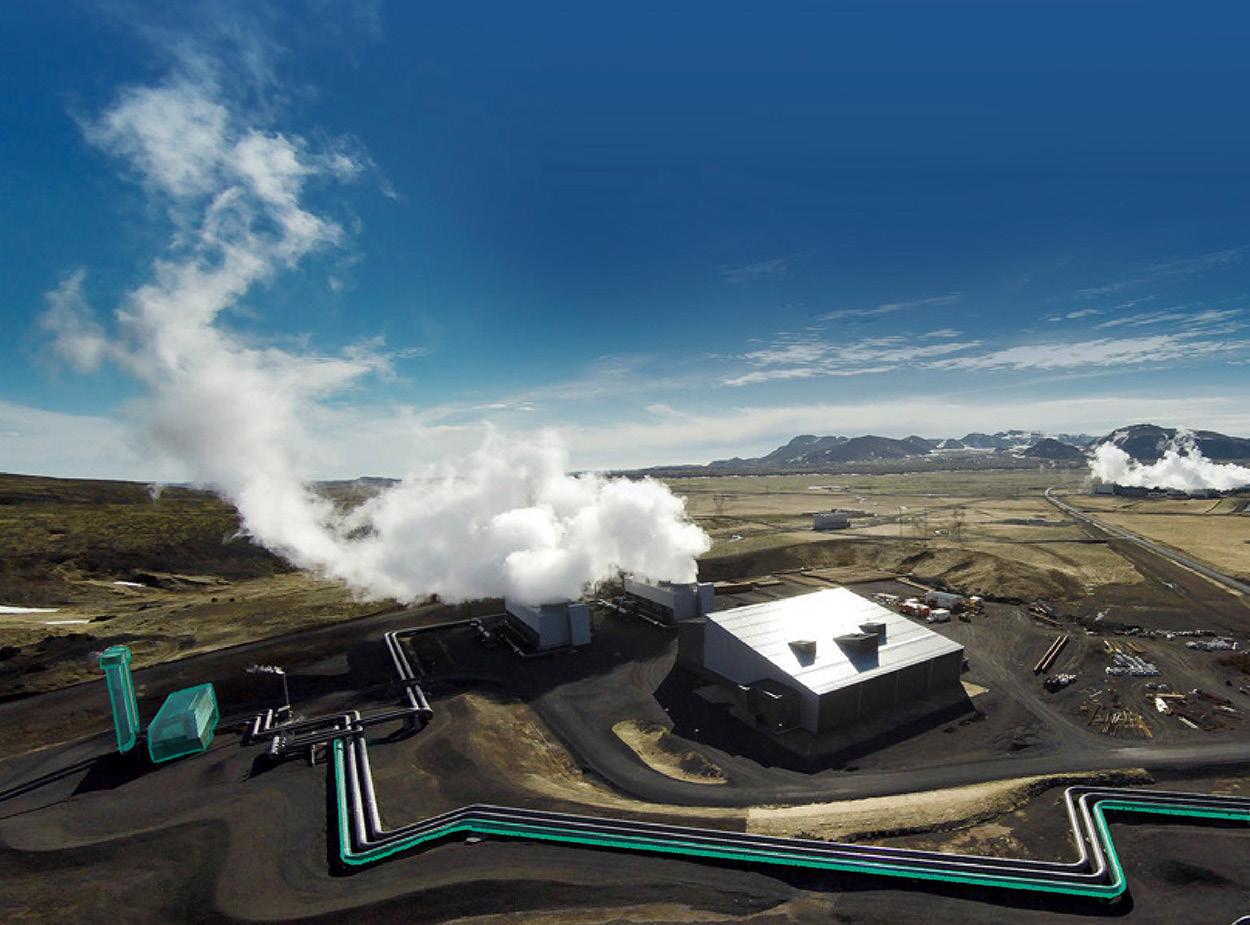
Utilising renewable energy
When considering the wider topic of using renewable energy for datacenter or crypto farming, the key to success is secure, reliable, and competitively priced power. There have been some attempts at vertically integrated wind, solar, or hydroelectric power plants for 100% offtake for datacentres. But delivering this key element to the
table is difficult and costly. The capacity factor of these technologies can barely reach 50%, unless a costly storage system is built as well.
Since geothermal is a baseload energy source with more than 95% capacity, a vertically integrated power solution is highly attractive, as it can bring competitive, consistent, renewable power to the datacentre.
Baker Hughes has developed a unique geothermal solution, as its modern technology and capabilities range from reservoir engineering to an understanding of the geothermal resource, to the drilling and construction of wells, to accessing the resource, to the turbines needed for power generation. This portfolio stretches across the entire scope of geothermal project development and minimises the cost, risk, and time required to get the power or the heat online.
Conclusion
Overall, the use of geothermal for datacentres and crypto mining is still considered new, but as more players in this space work to achieve their net zero carbon emission goals, this is an area of the market that is projected to grow rapidly.
Figure 3 . Geothermal energy has been used to generate heat and power for more than 100 years.•
•
Benefits Challenges and Solutions
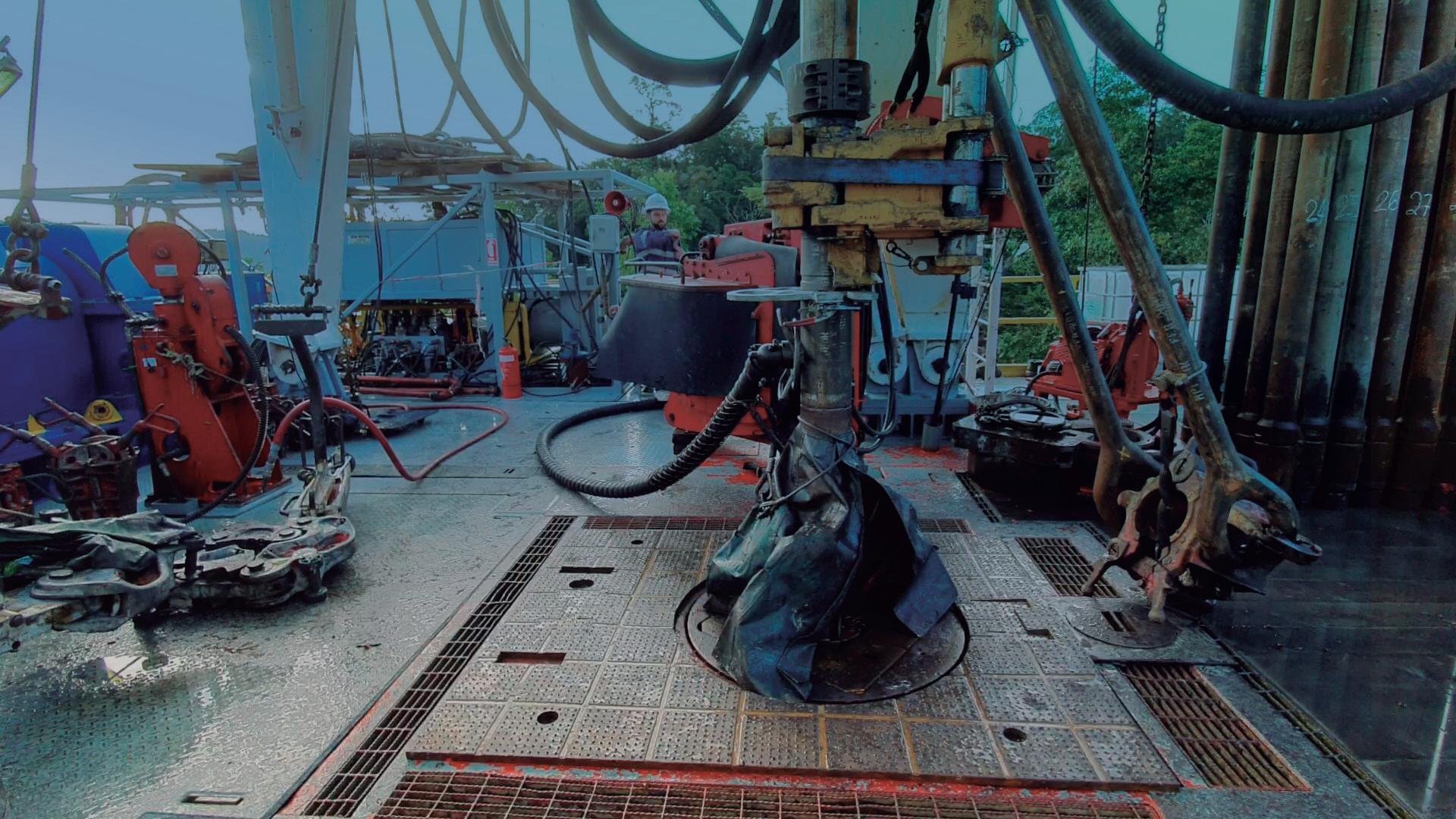
•
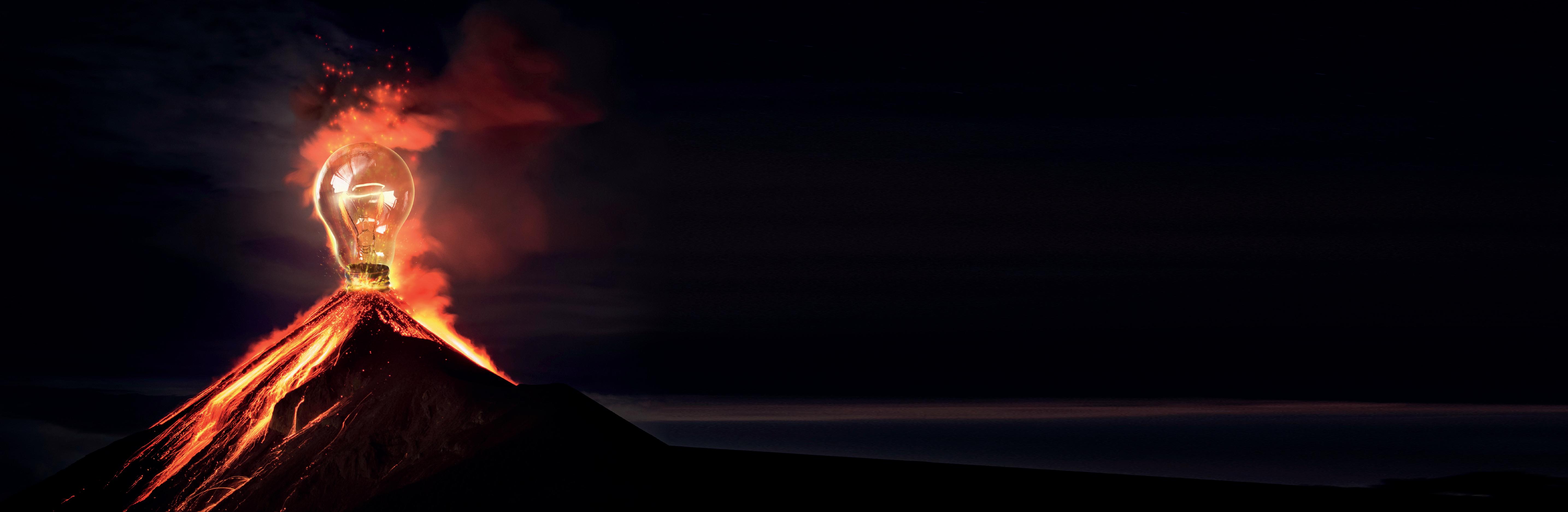
n these challenging and unpredictable times – climate change, rising energy costs, financial instability, and the uncertainty of the geopolitical landscape – the energy the world uses and the security around this has become ever more important.
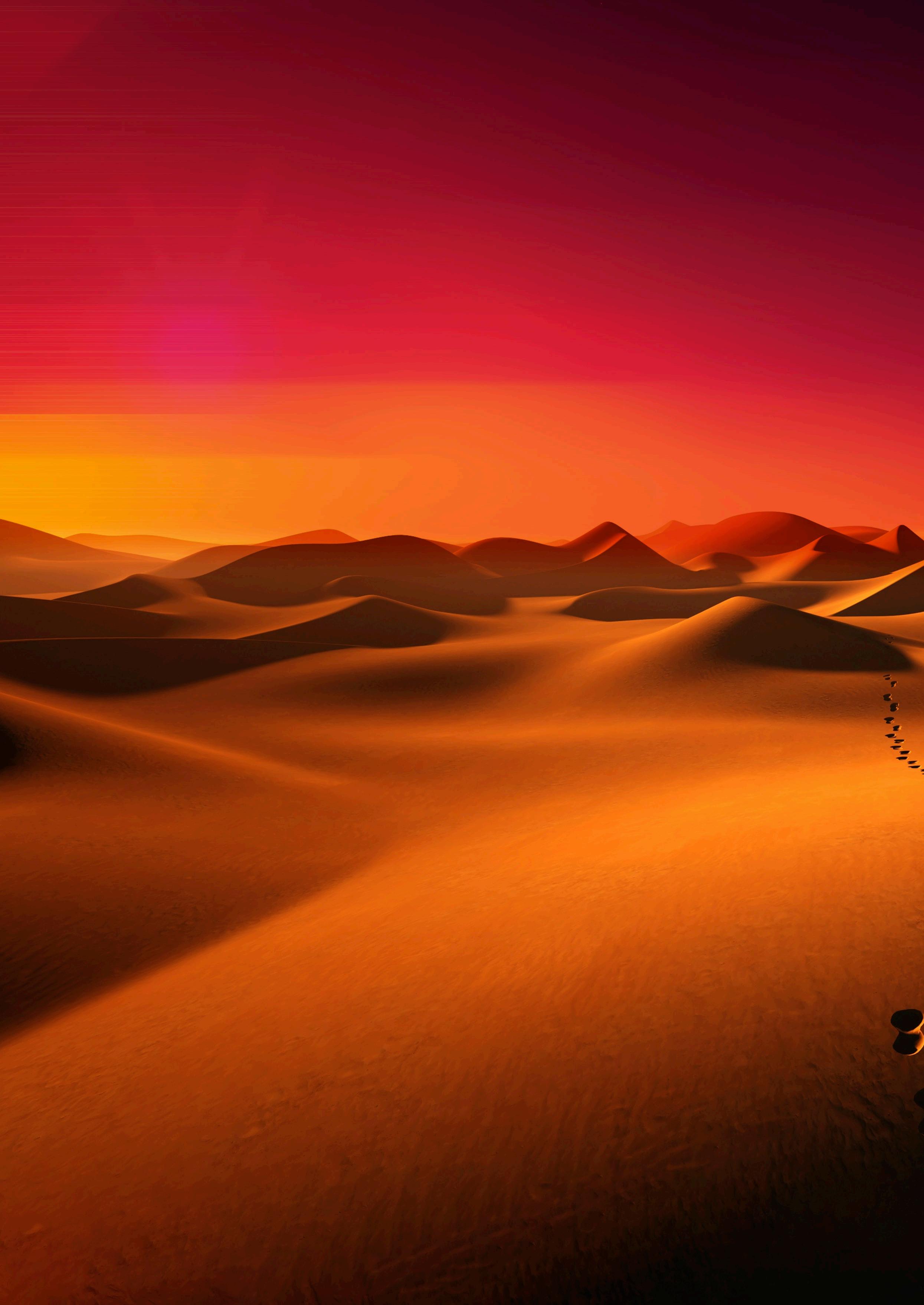
Geothermal as an energy source has been available for centuries, and is the only true baseload energy. Available everywhere and anywhere – and with 99% of the Earth’s volume having temperatures exceeding 1000˚C – geothermal is a baseload energy, meaning it provides 24/7 energy and can be viewed as one giant battery with enough clean renewable energy to help meet the 2050 Paris Agreement’s net zero targets, and to potentially last billions of years.
What is geothermal energy, and how does it fit into the energy mix?
To meet the Paris Agreement targets and set a global warming target of 1.5˚C, global energy use must transition from oil and gas to cleaner and more sustainable energy sources.
Realistically, no one source of renewable energy can completely replace the current energy supply from
Karl Farrow, Founder and CEO of CeraPhi Energy, UK, talks about the opportunity geothermal and gas sector could be harnessedgeothermal presents as a viable and realistic part of the energy mix, and how the skill set of the oil harnessed in this transition.
fossil fuels such as oil and gas – and it is a blend of all energy sources that will enable this transition to happen. But, what needs to be grasped is that geothermal energy is always available, unlike solar and wind (which are discontinuous and require ancillary systems to operate), or biomass and hydrogen (which require feed stock from fossil fuel or cultivated source, in turn having a damaging environmental impact). Furthermore, a key benefit of geothermal is that it provides heat directly.
Geothermal energy is heat created from radioactive decay penetrating through the sub-surface of the earth.
Different geothermal technologies with distinct levels of maturity exist. Technology for direct use including district heating, geothermal heat pumps, greenhouses, and other such applications are widely used and already considered mature. Technology for electricity generation from hydrothermal reservoirs has been used commercially for more than 100 years, and is completely reliable. Yet, despite the staggering amount of geothermal energy contained within the Earth’s sub-surface, only 16 GWe of energy is currently being commercially produced from this source globally.
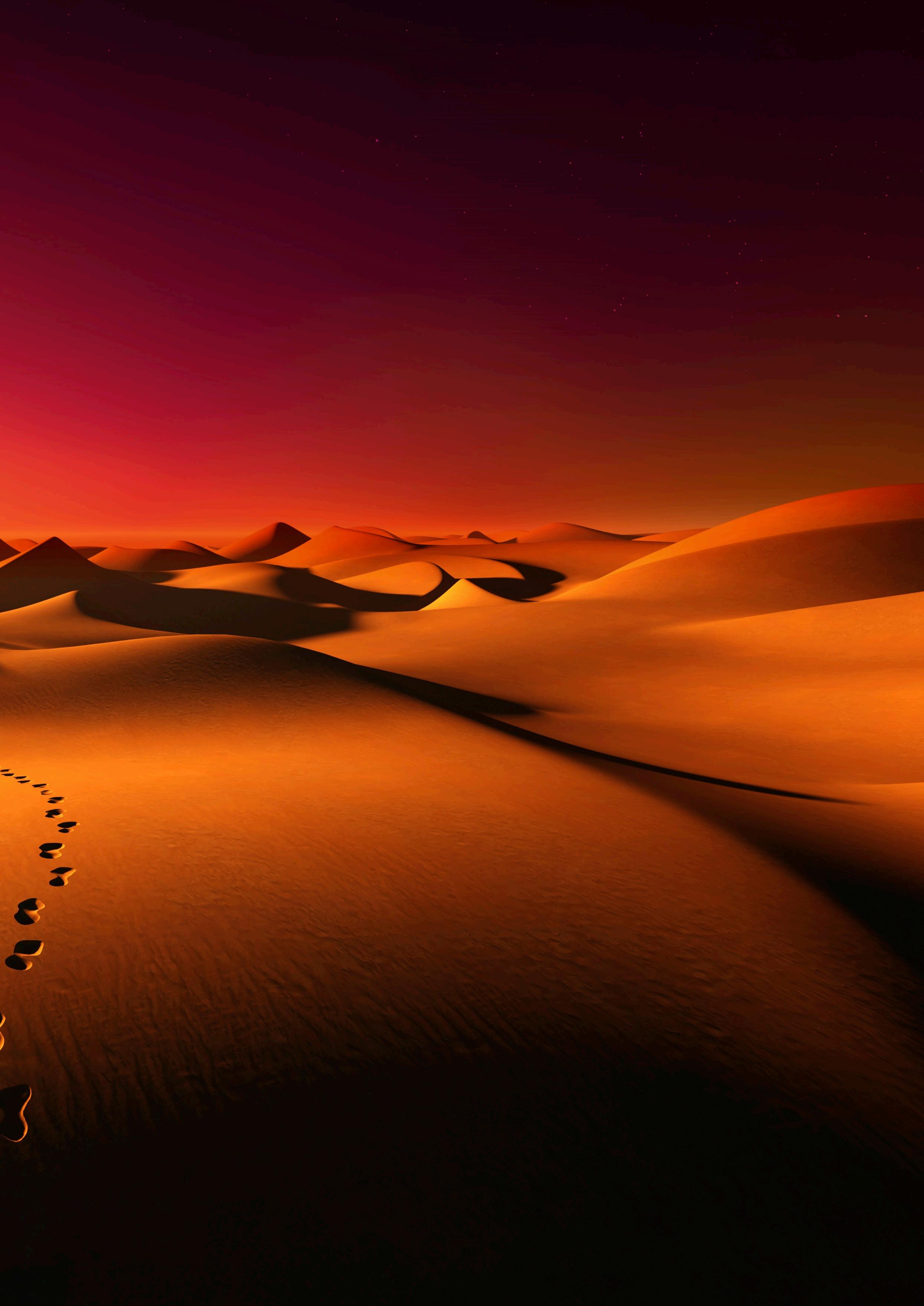
Many of the world’s continents are already utilising geothermal energy to meet their energy needs and there is, undoubtedly, huge potential in this sector; however, the UK and the world needs to ramp up use of this viable and natural energy resource in order to remain serious about hitting the global environmental targets.
How can the transition be achieved?
There are two strands to energy transition:
F The movement of energy resourcing from non-renewable energy resources to renewable energy solutions.
F The repurposing of oil and gas wells for geothermal, and the utilisation of an already highly-skilled existing oil and gas work force who can seamlessly make the transition into the geothermal energy space.
There are currently an estimated >20 million oil and gas wells across the world, with an estimated 3.2 million located in the US. Many of these are end of life and non-producing. Acknowledging there are some differences between oil and gas and geothermal energy production – geothermal requires deeper accessibility and functions at higher temperatures – the expertise and services from the oil and gas sector can access it easily.
Couple this with the existence of an already skilled work force, in the hundreds of thousands of people who work in the global oil and gas sector, and the geothermal energy industry has a ready-made, hugely skilled work base that already uses much of the technology and work practices that would seamlessly transition into this renewable energy space.
What does the future look like for geothermal energy?
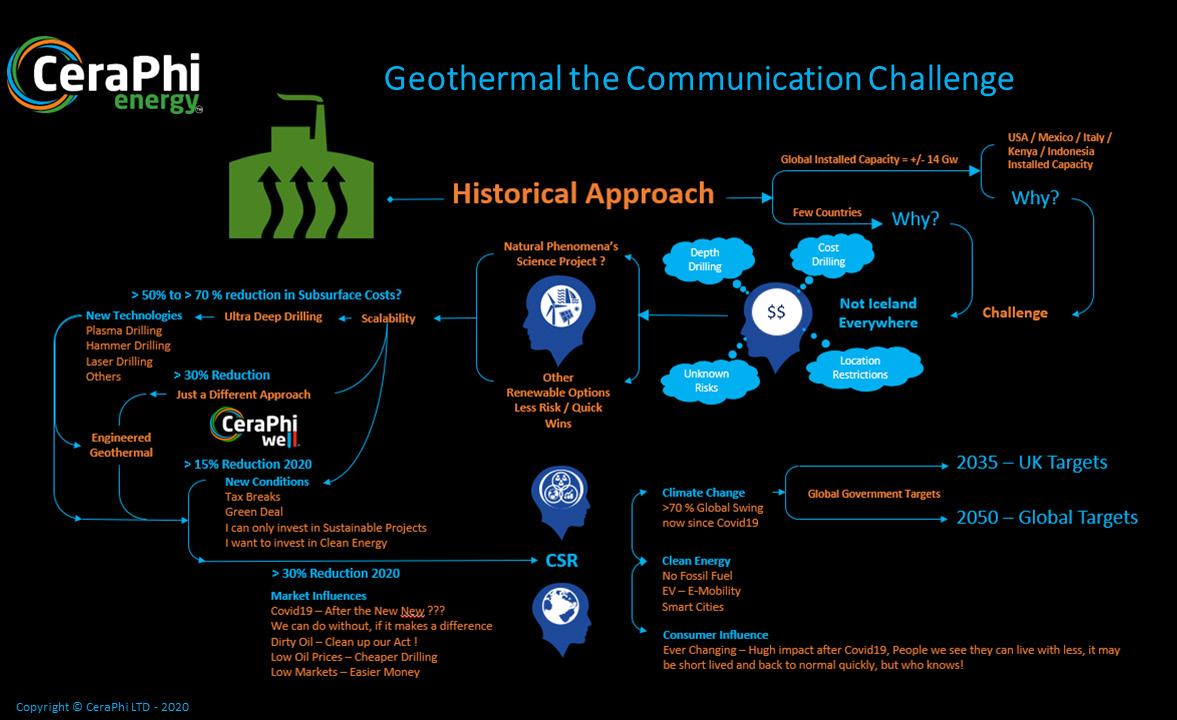
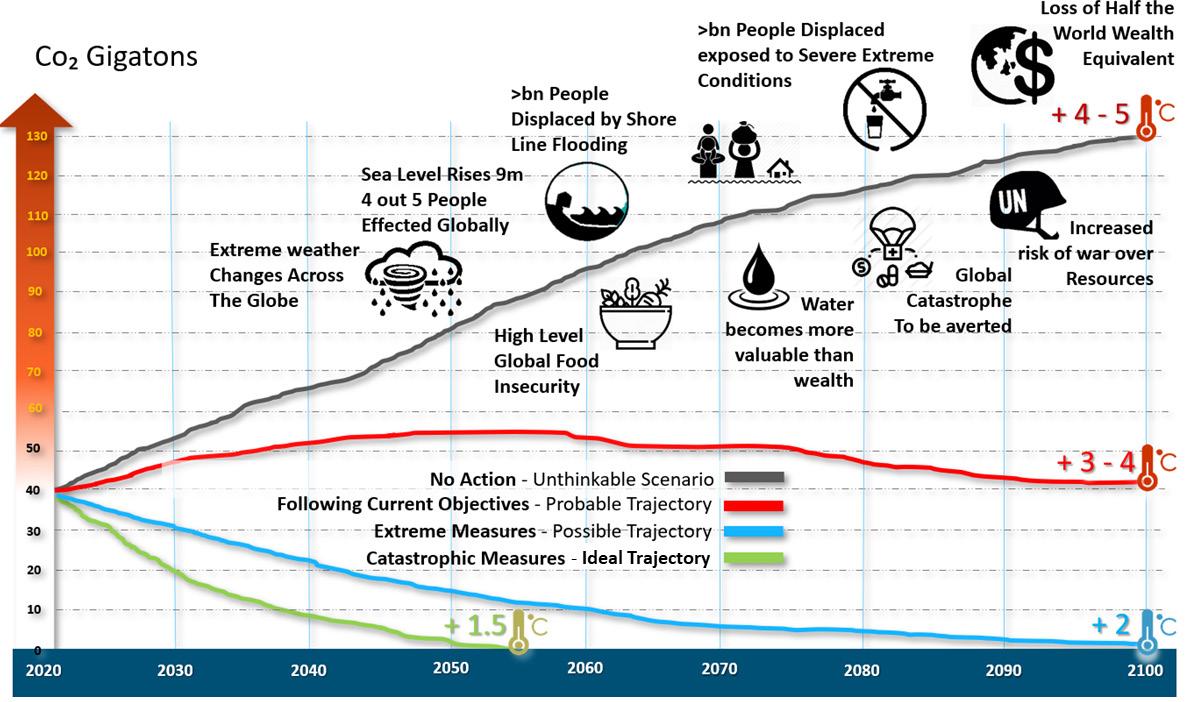
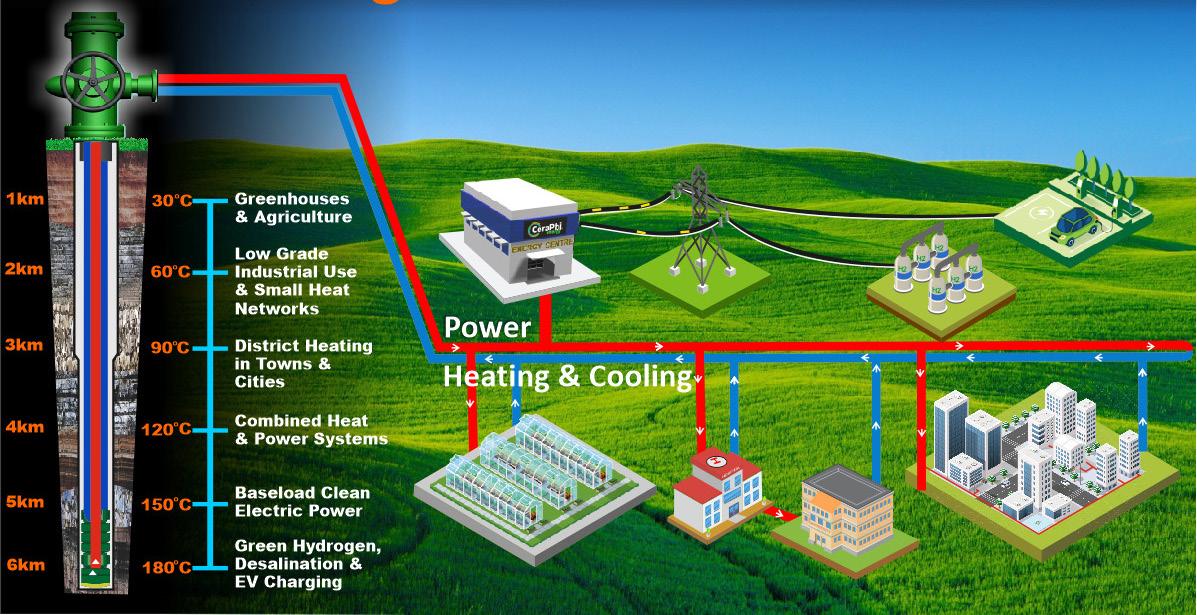
A huge challenge for the geothermal energy industry is perception. Drilling in geothermal needs to be looked at in the same way as in oil and gas. The only difference being, with oil and gas the requirement is to get the resource as quickly as possible and then shut down the field. Geothermal is about getting the fuel for as long as possible, at a lower but longer rate of return – a baseload replacement for heat that can be monetised with a bottom line of double digits.
Wider political and funding support and a commitment to the development of geothermal technology as a viable part of the new renewable energy mix is also much needed and – working with, and as a founding member of the Geothermal Energy Advancement Association (GEAA) – CeraPhi Energy is already starting to make significant headway accelerating the development of geothermal solutions.
Similarly, the company is already well underway advocating and initiating the migration and application of oil and gas expertise. Earlier this year, CeraPhi appointed Petrofac to support it on a ‘first of its kind’ study that will evaluate whether
Figure 3 The communication challenge for geothermal. Figure 2 The CeraPhi geothermal energy cascade.oil and gas wells in the North Sea can be repurposed for geothermal energy. The work on the Enquest Magnus will determine whether heat from the wells can be used as a direct power and/or as a heating or cooling for services and utility.
The opportunities for geothermal energy as a viable, clean, continuous, and secure part of the energy mix are considerable. The resources are already there in the millions of unused oil and gas wells across the world. The skill set of oil and gas professionals is already there to draw on, with engineering skills in well integrity management, root cause analysis, corrosion and erosion, wellbore management, fracturing, and stimulation of all areas of expertise transferable to the geothermal energy sector.
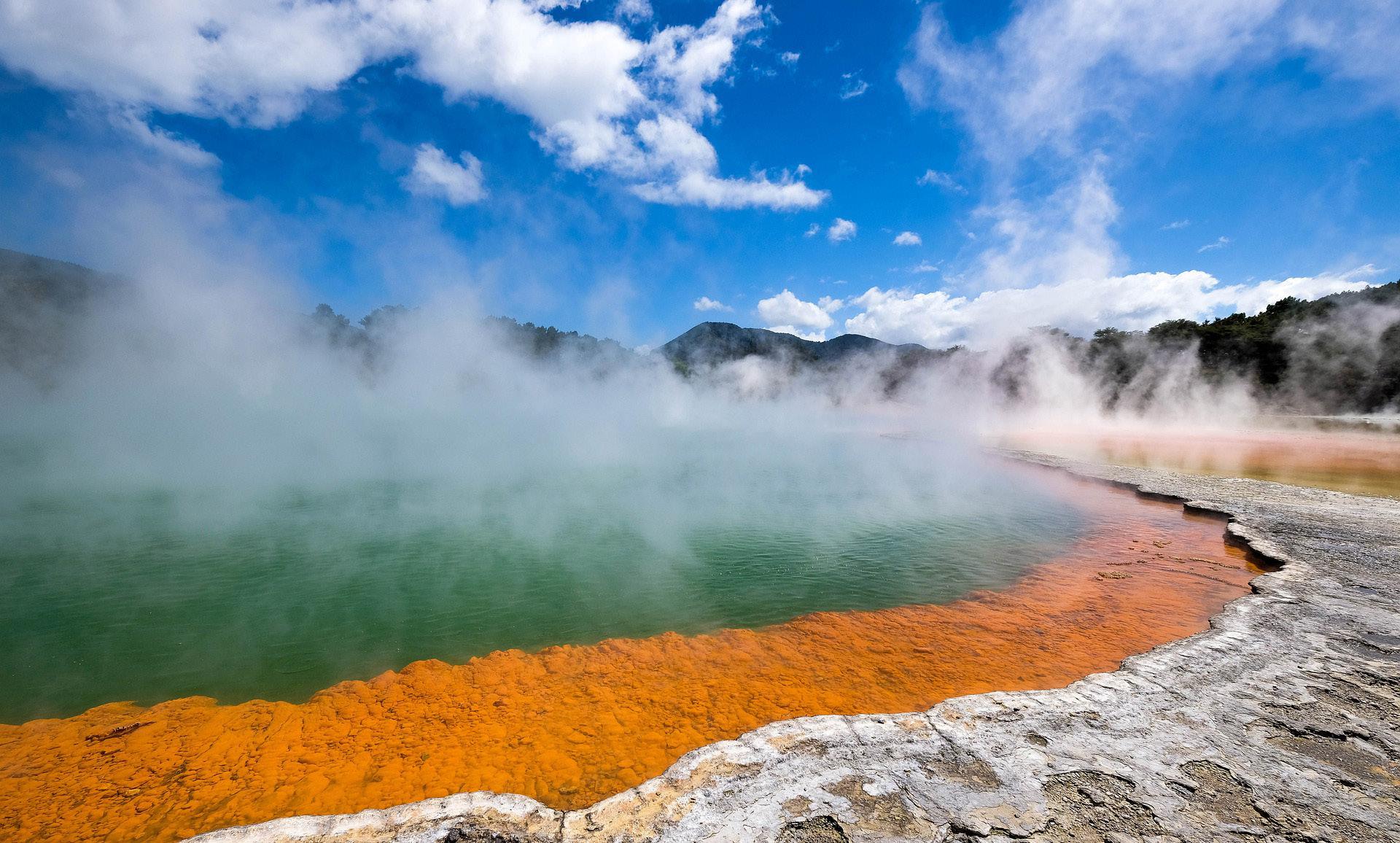
The technology
Technology is key in the production of geothermal energy, and CeraPhi Energy are already well advanced in the development and utilisation of it. One of these technologies is CeraPhiWell TM , which enables the recovery of commercially useable heat energy from the sub-surface virtually anywhere in the world. A downhole heat exchanger, CeraPhiWell is designed to circulate proprietary working fluids, moving the heat to the surface where it can then be processed into direct heat or power use before being returned to the well.
Additionally, the technology offers a ‘fall back’ for those wells that fall short of production expectations
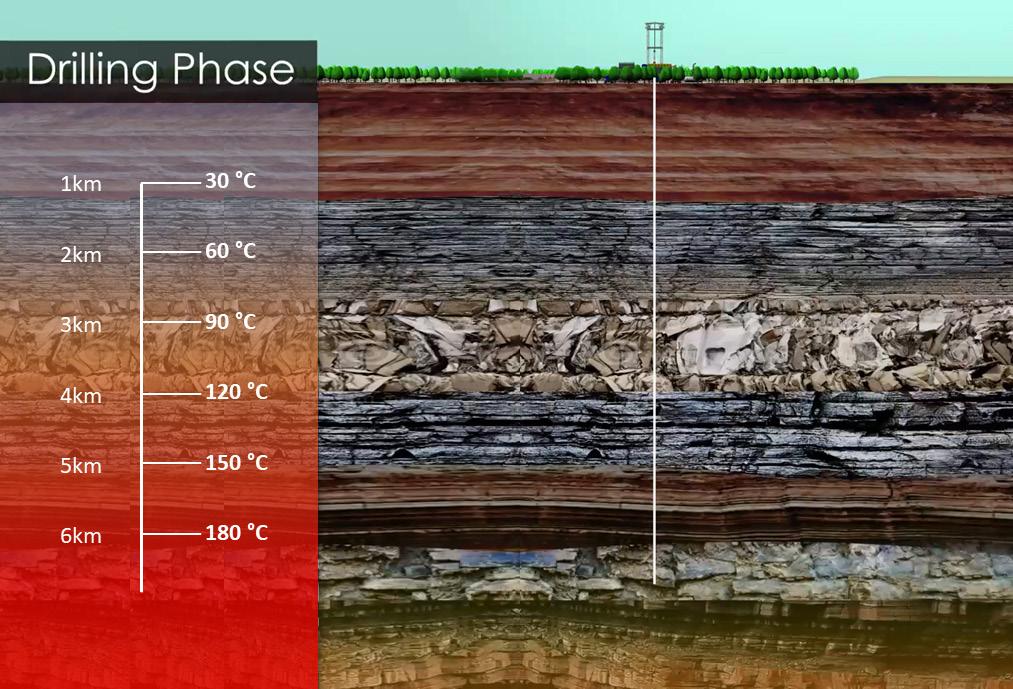
and where retrofit architecture can be installed to leverage some commercial return as opposed to a costly well abandonment.
CeraPhi Energy is well positioned to advance the use of geothermal energy both across the UK and the world and to demonstrate the real viability of this energy source as a key player in the energy mix. The company’s turnkey capabilities and highly skilled team are already operational on projects across the globe and continue to advocate geothermal energy as the cleanest, cheapest, and most efficient 24/7 baseload energy.
Figure 5 A geothermal hot spring.use of geothermal energy is not new. People have been using the hot water and steam emanating from natural pools and hot springs for thousands of years for heating their homes, bathing, and cooking. Of course, these uses were limited to areas having hot springs. In more recent times, with the advent of modern technologies, the use of geothermal energy has begun to expand. In fact, although not widely used until the late 19th Century, the world’s first district heating system was installed at Chaudes-Aigues, France, in the 14th Century. Geothermal district heating systems are now used in many places around the world.
The use of geothermal energy to produce commercial power first occurred in 1911 in the very first geothermal power plant built in the Devil’s Valley in the Larderello, Italy, dry steam field. This plant provided electricity for the Italian railway system. In 1958, New Zealand’s Wairakei power station became operational. This power plant was the first in the world to make use of flash steam technology. Since the 1980s, the use of binary and organic rankine cycle (ORC) power production technologies has grown, especially when harvesting power from lower temperature geothermal resources. Over the next couple of decades, many countries including the US, Iceland, Turkiye, Kenya, Indonesia, the Philippines, New Zealand, Italy, Germany, and Mexico, have invested
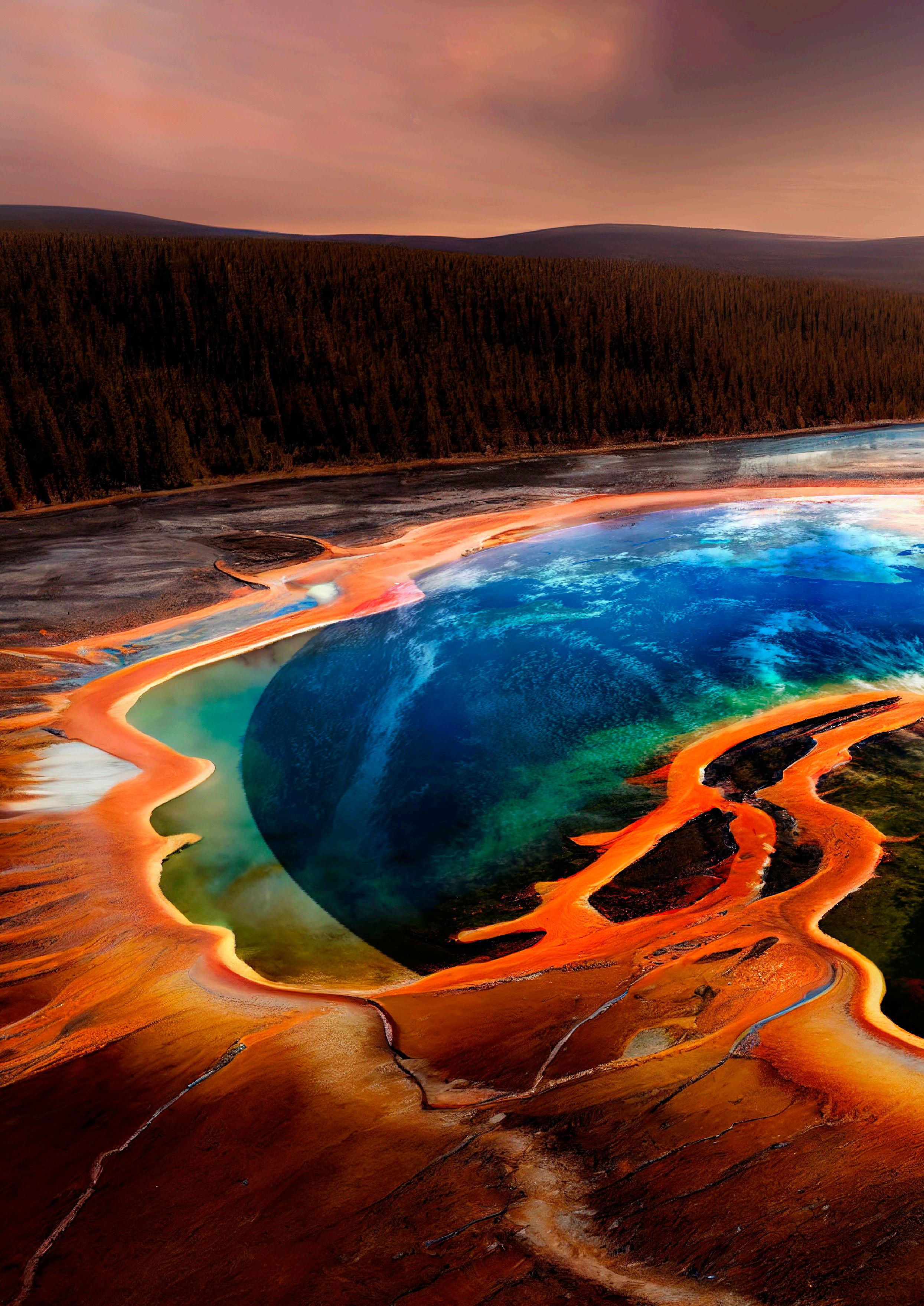
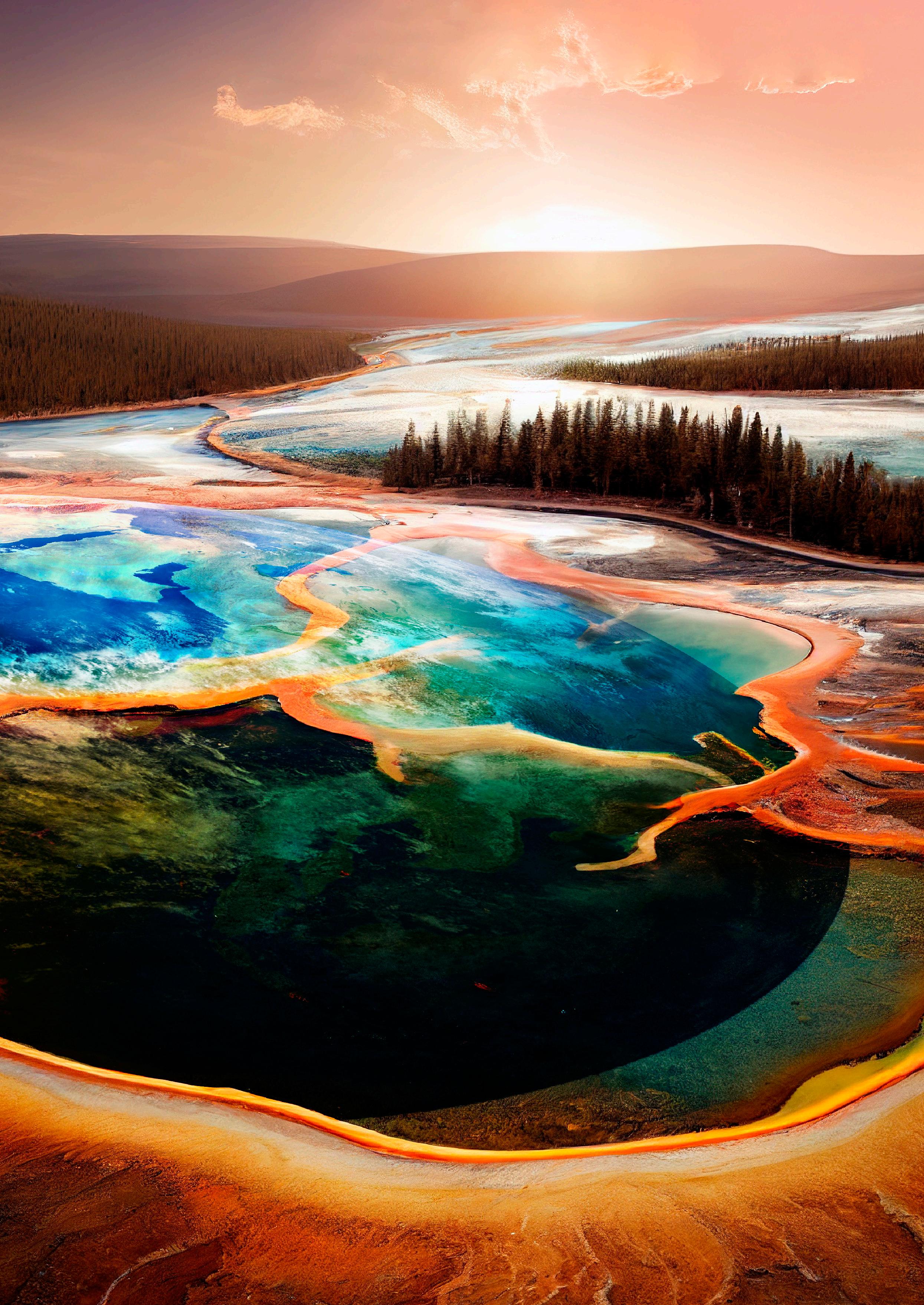
in geothermal power technologies. Today, a total of 17.4 GW of electric power is generated from geothermal energy sources.
Generation one: Hydrothermal systems
Until recently, other than the use of modern technologies, the harvesting of geothermal energy for heating and power production has not changed all that much. Essentially, the majority of the 17.4 GW of electricity that is being generated around the world is still being done in areas where hot water and steam are close to the surface. These are areas around the plate boundaries, known for volcanic activity (such as the Ring of Fire, New Zealand, Indonesia, and Iceland). These plants take advantage of existing natural hydrothermal systems –heat close to the surface (magma), underground fractures (plumbing system), water (oceans) – bringing that water and steam to the surface for district heating or to run power plants.
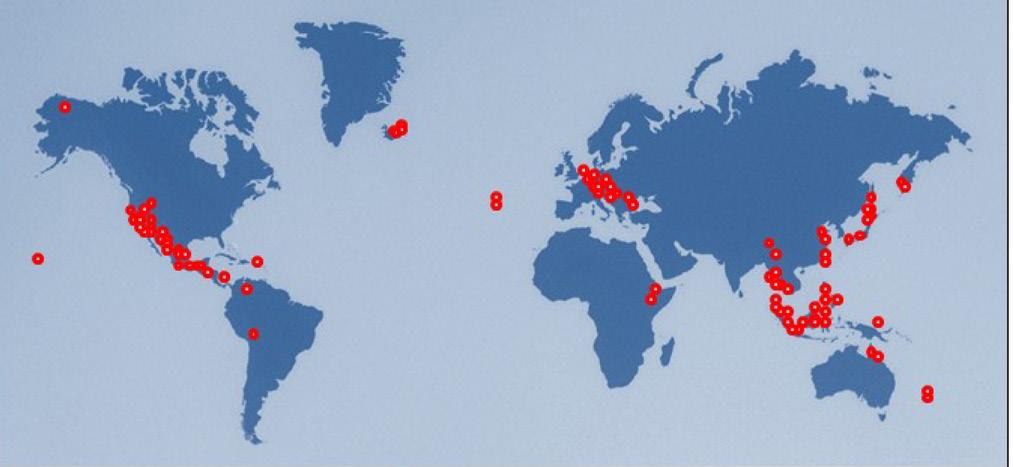
All good, except for the fact that natural hydrothermal systems are rare, representing only approximately 2% of the Earth’s surface (Figure 1). While a great approach, this first generation of geothermal energy is simply not scalable.
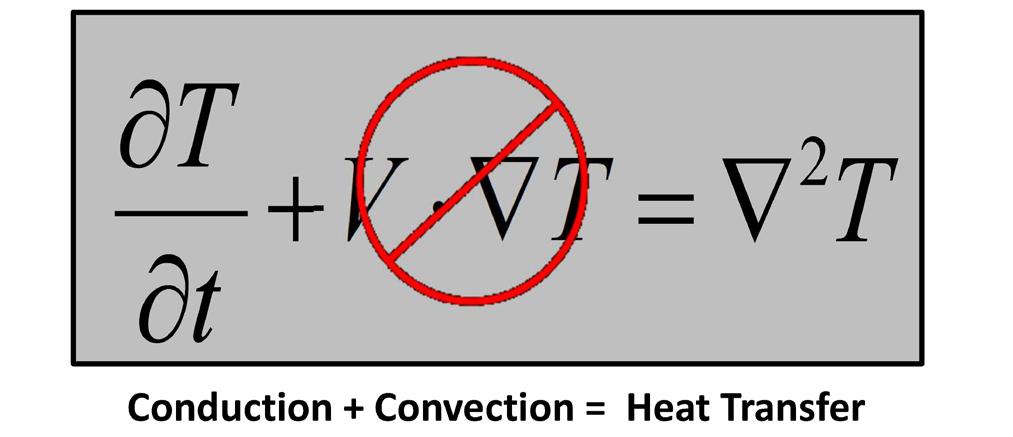
Generation two: Hot dry rock, enhanced geothermal systems
Acknowledging this geographical limitation, and knowing that basement rock is very hot, scientists and engineers began working on creating an engineered system to harvest the heat from hot dry rock (HDR). Such a system can be constructed virtually anywhere. The deeper you drill, the hotter it gets. The first enhanced geothermal systems (EGS) effort took place at Fenton Hill, New Mexico, the US, with a project run by the US’s federal Los Alamos laboratory. It was the first attempt to make a deep, full scale EGS reservoir (Figure 2). Since then, billions of US dollars have been invested in the development of EGS systems in Australia, France, Germany, Japan, Switzerland, and the US. To date, this approach has proven unsuccessful. The reasons are four-fold: (1) The water that is pumped into the injection well sometimes finds its way into the HDR’s natural fracture system and disappears; (2) The water that does make its way to the production well leaches minerals from the rock, corroding pipes and causing numerous maintenance and operational problems; (3) The basement rock is very dense and cannot be adequately imaged using existing technologies. Therefore, drilling operations are often ‘wildcatting’, with drillers hoping to hit a suitable fracture system; (4) Rock is an insulator, not a conductor. It has a very low thermal diffusivity. Because the process is dealing with rock, conduction is the only mechanism for heat transfer to occur (Figure 3), as convection only occurs in liquids and gases. Therefore, the geothermal resource is quickly exhausted as the rate of heat extraction exceeds the rate of natural heat recharge (conduction through the rock).
Much work is being done to address these problems. In the US, for example, the Department of Energy has funded the FORGE project which is looking to find solutions.
Generation three: Closed loop systems
In recent years, new technologies have been emerging which address many of the problems in these second generation EGS systems. These are the closed loop designs. There are several companies commercialising closed loop systems, including Eavor Technologies, GreenFire Energy, and Sage Geosystems. All have a different approach, but all involve circulating a heat exchange fluid in an underground closed loop system to harvest the heat from the geothermal resource. With these systems, there is no loss of water (circulation), there are no leaching/corrosion problems, and imaging/wildcatting is not an issue. If used for district heating purposes, these technologies are certainly up to the task. What remains to be seen is how these technologies will be able to overcome the problem of the exhaustion of the geothermal resource that will occur due to the rapid heat extraction that is required to produce economic power.
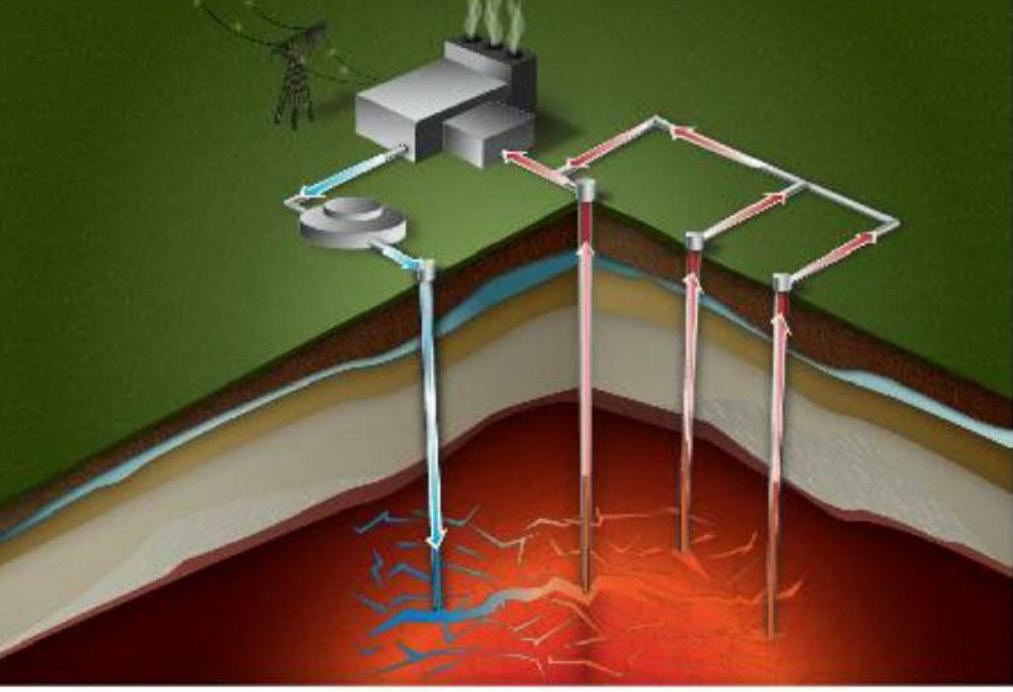
Generation four: Hot sedimentary aquifers
Hot sedimentary aquifiers (HSAs) have been used in various places around the globe for district heating, but due to various
Figure 1 . Existing geothermal power plants (2021) are geologically and geographically very rare. Figure 2 . Hot dry rock (HDR)/enhanced geothermal systems (EGS) system. Source: Department of Energy.temperature and flow rate limitations, have found minimal use for large scale power generation. However, recent advances in oil and gas exploration and production technologies are about to change this dynamic. Novel approaches to mining the heat from HSAs are now being taken in an attempt to address the problems of the first three generations of geothermal power production. Several companies, such as Geothermal Technologies, Inc. (GTI) in the US and DEEP in Canada, are working in the area and are bringing these new technologies to bear. HSAs are an interesting geothermal resource because:
1. HSAs are globally abundant, existing in many areas of the world – solving the scalability problem of first generation geothermal power production.
2. HSAs contain large volumes of hot water that can be harvested. And, that water will not be wandering off through the natural fracture system – solving the loss of water problem. In fact, the fractures will help with the circulation of water within the aquifer, thereby bringing water/heat to the system from the far field.
3. The water in the aquifer is at chemical equilibrium with the surrounding sandstone – eliminating the water leaching/corrosion problem. The composition of the water is constant and predictable, allowing for proper metallurgy selection in the facility’s design.
4. The geology of these reservoirs consists of sandstones which can be thoroughly imaged with the 3D seismic, passive seismic, and electromagnetic imaging technologies that have been developed for the oil and gas industry – solving the wildcatting problem and enabling the placement of the wells in locations for optimum system performance.
5. Water, being a liquid, allows for both convective and conductive heat transfer. This combination maximises the heat transfer within the system – eliminating the exhaustion of the geothermal resource due to the convective recharge that occurs (Figure 4).
Case study: GTI’s geothermal power plant development in Colorado
GTI is currently developing a GenaSys geothermal power plant within the sedimentary basin not far from the US city of Denver, Colorado. Much is known about the subsurface geologic formations from the decades of oil and gas exploration that continues to this day. Beneath the oil and gas fields lies a hot (approximately 135˚C), saline non-potable aquifer that is contained within a permeable sandstone formation. GTI has been able to repurpose many of the technologies developed recently to explore for hydrocarbons. Proprietary technologies have also been developed that are focused on optimising the geothermal power system for longevity and for reducing the cost of the electricity.
Some of the key technologies applied by GTI in the development of the Denver-Julesburg Basin GenaSysTM geothermal plant are summarised in Figure 5. Forward modelling that incorporates the geoscientific data obtained from oil and gas developments and other sources allows GTI scientists and engineers to optimise the
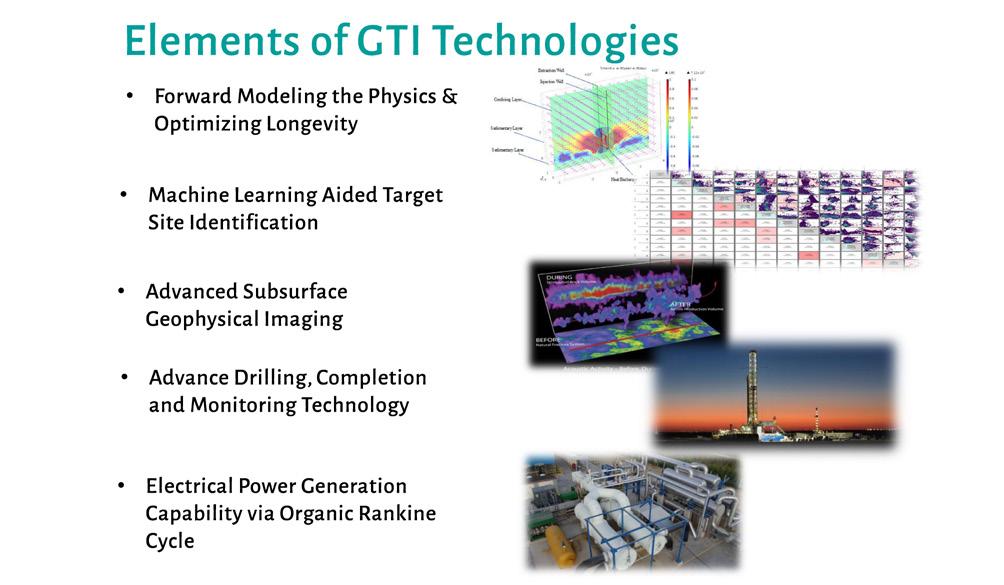
Figure 4 Illustration of Geothermal Technologies, Inc.’s GenaSysTM Convective Recharge.
Figure 5 Key technologies incorporated in identifying optimised GenaSys geothermal plant locations and optimising power generation.
design of the underground power system. GTI’s forward modelling efforts are coupled with proprietary machine-learning algorithms that facilitate the fusion of multiple data layers that parameterise the geology of the underground power system. GTI is in the process of leveraging subsurface imaging technologies – both active and passive seismic to not only better understand the subsurface structure, but also the magnitude and velocity of the convective heat recharge system.
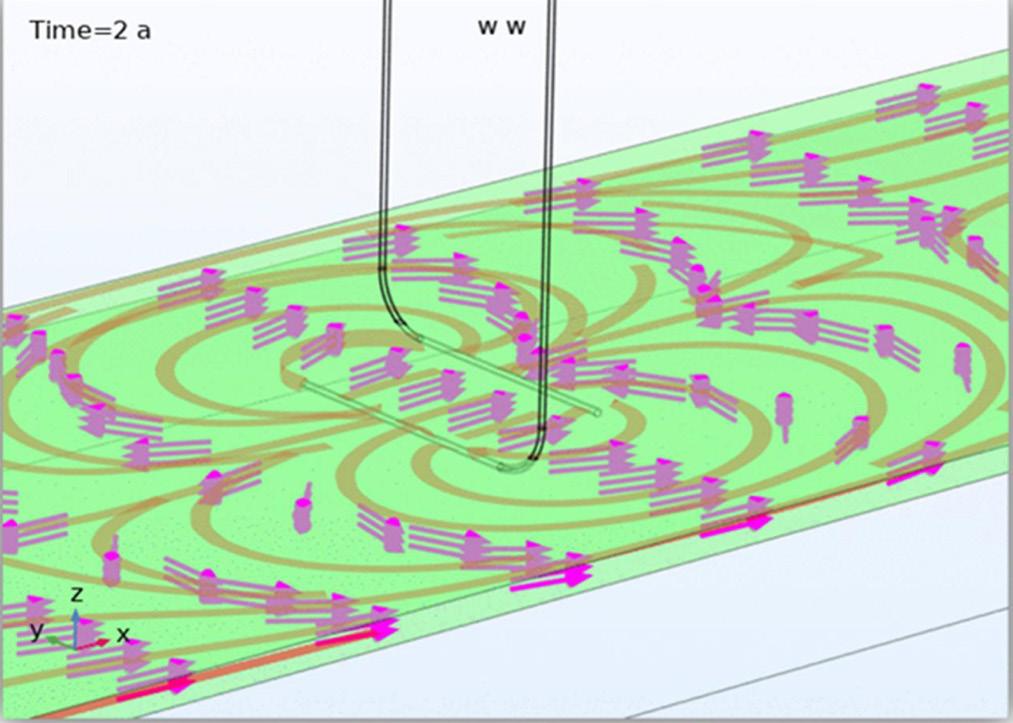
GTI has secured the surface and subsurface rights to drill and construct a power plant from a local landowner and is in the process of receiving a permit from the State of Colorado to begin drilling operations.
In addition to the application of geoscience technologies, GTI is collaborating with many of the world’s experts from the oil and gas industry, including Helmerich and Payne (H&P), an experienced and technically advanced drilling company based in the US, and the global oil field services company Halliburton who will lead the effort of completing and instrumenting the wells in preparation for power production. The above ground system will be provided by one of the many ORC power generation system vendors who GTI is in the process of vetting. Considering the permitting and construction time, GTI expects to be making clean, baseload, economic power by 2024 and supplying that power to either a local customer or to the electrical grid that supplies Denver and its neighbouring cities for decades.
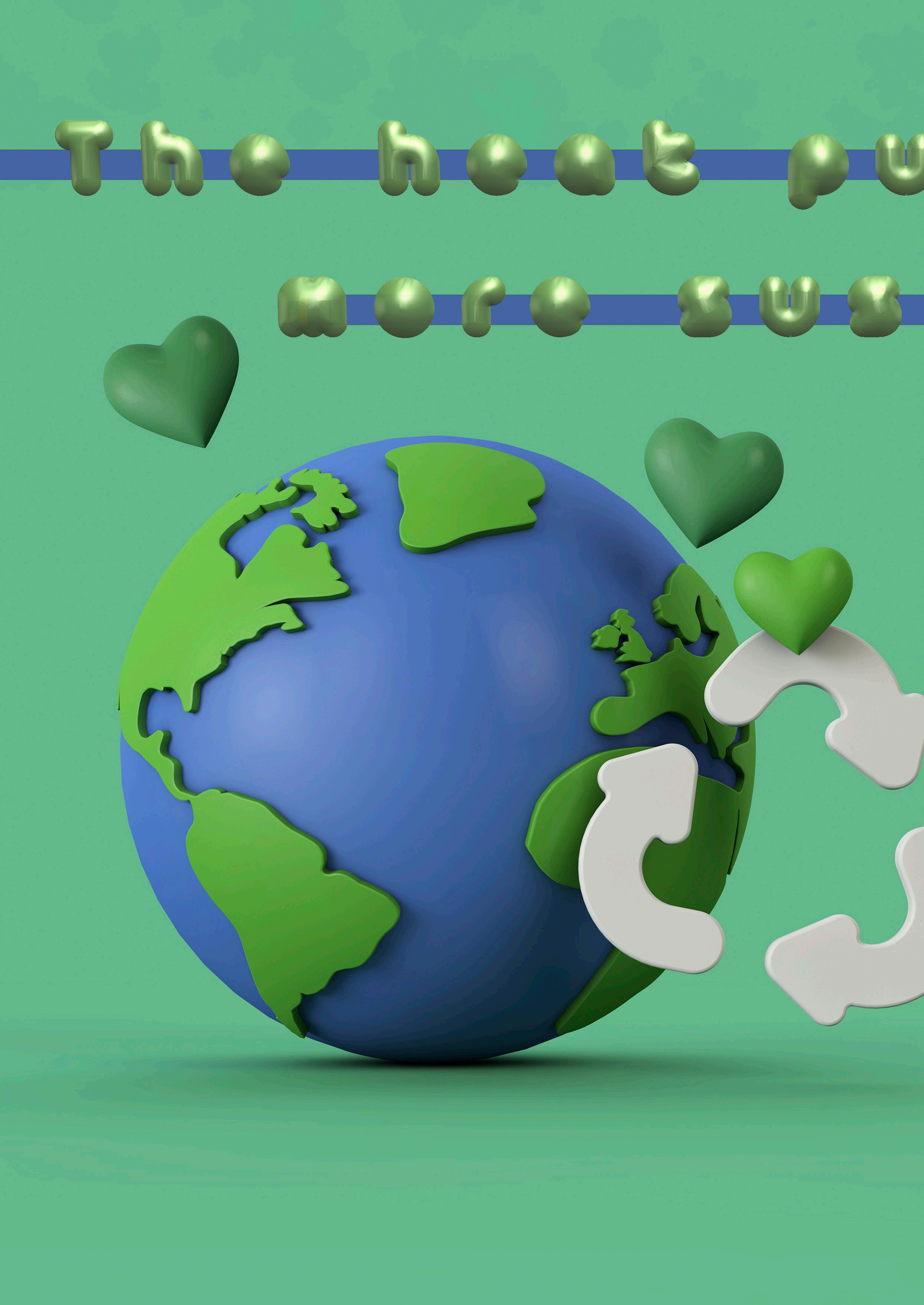
For decades, it was almost a certainty: energy was available when it was needed. The price: negligible to acceptable. Be it coal, oil, or natural gas, these cheap fossil fuel energy sources have been powering production around the world since the Industrial Revolution.
If one looks back at the history of the industries, the last two centuries in particular have been characterised by constant increases in the speed, efficiency, and capacity of production. On the one hand, this development has brought wealth and the convenience of a modern lifestyle to many areas of the world. On the other hand, burning fossil fuels to power modern industry is the main cause of climate change, experienced in extreme weather, poor air quality, and species extinction.
But something is happening, and not only since recent climate demonstrations have put greater pressure on decision-makers and supervisory boards. For the first time in modern history, the goals of many politicians, industrial decision-makers, and climate activists are narrowing: “Things cannot stay the way they are” is the mantra. Admittedly, the individual motivations may be different, with one group more concerned about protecting the planet, for example, and another more concerned about the continued existence of a business or business model. Whatever the reasoning, the aim is greater sustainability.
Energy independence
The most recent geopolitical developments of 2022 have further revealed the dangers of countries depending on fossil fuels for energy: the intensive interdependence of the energy markets make fossil fuels highly susceptible to international crises. Without a constant supply of energy sources, the lights literally go out. This dependency not only makes national economies vulnerable to crises, but also, to a certain extent, it makes them externally determined.
So, it is not just the idea of sustainability that is leading to a rethink across many political parties; it is also about
efforts to minimise the effect of crises, volatile prices, and imports.
Green hydrogen, widely praised as an energy game-changer, appears in many industries as a simple and pragmatic solution to help end dependence on fossil fuel energy sources. However, it is produced from electricity with high energy losses and requires greater efficiencies before its wide scale deployment. Moreover, for ordinary applications, heat pumps can do the job as good as hydrogen, and increases in electrolyser efficiency will not change this fact.
Of course, there are many industrial applications for which hydrogen can be used sensibly – in particular, applications that require high temperatures for combustion, a direct chemical reaction, or for long-term energy storage. Examples of this are in steel production, cement production, the glass industry, or hydrogen as a feedstock in the chemical industry.
Temperatures – only as high as necessary
As well as these example industries, there is a lot of energy demand below the high-temperature level. Still, even low temperatures are generated with extremely hot flames, something that is not technically necessary. In paper production, the food industry, parts of the chemical industry, and also district heating, temperatures of only approximately 100 – 250˚C are required.
Reflecting the recognition that in many situations, lower temperatures are sufficient to produce the required energy or fulfil process needs, a technology that is now almost a century old is gaining greater attention: the heat pump.
Technically, heat pumps are comparable to the working principle of a normal refrigerator: a liquefiable gas, the so-called refrigerant, is evaporated in a cyclic process at low pressure, compressed in a compressor, and condensed at higher pressure. The cycle is closed by a pressure reduction component, usually an
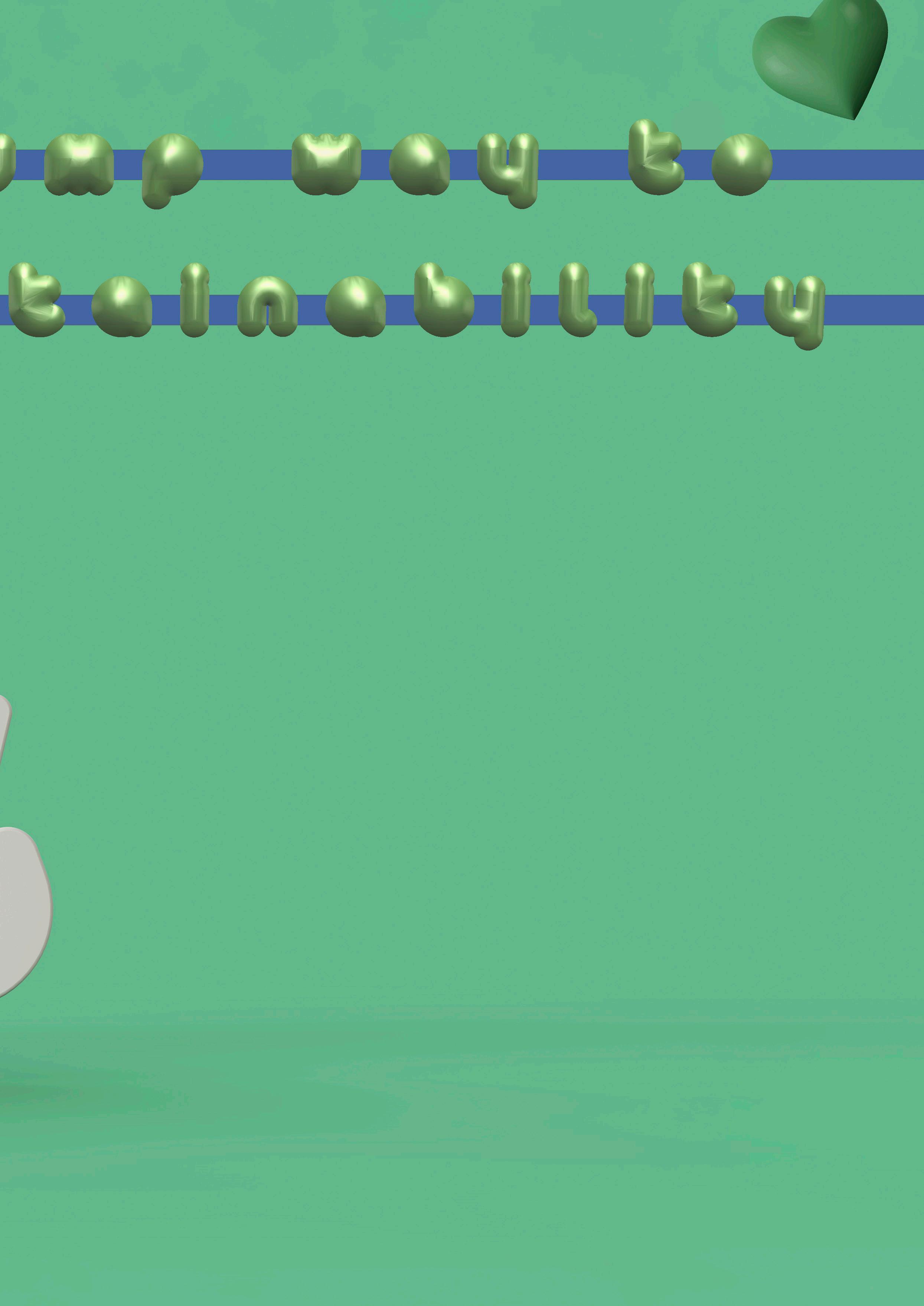
expansion valve. During evaporation, the refrigerant absorbs heat, such as from inside the refrigerator or from a low-temperature environmental or process heat source. The gaseous refrigerant condenses after compression at high pressure and high temperature. In the case of the refrigerator, this heat is released into the ambient air. With a heat pump, this heat is used for something useful, such as heating, district heating, or process heat.
COP, and getting the most from the electricity
The magic of the heat pump lies in precisely this trick: the heat is loaded into a refrigerant and raised to a higher temperature level using additional energy. This means that typically 2 – 4 kWh of heat can be pumped with 1 kWh of electricity. This ratio of electricity used to usable heat is referred to in technology as co-efficient of performance (COP).
Electric heaters have a COP of 1, and the electrolysis and combustion of hydrogen is typically 0.6, mainly due to losses in electrolysis.
A heat pump, therefore, generates much more usable heat from the same amount of electricity compared to other technologies. Furthermore, no carbon dioxide (CO 2 ) is emitted if climate-neutral electricity from the sun, wind, water, nuclear power, or other sources is used,
though even operated with electricity from efficient gas- and coal-fired power plants may save CO 2
Farewell to fossil fuels
While the technology has long lived in the shadows of cheaply available fossil fuels, the current extremely high fuel prices and the lack of security of supply in parts of the world now finally seem to be setting the stage for the heat pump’s grand entrance. It seems ironic that the first large scale installations in Switzerland, dating back to 1938, were installed with the same motivation of minimising dependence on imported coal.
Several large Atlas Copco Gas and Process heat pumps with turbocompressors have been in operation in Scandinavia since the 1980s, which speaks for the longevity of the technology. The heat source is cleaned waste water from a sewage treatment plant, and the heat sink is the urban district heating system. The systems achieve thermal outputs of over 60 MW per unit.
Regarding CO 2 savings, heat pumps in industry offer enormous potential: two Atlas Copco Gas and Process heat pumps installed in Stockholm’s heating network, each with 40 MW thermal output, save 90 000 tpy of CO 2 emissions (compared to the previous use of heating oil). To achieve a comparable saving in road traffic, it would mean that the average Swedish gasoline-powered cars would have to drive 500 million km fewer every year (approximately 400 times to the moon and back).
The high impact areas of industry
There are five areas of production in particular that stand out for the use of heat pumps: paper production, food production, chemical/petrochemical, general production, and the aforementioned production of district heating.
Figure 1 . Cumulative amount and temperature levels of waste heat/process heat demand in the EU. The total amount of available waste heat adds up to 314 000 GWh/y. Data source: TNO 2021.
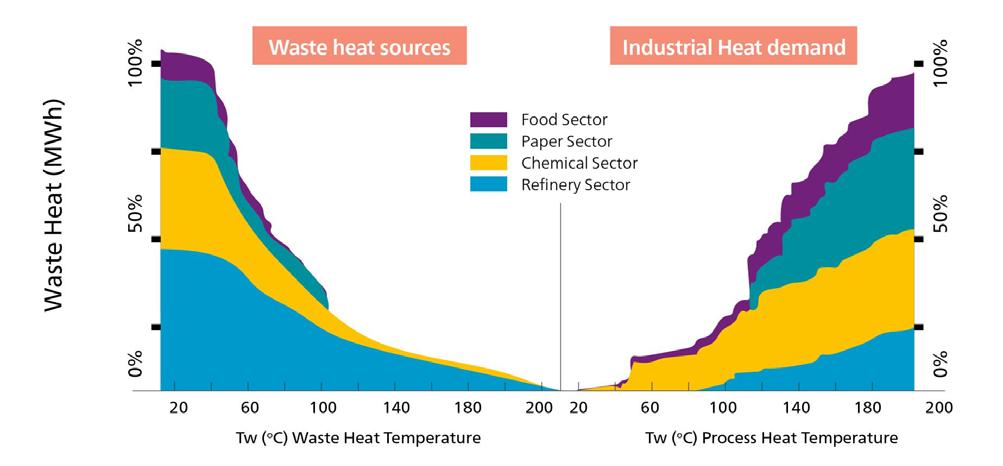
In these sectors, a large part of the required heat is used at temperatures between 80 – 250˚C. At the same time, low-temperature waste heat is available in all production plants, which is rejected via cooling towers. From an energy point of view, this conventional use of heat is an open process that can be converted into an energy circular economy by using a heat pump.
As well as from industrial production, there are more heat sources that are readily available which may not be immediately recognisable as such: municipal sewage treatment plants and hydrogen electrolysis plants represent a continuous flow of low-temperature heat that can be made usable again (similarly, river and sea water).
Example: Chemical industry
Figure 2 Basic principle of a heat pump. Here, a single stage, subcritical cycle is shown. The co-efficient of performance expresses the efficiency of the heat pump at given temperature lifts and temperatures.
Using an example from the chemical industry, the possibility of seamless integration of a heat pump into existing systems becomes clear. In the industrial production of bioethanol, the ethanol/water mixture obtained through fermentation is separated by a multi-stage distillation at approximately 80 – 100˚C. The distillation column is usually heated with low-pressure steam, which is generated from natural gas in a power plant or boiler. After separation, the purified ethanol
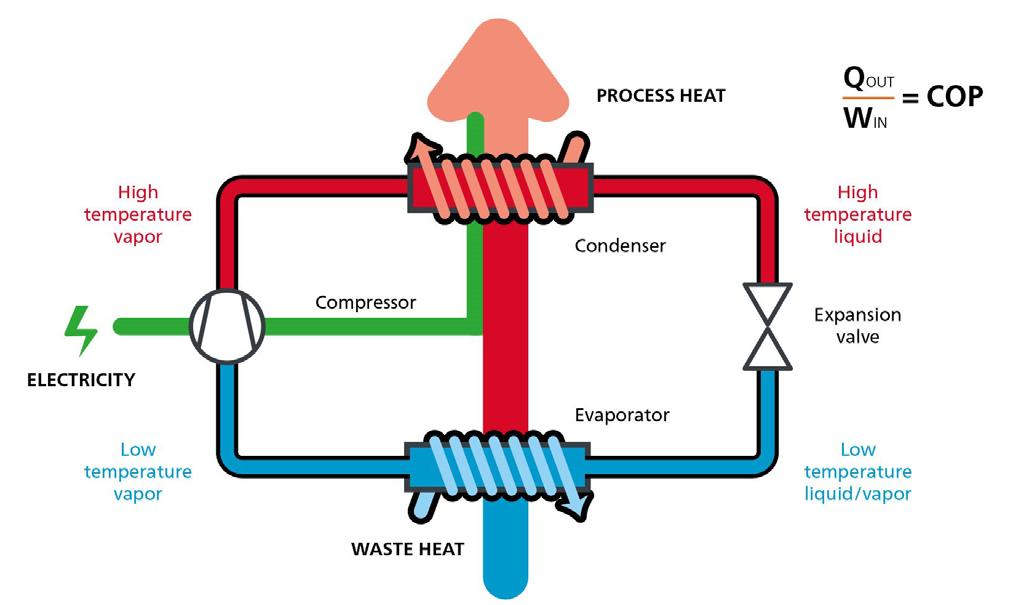
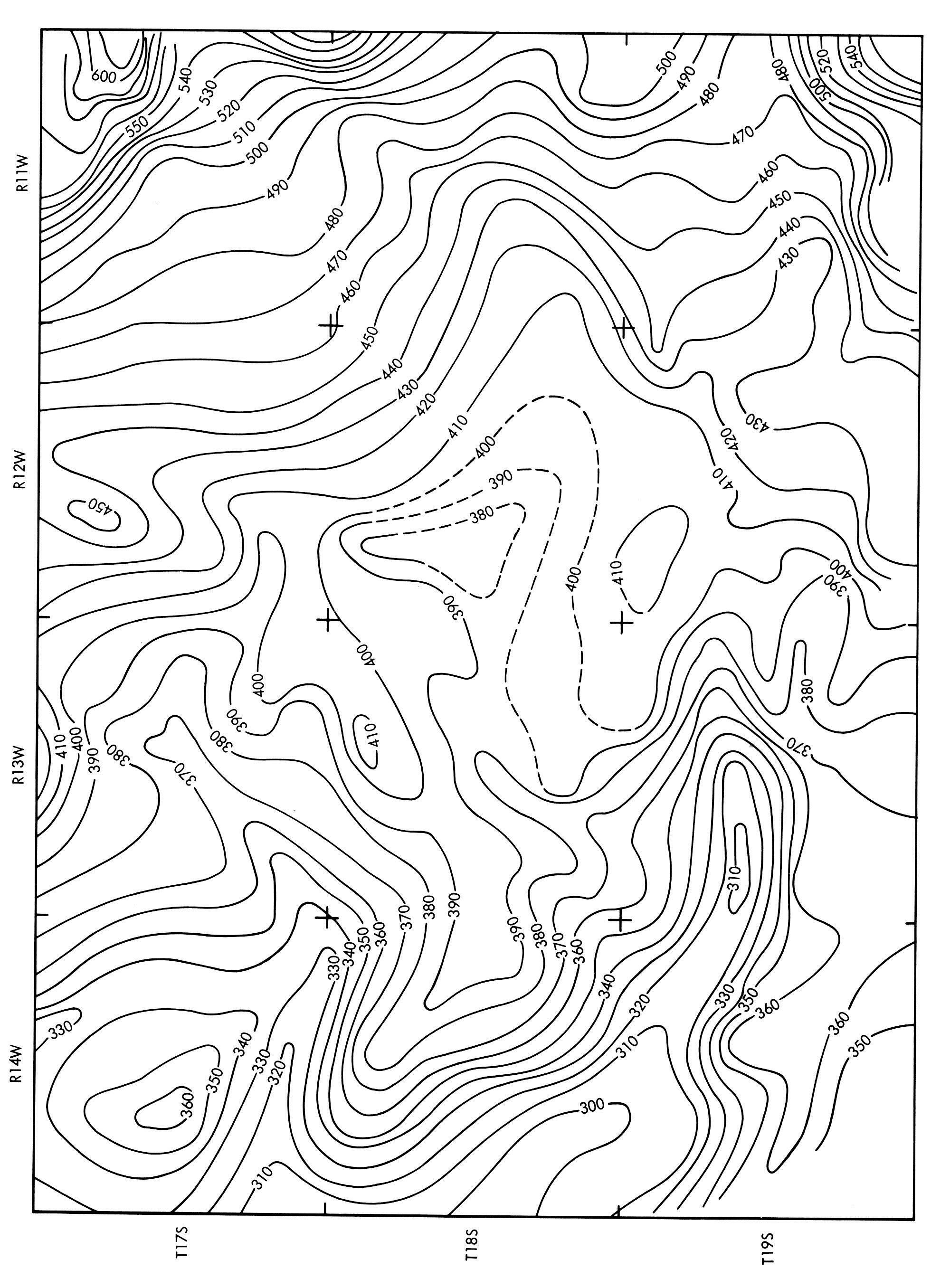
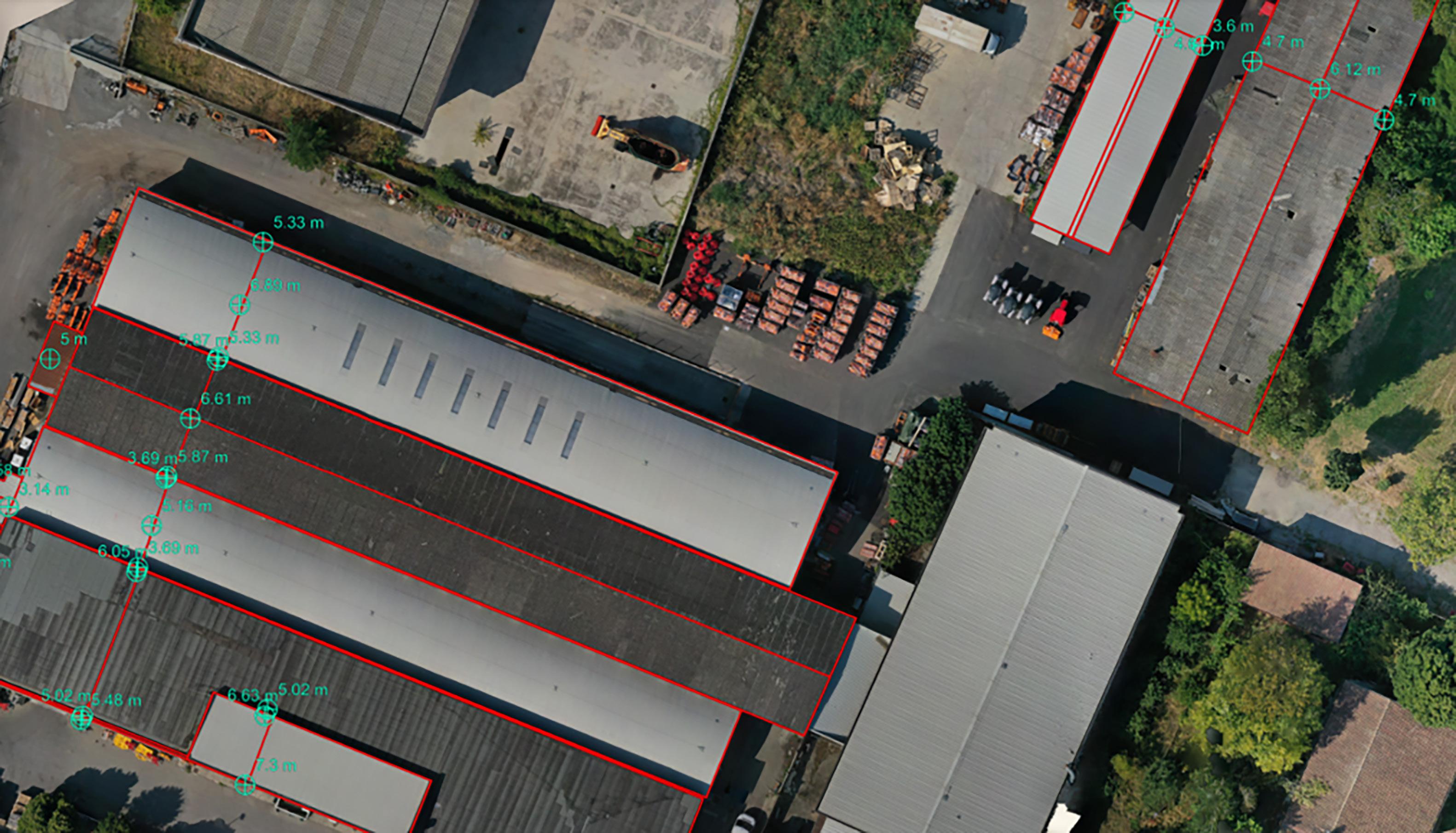
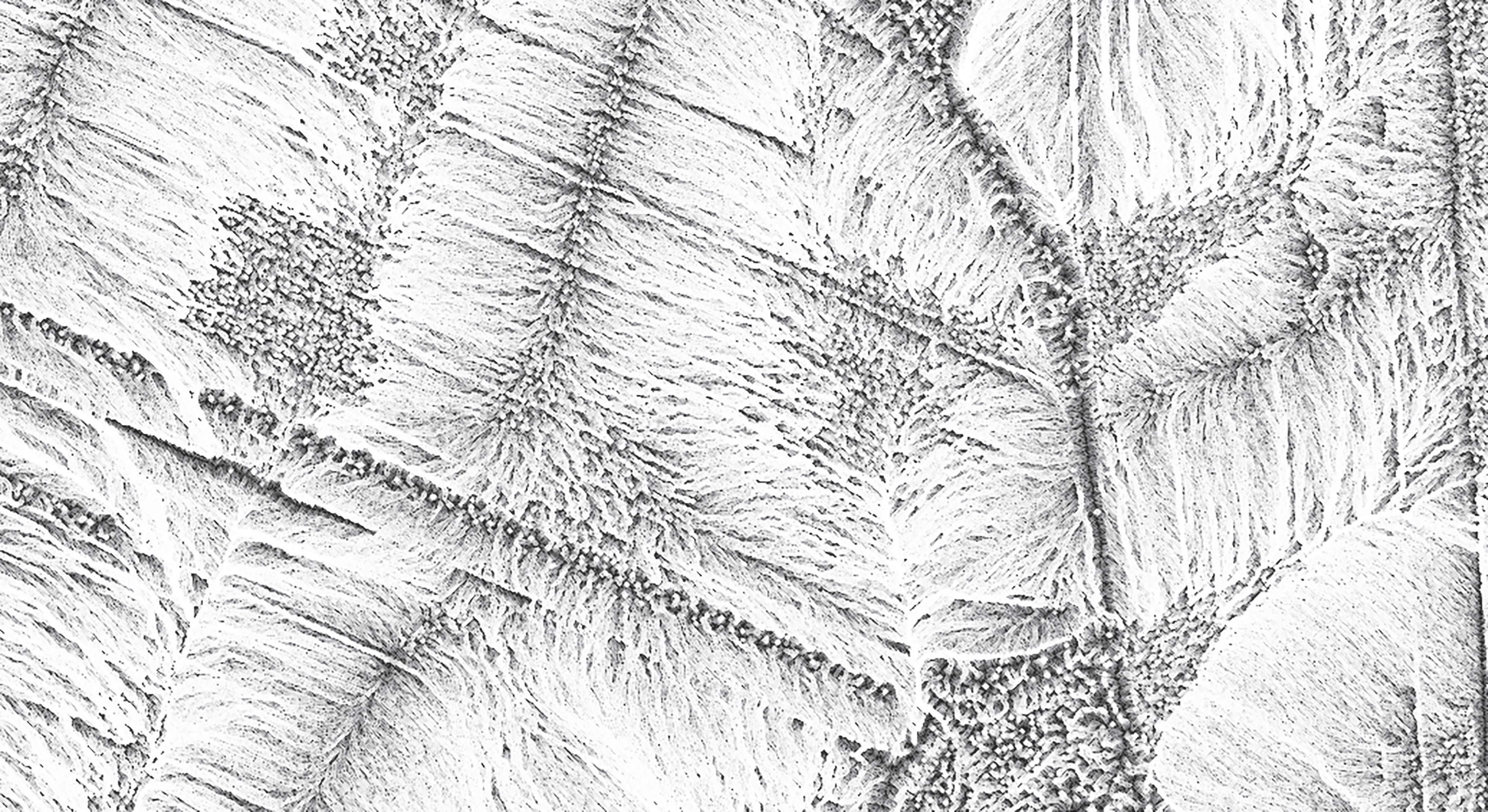
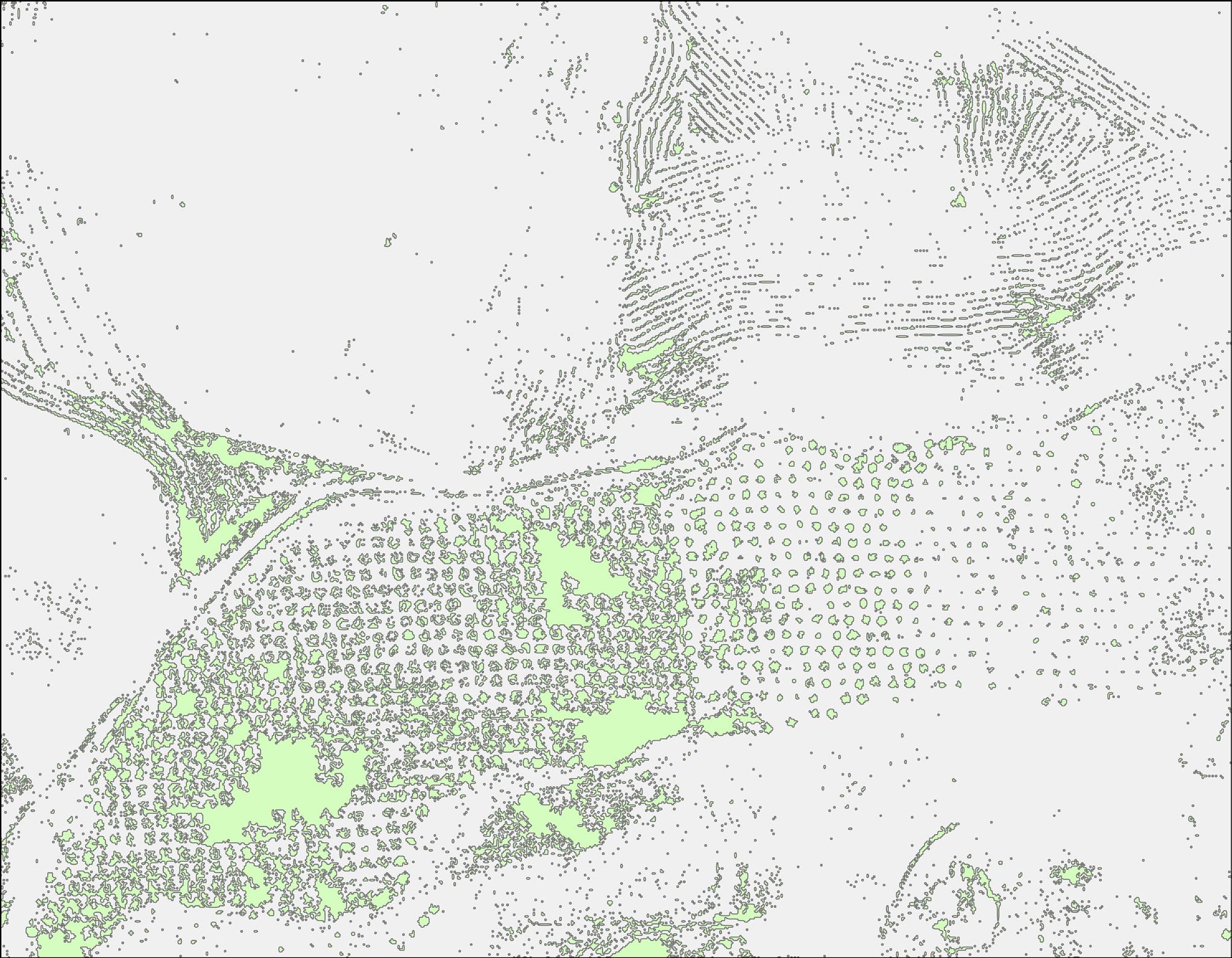
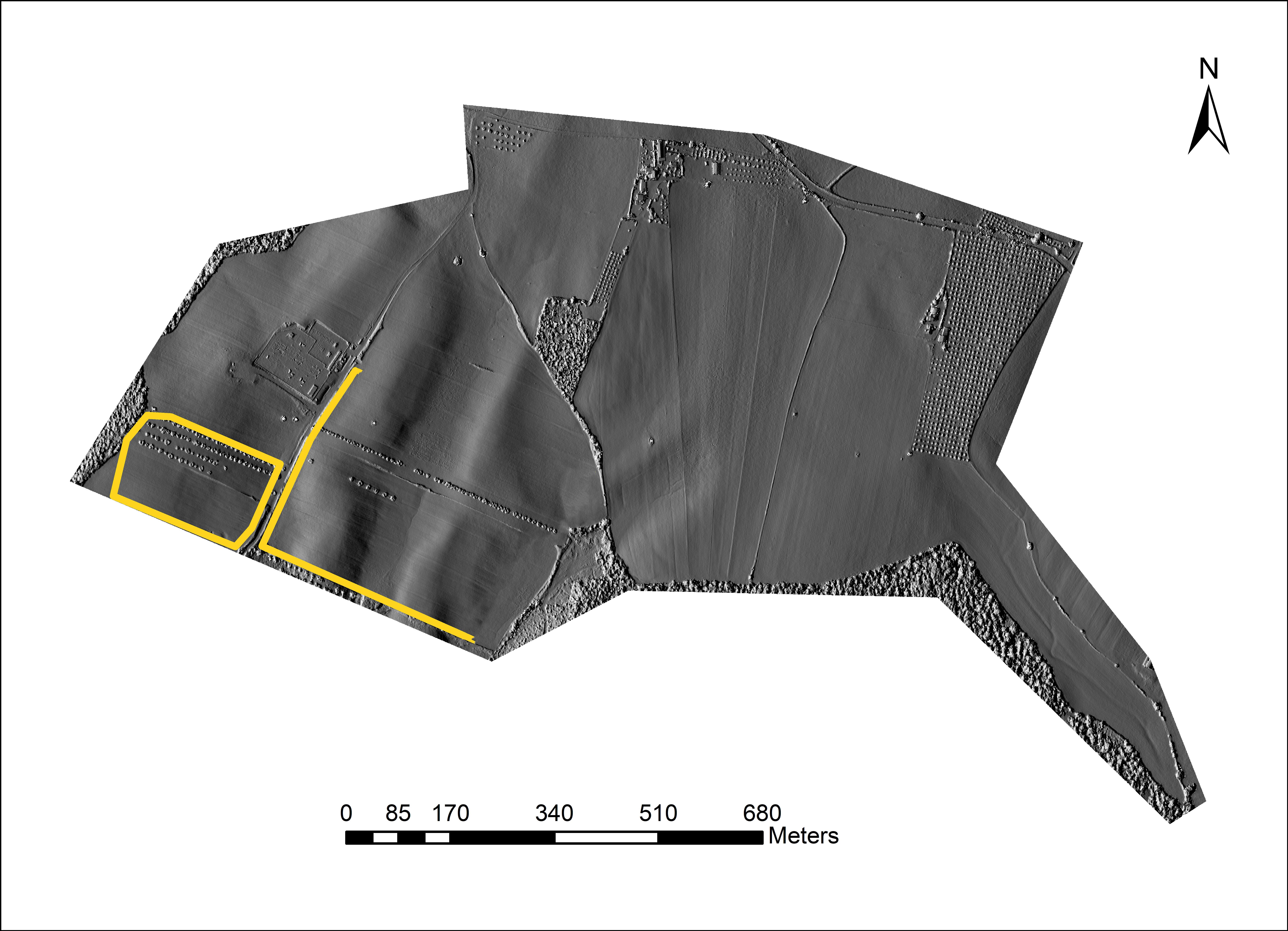
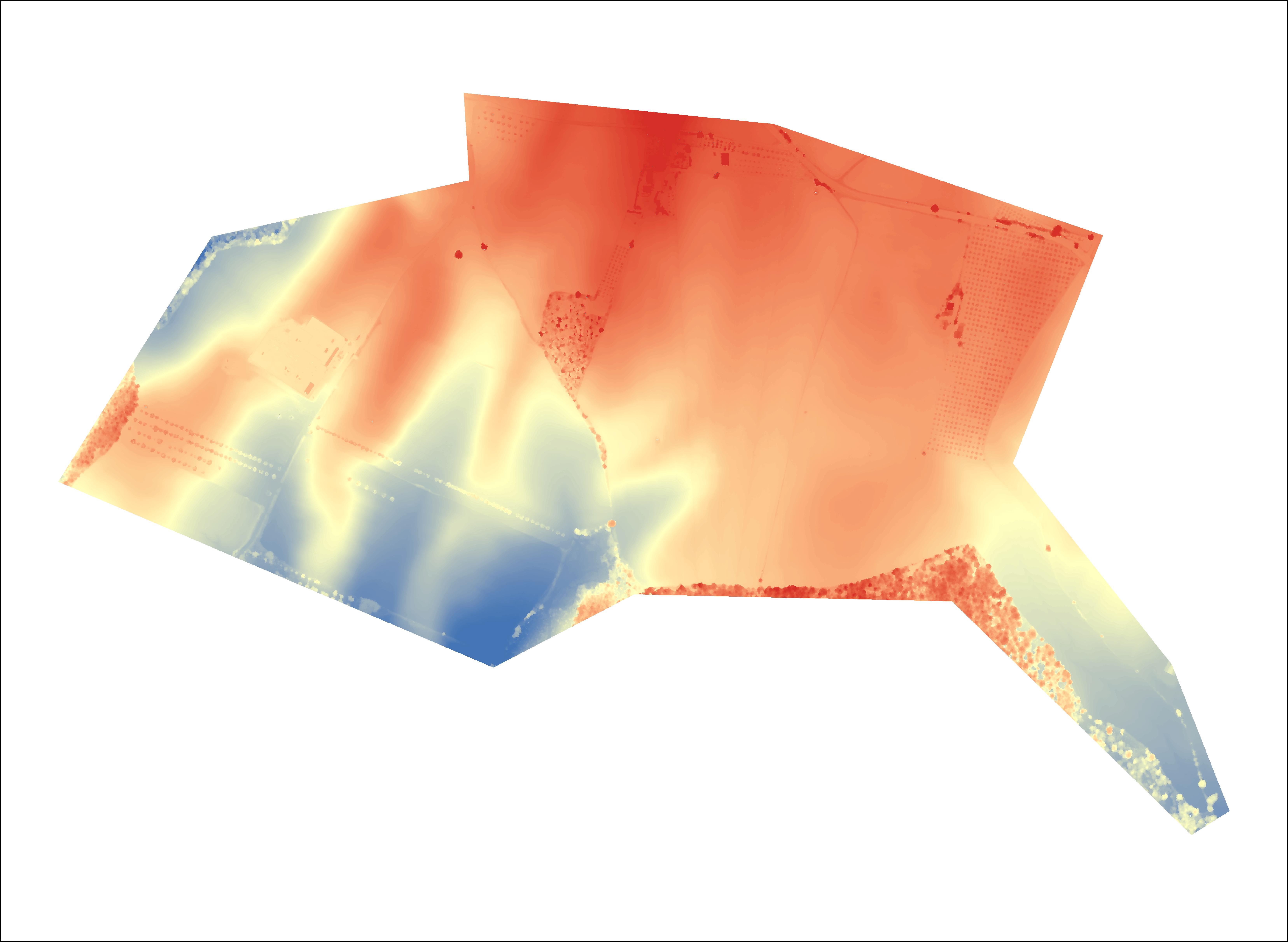
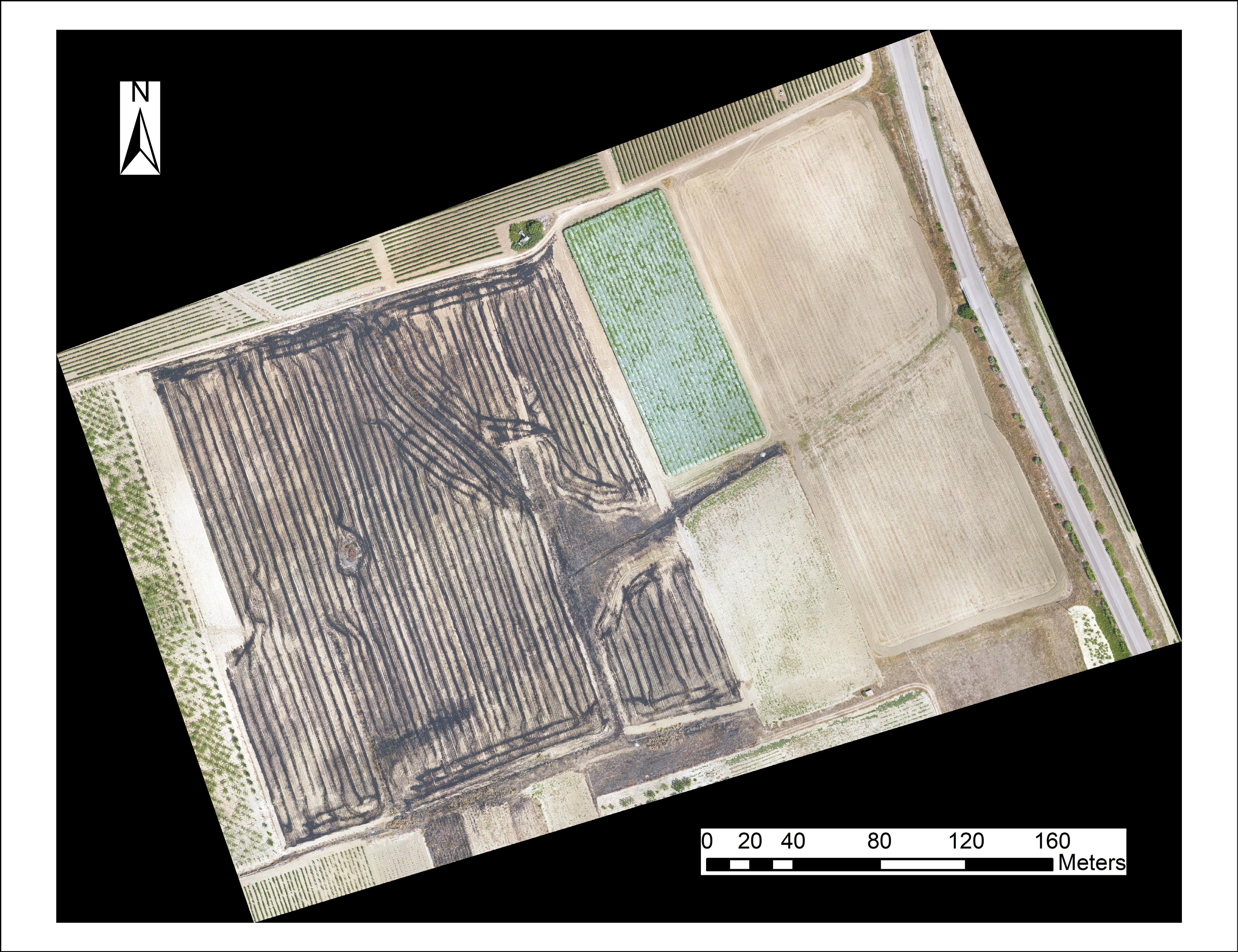
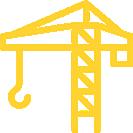
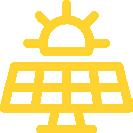
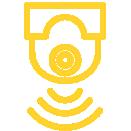
steam is condensed in a water-cooled condenser and the heat is released into the environment via cooling towers. However, if the heat of condensation of the ethanol is transferred into the circuit of a heat pump, the heat from the distillation can be fed back in as usable heat.
Such a system with a temperature rise from 70˚C to 110˚C may achieve a COP of 4, while thermal outputs of up to approximately 50 MW can be achieved per machine.
Example: Food production
In the production of sugar, large amounts of heat are required to first produce syrup and then crystal sugar from the aqueous sugar extract of the beets or sugar cane.
In conventional systems, the waste heat of the first apparatus is already used several times to heat the downstream processes, but eventually, it becomes waste heat at approximately 50˚C, which can no longer be used economically and is rejected via a cooling tower. This lost heat must be replenished by fresh energy, which is usually generated from fossil fuels.
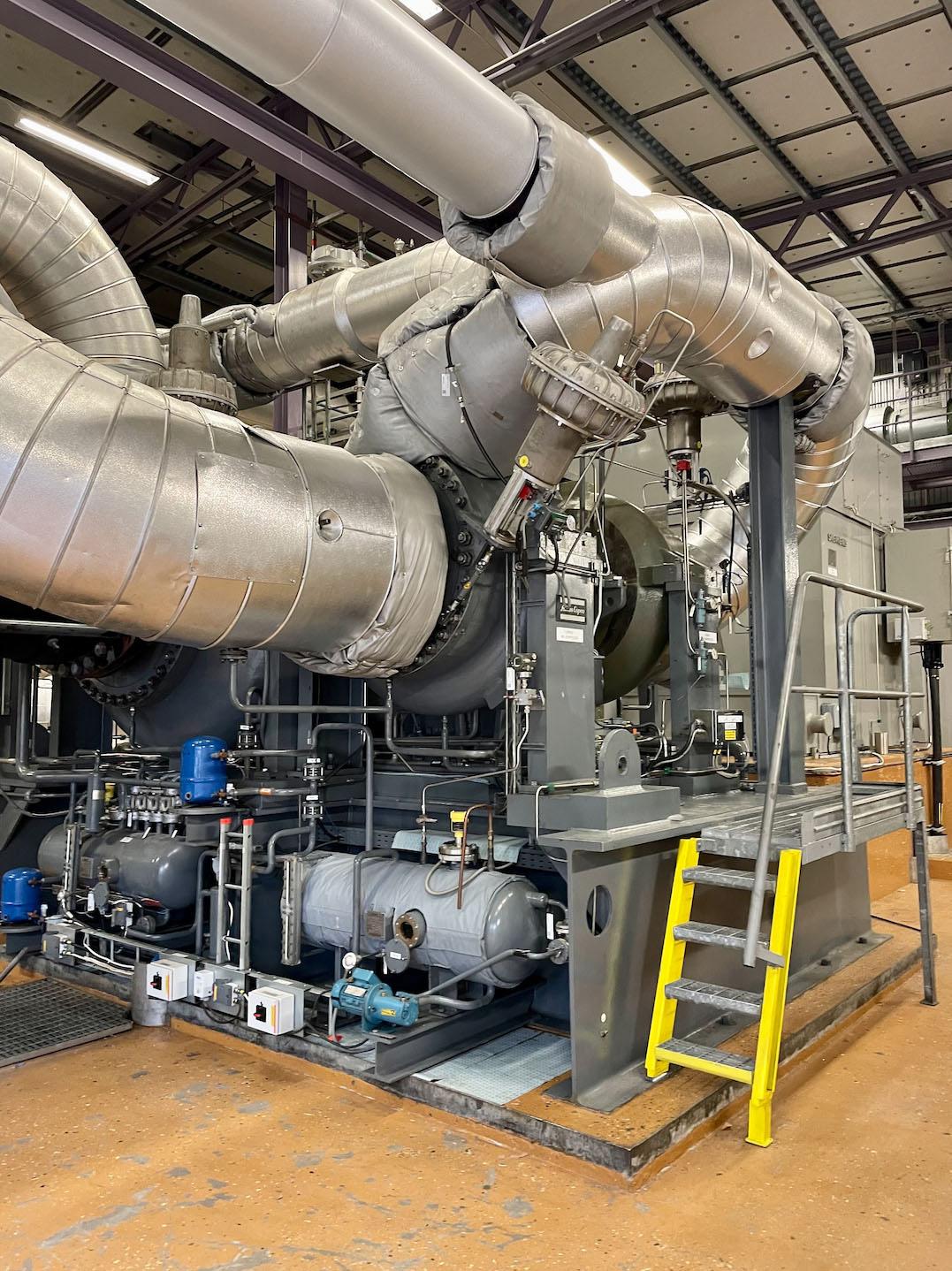
A heat pump can be used here instead of the cooling tower. Since the sugar production apparatus is an expensive, specially manufactured component, the heat pump has to meet the existing requirements for the available heating medium, which is usually water vapour at low pressure.
A heat pump with an oil-free integrally geared turbocompressor can easily achieve the necessary temperature rise from 50˚C to 140˚C to produce the required steam at 2.5 bar. Due to the higher temperature range, the COP is lower, but it still achieves good values of approximately 3, depending on how low the cooling water is to be cooled.
Example: Hydrogen electrolysis
No system has perfect efficiency, and this is especially true for large scale electrolysis for the production of green hydrogen. The prevailing polymer electrolyte membrane (PEM) technology achieves 60 – 70% efficiency. With a 100 MW electrolysis, approximately 30 MW waste heat is generated at a level below 80˚C. In industry in particular, upcycling this waste heat represents an easy way to exploit the full potential of the hydrogen economy: on the one hand, replacing fossil fuels with hydrogen where really necessary (such as in furnaces, cement, and metallurgy), and on the other hand, replacing fossil-fuel generated steam with hydrogen ‘green steam’ from the waste heat of the electrolysis.
Proven turbocompressor technology
The optimisation of industrial heat pumps represents a system with a number of variables. Larger outputs generally require high-performance turbomachines, while continuously operating production processes in industry require maximum reliability and economy.
Integrally geared turbocompressors represent a technology that has been tried and tested for decades, and they can be ideally combined with the above-mentioned requirements for performance, reliability, and economy. Due to the possibility of
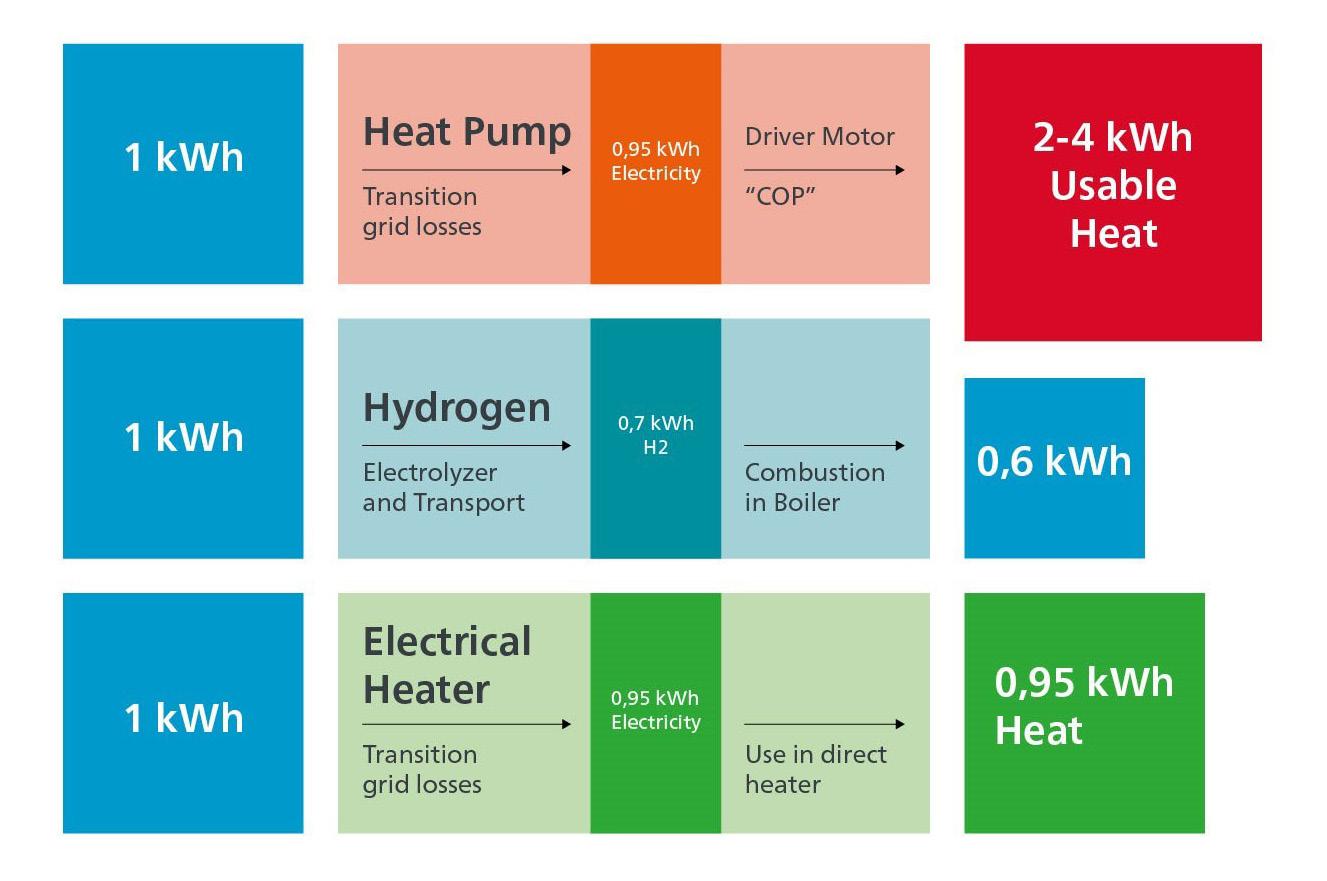
flexible arrangements of up to eight individual compressor stages on a gearbox, there is the possibility of multiple inter-stage cooling. The COP benefits enormously, especially in the case of heat pumps with a large temperature lift, but the setup of the machine remains simple because only one drive motor is required.
To compensate for fluctuations in heat demand, the compressor stages can be precisely controlled via inlet guide vanes. Due to this entirely mechanical control, expensive power electronics for speed control can be dispensed with in most cases. Especially in critical applications in large production plants, a simple, mechanical power and capacity control is usually a preferred option.
Customised systems welcome
Figure 5 . By combining heat pumps and steam compression systems, steam generation from even very low grade temperatures is possible.
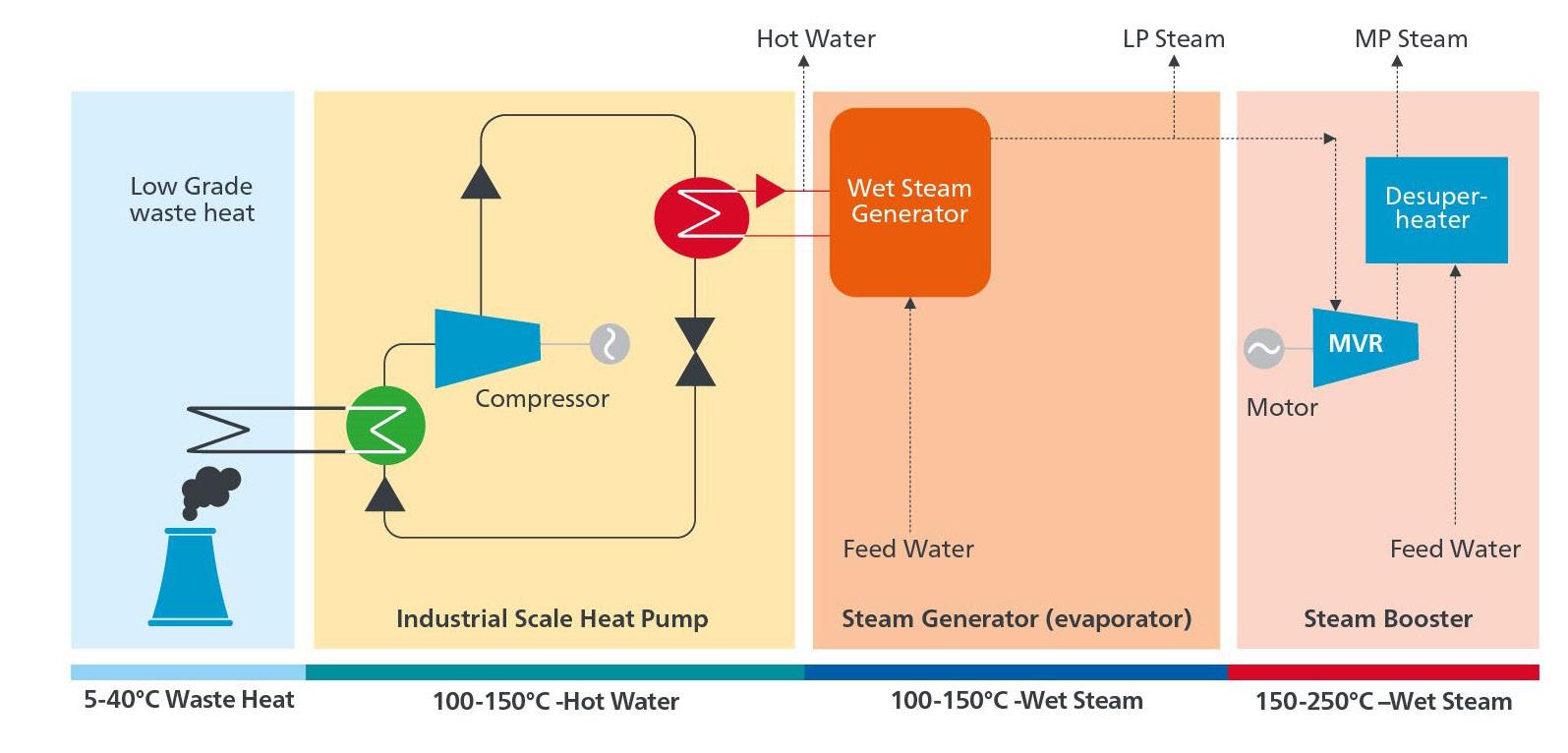
However, the secret star of the heat pump is the refrigerant. Without beneficial properties, no heat pump can achieve high COP values. Many systems with lower performance use components from the heating, ventilation, and air conditioning (HVAC) industry, which is why established refrigerants such as hydrofluorocarbons (HFCs) of the latest generation (such as R1233zee and R1234yf) are also used here. Manufacturers promote these refrigerants as a future-proof choice, with no negative impact on the ozone layer and very low global-warming potential. However, long-term consequences, such as the environmental impact of the degradation-product trifluoroacetic acid (TFA), raise legitimate questions and become more important given the normal service life of Atlas Copco Gas and Process compressors is over 30 years. With filling-quantities in the ton scale, the high price of HFCs is accompanied by its high weight, while natural hydrocarbons in the form of LPG are freely available and at low cost.
Figure 6 . Depending on the heat source and sink temperature, Atlas Copco Gas and Process heat pumps can use a wide range of suited fluids for the given application.
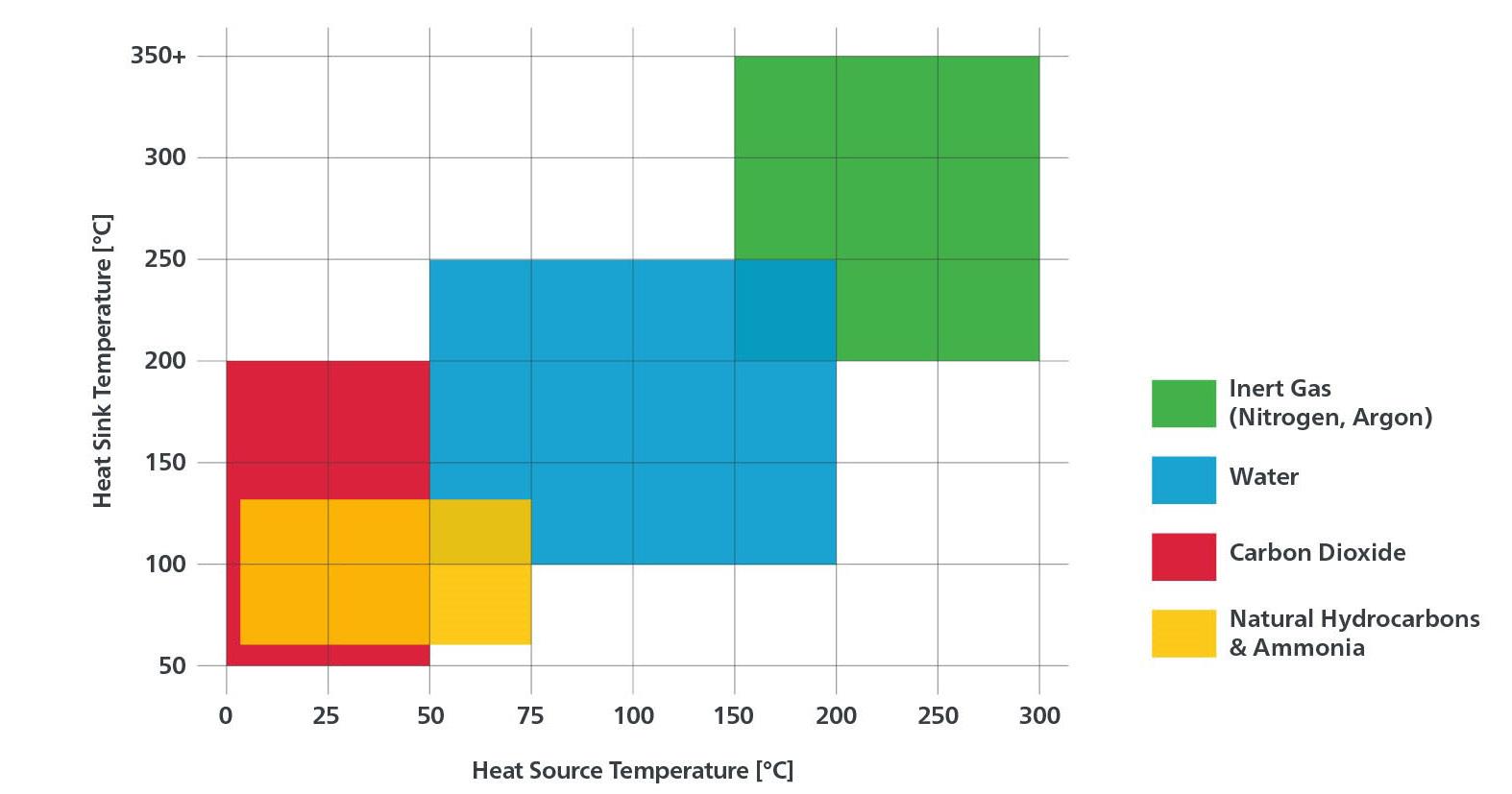
construction of the core component of the heat pump: the centrifugal compressor.
Because of these points, Atlas Copco Gas and Process heat pump compressors use natural refrigerants, specifically hydrocarbons, water, inert gases (nitrogen and argon), and carbon dioxide. Of course, machines with HFCs and ammonia as the working fluids are also available.
As an expert for turbomachinery in oil and gas processing, the handling of combustible gases is an everyday task for Atlas Copco Gas and Process that fits into any machine concept without problems. A suitable configuration can be created from the modular range of machines for applications up to 500˚C, with the different temperature ranges requiring different refrigerants and designs.
Focus on the core know-how: Integrally geared turbocompressors
Reflecting on one’s strengths is a good way to be sustainable and successful in life. And true to this motto, Atlas Copco Gas and Process focuses on the design and
This means that a strong engineering/EPC partner is needed to build a complete heat pump system. Based on many years of experience in working with internationally active EPCs, Atlas Copco Gas and Process selects the optimal partner depending on the requirements of the project. For projects in Europe, for example, it has an exclusive partnership with EPC STRABAG Environmental Technologies.
Summary
There are three obvious reasons for using heat pumps: CO 2 emission reduction, cost reduction (fuel savings, CO 2 emission certificates, tax breaks, and so on), and security of supply.
The heat pump as an industrial technology has been mature for decades, but it has existed for years in the shadow of fossil fuels due to a lack of need. Now, however, with sustainability demands and the price of fossil fuels, it is fair to say that the age of the heat pump is beginning.
Regardless of the motivation behind the investment decision for an industrial heat pump, in the end it is a further step towards the decarbonisation of industry, something that is urgently needed to meet the climate goals of the Paris climate agreement.
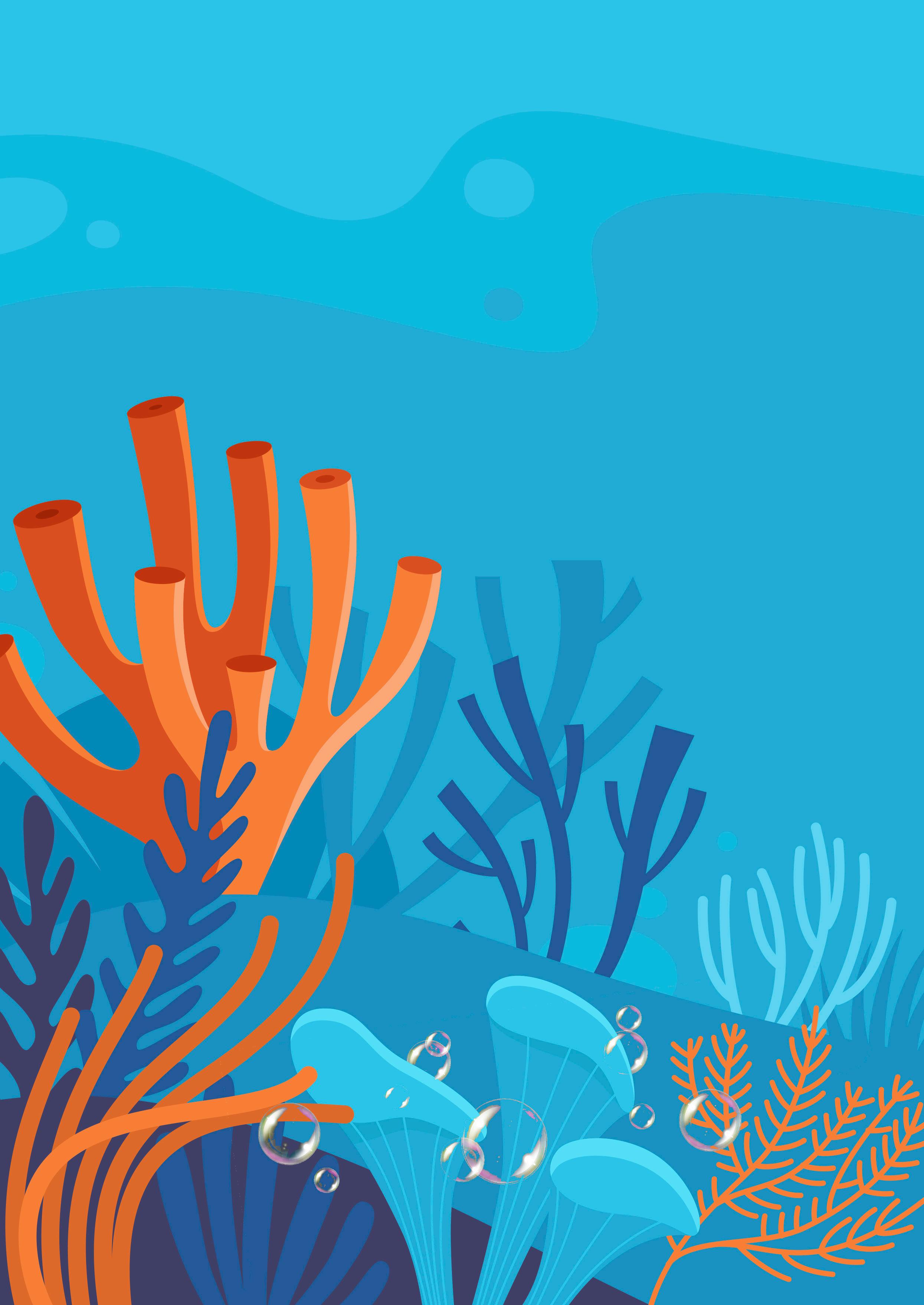
Austin Harbison, Smart Technology Manager, CRP Subsea, UK, talks about the importance
of safely
protecting subsea electrical cables in the shallow waters of offshore wind farms.
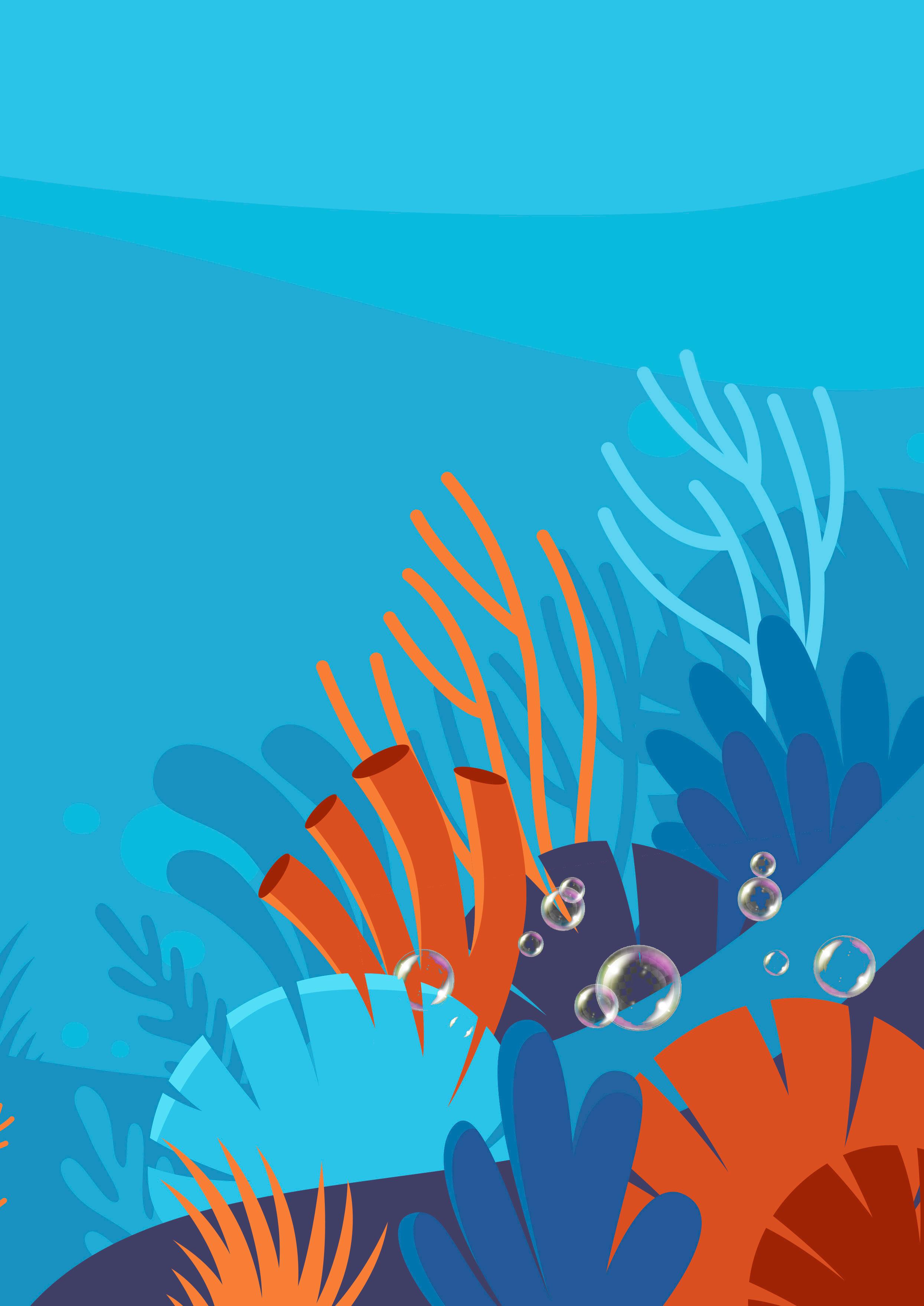
s the world balances its transition from fossil fuels to renewable sources of energy, the changing tide of supply will inevitably lead to unforeseen industry challenges and failures, resulting in new approaches and improvements to support further growth and cost-effectiveness in these sectors. These lessons must be learnt in order to see how best to identify and adapt so as to guarantee reliable energy supply and security in the future.
Over 30 years ago, offshore wind farms started to emerge in Europe, with slow adoption through the turn of the millennium, to almost meteoric growth over the past decade, and ever more coming online as 2030 comes closer.
Development focus has led to great gains in the most visible part (the turbine and its blades), but as a result, is the whole system being mistakenly labelled a mature technology not in need of further investment and improvement?
As with any system, it is only as strong as its weakest link, and some are beginning to argue that its very connection to people’s homes might just be that. Given the environment in which these systems are immersed, and the unforgiving nature of coastal waters, small risks are being exacerbated, but through the lessons of failure, new approaches are uncovered to mitigate, analyse, and eliminate them.
To facilitate the world’s burgeoning thirst for renewable electricity, submarine cable production has been increasing like never before to meet with the demand for offshore wind, with the cumulative deployed length of cables now exceeding the diameter of the planet, and an eight-fold increase coming online within the decade. Thus, any risk to their integrity could impact the
entire industry and, without cable redundancy on most fields, any single point failure could lead to considerable loss of power to homes and businesses.
Cable protection products
CRP Subsea has spent nearly half a century refining its cable protection products, to either offset cable weights, or to protect them from abrasion, overbending, and fatigue. With over 100 000 buoyancy modules deployed and over 300 km of cable protection supplied, the product’s tenure is not in doubt. But these numbers, whilst impressive, pale into insignificance when compared with the lengths of cables now being deployed globally. However, CRP Subsea’s focus is not the overall cable itself, but arduous hot spots along it where, without additional support, it may suffer catastrophic failure.
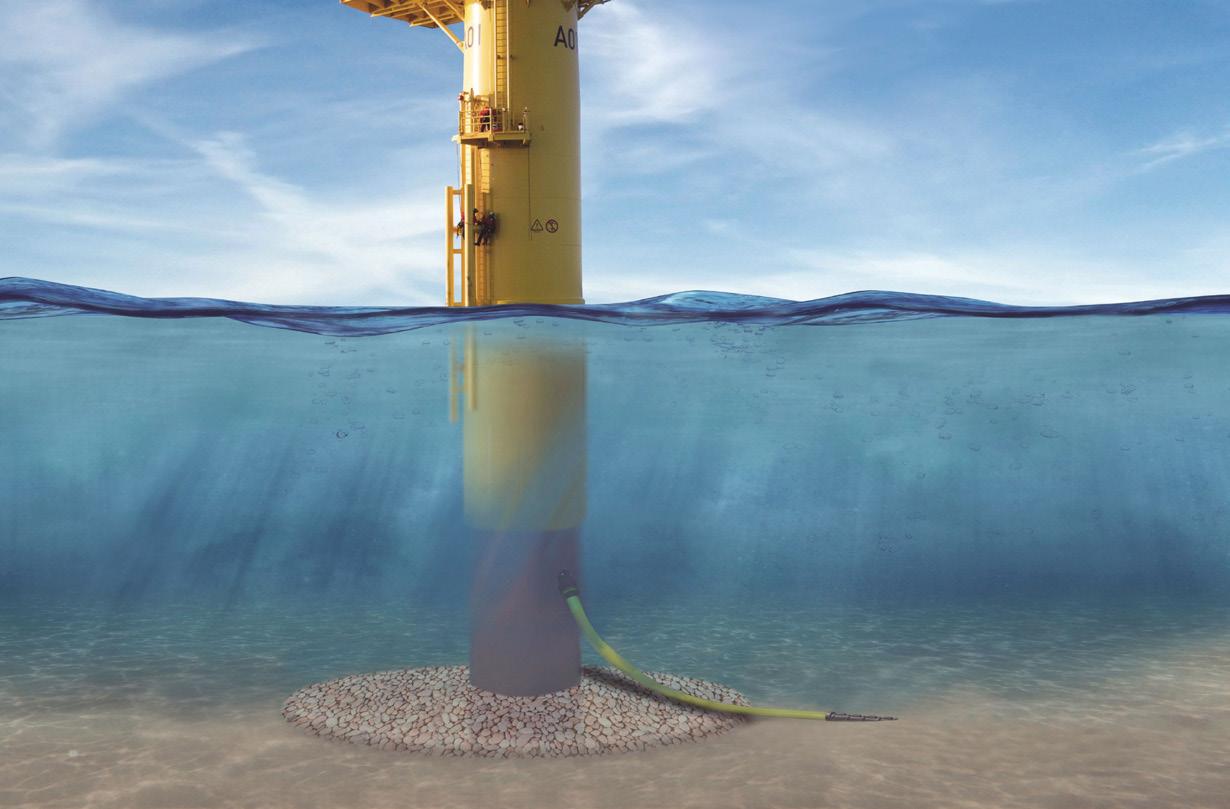
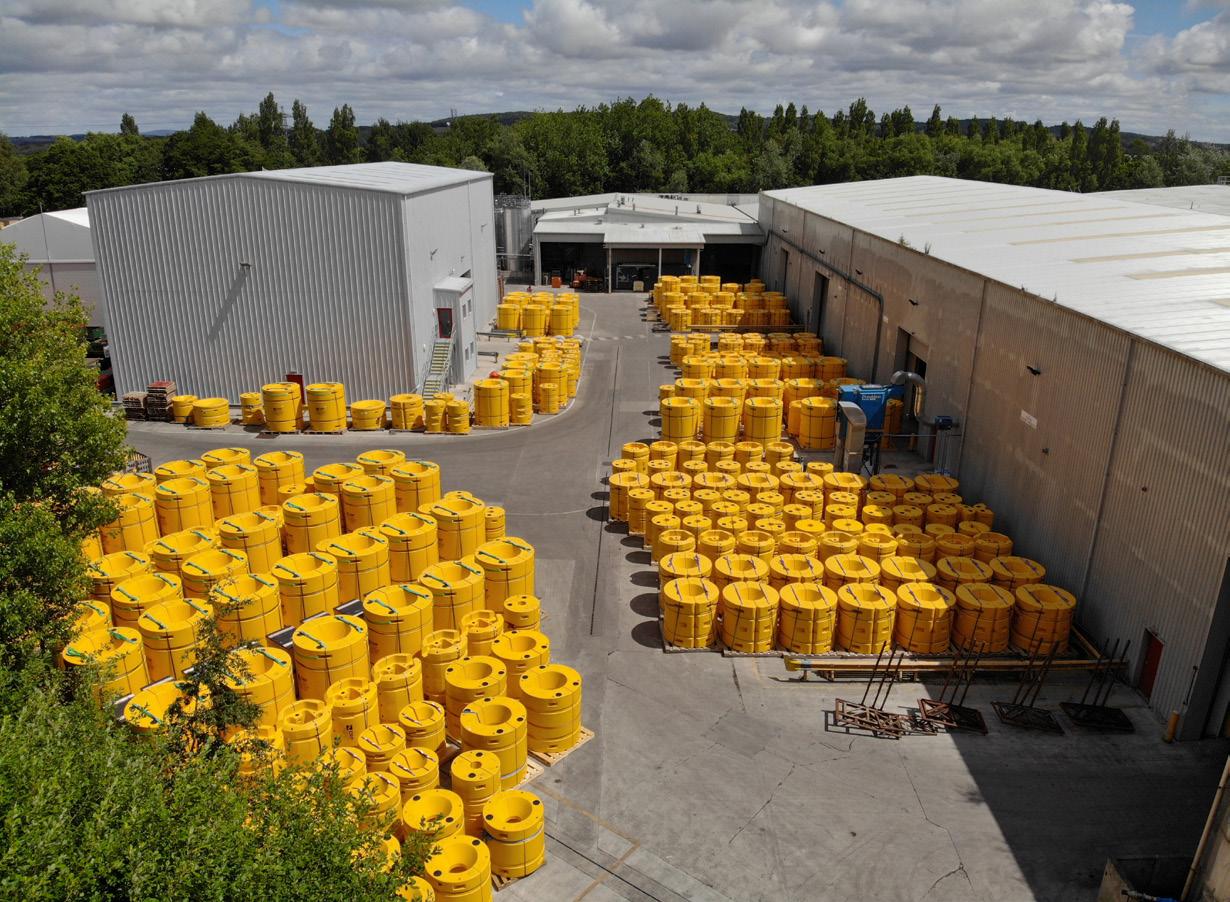
One such location is the transition of the cable from the security of burial in the seabed toward its connection
to the turbine’s monopile. Cable management during the installation of such inter-array cables sees great care taken in the cable’s submergence and movement toward its connection point, offsetting historical installation failure risks. The cables are laid in such a way as to eliminate overbending or stress – as they are pulled toward the connecting turbine, they are provided with protective cladding (which accurately controls bending radii) as winches on the turbine pull them ever closer, then upward into the turbine’s monopile without a kink. This protective cladding generally meets the point of entry of the monopile, which could be a cut orifice or j-tube, where the cladding becomes permanently affixed to the pile, permitting the subsequent cable to be hauled through it without overbending. This cladding, evolving to support safe cable installations, can then also be used to strengthen the elevated section from the heave and sway of waves and tidal currents as they accelerate around the pile and over the sandbank. Thus, the cladding, or cable protection system (CPS), has a dual purpose: 1) to aid installation, and 2) to mitigate this hotspot’s operational response to the hydrodynamic environment. The primary goal of both purposes is to reduce overbend and excessive movement.
Prior to the cable’s insertion into the pile, a blanket of rock is laid to protect the pile’s foundation from localised erosion, created by the water currents accelerating around it. This scour protection, which the CPS sits atop, introduces the first operational risk to the cable’s integrity: abrasion. The second most prominent operational risk involves a slower, but no less severe, deterioration. As the cable moves back and forth in the water current, it undergoes cyclic tensile strain, which will inevitably lead to cable fatigue failure.
Overcoming operational risks
As initial CPS designs sought to reduce overbending damage during installation, the operational risks of fatigue and abrasion were not as well understood; as such, the primary component, seen in legacy CPS’, were static bend restrictors, whose articulating form would lock up at a designated curvature to inhibit overbend. This product is commonplace within oil and gas, where they are used to stop overbending during installation, when cables are lifted, or when they are pulled into a structure, and have long proved effective. The difference in this application is the operational phase of such a system. If a cable sits within protection that locks out at a curvature, it does not dampen movement until it hits this hard-stop, introducing an impact both to the cable and its protection. Furthermore, its increased diameter in the water current can actually double the
Figure 1 CRP Subsea site in Skelmersdale, UK.Global Energy Transition 2023
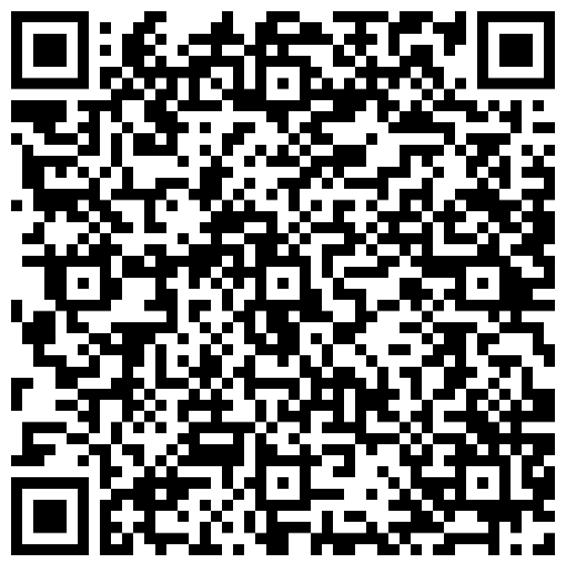
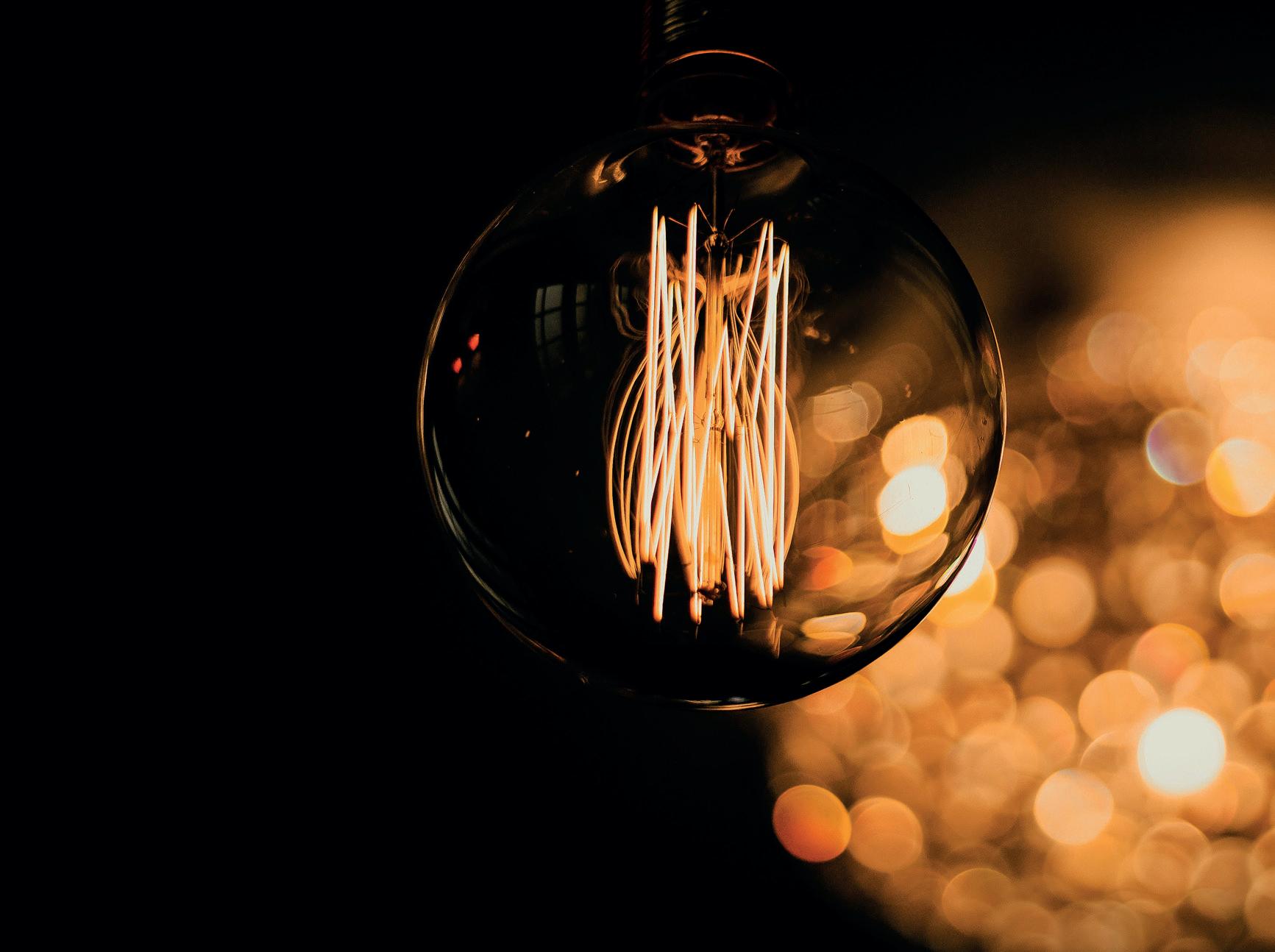
distance it travels, with predicted movement accruing over 10 000 km of travel back and forth. Unfortunately, this is not the end of the story as the material property which provides the strength to lock out at curvature makes it more brittle and subject to increased abrasion.
Again, looking to the learnings from oil and gas, any cable subject to dynamic loading will utilise a cone-shaped system called a bend stiffener, which stiffens the cable through the use of elastomeric (rubber-like) material. This is selected as it dampens and inhibits movement, acting against water currents, whilst also offering a more streamlined profile than restrictors. Such products have been deployed for decades on oil risers without failure. Furthermore, its elastomeric nature greatly increases its inherent abrasion resistance, with added sections being designed with yet more suitability to combat abrasion as it meets with the scour bed of rocks. This design is now preferred across the industry, as it better manages both installation and operational risks, with retrofittable variants
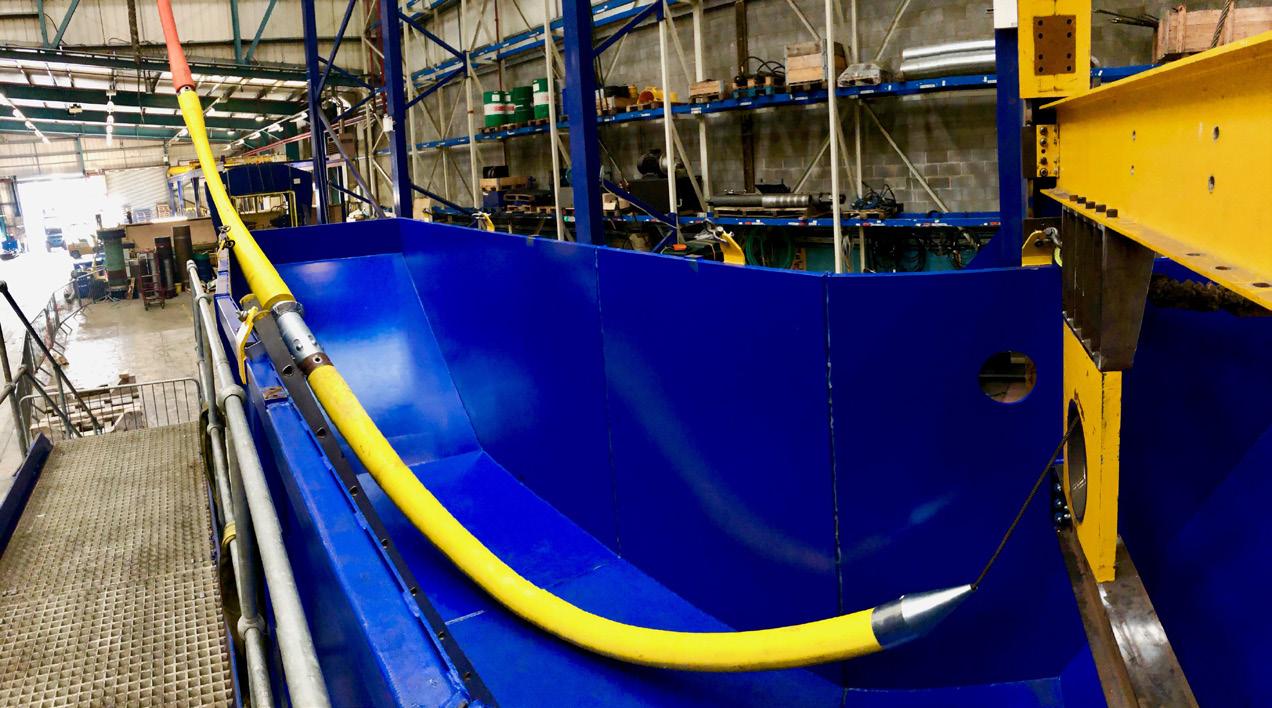
of CRP Subsea’s bend stiffening design, NjordGuard, being used to shore up legacy bend restrictor designs.
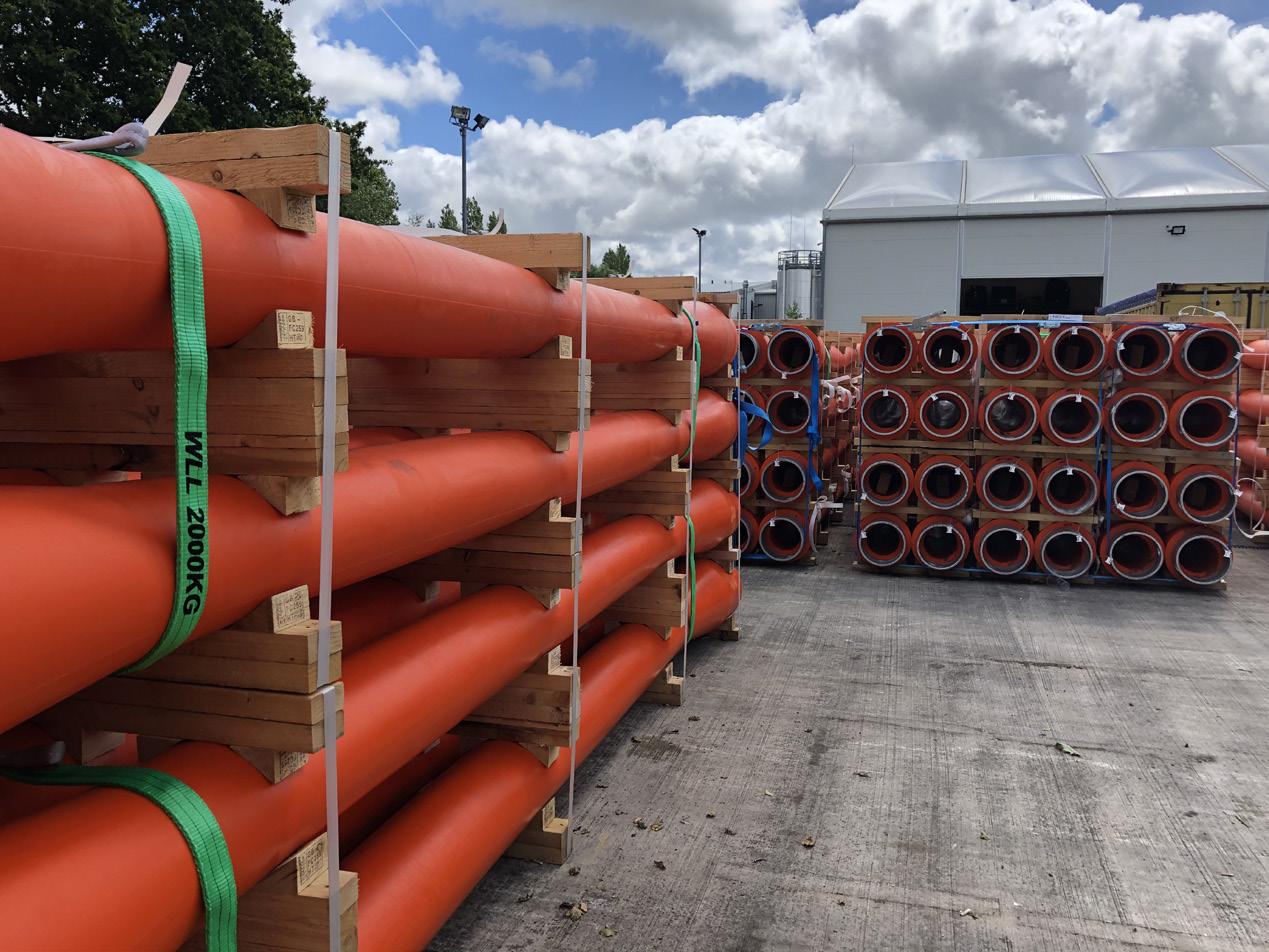
As wider appreciation of protection system types take hold, so too does the response of the cable at this location. One of the most vital aspects to any protection system design is the predictive software used to determine the extent of movement. Global analysis software, originally designed to predict riser motions in deep water, has been repurposed to predict cable movement at wind turbine sites; but, as with any model, it is only as good as the conditions and constraints placed within it. Unfortunately, the company’s knowledge of these sites is currently brought about by the costly findings of failure, as locations prove to have more complex bathymetric aspects, such as migratory sand banks, and more complex hydrodynamic flow patterns, with individual idiosyncrasies at each field and even each location, each one far beyond the capability of modern analysis to fully predict. With extensive and constantly expanding design cases seeking to cover every eventuality, even the most encompassing prediction is based on an oversimplification of the field itself.
In an attempt to manage the almost infinite variation needed in design, secondary mitigation of rock dumping on top of the CPS is re-emerging, firstly to shore up current fields deemed at risk but also to add perceived stability to new ones. This method is not without its pitfalls; aside from the cost of vessel mobilisation, it places new risks onto the cables as heavy rocks impact, compress, and shear them, further restricting the ability for replacement. If they are insufficient in suppressing the motion, does that mean ever more rocks must be dumped on to the site? What if the seabed migrates, or the rocks bridge over the cable? The biggest challenge here is the unknown or unquantified effectiveness.
Looking once more to oil and gas, concrete mattresses or rock dumping is seen as a challenging mitigation to implement effectively, coupled with the disadvantage of permanently entombing the pipeline and potentially not restraining tensile fatigue along the pipe’s axis, as thermal cycling creates the caterpillar effect of axial walking. The alternative here is axial resistors (motion stabilisers) placed along the length of the pipeline to increase soil adhesion, gluing it to the bed, but in any event the biggest challenge remains the unknown response to mitigation.
If a Six Sigma improvement framework is considered for a moment, such as DMAIC, the problem and output to be achieved is defined, it is measured by collecting data to establish a baseline for improvement, the data is analysed to understand its implication,
Figure 4 . NjordGuard scour protection sections awaiting collection.the process is improved, and controls are implemented to sustain those improvements. There is a well-defined problem to address, with established software to aid root cause identification, but there is little, if any, actual field measurement to complete this analysis, improve the company’s approach, or control it by inspection. Offshore inspections identified during initial risk assessments cover everything from pile corrosion rate tracking to the reliability and predictive maintenance of the turbine machinery, with their mitigating backup systems, controls, and omnipresent support crews, but what of the cable itself? If movement with associated abrasion and fatigue are the emerging risk factors associated with long-term cable integrity, inspections which merely check the cables’ location every few years omit actual measurement and eliminate the ability for the industry to analyse, learn, and improve future systems. If current inspections only identify failures when it is too late, there needs to be a push for data driven measurements based on field accrued data or people will forever learn by failure.
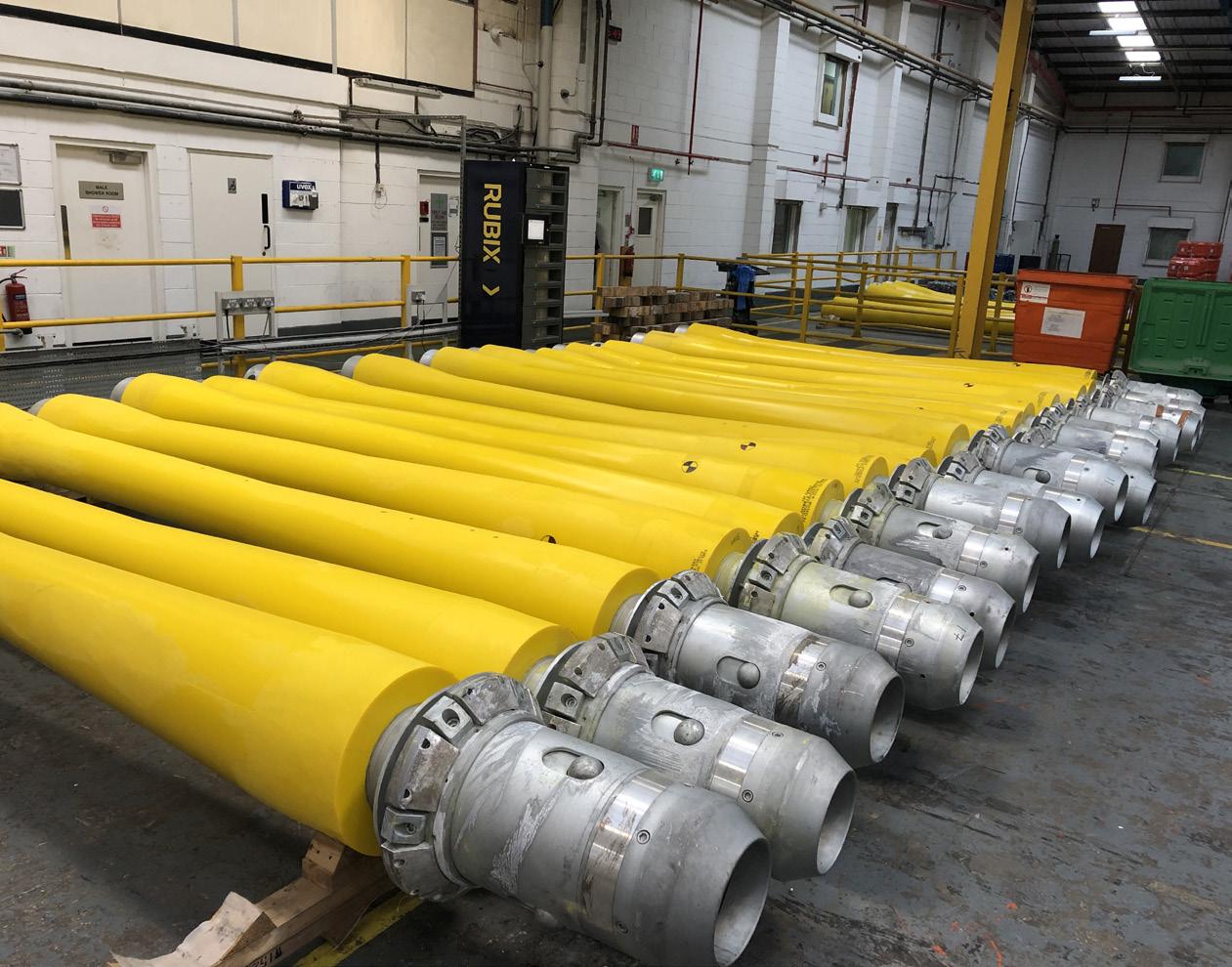
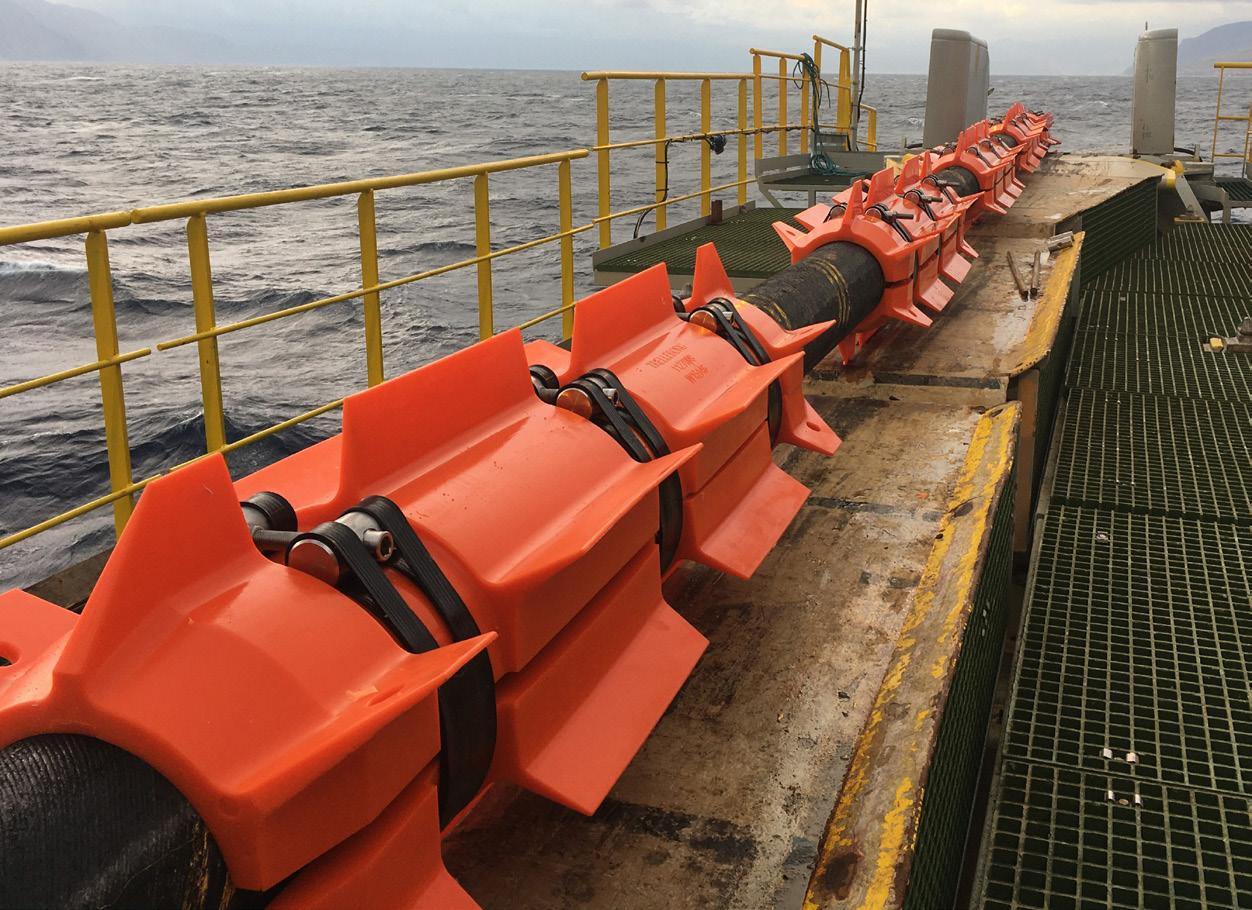
As with improvements in cable protection devices, the ability to monitor is scaling to meet this need, with further support from operators, monitoring can be deployed by small vessels and smaller remote operated vehicles to capture key motion data, better informing operators as to the health of their cables. Subsea Insight, an independent subsea integrity monitoring company, in collaboration with CRP Subsea, have developed a fully-integrated monitoring device for inclusion in CPS systems to aid tracking and provide closed loop design improvements to their products. Subsea Insight’s products are also seeing deployment as a retrofittable solution on existing fields at minimal cost and impact, due to their ability to sequence with typical site inspection schedules. This approach is providing billions of data points, capable of correlating met ocean conditions to responses and introducing data-driven models to predictive software, aiding in life expectancy calculations, whether on mitigated or unmitigated sites – not just capturing motion, but also thermal changes and impact to marine ecologies.
The next phase
The next phase of protecting subsea electrical cables lies no longer with CPS or stabilisation approaches, but with the field measurements that can bring about a conclusion to it; confirming life expectancy is equivalent to the turbines they connect and enabling data guided refinements to future systems. Just because there are questions on what the data will look like and how it will be interpreted, this is not a reason not to monitor, it is
validation that what will be found is unknown. True subsea insight into systems should not be seen as arduous. Indeed, Subsea Insight are actively working with partners to bring about resident autonomous vehicles which can correlate met ocean data with cable monitoring hubs to build quasi-real-time tracking of cable health, and to identify at-risk locations where mitigation may be needed in the future, allowing for efficient planning in the event that some locations see more adverse conditions than others. The industry has learned a painful lesson in the past few years, but it must not stop learning about the environment in which it now wishes to place its energy infrastructure. The industry should not continue learning by trial and error, but by the data the current systems can provide.
Figure 6 . Motion stabiliser installation.he UK’s offshore wind industry is in a unique position to act as a springboard for broader maritime decarbonisation, being both a potential producer and user of clean fuels.
Using clean energy is expected to make up a far greater part of the maritime fuel mix of the future, whether that be by directly using the cleanly generated electricity or by converting this clean energy into combustible fuels, such as green hydrogen.
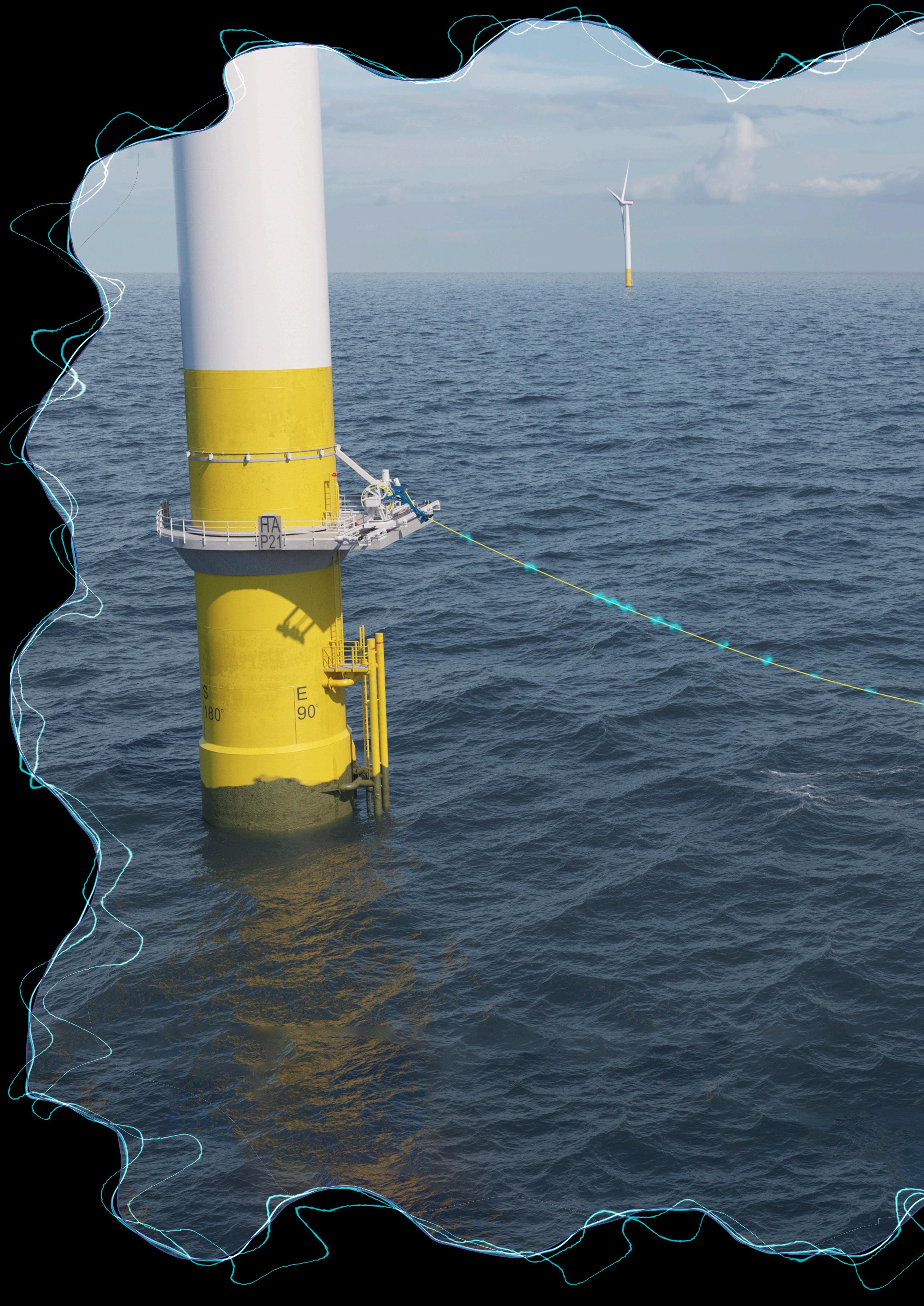
MJR Power and Automation – alongside a consortium of partners – is at the very forefront in driving the transition to utilise the energy generated by offshore wind farms and create fuels for the maritime industry.
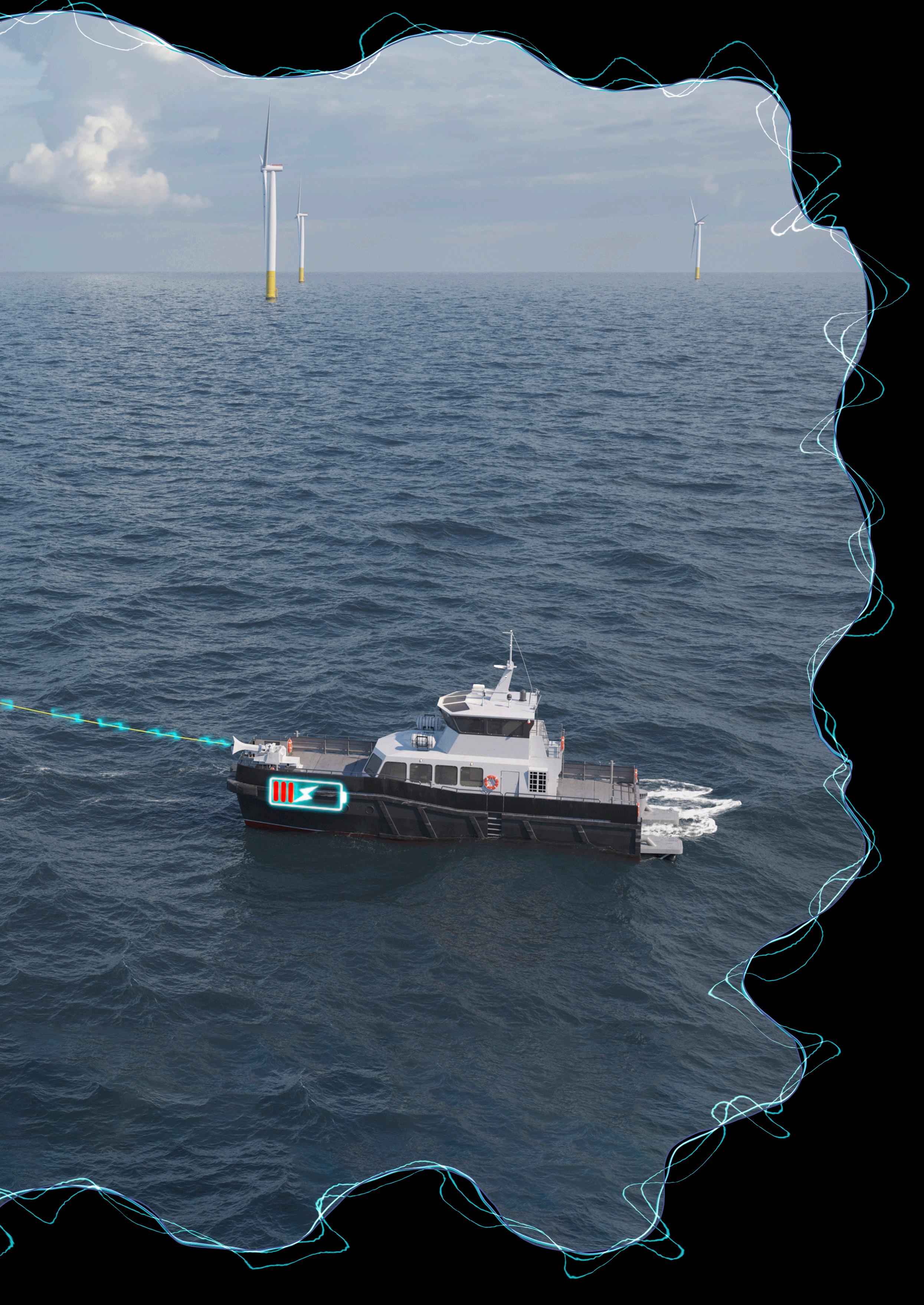
The challenge
Presently, most offshore wind turbines are maintained using marine gas oil (MGO) powered vessels, most notably crew transfer vessels (CTVs), which are calculated to contribute
technology can revolutionise the future for electrification in the
10 – 20% of the lifecycle carbon emissions of an offshore wind project. Converting or replacing MGO-fuelled CTVs with vessels powered by clean fuels is estimated to save between 1278 – 2500 tpy, depending on vessel type, of carbon dioxide (CO2) emissions, per vessel replaced.
With offshore wind farms typically chartering several CTVs each, and the number of CTVs required to service
the industry set to grow rapidly over the next decade, the industry must act now – and it is paving the way in doing so – to significantly reduce its future increased emissions.
Addressing the challenge
The industry as a whole is very much focused on accelerating the electrification of the marine and offshore sector, which has seen industry-wide collaborations formed to achieve decarbonisation collectively, with widespread adoption of clean fuels on vessels, as well as big industry players setting clear commitments to reach targets of net zero by 2025.
One accessible route to reducing the carbon footprint of offshore wind operations and maintenance activities is through the adoption of electric CTVs (eCTVs). However, this presents several restrictions, as limited battery storage energy density severely reduces the operating capability and range of eCTVs, thus limiting their adoption and the decarbonisation of this area of the industry.
Having a method to charge batteries in the field – ideally during periods that vessels would otherwise be spent idle – will be a key enabler for the large scale deployment of eCTVs and help achieve the carbon emission reduction targets of the industry.
Taking the power back
As such, to meet the industry’s need to significantly reduce its emissions, MJR Power and Automation has successfully fast tracked the design and development of an electrical vessel charging system following the company being selected as winners of the Clean Maritime Demonstration Competition, receiving funding from the Department for Transport (DfT), with the scheme being delivered in partnership with Innovate UK.
The company has led a consortium of partners – ORE Catapult; Xceco; Artemis Technologies; and Tidal Transit – each with expertise in their given field to develop the first of its kind technology.
The charge points enable all eCTVs and other offshore support vessels to connect in the field to a 100% green energy source generated directly by the offshore wind turbines for the efficient, safe, and reliable transfer of power.
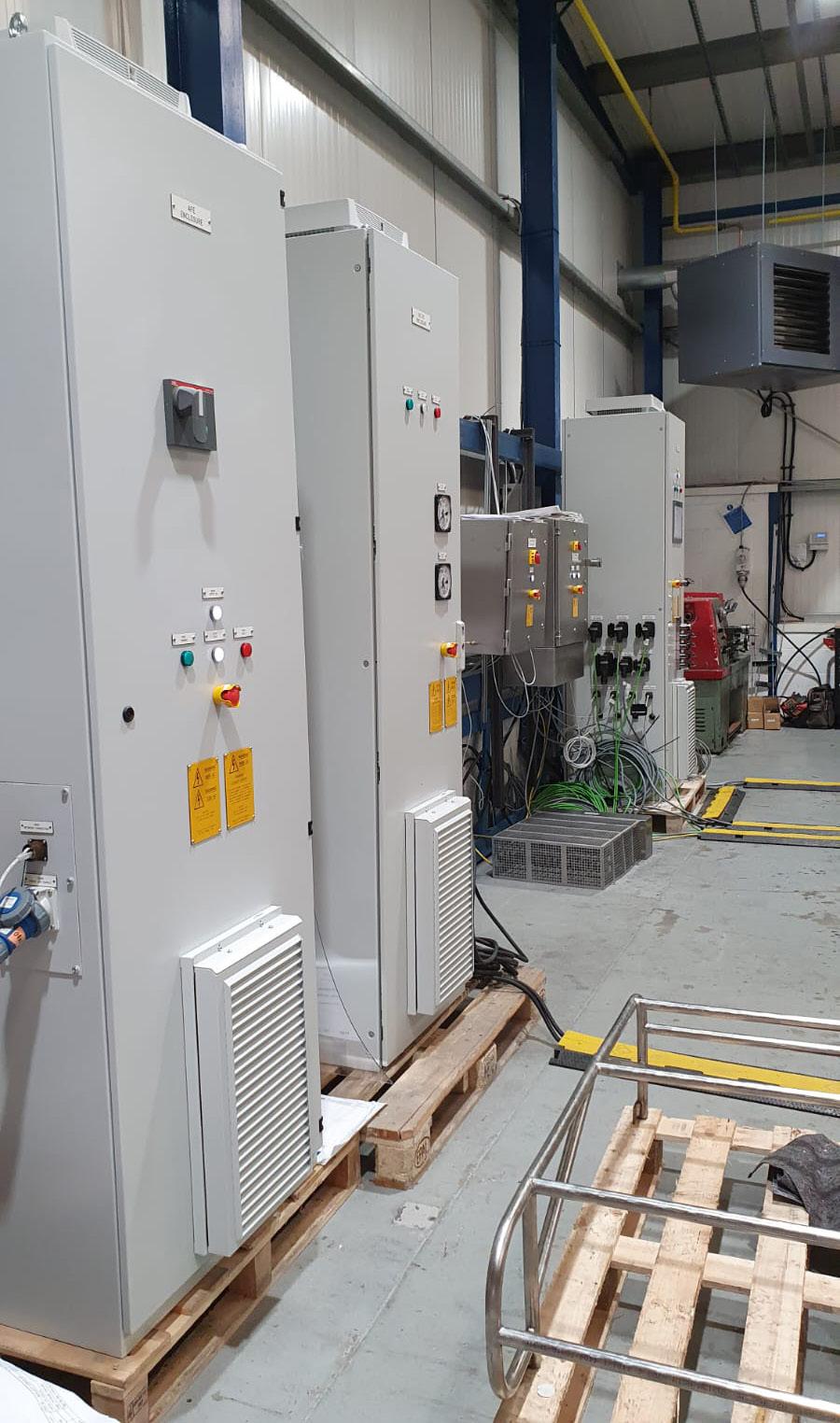
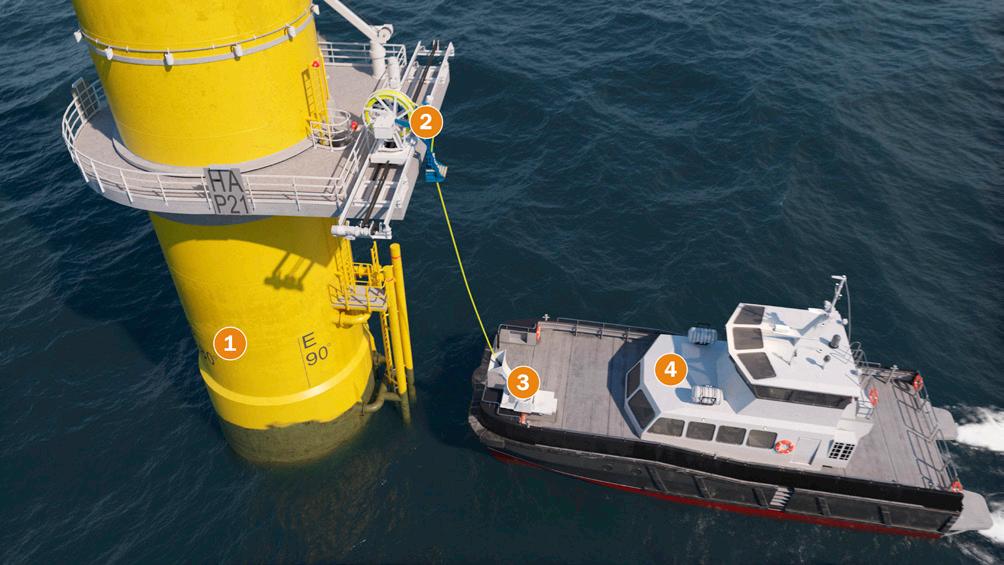
This new technology innovation – currently in final stages of testing for deployment offshore – will break down the existing range barriers and thus increase the uptake by vessel owners and operators with transition to fully electric and green propulsion systems, for retrofit and new build vessels. In combination with other field proven technologies, the charging system will be an important part for government and offshore wind owners and operators, to achieve their net zero maritime operations targets, and switch away from fossil fuels.
The ability to charge when in the field will significantly accelerate adoption of current emission-free propulsion systems which will be a major asset for the decarbonisation of the UK and global maritime sector.
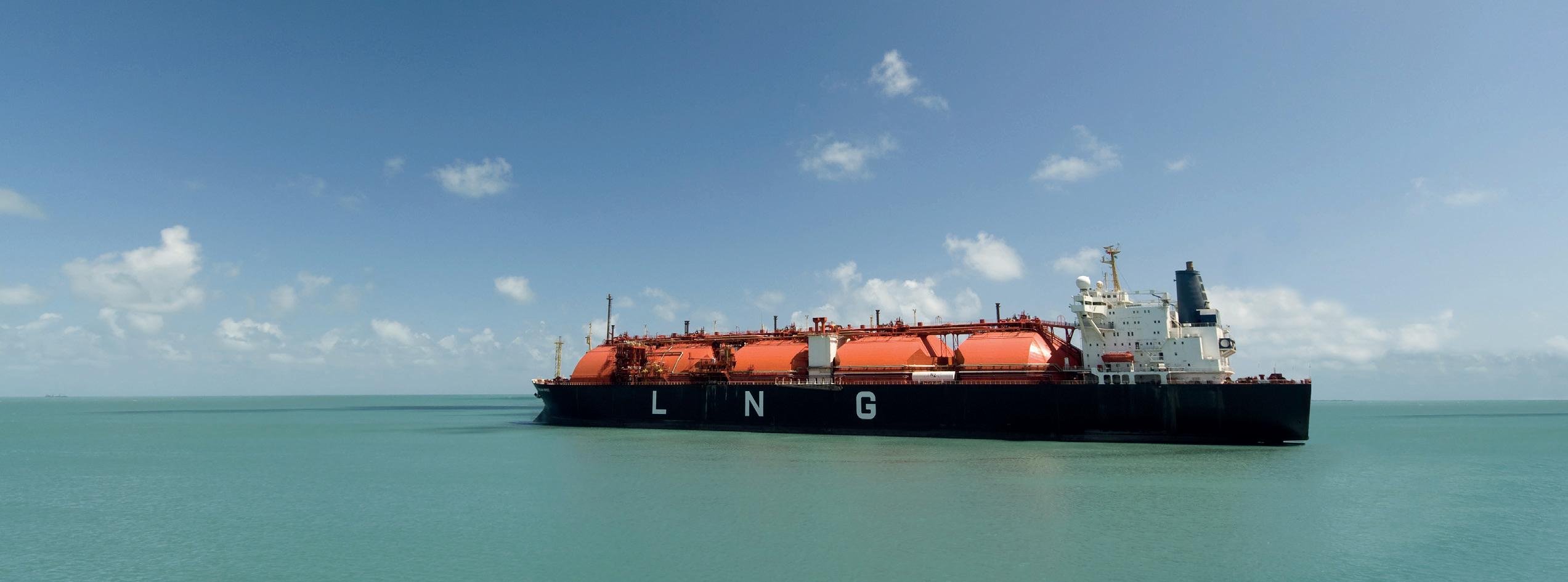
System overview
The technology accesses the infrastructure already in place, including turbine platform and electrical power, to provide renewable electricity to vessels. As an eCTV docks with the turbine, a cable reel lowers down an electrical charge connection which plugs in to the vessel and charges a battery onboard.
Although some of the technology necessary for this is relatively mature, significant technology gaps remain to making offshore charging safe and practical.
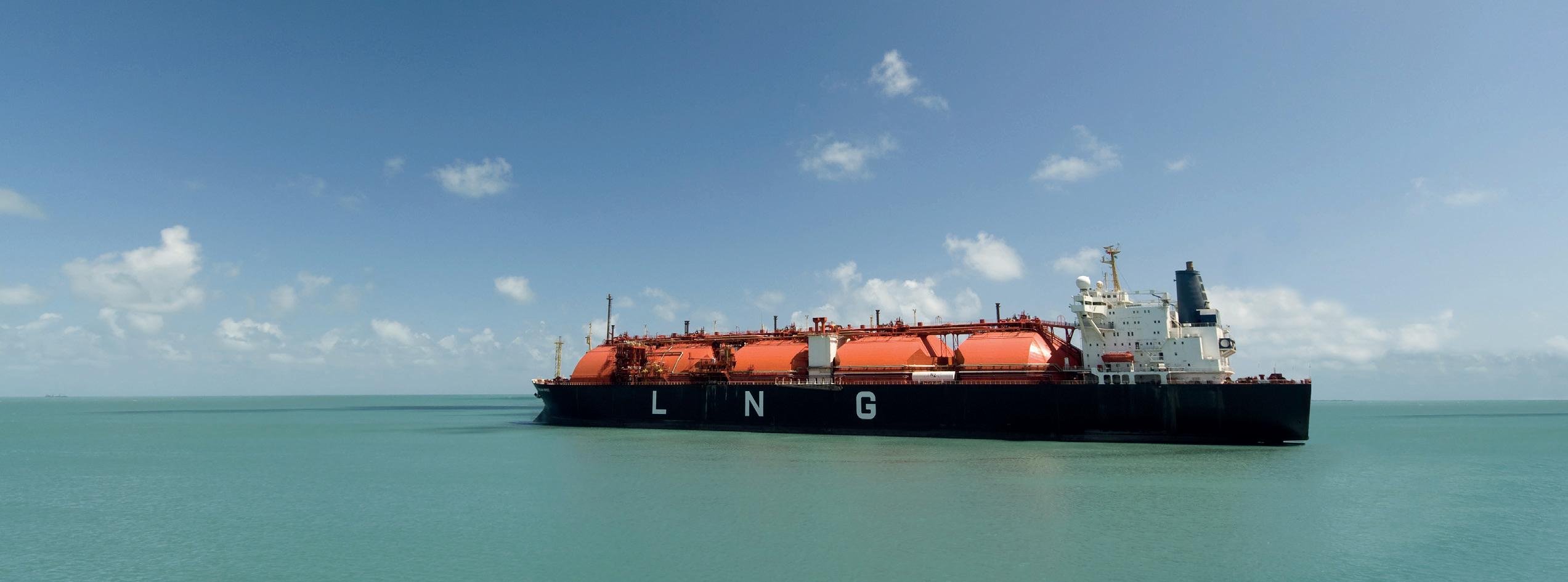
MJR Power and Automation has successfully developed the technology to fill these gaps and developed standards, working practices, and procedures in order to safely carry this out at sea.
The overview of the charging system shown in Figure 2 includes, but is not limited to:
F On turbine power converter – converts the wind farm medium voltage AC power into DC power that is compatible with the CTV propulsion and battery system (see item 1 in Figure 1 and Figure 2).
F On turbine mooring/charging cable and connector deployment system ‘reeler’ (see item 2 in Figure 2 and Figure 4).
F Vessel connection and mooring system (item 3 in Figure 2 and Figure 5).
F Control and safety system (item 4 in Figure 2 and Figure 6).
Real-time control and safety system
The technology is linked to a real-time control system to enable safe control and the charging from both the vessel and wind farm control room operating locations, and is designed to embed safety throughout the charging process.
The control and safety system has been developed according to marine classification society rules and standards, coupled with the company’s 25 years of experience of designing and delivering safety and mission critical systems to the offshore energy industry.
A real-time deterministic marine grade operating platform is joined with the added functionality of a web portal system used for the booking and monitoring of the wind turbine charging system from the vessel, the wind farm control room, and also via desktop access.
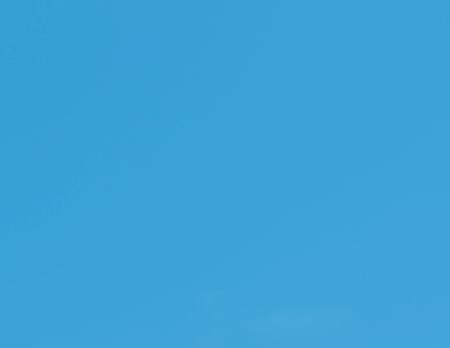
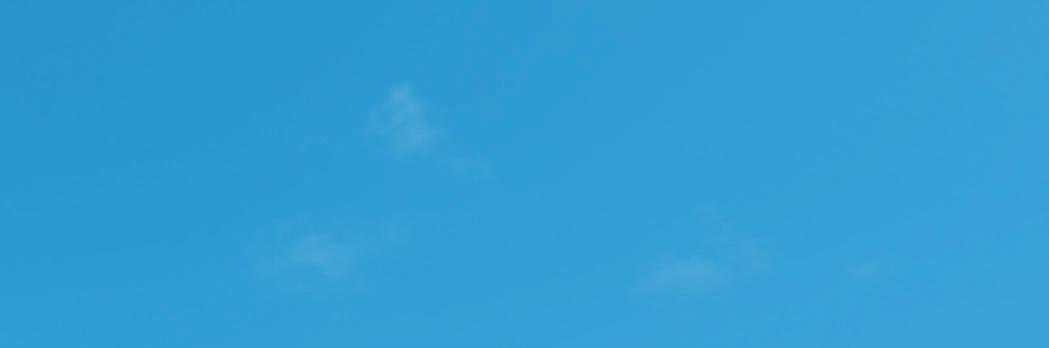
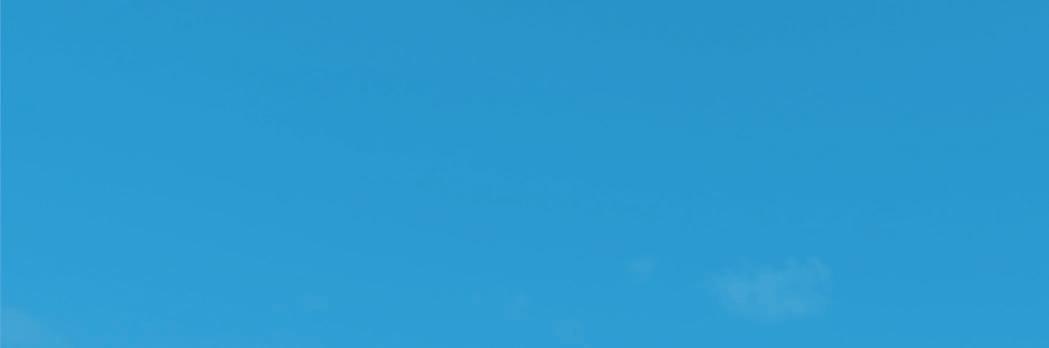
The control software has been developed for full operational control of the charging system, reeler system, and vessel connection system. The high-level functions of the control modes are:
F Manual control.
F Follow mode.
F Mooring mode.
Throughout the process of charging the vessel battery system, the vessel personnel have full visibility of the status of both the charging and deployment system with the operator control station that would be situated on the bridge of the vessel.
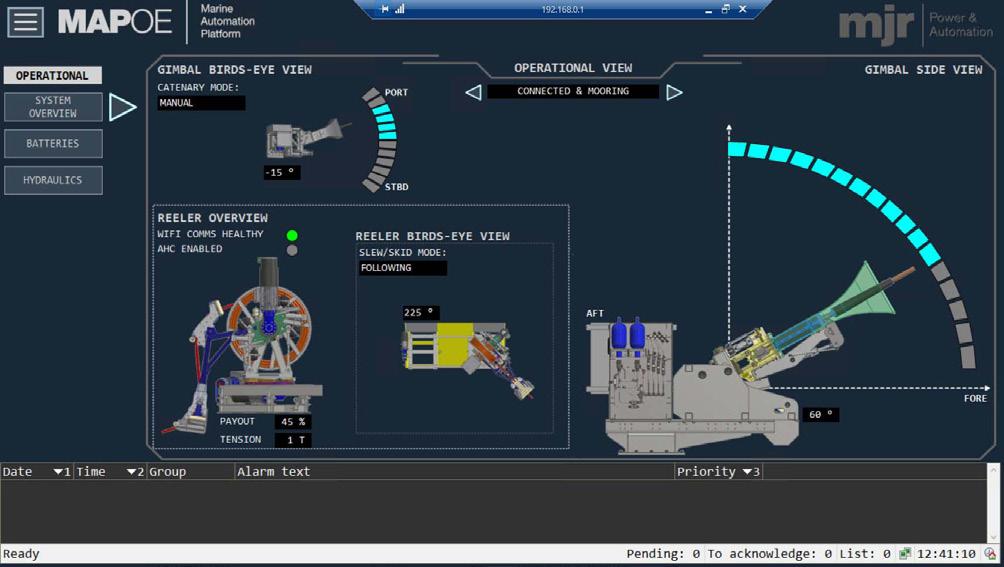
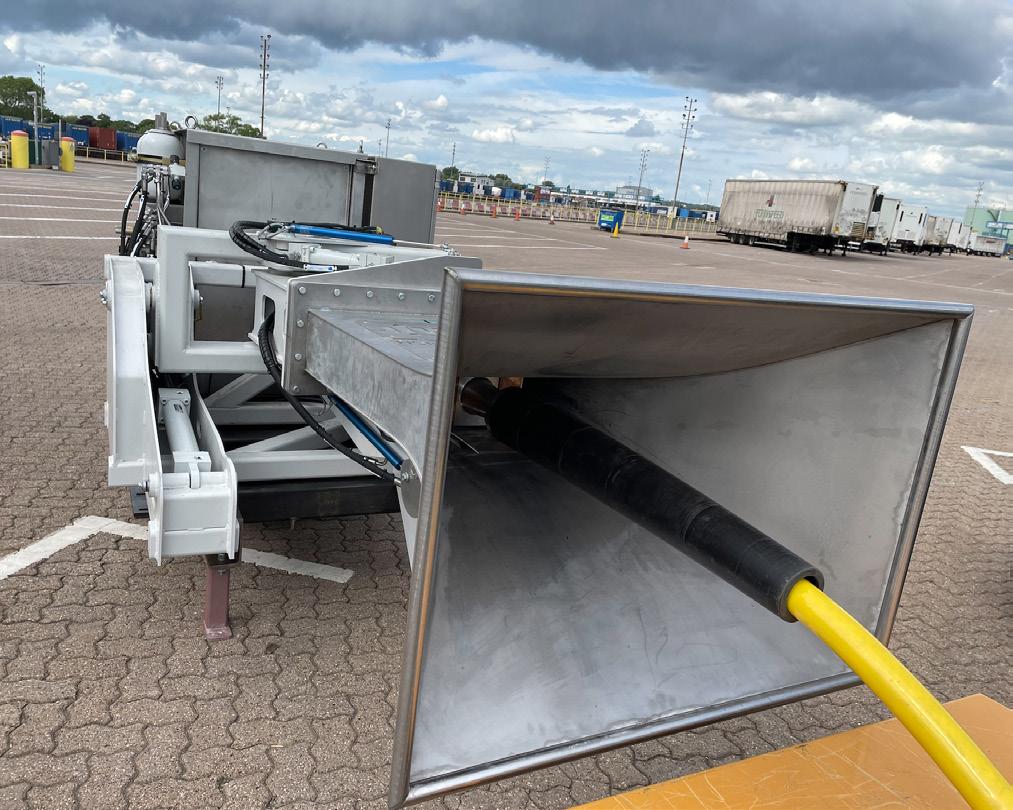
Accelerating electrification
Through the adoption of such technology, it is estimated that a single vessel switching from diesel to battery electric propulsion will save 1278 tpy of CO 2e emissions. As such, this technology has huge potential to enable the UK’s marine and offshore wind industry to seamlessly convert to electric operation of its CTV fleet.
If 50% of the UK’s CTV fleet converted to electric operation, this would eliminate approximately 131 100 tpy of CO 2. These figures are staggering and highlight that such technology and adoption of it will be absolutely pivotal in reducing emissions in the field and helping the UK to achieve net zero targets.
The offshore charging solution will enable the immediate conversion to electric vessels and the removal of over 300 diesel-powered CTVs from UK coastal waters. And with the ever-growing number of wind farms both in UK waters and across the world, this will play an integral part for owners and operators to meet their emission targets.
Industry recognition
As part of the Clean Maritime Demonstration Competition Final Showcase hosted by DfT, Innovate UK, and Innovate UK KTN, MJR Power and Automation showcased its offshore charging system earlier this year amongst 54 other projects from across the UK that also received funding at Portsmouth International Port.
At this year’s Seawork event, the company secured a highly acclaimed award and was announced as winners of the Innovations Showcase Category for renewable energy in recognition of its offshore charging system.
The business also received the accolade of the Supply Chain Innovation Award at RenewableUK’s Global Offshore Wind awards, as well as shortlisted for the Technology and Business Innovation Award at 2022’s Scottish Renewables’ Net Zero Transition Awards.
Next phase of development
With the offshore charging system scheduled to be ready for deployment in the 1Q23, MJR Power and Automation has been further awarded a second round of UK government funding for development of its offshore charging system technology, to allow the system to further connect and charge service operation vessels and platform supply vessels working in the field.
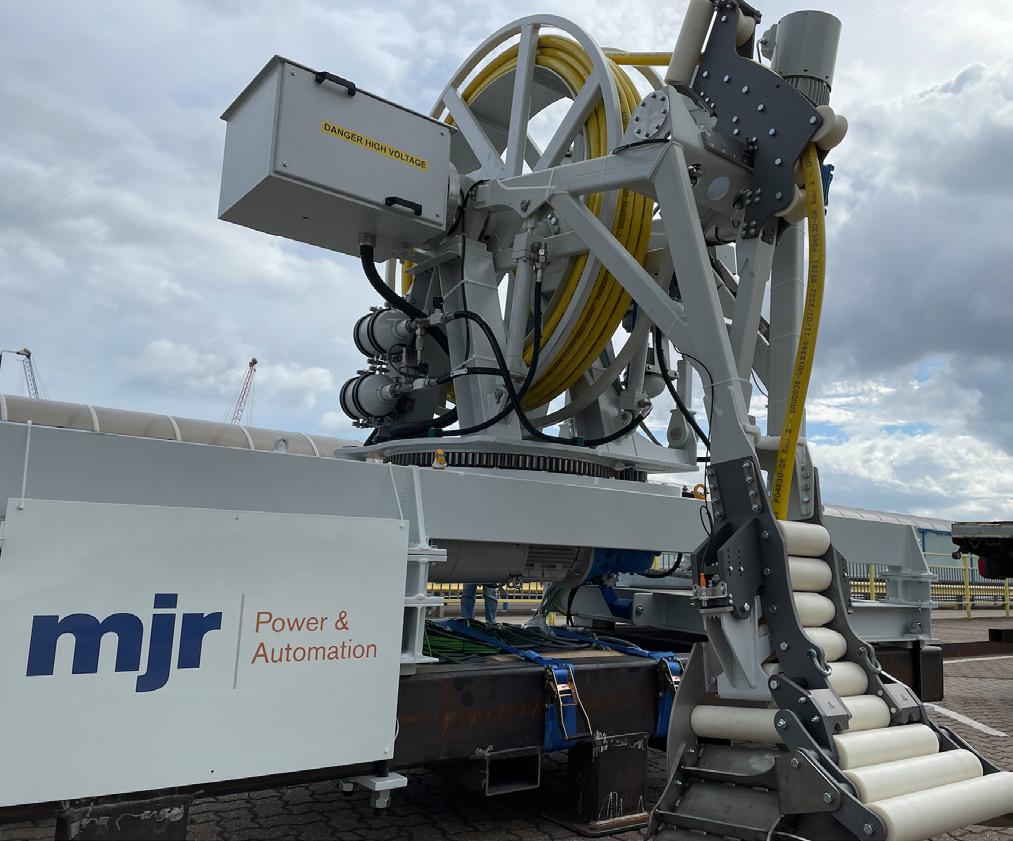
The Service Operational Vessel Offshore Charging System project is part of the Clean Maritime Demonstration Competition Round 2 funded by the DfT and delivered in partnership with Innovate UK.
This second round of funding from the DfT will be absolutely pivotal in supporting the decarbonisation of marine operations. MJR Power and Automation will work with their key partners, who are all experts in their fields, and take the lessons learned from the first project, applying extensive knowledge to develop this solution and bring it to market, where there is already a huge demand for it.
Figure 4 . On turbine mooring – charging cable and connector. Figure 5 Vessel connection and mooring system.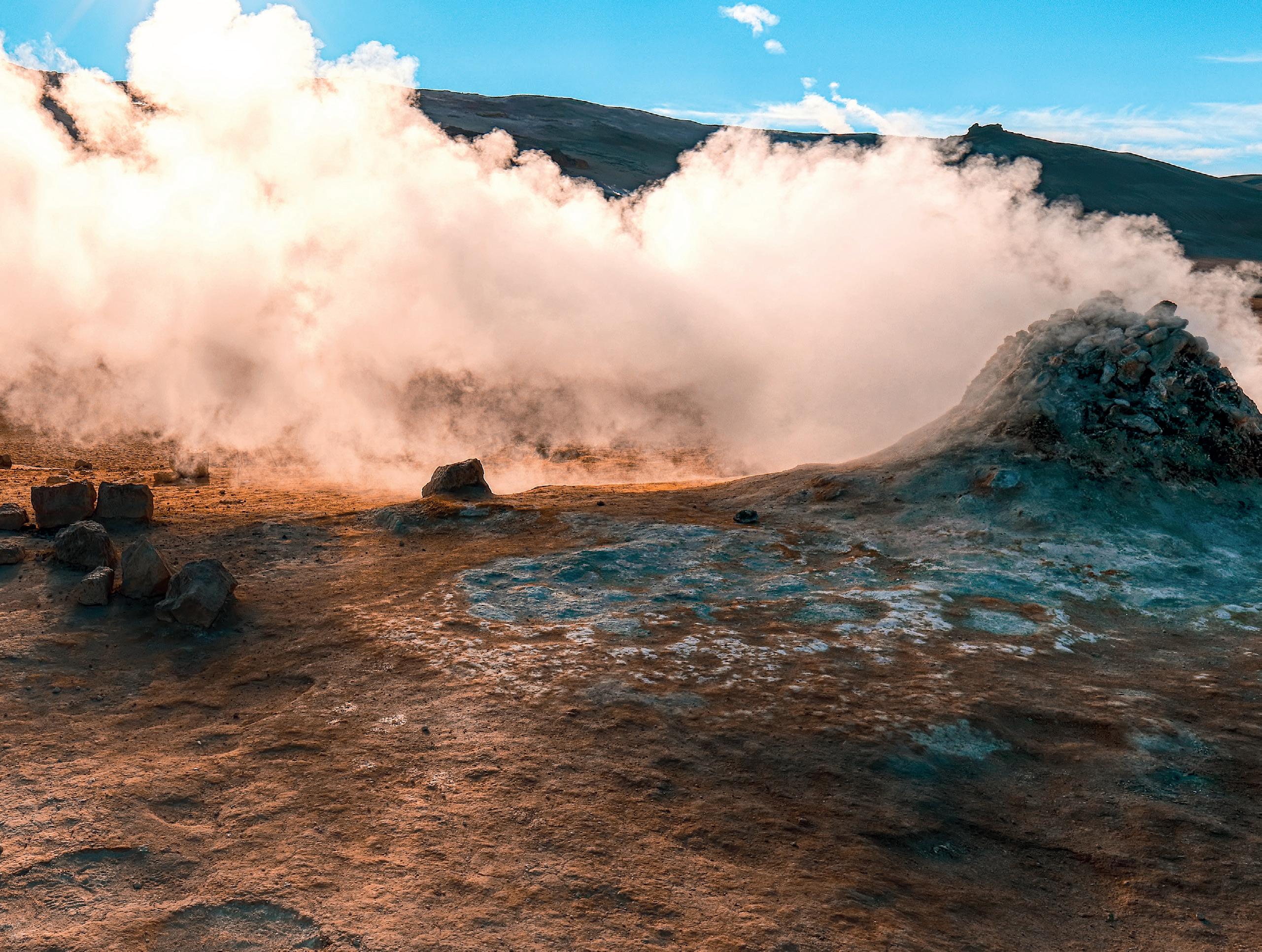
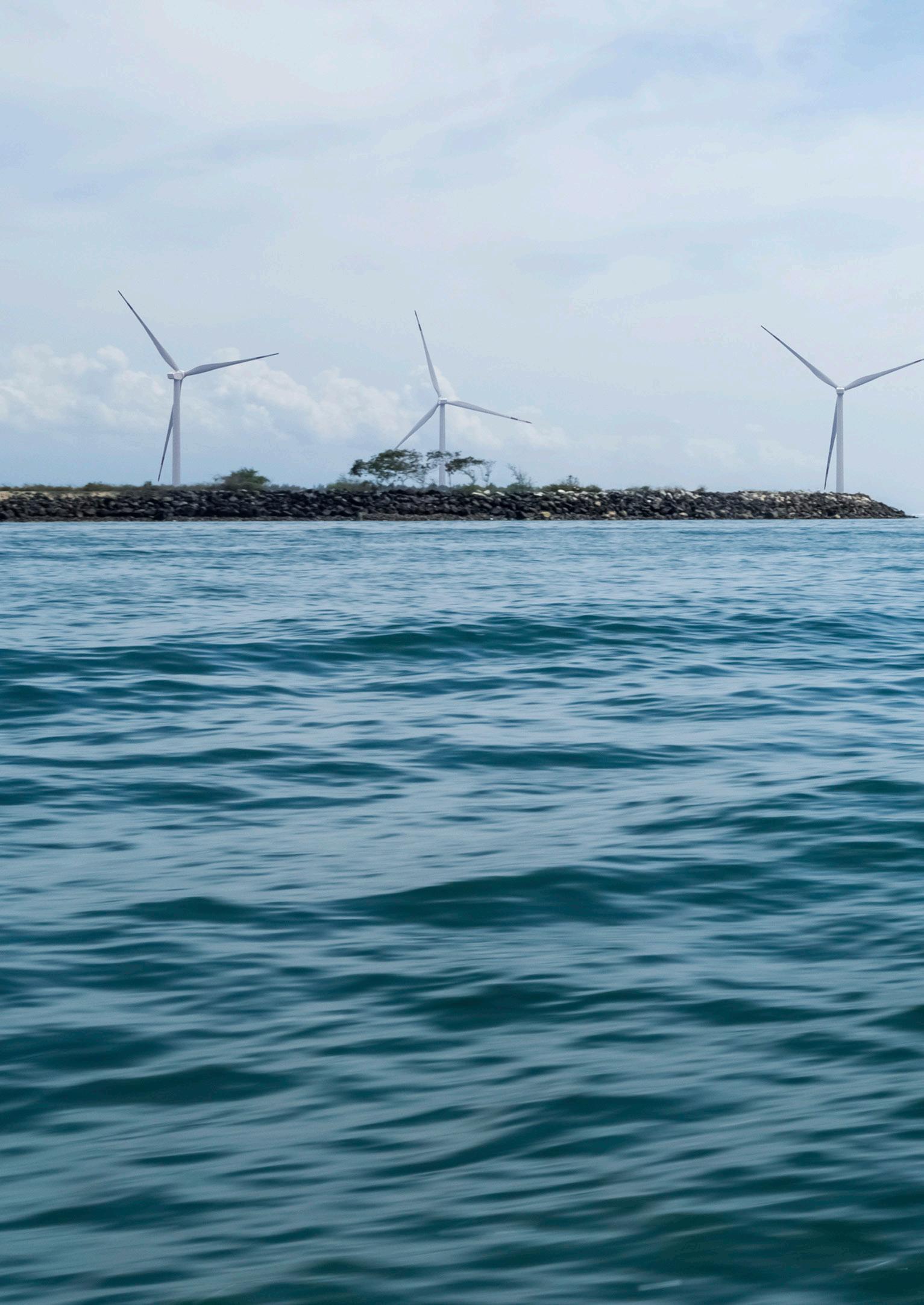
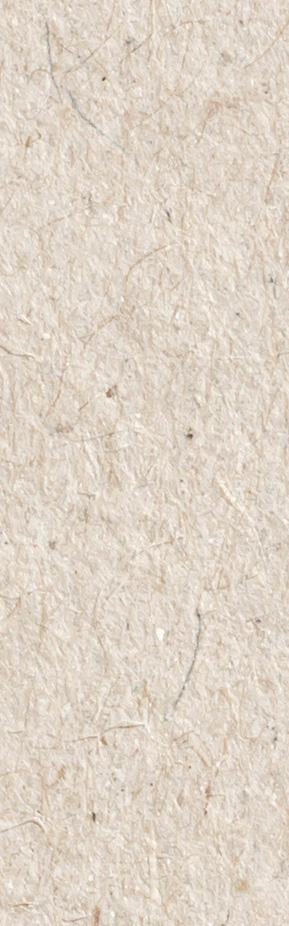
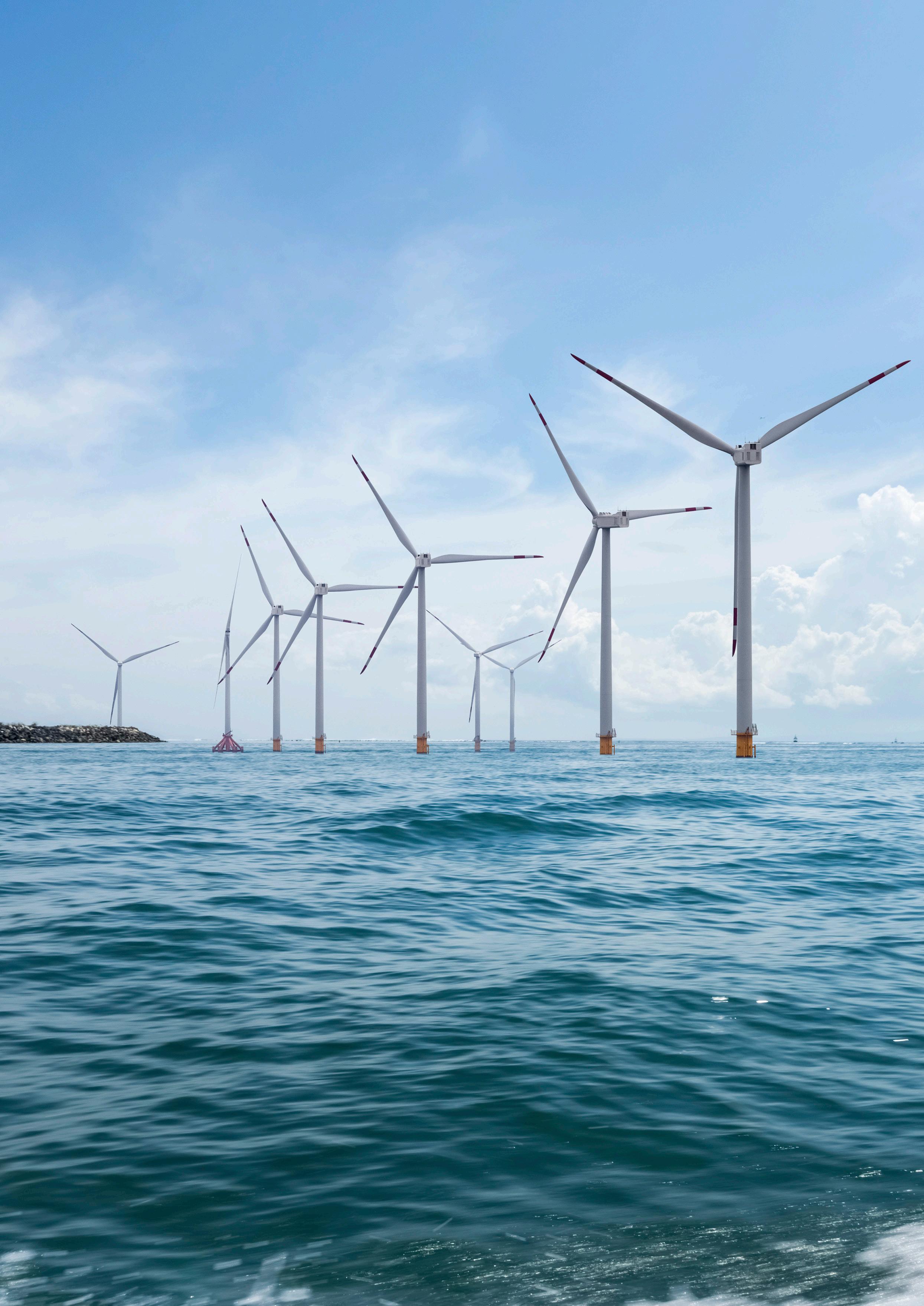
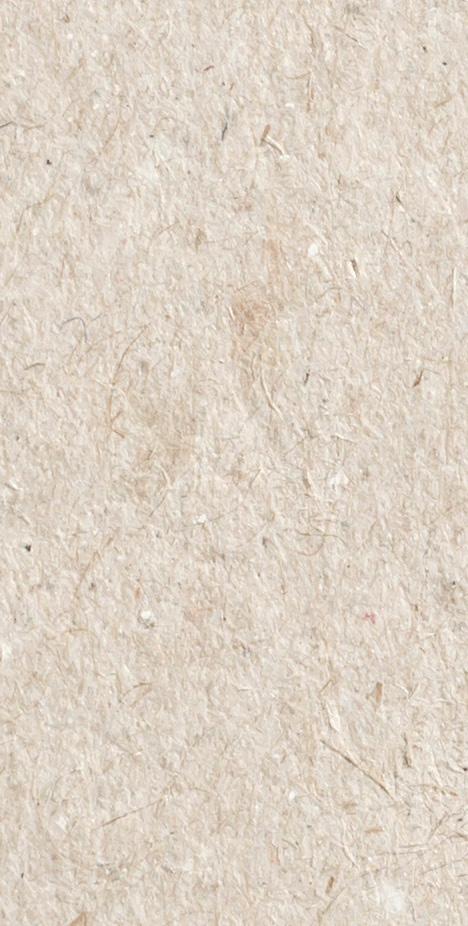
Since the Paris Agreement, global governments and leaders have outlined, defined, and refined their net zero aspirations, targets, and, more recently, frameworks. As a result, the last two years have seen a significant increase in the number of global seabed leases being awarded for new offshore wind farms or extensions to existing offshore wind farms. Due to events in Ukraine, many countries are now accelerating their plans to move away from dependency on other countries to supply their energy needs; notably both the UK and the US have announced commitments to accelerate the installation of offshore wind farms in their respective countries.
New leases have encouraged new offshore wind developers to move into the market. This, alongside the accelerated commitments, means that the global offshore wind market is looking to those with experience to help navigate these challenging demands. The world is looking at the existing UK and European supply chain to share its experiences and lessons learned; to mitigate repeated mistakes and to move the sector forward at the speed now required.
The recent GWEC report 1 references analysis undertaken by the International Renewable Energy Agency (IRENA) of the human resource requirements of the onshore 2 and offshore wind industry 3
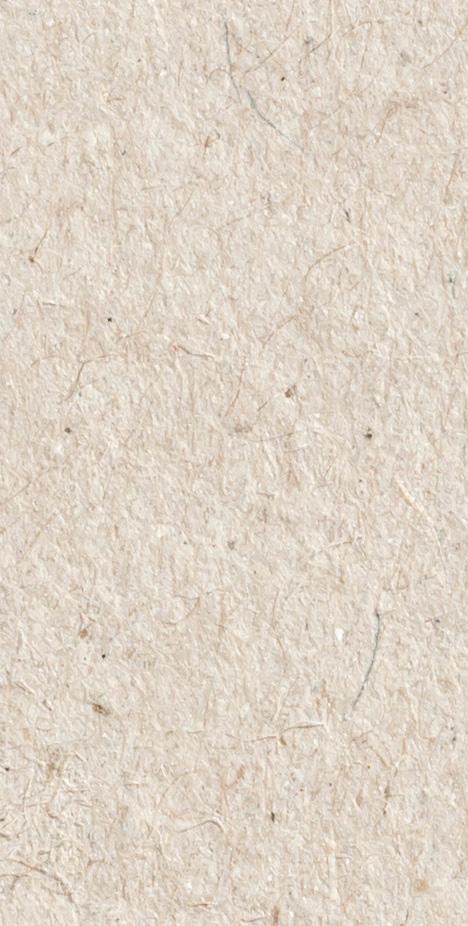
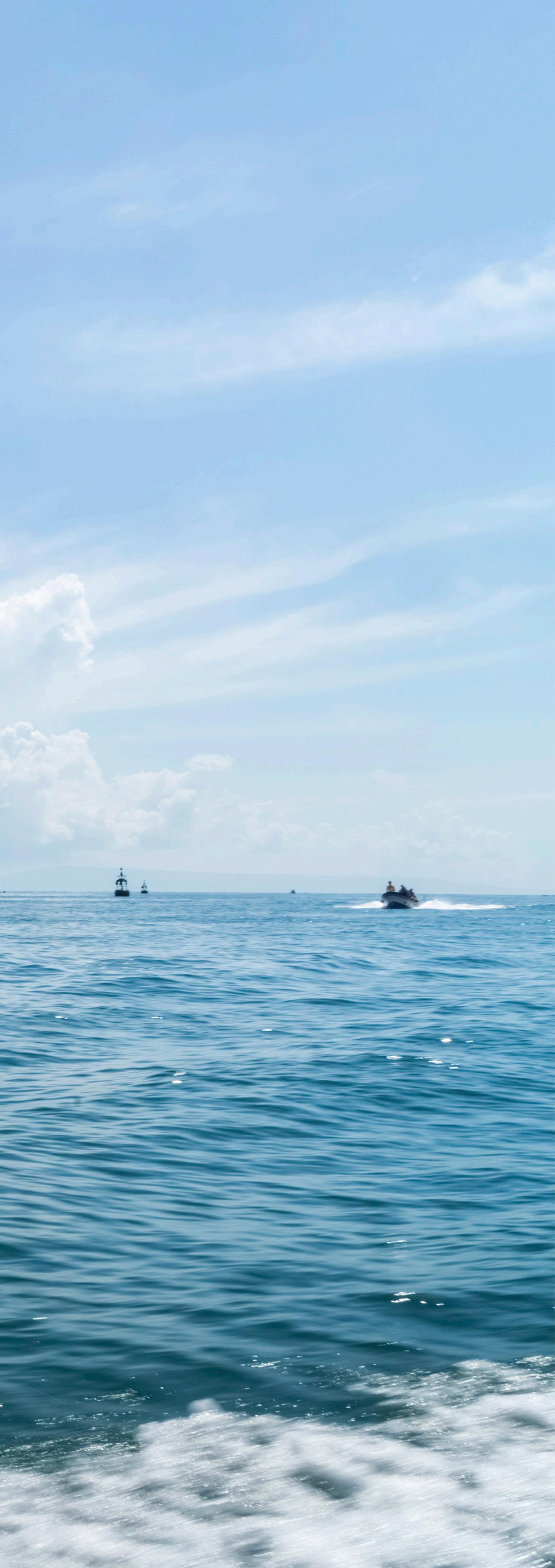
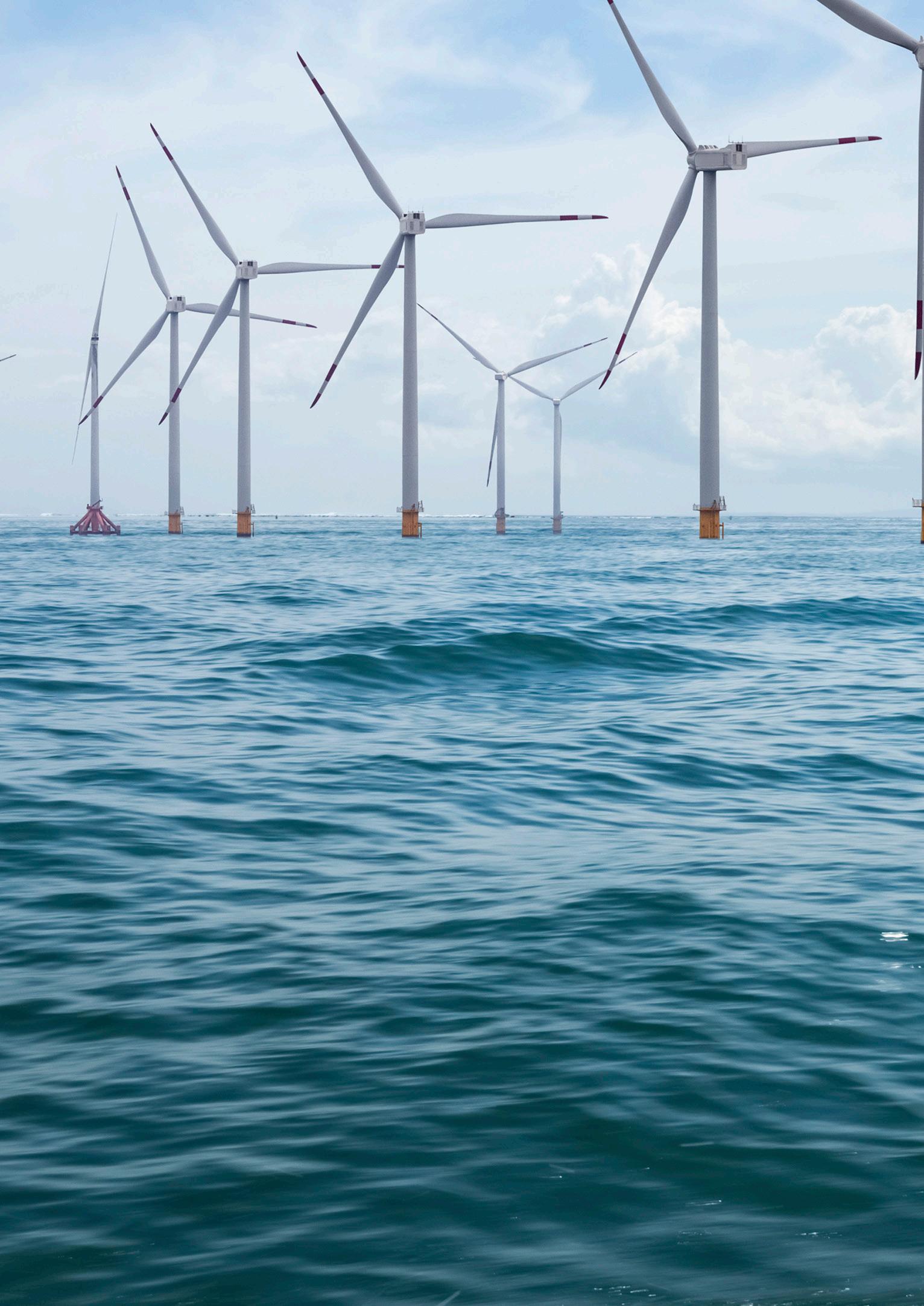
The analysis states that each offshore wind development requires 2.1 million person days of effort for a 500 MW fixed bottom to move from the development stage through to decommissioning. By comparison, only 144 000 person days of effort are required for a 50 MW onshore wind farm. The environmental differences between onshore and offshore developments are clear. As a result, offshore wind developments rely on the harmonious efforts across an extensive number of independent organisations, all resulting in points of interface; developers, designers, original equipment manufacturers (OEMs), certifiers, marine warranty surveyors, fabricators, installers, and operations and maintenance teams, to name but a few.
The last 20 years of offshore wind in the UK and Europe have demonstrated that the overall success of a project is determined by how well aligned and managed project interfaces are. There are a significant number of specialist companies and organisations that need to align for each project to be planned, designed, fabricated, installed, and operated. There is no time in the history of offshore wind that this challenge has been more important to overcome than now.
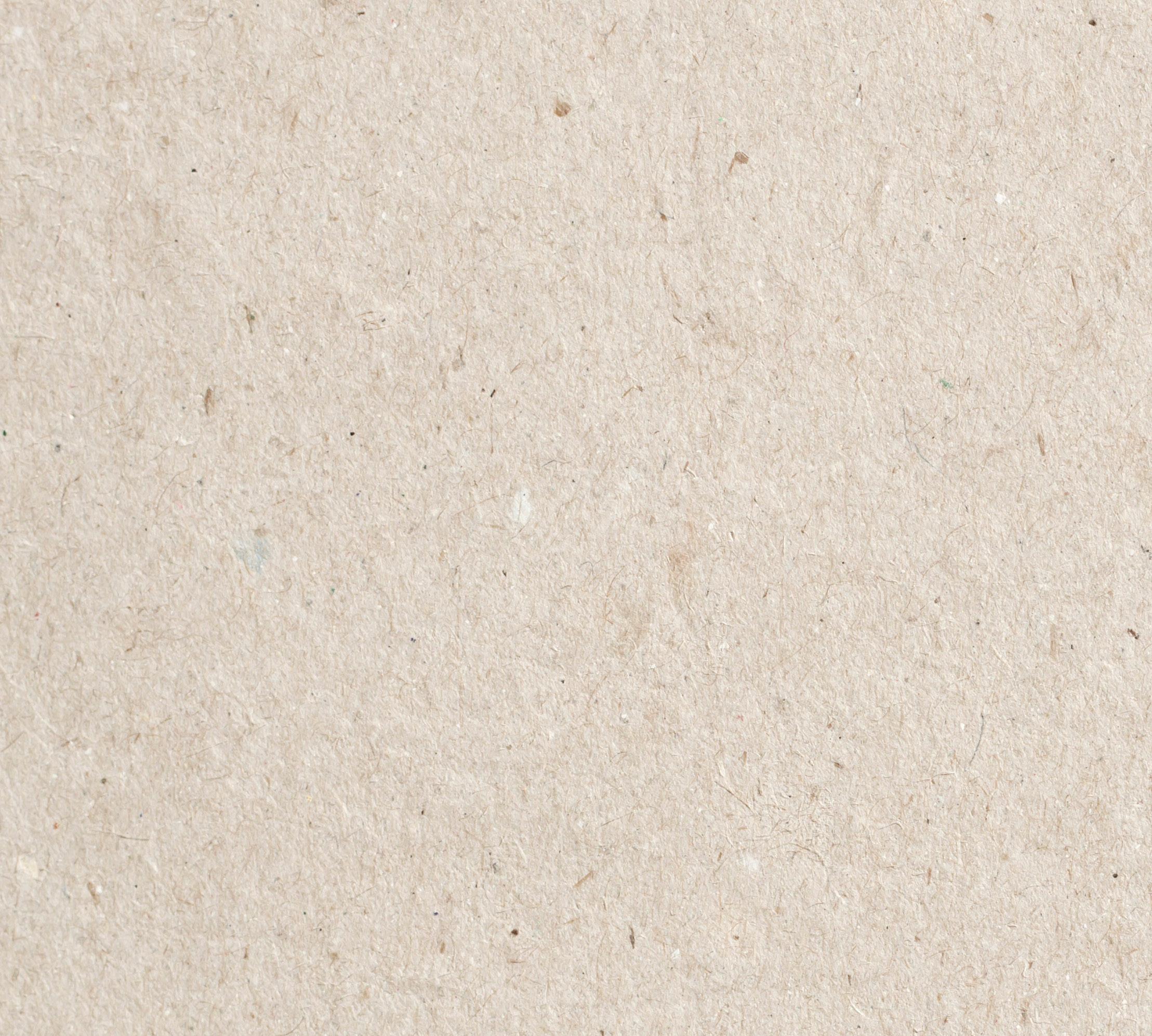
The increase in demand is putting significant pressure on all parts of the existing supply chain, whether that is in the shape of available installation vessels, suitable fabrication facilities, availability of component parts or material, capacity and suitability of ports and harbours, or suitably qualified and trained people. It is no surprise that the market is heading towards colossal supply and demand challenges and the additional costs that demand
will bring. Perhaps the reducing cost base seen in offshore wind is about to change?
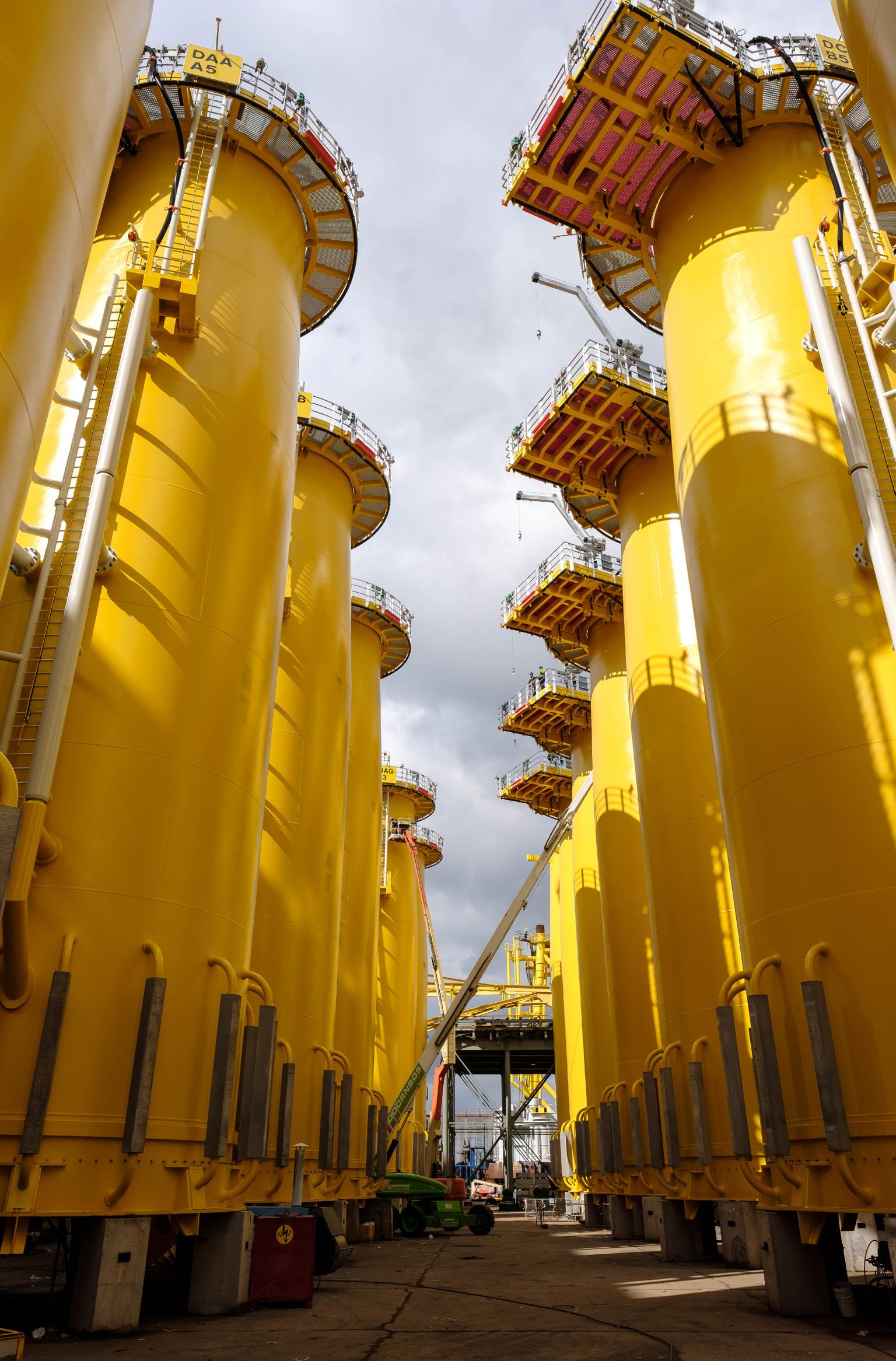
In addition to mobilising the experience developed and gained in the last 20 years in the fixed bottom offshore wind industry, the global markets are in parallel pushing forward with the more novel floating offshore wind. Whilst floating wind is seeing similar challenges to fixed bottom, there are a few differences; of note, different manufacturing, assembly, and wind turbine generator (WTG) integration requirements. In combination with the high levels of uncertainty on hull size, shape, and WTG size, how does the supply chain know where to invest? There is some good work being led by ORE Catapult Floating Offshore Wind Centre of Excellence to begin to address this, but the reality is the floating offshore wind journey is still at the beginning.
So how can the offshore wind market share the lessons of experience, manage, and provide the required resources and meet the aspirations and targets as set out to be net zero?
Early supply chain engagement
Most projects recognise the need for early supply chain engagement and alignment. Identifying and agreeing project requirements at the earliest opportunity saves time and money, reducing the number of iterations and permeations. The key is facilitating, co-ordinating, and contracting parts of the supply chain to come together at the optimal time in the developments programme.
In recent months, as a direct result of the known limitations in vessel availability and fabrication slots, and to mitigate potential programme risks, many developers are coming to market before renewable energy auctions have completed. This approach has had some limited success. Is this engagement too early?
With a need to prioritise enquiries, the supply chain is naturally focusing its efforts on those enquiries that will have the most chance of leading to contract awards. All enquiries require resources to be deployed at the supply chains costs, and, whilst business models recognise the need for the investment at the enquiry stage, the reality is that parts of the supply chain no longer have the resource availability to respond to the number of enquiries that are being released into the market place.
Partnering
One solution that appears to be addressing some of these challenges is partnering.
Those following the offshore wind media channels will likely have noticed that frequent new memorandum of understandings, partnerships, investments, or framework agreements are being announced. Examples include:
F SSE’s financial backing of the Port of Nigg factory, Scotland.
F Ørsted’s commitment to EEW to develop a new monopile production facility in New Jersey, the US.
F Implenia and WindWorks Jelsa agreement to construct floating concrete platforms.
These commitments have been required to manage and share risk and provide confidence to the supply chain.
Appropriate risk allocation
All projects, developers, and businesses consider risk and where risk should be allocated. It is taught that risk should be allocated to those most able to mitigate and manage risk. To date, it is easy to argue that this has not always been the case in offshore wind. Each year, some company annual financial reports record challenges that businesses have seen in the offshore wind market and the impact those difficulties have had on business performance. People hear about claims that have been made resulting in court cases when they could not be settled. With the reduction in supply and the increase demand in the market, now more than ever, businesses are assessing the ‘attractiveness’ of new contracts based upon the contracts risk allocation. The supply chain is no longer willing to agree to contracting terms that push all of the risk into the market place. Now is a good time to review, assess, and refresh contracting terms to ensure appropriate risk allocation and the fair and reasonableness of the contracting terms. Fair and reasonable contracting mechanisms will facilitate true collaborative working.
True collaborative working
True collaboration requires those collaborating to be committed to maximising joint performance in order to achieve the same objectives; it takes significant investments of time and effort but the potential rewards from collaborative working are numerous. Collaboration enables improved delivery of integrated services,
often with efficiencies and savings, allowing the sharing of knowledge and information. It is worth noting that separate organisations can maintain their independence and collaborate.
Collaboration in the offshore wind market would allow organisations with resources and expertise to offer assistance to others with lesser experience. Interfaces aligned to meet the same objectives will share and discuss challenges and align on how to overcome the difficulties to the benefit of the project as a whole; in the meantime, educating and informing each other of different perspectives. Collaborative teams become agile and bond over shared goals. This is likely to lead to comradery, increasing productivity, and perhaps, more importantly, improving mental health in the world of work. Ultimately this could also lead to accelerated project delivery and improved resource retention.
It is therefore no surprise during this year that there have been announcements encouraging and supporting collaborative working; the Scottish Offshore Wind Energy Council’s Collaborative Framework Charter is a demonstration of the commitment of ScotWind projects to work collaboratively, and the Global Offshore Wind Alliance from IRENA, GWEC, and the Danish government, which is aimed at becoming the go-to enabler for unlocking resources. Whilst these commitments are being outlined and endorsed at the highest levels of the offshore wind supply chain, now is a good time to think collaboratively top to bottom, end to end.
Conclusion
In summary, if the global net zero and government targets are to be met, and the Paris Agreement satisfied, an environment needs to be cultivated that allows the offshore wind supply chain to openly share the lessons of experience, manage, and provide the required resources to ultimately thrive and grow.
This will only happen if the sector and projects foster a more transparent working environment.
Consideration needs to be given to: right time supplier engagement, partnering to provide investment confidence, appropriate risk allocation resulting in fair and reasonable contracting terms, all of which will result in shared end goals and encourage true collaborative working.
Collaborative working brings many benefits: shared learning, increased productivity, and accelerated project delivery. Many in the sector are realising that to meet the worlds ever growing energy needs, collaboration will be fundamental.
References:
1. ‘Global Wind Report 2022’, Global Wind Energy Council https://gwec.net/global-wind-report-2022/
2. ‘Renewable Energy Benefits: Leveraging local capacity for onshore wind’, International Renewable Energy Agency (IRENA), (2017), www.irena.org/publications/2017/Jun/Renewable-Energy-Benefits-LeveragingLocal-Capacity-for-Onshore-Wind
3. ‘Renewable Energy Benefits: Leveraging local capacity for offshore wind’, IRENA, (2018), www.irena.org/publications/2018/May/Leveraging-Local-Capacity-forOffshore-Wind
R e s h a p i n g K natshkaza s’ grene y sector
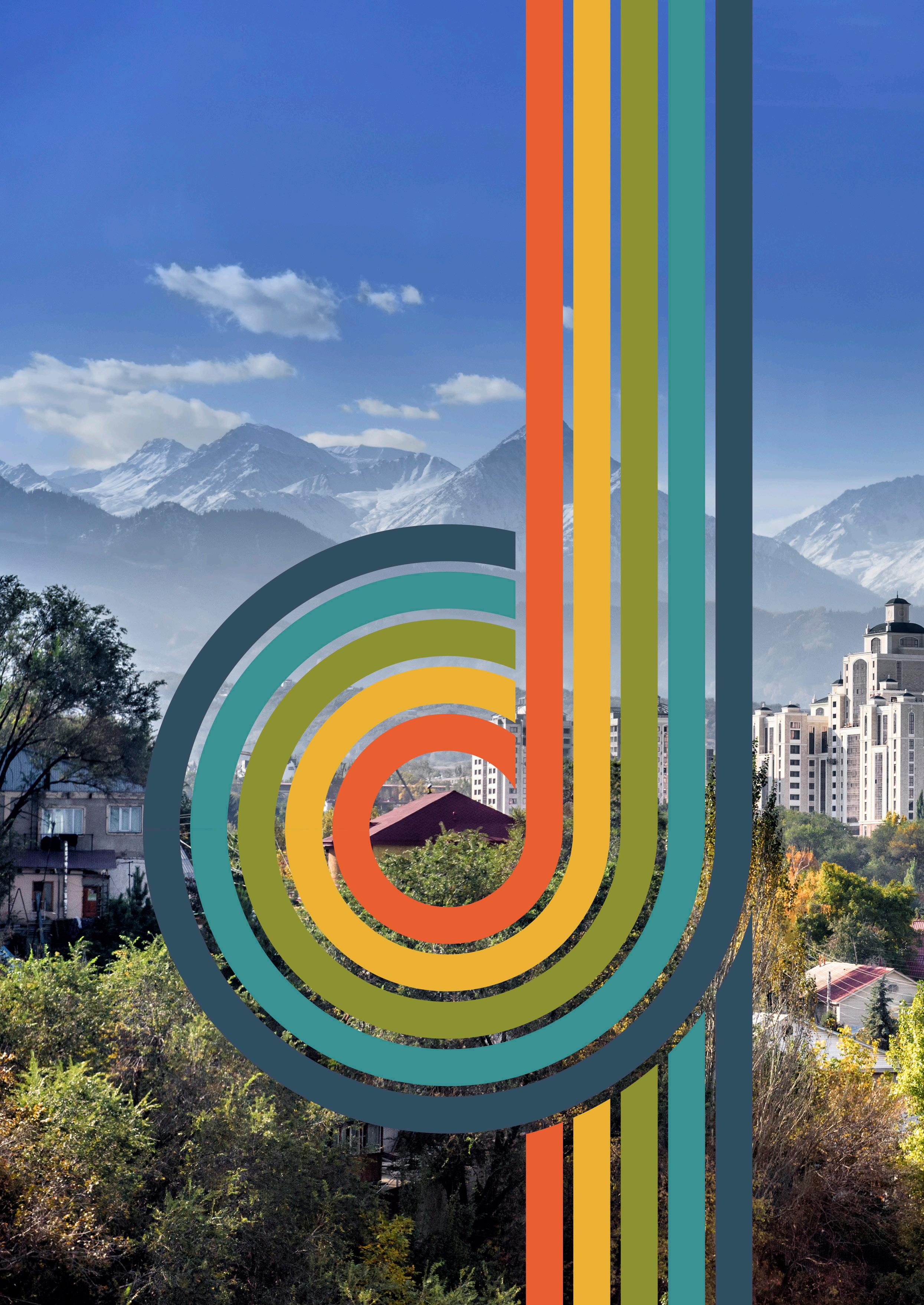
n 2020, during the UN’s Climate Summit, President Kassym-Jomart Tokayev announced Kazakhstan’s ambitious plan to reach carbon neutrality by 2060. While governments around the world have been making similar pledges in recent years, this target is particularly notable for a country with significant fossil fuel reserves. Following Tokayev’s announcement, Kazakhstan’s share of renewable energy is set to increase more than twenty-fold from approximately 3% currently to 70% by 2060.1 In other words, there is an expectation of a considerable, tectonic shift in the composition of Kazakhstan’s energy sector in the coming decades.
Kazakhstan’s carbon neutrality strategy
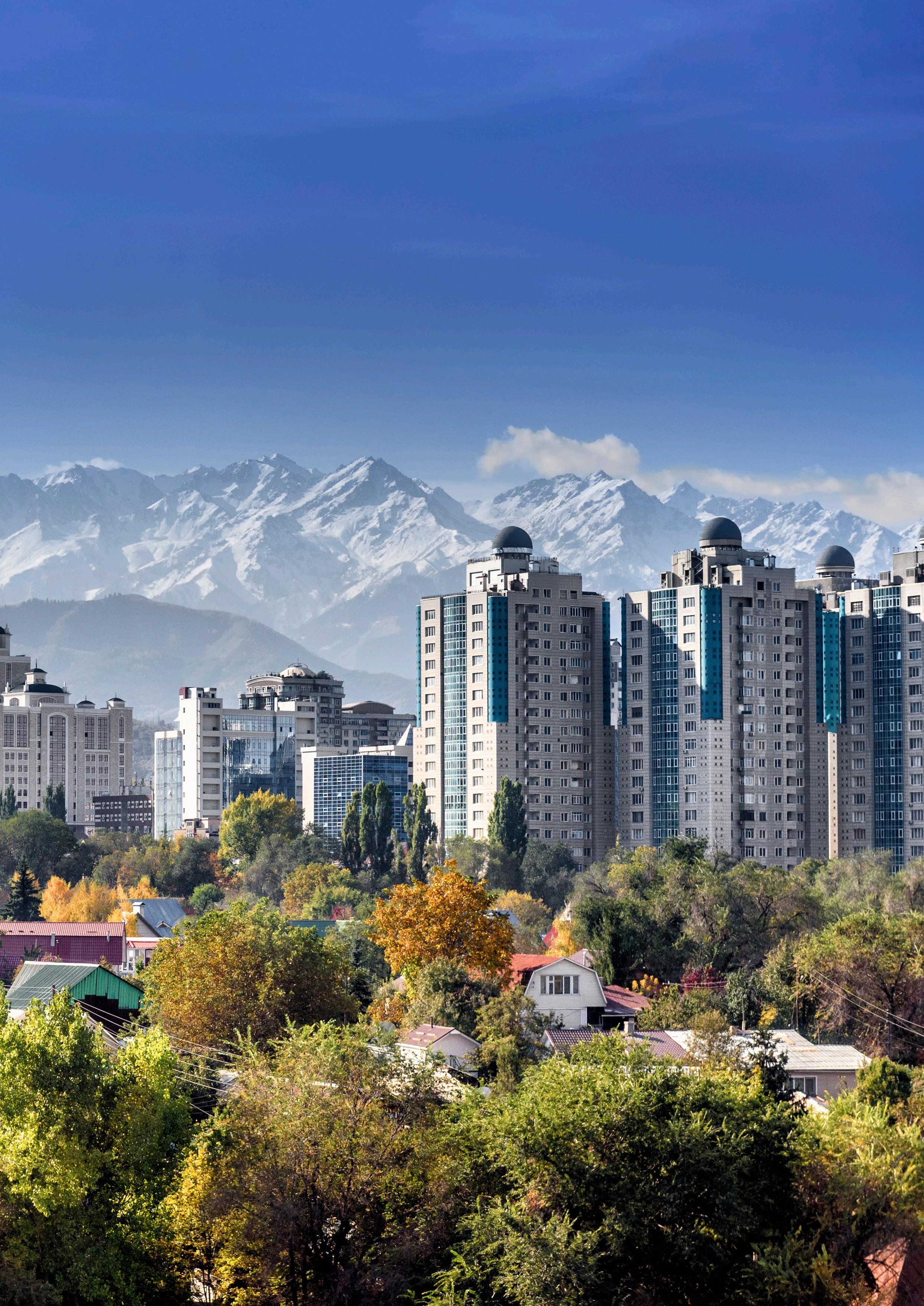
To achieve this, governmental bodies and ministries, in collaboration with international experts and organisations, have been developing a long-term strategy for achieving carbon neutrality until 2060 – a framework with a clear pathway for achieving decarbonisation, the energy sector transition, and the
development of clean technologies. Among the key measures outlined in the strategy is a doubling of the share of renewable energy sources in electricity generation; 100% electrification of personal passenger transport; and the use of green hydrogen.
Put simply, the realisation of the strategy will bolster market-focused and regulatory policies for renewable energy, as well as attracting substantial public and private investments to support these objectives. President Tokayev reiterated the importance of mobilising investments during a meeting of the Foreign Investor’s Council in June 2022,2 and announced that the draft strategy was close to being finalised during the recent Climate Dialogue Conference.3
Exploring renewable energy sources
The need to transition to net zero is clear. However, a key question to address is: Which energy sources should the country prioritise switching to? As highlighted by the team of international researchers from Rystad Energy, Kazakhstan has been focusing
on wind and solar power, as well as looking into the potential of green hydrogen.4 For example, the Svevind group of companies signed a roadmap with Kazakhstan’s government last year for 30 GW in green hydrogen developments by utilising wind and solar power near the Caspian Sea.
In September 2022, the UNDP and the government of the Republic of Kazakhstan published a joint study funded by the Global Environment Facility,5 which analyses the country’s renewable resources potential and the opportunities for renewable energy technologies in heating, cooling, and hot water supplies in various geographical locations. The study provides, among other things, an informative overview of the state’s policies in the sphere of renewables and other sources, including solar, wind, hydro, geothermal, and biomass energy. Despite the great variety of energy sources available to Kazakhstan, wind power still remains high on the agenda, partly due to its economic feasibility.
The US Agency for International Development also explored the potential of various renewable energy sources in Kazakhstan in some depth in a 2020 report.6 The report claimed that whilst hydro, geothermal, and solar energy each had their advantages, wind energy has “the greatest potential among all [renewable energy sources] in Kazakhstan.” This is because approximately half of Kazakhstan’s territory reportedly has promising conditions for producing wind energy. According to the aforementioned 2022 study, for a wind zone with a speed range of between 3 – 9 m/sec., the power potential is approximately 7466 GW.
Potential of wind power in Aktobe
According to the Kazakh Ministry of Energy, wind farms are now responsible for generating 45% of the total renewable energy in Kazakhstan.7 The 2020 and 2022 reports suggest that the greatest potential for wind power is in northern and southern Kazakhstan, as well as the Atyrau and Mangystau regions in the west. But what about the neighbouring western region of Aktobe, a key industrial area and the second-largest region in the country? After all, Kazakhstan’s shift towards a fully sustainable energy landscape is contingent on renewables being advanced and accessible across the entire country.
At first glance, Aktobe may not appear to be the first choice for developing a wind farm. According to existing research,5 it does not have regular wind speeds of above 7 m/sec. As mentioned in a research paper on wind power generation in Kazakhstan, “the winds around Aktobe City are strong, but inconstant, with […] seasonal fluctuations and wind swings.” This vast area is dominated by low and medium wind speeds, according to a study by PB Power and Windlab Systems.5
Whilst these are valid concerns, it is important to also consider the latest advances in wind turbine technology, which enable wind power to be produced in more wide-ranging weather conditions. Eurasian Resources Group (ERG) – a diversified natural resources producer representing one-third of Kazakhstan’s metals and mining industry – can attest to this. ERG recently announced plans to invest approximately US$230 million in constructing a large scale wind energy facility in Aktobe, which will have a capacity of up to 155 MW, making it the most powerful plant in the region. Named Khromtau-1, the wind power plant will extend over 150 ha., with potential to double generation capacity of the site in the future. Construction of the plant is planned to start in 2022.
With regards to the weather conditions in Aktobe, ERG’s 2021 feasibility study confirmed the viability of installing the planned capacity at the Khromtau site, located just east of Aktobe City. The necessary power output can be generated using appropriate engineering and technology to overcome the extreme continental climate: The wind farm will be built with powerful IEC S-class wind turbines, which have been specially designed to site-specific conditions and wind regimes. The turbines will have been made to consider the particular wind field parameters of the region and will generate electricity in a range of weather conditions, with wind speeds from 3 – 25 m/sec.
In doing so, the wind power plant is expected to reduce carbon dioxide levels by approximately 520 000 tpy. It will supply energy to ERG’s Donskoy GOK extracting and processing plant, the largest industrial enterprise in the Aktobe region, and cover its growing energy needs without relying on the region’s energy system. Khromtau-1 will also supply energy to neighbouring industrial facilities and the Aktobe region more widely, thereby reducing Kazakhstan’s current usage of fossil fuels. After launching this wind park in Aktobe, ERG plans to implement its know-how and experience in green energy at its other sites of operations in Kazakhstan.
Regulatory environment
It is worth noting that from 2026, Kazakhstan’s producers will have to pay a carbon border tax when exporting into EU countries,2 which may affect the competitiveness of some Kazakh exporters. That is to say, the Khromtau-1 project demonstrates how technologies used in the renewable energy sector can minimise the industry’s carbon footprint, provide energy security for the region, and increase the competitiveness of the country’s products in the international arena.
Overall, Kazakhstan has a favourable regulatory framework for renewable energy projects, which significantly enhances opportunities for development in this area. For instance, government regulation allows for the annual indexation of prices in the power purchase agreements (PPAs) signed by investors, which takes into account inflation as well as the fluctuations in KZT/USD exchange rate, providing cover against exchange risks.
Additionally, these PPAs for renewable energy projects are signed for 20 years at the tariff proposed during the auction, resulting in a very predictable rate of return for companies investing in renewables. Moreover, the regulatory environment enables contracts to be signed directly with major industrial or commercial consumers committed to purchasing green energy. All of this results in a healthy market to attract significant investment and innovation, leading to the optimisation of all cost components and maximising the opportunities created by the clean energy transition.
So far, ERG has identified other potential sites across Kazakhstan for large scale wind and solar power generation projects. These projects will be executed in the next 5 – 10 years, with additional research and assessments taking place in 2022.
Technologies and emissions reductions
To achieve carbon neutrality and make the country’s exports ‘greener’, businesses in Kazakhstan clearly need to focus on emissions reductions as well as absorbing and capturing technologies. One example of applying progressive
technological solutions to reduce emissions and energy consumption from conventional sources is using off-gases produced by refining and manufacturing. For example, major energy producer, POSCO Energy, has been successfully implementing such an approach in South Korea for years. By using off-gases from its steelmaking process, its Pohang Off-Gas Power Plant reduces greenhouse-effect gases by 180 000 tpy and provides a viable and cheaper substitute for oil.
Could this technology provide an energy solution for Kazakhstan? After an exhaustive feasibility study, ERG plans to furnish its Aktobe Ferroalloys Plant with an off-gas fuelled power station with a capacity of up to 100 MW. This will generate energy using off-gas from its ferroalloy production process, thereby reducing its energy use from conventional sources. Undertaking substantial preparatory research is key for the successful realisation of projects such as this. A thorough data collection and viability analysis is being conducted for the potential conversion of the boiler house at Aksu Ferroalloys Plant, as well as the possible construction of a thermal power station, both of which will use the off-gases.
As well as introducing off-gas power facilities at existing operations, another energy solution for industry is incorporating such facilities into their premises at the level of planning. For instance, ERG is considering the development of an off-gas fuelled power unit at its new Special Coke Plant at Shubarkol Komir in Kazakhstan. The plant is scheduled to be put into operation in the coming years and involves collaboration between ERG and China Nonferrous Metal Industry’s
The scene is set for Kazakhstan to become an increasingly important player contributing to the net zero transition and development of renewable energy sources. The recent UNDP publication commended the country’s ambition to achieve carbon neutrality by 2060 and highlighted the need for further modernisation, diversification, and adaptation of ‘green’ technologies at its production facilities. Kazakhstan has a history of successfully attracting major international investors in its energy industry. With the help of national partners and international organisations, now is the time to attract similar investments and innovation to secure Kazakhstan’s low-carbon future.
References
1. ‘By 2060, Kazakhstan will achieve carbon neutrality,’ Official Information Source of the Prime Minister of the Republic of Kazakhstan, https://primeminister.kz/ru/news/ reviews/do-2060-goda-kazahstan-pereydet-na-uglerodnuyu-neytralnost-1103515, (27 October 2021).
2. ‘President Kassym-Jomart Tokayev takes part in the 34th plenary session of the Foreign Investors’ Council,’ Official website of the President of the Republic of Kazakhstan, https://akorda.kz/en/kassym-jomart-tokayev-takes-part-in-the-34thplenary-session-of-the-foreign-investors-council-1054121, (9 June 2022).
3. SHAYAKHMETOVA, Z., ‘Officials, Experts Discuss Kazakhstan’s Draft Carbon Neutrality Strategy’, The Astana Times, https://astanatimes.com/2022/10/officials-expertsdiscuss-kazakhstans-draft-carbon-neutrality-strategy/, (11 October 2022).
4. KHOI LE, M., YEE CHEW, J., YU, D., R, P., and ITTAN, R., ‘A splash of Asian flair,’ Energy Global, Autumn 2021, pp.6 – 11, https://issuu.com/palladianpublications/docs/ eg10fhuwl, (19 October 2021).
5. ‘Potential analysis of introducing various renewable energy technologies, including heating, cooling, and hot water supply in different geographical areas, taking into account the resource potential,’ UNDP and the government of the Republic of Kazakhstan, https://procurement-notices.undp.org/view_file.cfm?doc_id=260801
6. ‘Investor’s Guide to Renewable Energy Projects in Kazakhstan,’ USAID
7. ARYSTANBEK, A., ‘New Wind Farm in Aktobe Region to Expand Kazakhstan’s Wind Power Capacity,’ The Astana Times, https://astanatimes.com/2021/06/new-wind-
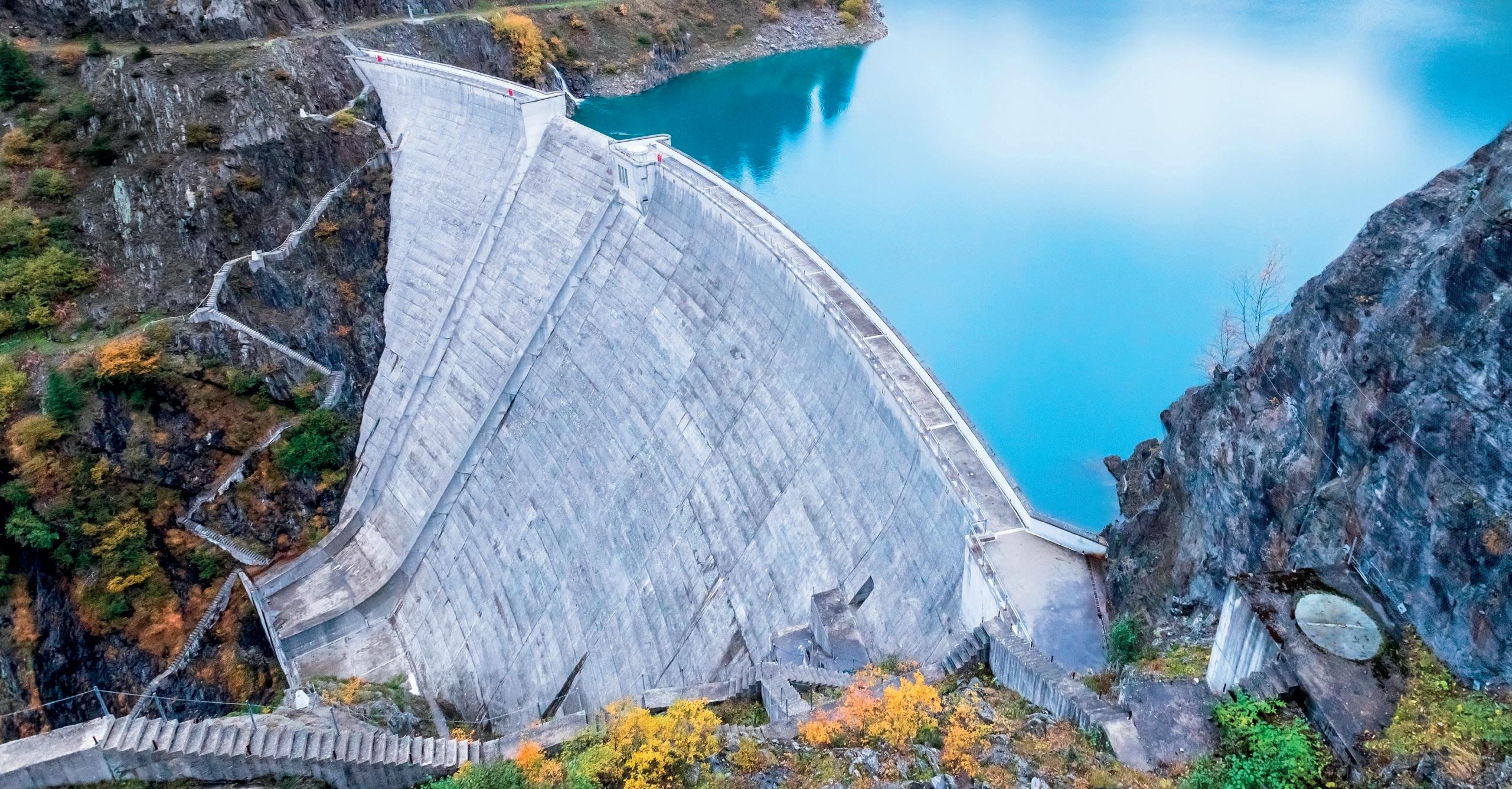
outlines the essential technologies needed to integrate solar into the power grid.
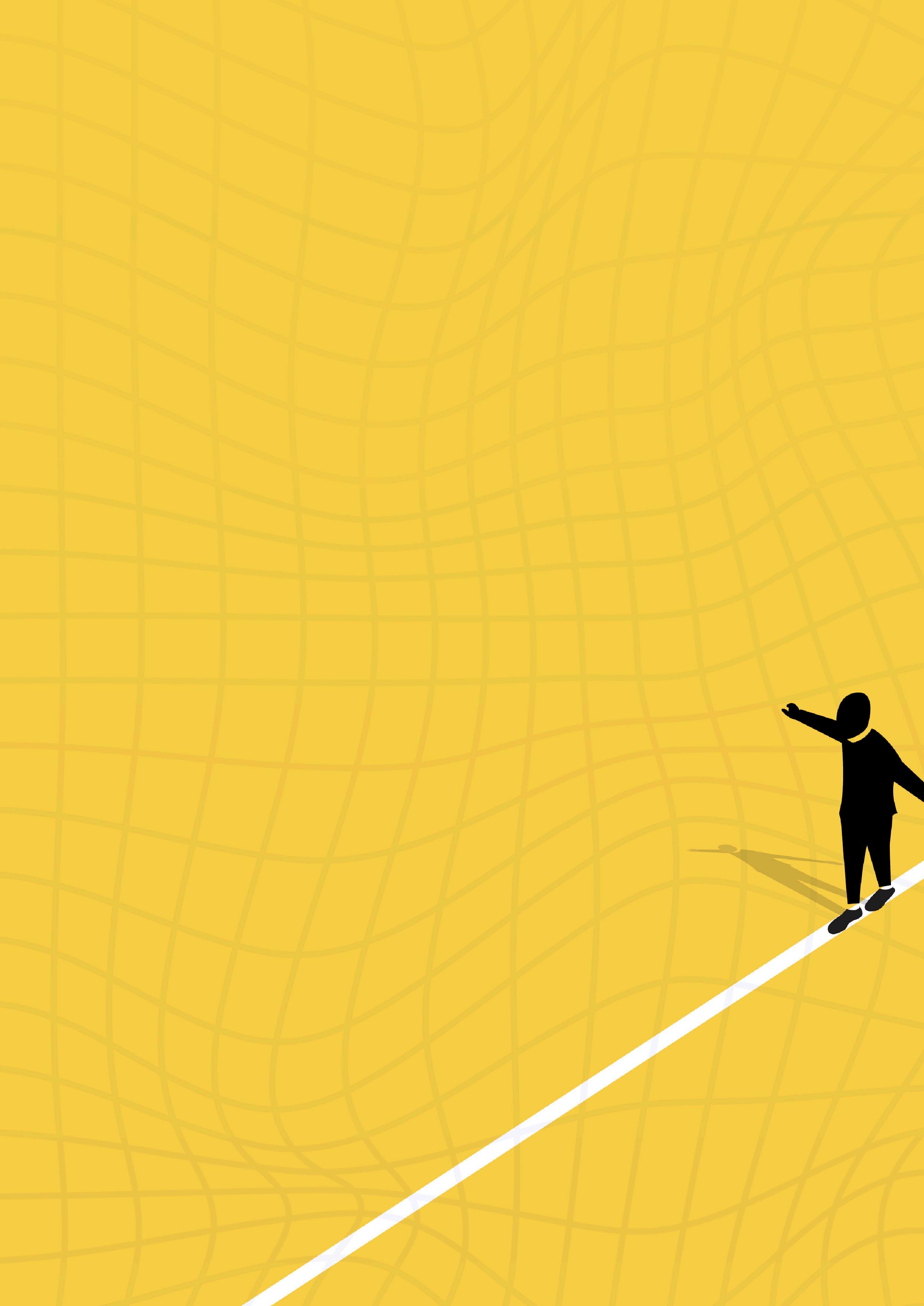
The transition to a more sustainable energy future is well on its way.
Global electricity demand is expected to more than double in the coming 30 years, making electricity the backbone of the entire energy system and accounting for more than half of total energy consumption by 2050.1
Renewables are central to that transition, representing more than 80% of new power generation capacity installed today.2 Of renewable energy sources, solar is the fastest growing, registering a 19% increase in capacity in 2021, equal to 133 GW.2 By 2050, solar is expected to account for approximately half of all electricity generation.
Power quality is key
With so much variable solar generation in the power system, the grid connections that transfer electricity from the solar energy plants to the grid need to include additional power quality technologies alongside the traditional combination of switchgear, transformers, as well as protection and control.
This is because of increased requirements for reliability and flexibility, as well as the need to provide services to the power system that were previously provided by fossil fuel-fired power plants that some countries are now shutting down. Previously, when renewables represented a small share of power generation, the quality of that power was not critical; now, renewable energy plants need to operate with the same power quality as traditional generators.
The technologies that are becoming more common in solar grid connections are static synchronous compensators (STATCOMs), synchronous condensers, battery energy storage solutions, advanced energy management systems, and modular prefabricated grid connections.
These technologies are already available. The benefits they provide are crucial for power system quality and reliability. This includes better control of electrical parameters; improved voltage dynamic behaviour, frequency, and active and reactive power; increased short-circuit levels at the connection point; adding more inertia to the power grid; and faster, simpler, and more efficient grid connection solutions thanks to modularity and prefabrication. In short, these technologies enable the power grid to become more resilient and operate in accordance with compliance requirements.
Keeping the grid stable
The first group of requirements is related to inertia and short-circuit power. The inertia of the power system is traditionally provided by large electrical generators. When traditional power plants close down, there are fewer synchronous rotating masses in the power system, which weakens system resilience. In a weaker power system, the frequency is less stable. This can be solved by adding synchronous condensers, in some cases with additional mass in the form of a flywheel. For example, in the UK, Hitachi Energy has pioneered the combination of a synchronous condenser with a STATCOM to improve inertia and short-circuit power.
This world-first installation at an SP Energy Networks’ grid connection in Scotland proves that a machine with a flywheel increases the inertia of the power system. If a fault occurs, the solution feeds it with short-circuit current. The result is a stronger network, which in combination with the fast voltage control of the STATCOM further improves the dynamic behaviour of the system.
Energy storage
The second group of requirements is linked with the active power of the solar plant and its response to demand from the grid. Solar plants have predictable but variable generation and do not inject power into the grid at night. By combining energy storage with advanced energy management systems, the variability can be controlled and power can be delivered optimally when the sun is not shining.
Examples of solar power with energy storage are now very common, such as the combined solar and energy storage solution for Skagerak Arena football stadium in Norway. Battery energy storage with advanced grid automation helps the system optimally deliver the solar power generated to both the stadium and the neighbourhood when solar generation is low.
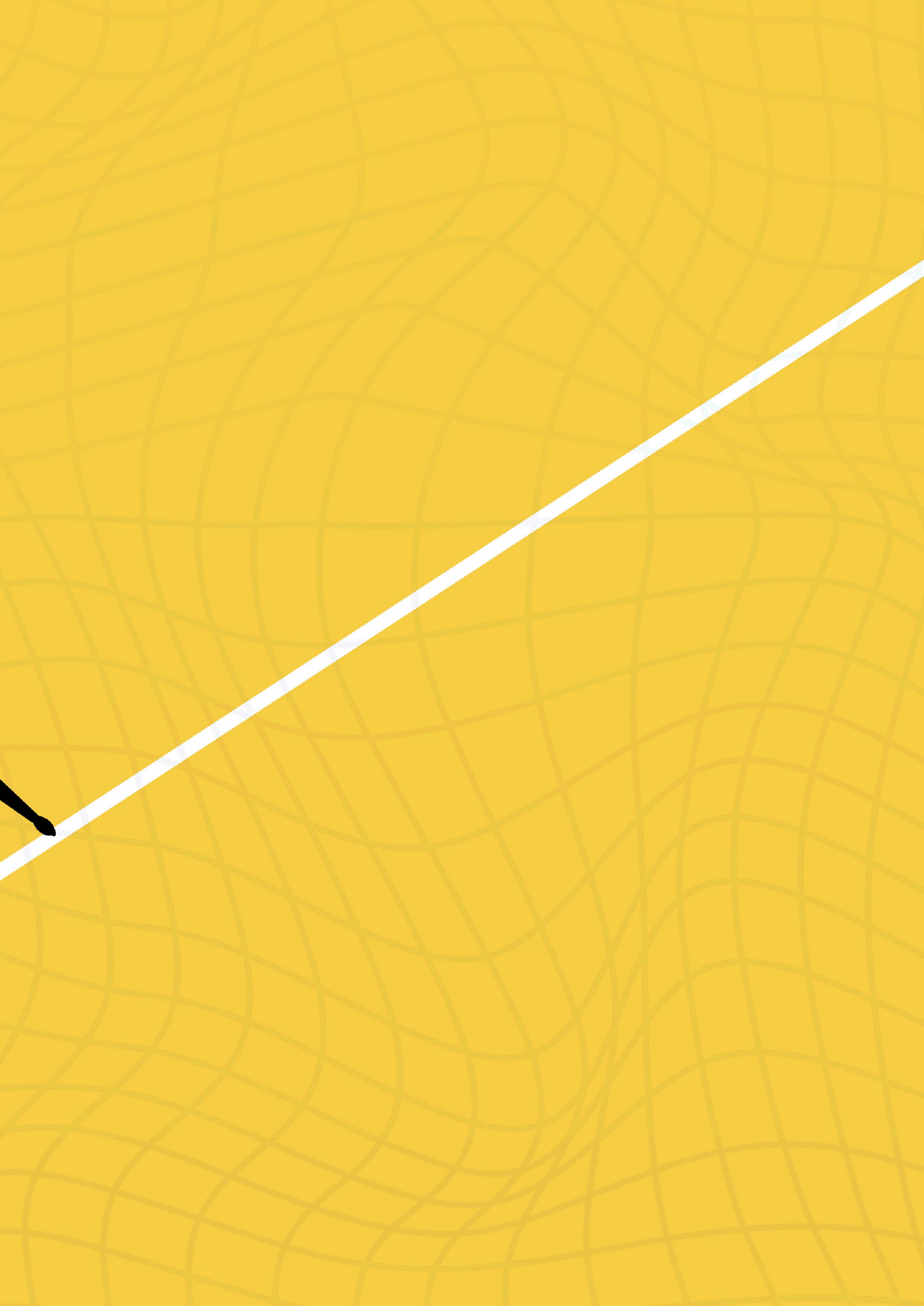
Additionally, it reduces peak demand on the network by automatically releasing the energy when needed.
Improved power dispatching is also achieved by larger solar plants, such as the Al Badiya 23 MW solar power plant in Jordan, which has 23 MW/12.6 MWh of battery energy storage. By using advanced power plant control, it is able to shift generation from noon to the evening, delivering the solar power when it is needed most.3
Simpler, faster grid expansion
The third set of requirements is making grid expansion simpler and faster to meet the influx of renewables and the rising demand for power in the new electricity-based energy system. Prefabricated and modular grid connections make this process easier and more efficient by shortening lead times, reducing the grid connection footprint, cutting the cost of construction, and speeding up energisation.
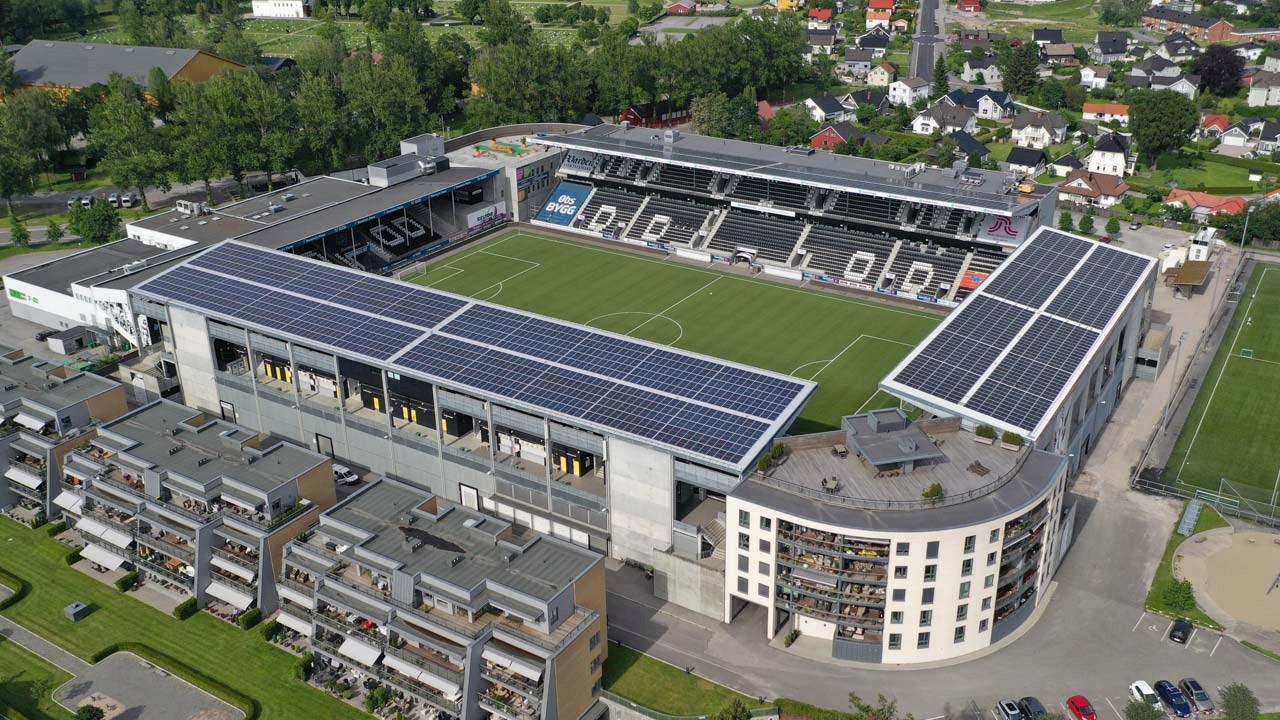
For instance, Hitachi Energy has recently released its Grid-eXpandTM portfolio of modular prefabricated grid connection solutions. One recent example is the 170 kV containerised gas-insulated switchgear (GIS) grid connection for a combined fish farm and 312 MW solar photovoltaic power plant in Southeast Asia. Owned by Sunny Rich Group, the site uses solar power and advanced technologies to farm fish sustainably. Compared to a conventional GIS grid connection, the solution has a 25% smaller footprint, was delivered and energised 15% faster, and reduced the cost of civil works by 50%.
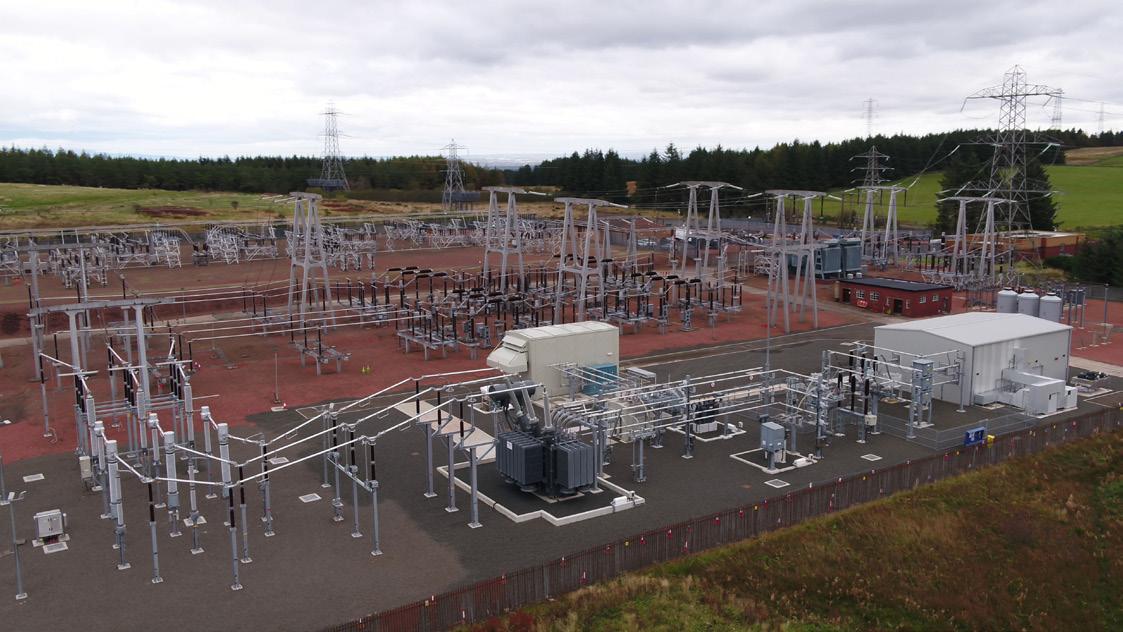
The environmental footprint of these grid connections can be further improved with high-voltage products that
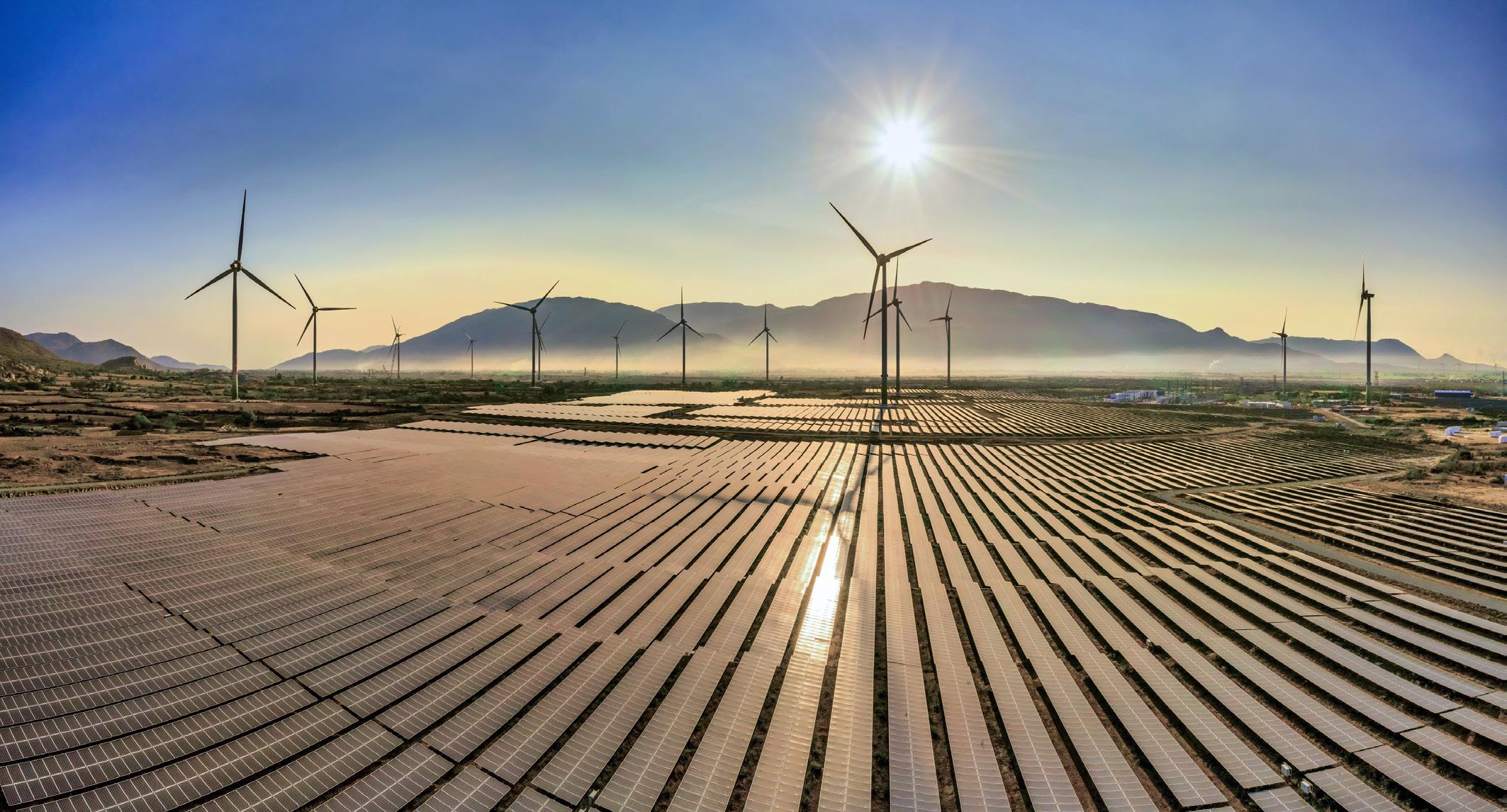
use an eco-efficient alternative to sulfur hexafluoride (SF6). For decades, SF6 has been the norm in the electrical industry due to its excellent insulation and switching capabilities. However, it is also a potent greenhouse gas. To address this challenge, Hitachi Energy pioneered EconiQTM high-voltage portfolio that eliminates SF6 with scalable and reliable solutions for the lowest carbon footprint. The innovative portfolio contains a range of EconiQ high-voltage products of up to 420 kV with the potential to reach ultra-high voltage levels. It also includes the replacement of SF6 in existing equipment called EconiQ retrofill and the newly added EconiQ gas-insulated current transformer that is built on a well-proven design. These eco-efficient solutions retain the best of SF6 and are proven to be 100% as reliable as conventional solutions while reducing equipment size, materials, and carbon footprint throughout the product lifecycle.
Digitalising the infrastructure
The fourth set of requirements is to improve operation and maintenance for a more reliable and longer service life. Advanced control systems not only operate automatically, minimising downtime and electrical losses during the lifecycle of the solar plant, but also monitor the condition of the equipment and enable predictive maintenance to extend the plant’s lifetime.
An example of this application is Metaenergia in Italy, for which Hitachi Energy provided grid connections for seven standby power plants that provide essential extra power when variable solar and wind energy does not meet demand. Each grid connection is equipped with digitally enabled lifecycle management and optimisation to enable predictive maintenance and ensure that all the equipment is working optimally. The intelligent software tools in the solution build on the experience and data of thousands of installations to predict and assess the condition of each component. Similar control systems are used for solar plant grid connections, such as a recently commissioned 750 MW solar plant in India. There, risk monitoring and diagnostics for the 220 kV grid connection are key features of the operations and maintenance platform.
Digital twins
In addition to digital condition monitoring and maintenance management software, digital twin technologies allow a virtual copy of each grid connection or power quality solution and provide a safe and efficient environment for analysis and learning.
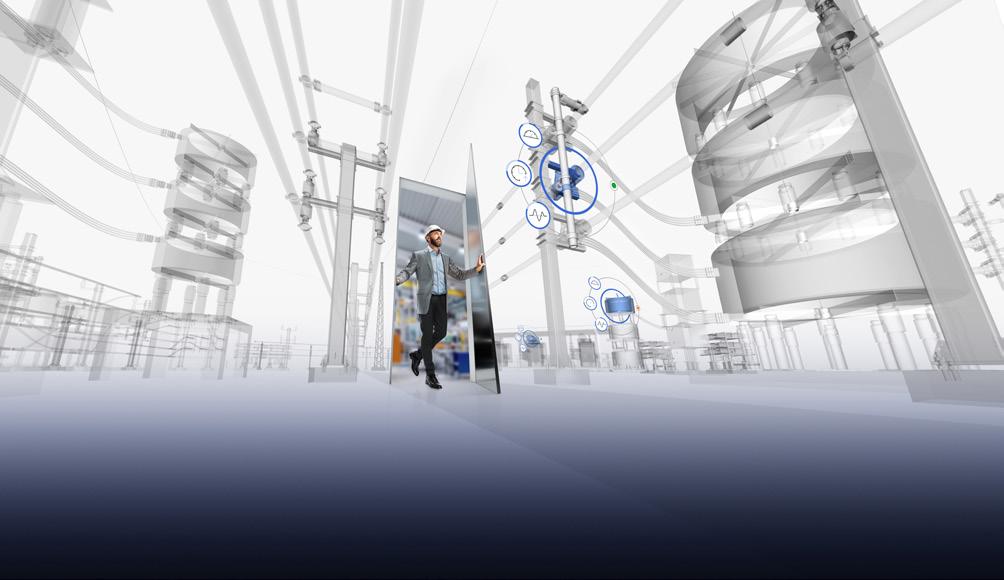
This is the case of the IdentiQTM digital twin from Hitachi Energy, which provides all the relevant asset information, analytics, and operational data in an intuitive and easy-to-navigate dashboard that users can customise to match their needs. IdentiQ includes 3D interactive visualisation of the complete asset, combined with one-click access to all the associated plant and equipment information, including engineering documentation, operational and maintenance procedures, safety training, and live operational data for monitoring and analytics.
Digitalisation is essential to making electricity the backbone of the energy system and advancing a sustainable
Figure 4 . The modular prefabricated connection at Sunny Rich’s combined fish farm and solar photovoltaic power plant.
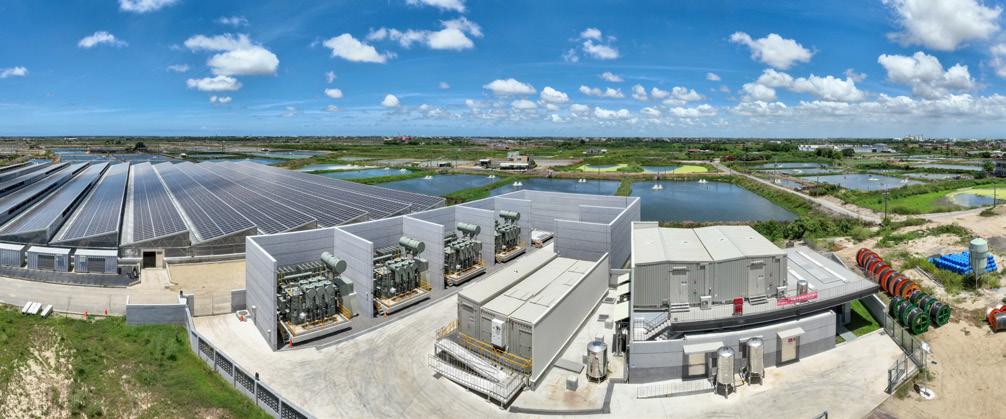
Figure 5 . IdentiQTM is a digital twin solutions for the complete life cycle. It provides clear insights and state-of-the-art data visualisation.
energy future for all. It is key to the integration of bulk and distributed renewables, as well as the electrification and decarbonisation of sectors such as transportation, industries, and data centres.
Collaborating to accelerate the energy transition
Hitachi Energy has pioneered many of the technologies needed for advancing a sustainable energy future for all and are committed to continue pushing the boundaries of innovation. Grid connection and power quality solutions, such as those presented in this article, facilitate the widespread integration of solar power into global power systems.
There are many alternatives available, and choosing the right solution can become challenging for developers, investors, construction companies, and operators. Performing the right engineering approach with an experienced power systems consultant is essential. Dynamic simulations, grid impact studies, and grid code compliance studies are among the first steps when developing a new solar power project or extending an existing solar plant. And, not least of all, these studies should include a lifecycle assessment of the environmental footprint and carbon emissions of the grid connection over its entire service life.
References
1. ‘Net Zero by 2050’, International Energy Agency, www.iea.org/reports/net-zero-by-2050
2. ‘Renewable capacity highlights’, International Renewable Energy Agency, (11 April 2022), www.irena.org/-/media/Files/IRENA/Agency/Publication/2022/Apr/ IRENA_-RE_Capacity_Highlights_2022.pdf?la=en&hash=6122BF5666A36BECD5AAA2050
B011ECE255B3BC7
3. Vietnam Trung Nam Tra Vinh Solar Power Plant.
he global transition to renewable energy is accelerating, spurred on by increased political will seeking higher energy security and independence in the wake of the global energy crisis.1 An increased proliferation of solar and wind power plants delivering intermittent energy supply is being experienced. In order to ensure that this increased energy production delivers a stable and reliable stream of energy, effective energy storage technology is required.
According to the International Renewable Energy Agency’s (IRENA) World Energy Transitions Outlook 2022, an increase in energy transition investment of 30% between now and 2050 will be required to achieve net zero by 2050.2 This represents a total of US$131 trillion, a multiple increase in investment to drive what amounts to a realignment of the global energy sector. A significant change is already well underway and accelerating, as a drive towards renewables ramps up all across the world. In the EU, there was a record 13% y/y increase in electricity generated from solar photovoltaics and wind from March to September 2022.3
Across the other side of the Atlantic, the renewable energy sector is thriving even more. According to data from the Latin American Energy Organization (OLADE), Latin America and the Caribbean have 25% of renewable energies in the composition of their primary energy grid.4 Renewables, grids, and storage now also account for more than 80% of total global investment in the power sector.5
Careful and strategic planning is required to ensure a reliable and steady flow of this new energy. This development towards renewables comes with a more variable and more volatile energy resource. Often characterised with regional and seasonal variations, this energy varies month by month, day by day, and hour by hour. Such variability creates challenges to providing the stable and reliable supply of energy which is so crucial to economies. Energy supply has been described as ‘the apex commodity of the global economy […] an input in the production process and an important part of the supply chain of commercial firms.’6
Energy storage systems are a foundation stone that ensure a balanced and reliable energy supply and, as such, empower self-sufficiency. This is true for the majority of nations, from developed economies, such as Germany, Australia, and the UK, across to small island development states, such as in the Caribbean or Asia Pacific. National policies are driving the building of renewable energy
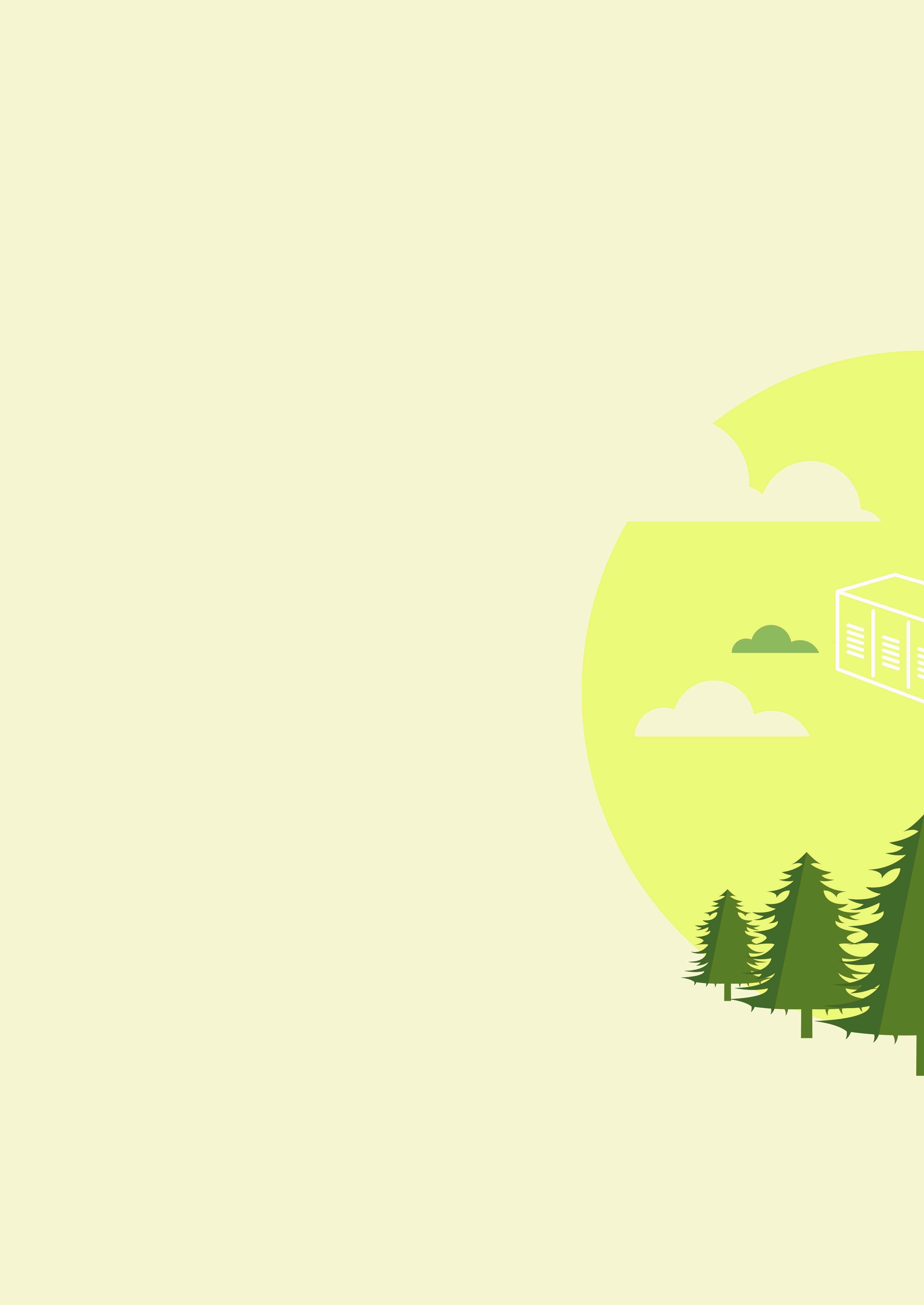
ance of energy storage technologyastheworld
shifts
storage to reduce dependence on energy imports, enhance systems reliability, and progress towards decarbonisation.7
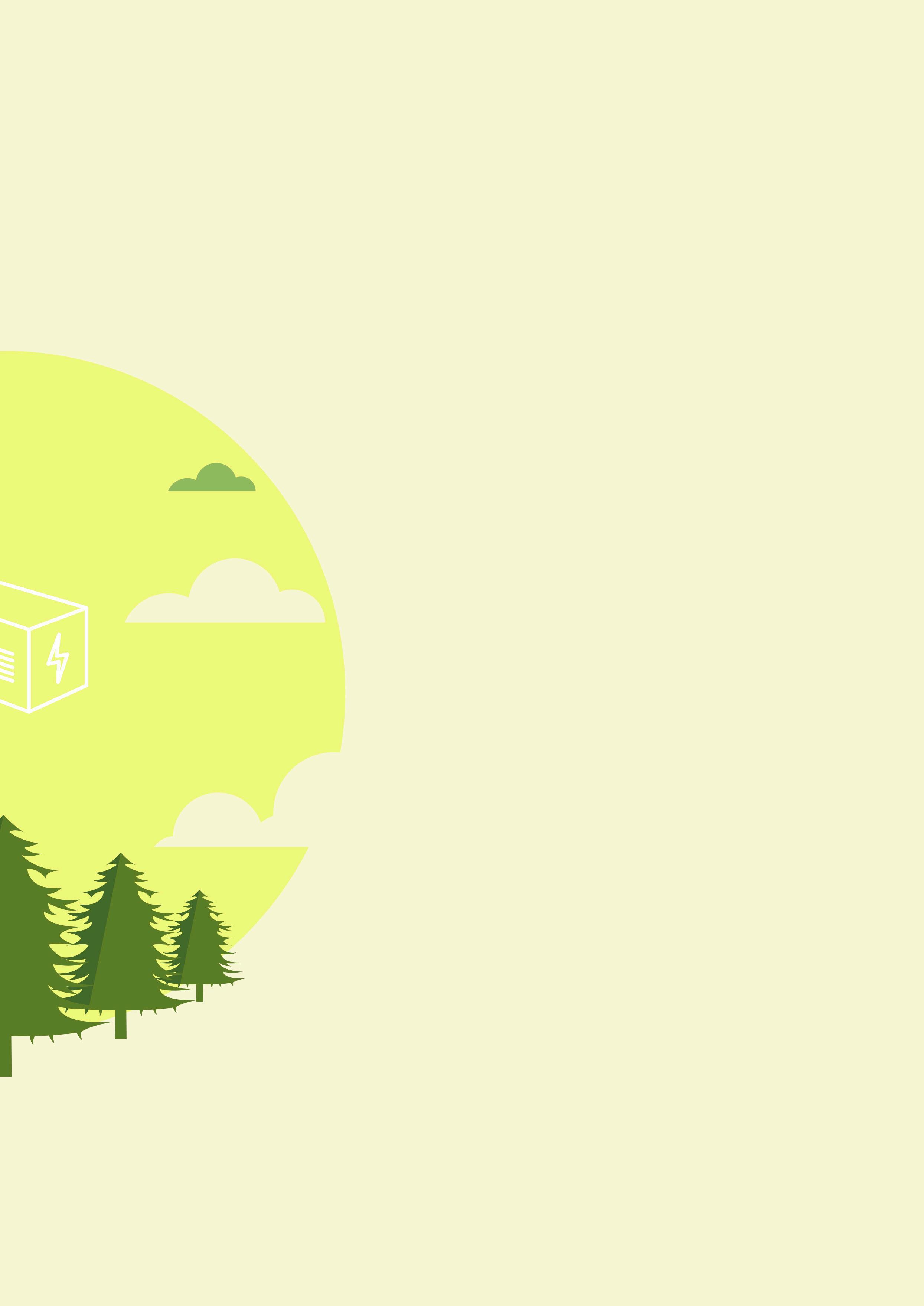
Energy storage technologies
One of the fundamental purposes of energy storage technologies is to secure an ongoing balance in energy supply. Excess energy is stored when demand is lower than energy production, with this excess released when demand exceeds supply. There are a variety of energy storage systems available, extending from systems employing mechanical techniques to battery technologies and more.
toward
s
renewab
l e ene r g y?
A battery energy storage system (BESS) is a prevalent choice, capturing energy from different resources, accumulating this energy, and storing it in rechargeable batteries. This energy can later be discharged for use to homes, electric vehicles, and industrial and commercial facilities, as batteries release energy during periods of peak demand, power outages, and other applications.
The battery storage sector is currently dominated by lithium-ion batteries, which includes a graphite negative electrode, and a lithium cobalt oxide or lithium iron phosphate positive electrode. The electrolyte is lithium salt in an organic solvent. Lithium’s lightness and high-energy density sees it hold a distinct advantage from others in the sector. However, the composition of batteries is still rapidly evolving, with developments addressing factors such as the application model.
Energy storage technology extends beyond batteries. Pumped-storage hydropower is one of the oldest types of storage and includes dumping excess energy. Renewable energy sources can utilise this approach, using excess energy to pump water uphill to fill a reservoir, water which is later released through a hydroelectric turbine to convert back to electricity.
Compressed air is an evolving technique employed in Latin America. Renewable energy is used to compress air, which is later relieved at a time when additional energy excess is needed. Another approach used is called mechanical energy storage, which harnesses motion or gravity to store electricity – for example, a flywheel is a rotating mechanical device that is used to store rotational energy that can be called up instantaneously.
Research and development
Battery technology is now amongst the most widely used of the energy storage options, and attracts the focus of much research and development in the sector. The battery market
will grow to US$168 billion by 2030, with most of the growth in battery revenues stemming from lithium-ion batteries. Battery storage power will also reach 353 GW by 2030, compared to 15 GW in 2022. The installed capacity is expected to grow at a CAGR of 43.3% from 2020 to 2030.8 This growth will drive a transformation of the global electric grid towards sustainable energy.
One challenge that can be addressed by research and development into batteries is increasing long-term duration storage. Battery storage can extend from only two hours to four or five hours, achieved by more mature and advanced technologies. For longer-term duration storage, newer technologies have evolved within the battery space with different combinations of metals. There is now increased pressure for cell makers and their component suppliers for ever more effective materials and chemical mixes. The next 2 – 3 years will see nickel increasingly substituted for cobalt as the cathode stabiliser in order to reduce dependence on supplies from the Democratic Republic of the Congo (DRC).8
Advanced battery chemistries are also being developed that may offer advantages over commercially available batteries. Performance advantages include a lighter weight, higher energy density, broader temperature tolerance, extended lifecycle, and improved safety. One such technology is a vanadium flow battery that stores energy in an electrolyte liquid form which is separated by a proton exchange membrane.9 This liquid is pumped around large tanks and driven through a ‘cell’ for conversion into power when required.10 Vanadium is an abundant metallic resource, more so than copper, plus the material is highly recycleable with ions simply moving between oxidation states rather than being destroyed or degraded.
Sustainable energy storage
Looking to the future, energy storage technology research should focus upon battery efficiency and extending related lifecycle costs and resources. Manufacturers should reduce the amount of rare earths that need to be extracted from the ground, lessening disruption for nature and for people in places such as the DRC. At present, the predicted growth of lithium-ion
batteries could put pressure on supply chains for a broad mix of materials such as lithium, nickel, cobalt, and graphite.
The World Economic Forum (WEF) has provided counsel on environmental, social, and governance (ESG) risks within the battery value chain, including working conditions, local air, water and soil pollution, and biodiversity loss. A WEF paper co-authored with the Global Battery Alliance emphasises that ‘acting now is […] a chance to shape an emerging value chain, while acting later requires costly reconfiguration and leads to the exacerbation of social and environmental impacts.’11
The EU is heeding the WEF’s call to legislate to address ESG challenges and in a timely manner. A draft battery regulation proposal has been tabled by the European Commission, to secure the sustainability and competitiveness of EU battery value chains. Proposed measures include minimum recycled content, performance and durability criteria, and due diligence obligations for sourcing raw materials.12 The advancement of such regulation will hold feet to the fire, advancing current and future battery storage technology in a way that rings true to the core aims of the Paris Agreement and incorporating broader ESG considerations.
The electrification of the energy sector should go hand in hand with a drive to consider the sustainability of storage technology materials, and a move towards recyclable, less resource intensive materials. A carbon neutral future requires storage technology to make renewable energy a viable option to drive dynamic economies around the world – the key to unlocking a vibrant renewable sector. It should be kept in mind that a holistic view of a sustainable future extends not just to reducing carbon emissions through cleaner sources of energy, but also the sustainability of the infrastructure that underpins this renewable energy.
References
1. ‘Ukraine crisis will speed energy transition in mid-term, says renewables agency chief’, Reuters, (2022), www.reuters.com/business/sustainable-business/ukrainecrisis-will-speed-energy-transition-mid-term-says-renewables-agency-2022-09-27/
2. ‘World Energy Transitions Outlook: 1.5˚C Pathway’, International Renewable Energy Agency, www.irena.org/publications/2021/Jun/World-Energy-Transitions-Outlook
3. DE POUS, P., PATULEIA, A., BROWN, S., and ROSSLOWE, C., ‘More renewables, less inflation: Restoring EU economic stability through investment in renewables’, E3G and Ember, (2022), https://ember-climate.org/app/uploads/2022/10/E3GEMBER-Briefing-More-renewables-less-inflation.pdf
4. ‘Towards the energy transition in Latin America and the Caribbean’, Latin American Energy Organization, (2020), www.olade.org/en/noticias/towardsthe-energy-transition-in-latin-america-and-the-caribbean/
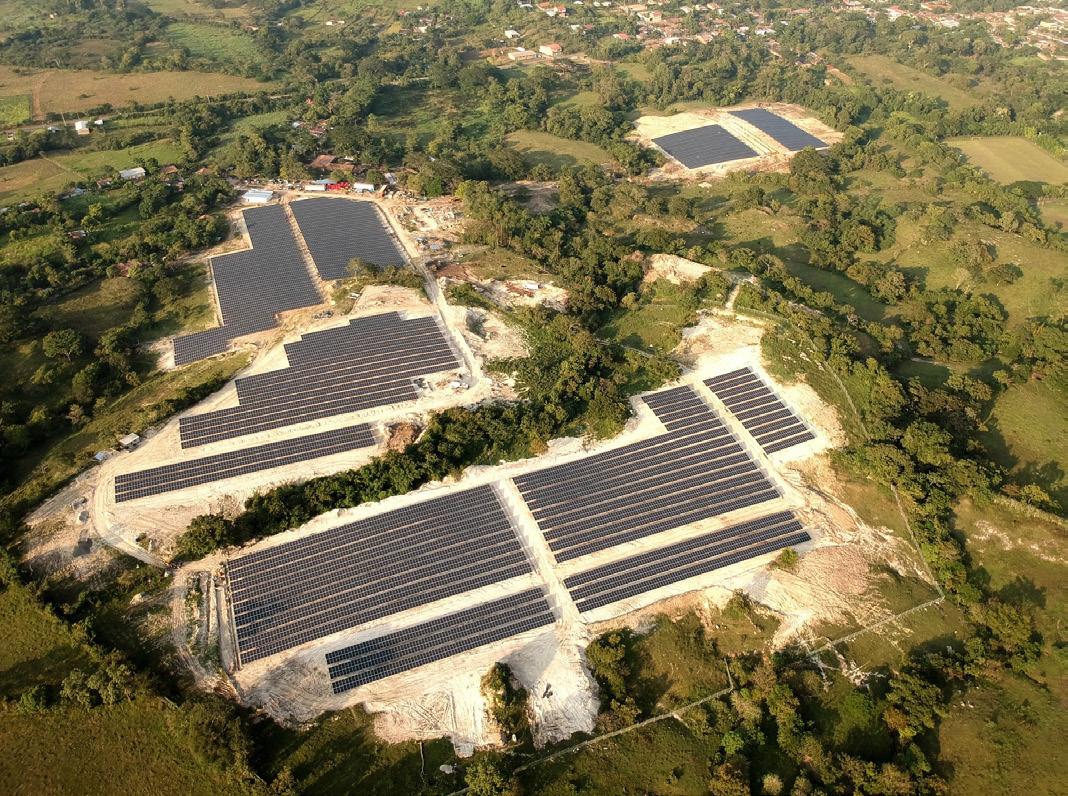
5. ‘Record clean energy spending is set to help global energy investment grow 8% in 2022’, International Energy Agency, (2022), www.iea.org/news/record-clean-energyspending-is-set-to-help-global-energy-investment-grow-by-8-in-2022
6. ‘Essays on energy the economy and the environment, University of Portsmouth www.port.ac.uk/study/postgraduate-research/research-degrees/phd/explore-ourprojects/essays-on-energy-the-economy-and-the-environment
7. ‘The global energy storage market’, Deloitte UK, www2.deloitte.com/uk/en/pages/ energy-and-resources/articles/global-energy-storage-renewable-energy-storage.html
8. ‘Batteries in Power – Thematic Research’, GlobalData, (2022), www.globaldata.com/store/report/batteries-in-power-theme-analysis/
9. RAPIER, R., ‘Why Vanadium Flow Batteries May Be The Future OF Utility-Scale Energy Storage’, Forbes, (2020), www.forbes.com/sites/rrapier/2020/10/24/why-vanadiumflow-batteries-may-be-the-future-of-utility-scale-energy-storage/?sh=2e7052662305
10. LIMB, L., ‘The race for renewable batteries: What’s the future of solar and wind storage?’, Euronews, (2022), www.euronews.com/green/2022/07/21/the-race-forrenewable-batteries-whats-the-future-of-solar-and-wind-storage
11. ‘A Vision for a Sustainable Battery Value Chain in 2030: Unlocking the Full Potential to Power Sustainable Development and Climate Change Mitigation’, World Economic Forum and Global Battery Alliance, (2019), www3.weforum.org/ docs/WEF_A_Vision_for_a_Sustainable_Battery_Value_Chain_in_2030_Report.pdf
12. ‘A new EU regulatory framework for batteries’, Plenary Session of the European Parliament, (March 2022), www.europarl.europa.eu/RegData/etudes/ ATAG/2022/729285/EPRS_ATA(2022)729285_EN.pdf
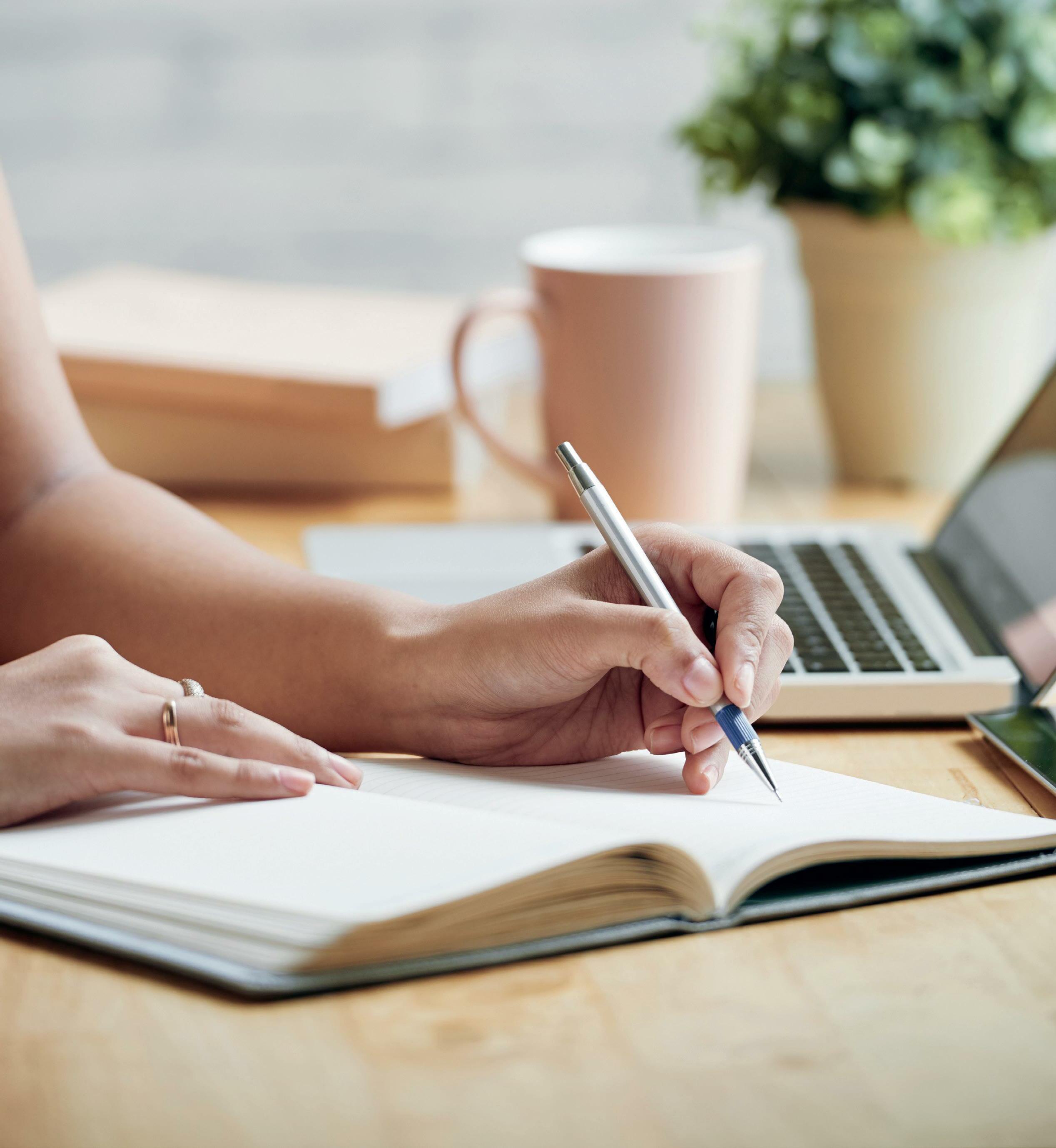
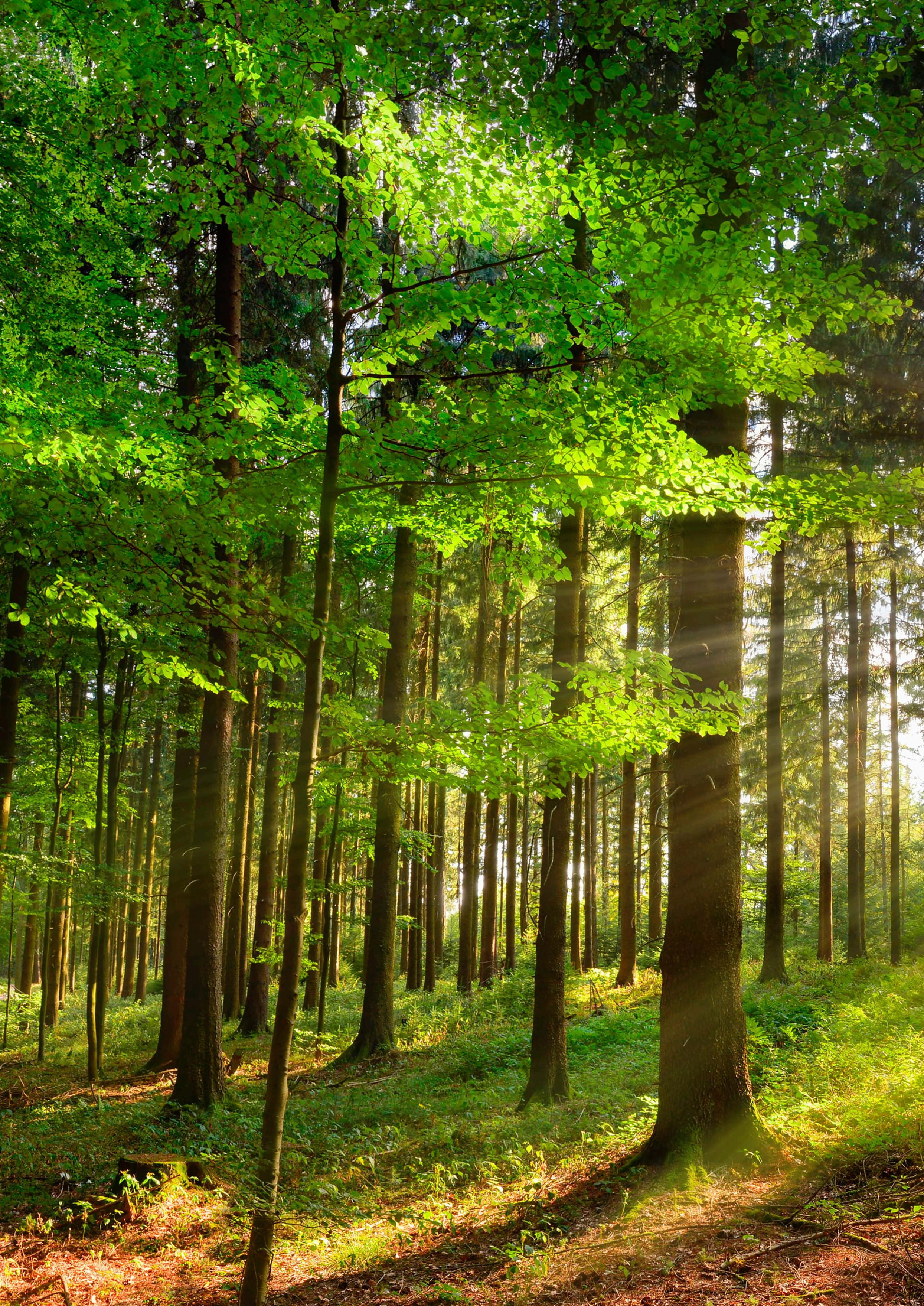
Mark Rowcroft, Development Director, Exagen, UK, considers the perennial solar issue of ‘dunkelflaute’ – and similar dark lulls for international solar development policy.
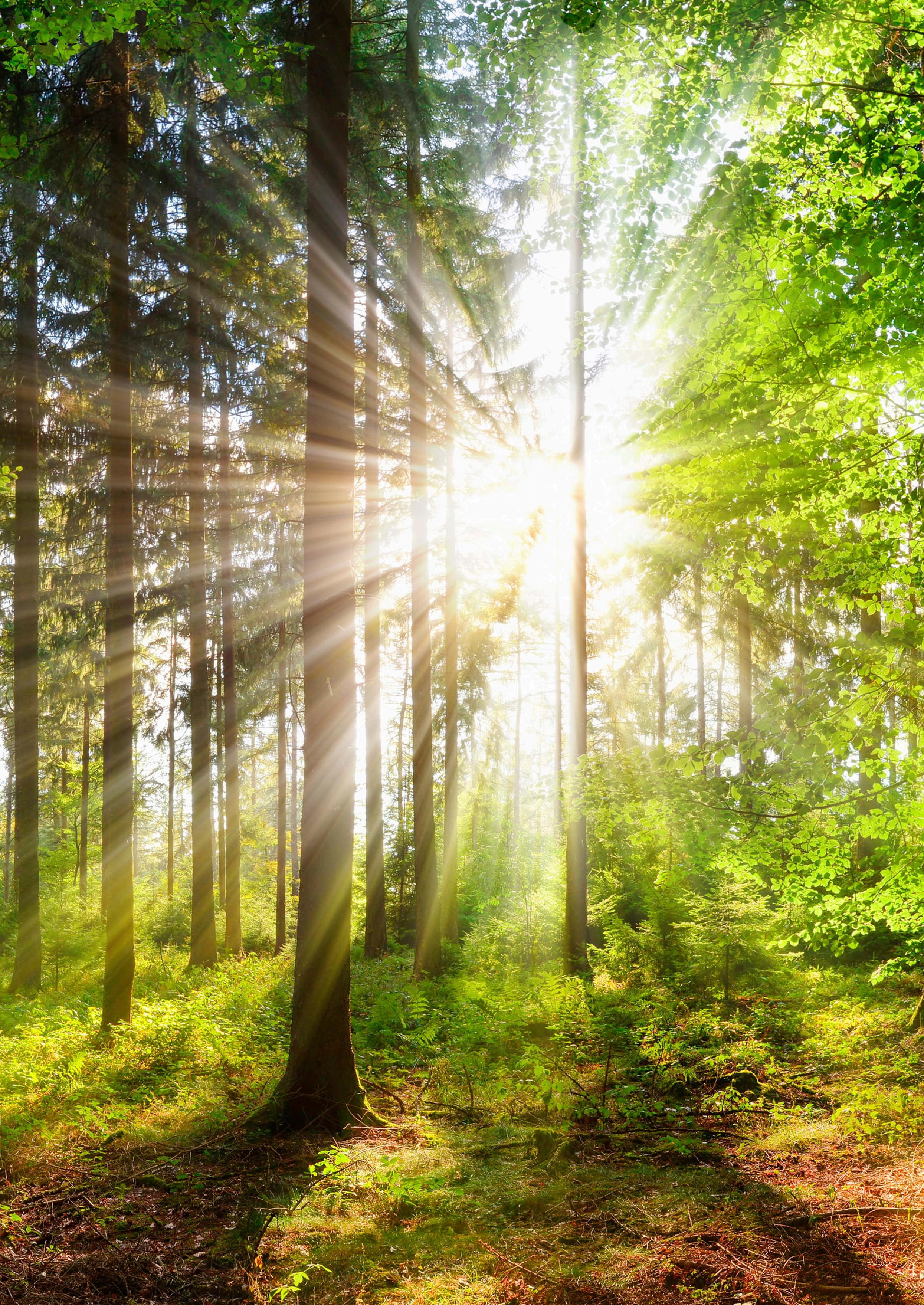
Writing from the UK, ahead of what will be a significant winter energy crisis with many people plunged into fuel poverty and struggling to heat their homes, it is difficult to picture a sunlit energy landscape. Driven in part by Russia’s invasion of Ukraine, the current energy outlook seems bleak: high prices, continued fossil fuel exploration, reneged promises, and climate crisis commitments going unmet.
But zooming out from this current crisis, the renewables picture is positive, and solar is currently enjoying its moment in the sun, with global solar generation increasing by a record 179 TWh (up 22%) last year. Furthermore, – according to the International Energy Agency, the world’s solar fleet reached 1 TW capacity in April 2022. It remains the fastest-growing renewable energy source and the third largest renewable electricity technology behind hydropower and wind.
The global governmental roadmaps towards net zero,
though, do not quite reflect this sunny outlook. According to the International Renewable Energy Agency’s (IRENA)
Renewable Energy Roadmap to 2030, total governmental projections yield less than 500 GW of solar PV in 2030 – vs the IRENA roadmap demonstrating that market trends and enabling policies combined could result in 1250 GW.
The global energy mix is still dominated by coal, oil, and gas, with the countries that lead on renewables doing so by leaning, naturally, on their natural resources: Sweden – on track for 100% renewable energy by 2040 – favours hydropower and bioenergy; Iceland takes advantage of hydropower and geothermal energy (which heats nine out of 10 homes); and countries abundant in sun lead the solar pack, such as China, the US, Japan, and Morocco – the latter of which houses the world’s largest concentrated solar farm, the Noor-Ouarzazate complex in the Sahara desert. And herein lies what has historically been one of solar’s biggest issues: dunkelflaute.
Dunkelflaute – literally, ‘dark wind lull’ – describes time periods where the sun (and wind) is not abundant, or not enough to meet energy demands, leaving countries to fall back on alternative sources: hydro, nuclear, fossil fuels and, more limitedly, energy storage. Treating solar as ‘making energy while the sun shines’ and using greenhouse gas-emitting fossil fuels as a safety net, undermines the benefits that renewables promise: cost, security, and emissions reductions.
Historically, renewables development has followed the same broad prescription globally: find land, find capacity, achieve consent, secure funding, hit subsidy window, build or sell. In the UK, this recipe has worked for onshore wind backed by renewables obligation certificates (ROCs), for 5 MWp solar backed by feed-in tariffs (FIT) and even for offshore wind underpinned by contract for difference auctions. All these schemes boosted renewables, growing operational fleets until the once-immature technology could stand alone. But now, solar needs to take the next step.
Policy casts a shadow over the future of solar
At the time of writing, the UK’s solar sector is lit up with rumours that the new environment secretary had plans to limit solar development by redefining ‘best and most versatile’ land
(BMV), which is earmarked for farming and from which solar development is discouraged, to include the middling-to-low category 3b. The redefinition would exclude solar from approximately 41% of England’s land area, with much of what is left – grade 4 and 5 land – unsuitable for solar developments, and threaten 30 GW and £20 billion capital investment in projects slated for 2025 onwards.
There are echoes here of a 2015 government decision, designed to appease rural Members of Parliament, that outlawed onshore wind in England, overturning local planning decisions and forcing developers to fold or shift focus to Scotland. If the government’s threat is realised, this change would affect landowners hoping to diversify their income, developers who will lose access to the most abundant and accessible land for generation, and stall one of the country’s fastest-growing industries. The decision has deep implications for multiple stakeholders in the industry – and the UK’s net zero ambitions.
It is a similar picture globally: last summer saw reports that China was weighing new restrictions on land use to restrict the ability of solar developers to build on arable land or on coastlines and riverbanks, while in its bioeconomy plan, the EU is weighing up just how much one piece of land can do, and the trade-off between energy security and food security – both heavily impacted by Russia’s war on Ukraine. With interim 2030 net zero targets looming, each solar-delaying policy decision begs the question: exactly how committed are governments to decarbonising the world’s power networks? Solar infrastructure and solar investment are queued: as projects threatened to wither on the vine.
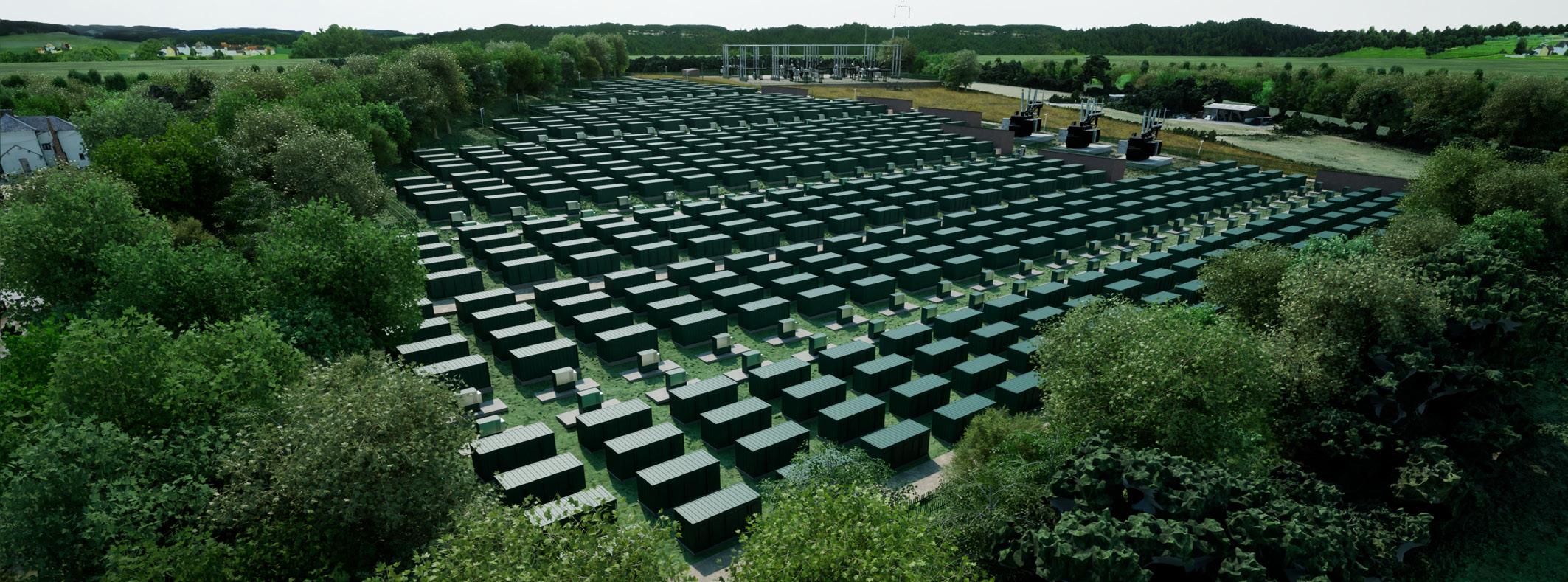
Grids need to get up to speed
Across the world, the solar technology is ready to plug and play – the problem is finding the proverbial socket. The grid networks simply are not able to keep up, and there are further policy decisions to be made around funding grids that are more accommodating to distributed generation. Where is the most equitable place to put that cost? Bill-payers around the world are already stretched to capacity by soaring energy bills, and increasing taxation to fund it is a difficult policy decision to take during a cost-of-living crisis.
Networks are also, as a rule, designed around peak capacity: a 50 MW solar farm needs a 50 MW hole to fill. But, often, the grid
is merely contractually ‘full’ – it is rarely physically full. The capacity is there but allotted to the peak export of a gas peaker plant or a wind farm with a 40% capacity factor – the balance going unused and with every new developer joining the ever-lengthening queue to plug in one supergrid transformer at a time. One answer might be to cluster development: for example, five solar farms designed together, with a common connection point. The caveat is that all five farms now have common programmes – if one farm hits a snag, such as planning, all five are delayed, and what investor will accept an open-ended programme? There is no one-size-fits-all, but there are more solutions.
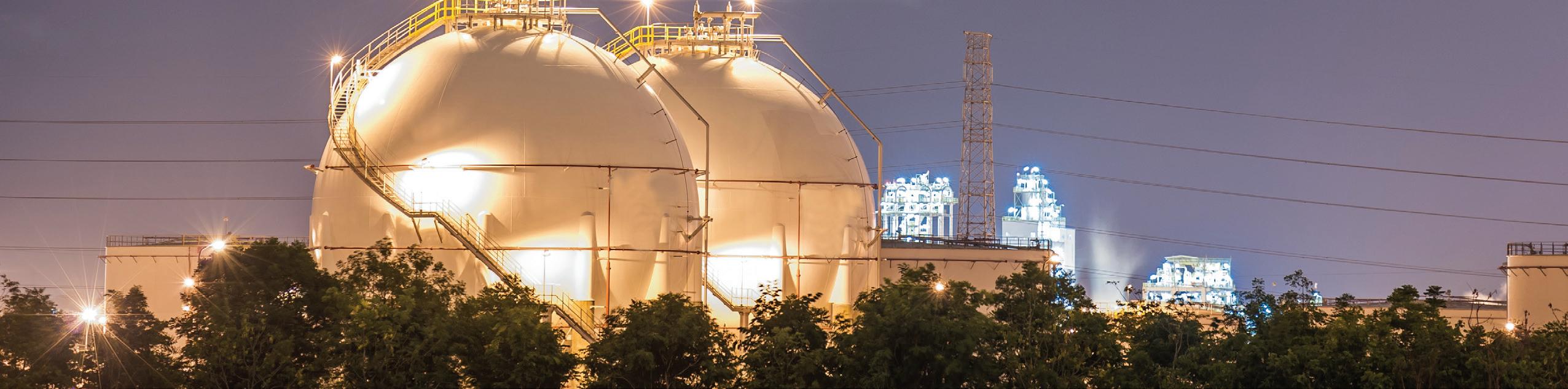
Batteries must be included
All of Exagen’s solar development projects are co-located with battery storage, to better manage the import and export on the connection and add flexibility to the network. Batteries can combine with initiatives such as time-of-use tariffs, incentivising people to move their load to times of day when there is more power available and lessening peak demand. Increasingly, network-connected tools, such as smart meters and smart appliances – Wi-Fi-enabled washing machines, for example – allow consumers to shift their power demand to a different time, which in turn makes the network more intelligent. And a flexible network with a greater level of intelligence will not need as much peak power, lessens the reliance on gas, and accommodates more renewables onto the network. Co-locating battery storage with farms is a way of working in tandem with this smarter network.
Exagen also has another battery-powered answer: the company’s first mega-battery project, Normanton Energy Reserve in Leicestershire, the UK. This 275 kV energy park has a peak capacity of 500 MW and a duration of 2 hrs, providing the energy equivalent to 235 000 UK homes’ average daily electricity usage while also maximising opportunities for nature enhancement, delivering a net biodiversity gain of more than 20% (double the requirements of the Environment Act). Ultimately, mega batteries could be the silver bullet that takes gas out of the picture, because they can store big volumes of power until the network needs it. Greater manufacturing capability and energy density improvements, as well as the general move to ‘electrify everything’, underpin the case for such projects, and emerging chemistries may increase their application further as durations and cycle counts increase.
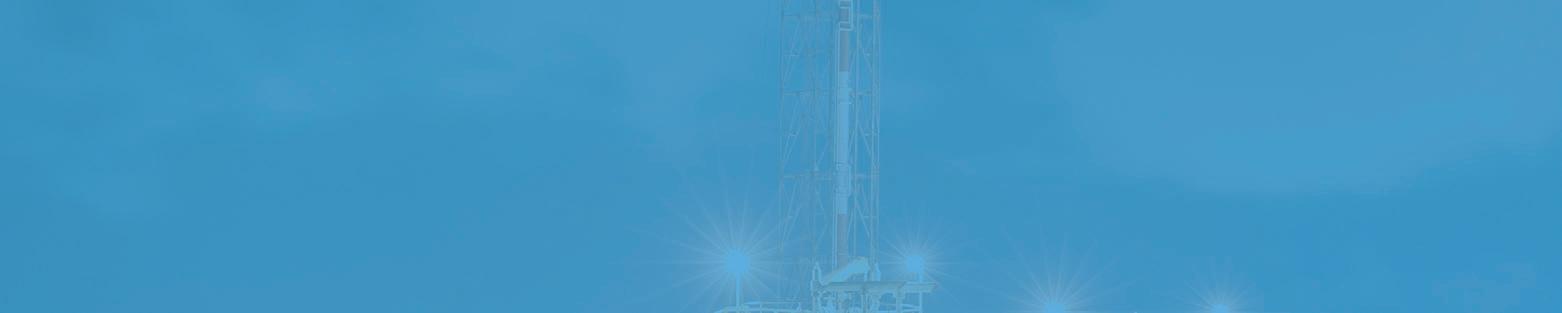
The time is now
Of course, smart technology, such as Wi-Fi-enabled washing machines, currently have a high bar to entry as it is all new-to-market. And at a time when, particularly in the UK, consumers are facing a squeeze on their budgets from all corners, smart technology cannot be expected to save solar. Network operators, governments, developers – everyone must start taking those first steps to commit to the smart path. Many of Exagen’s transmission-connected projects have a 5 – 6 year delivery horizon, so there is some way to go, but solar developers are not trying to deliver change overnight – they will wait for the sun to come up.
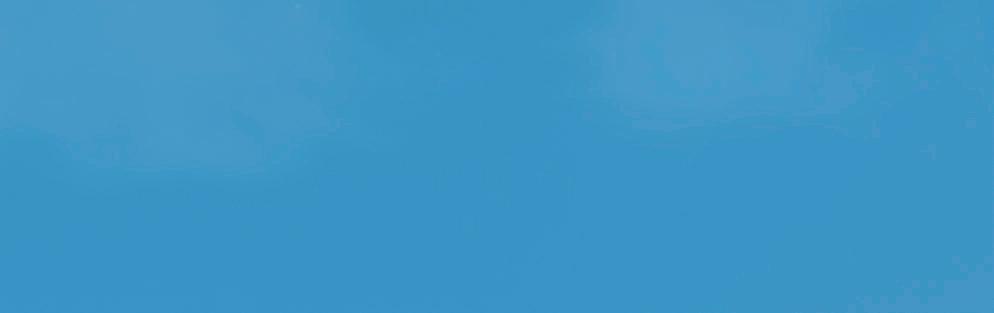
discusses how recycling solar
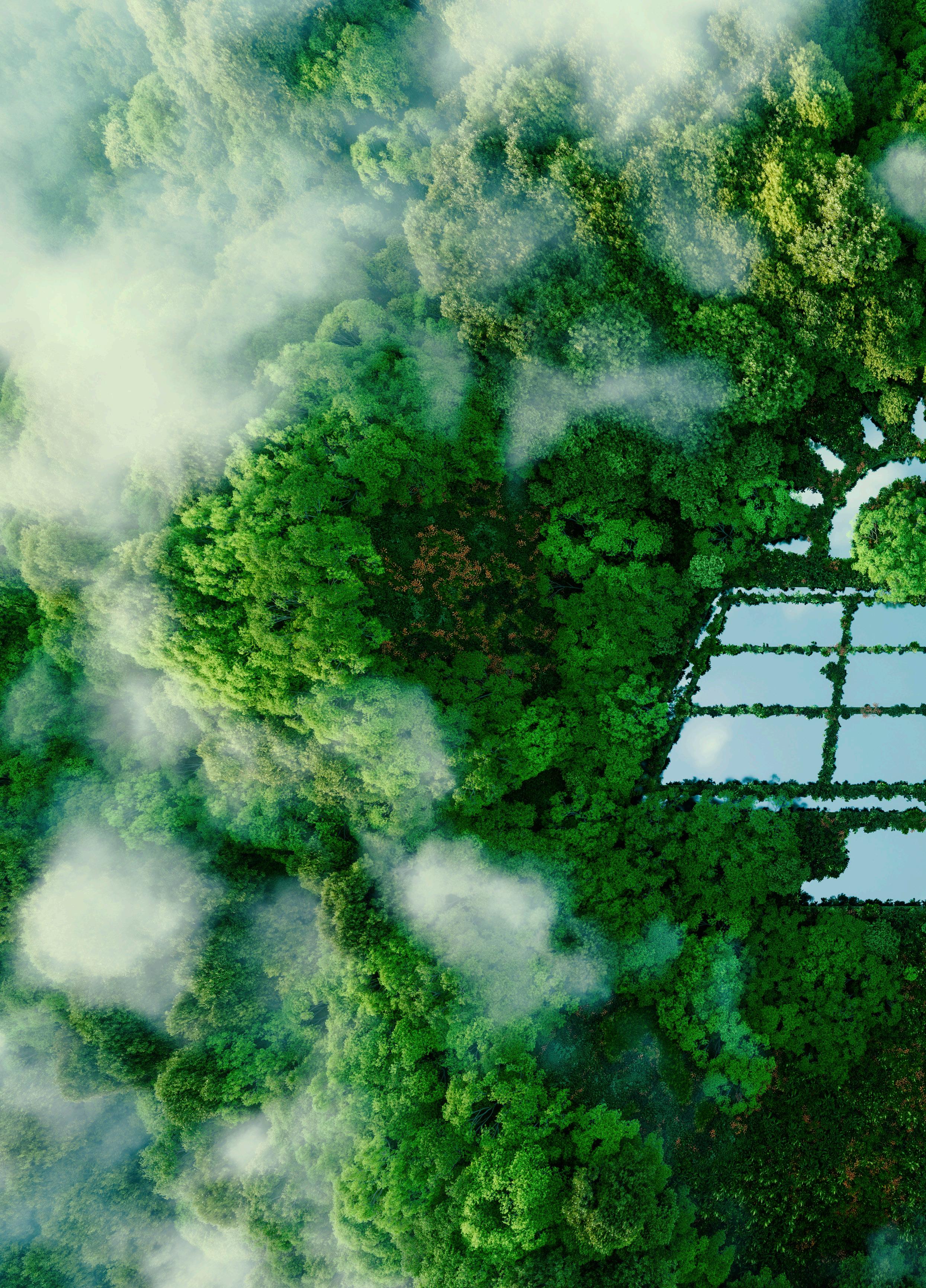
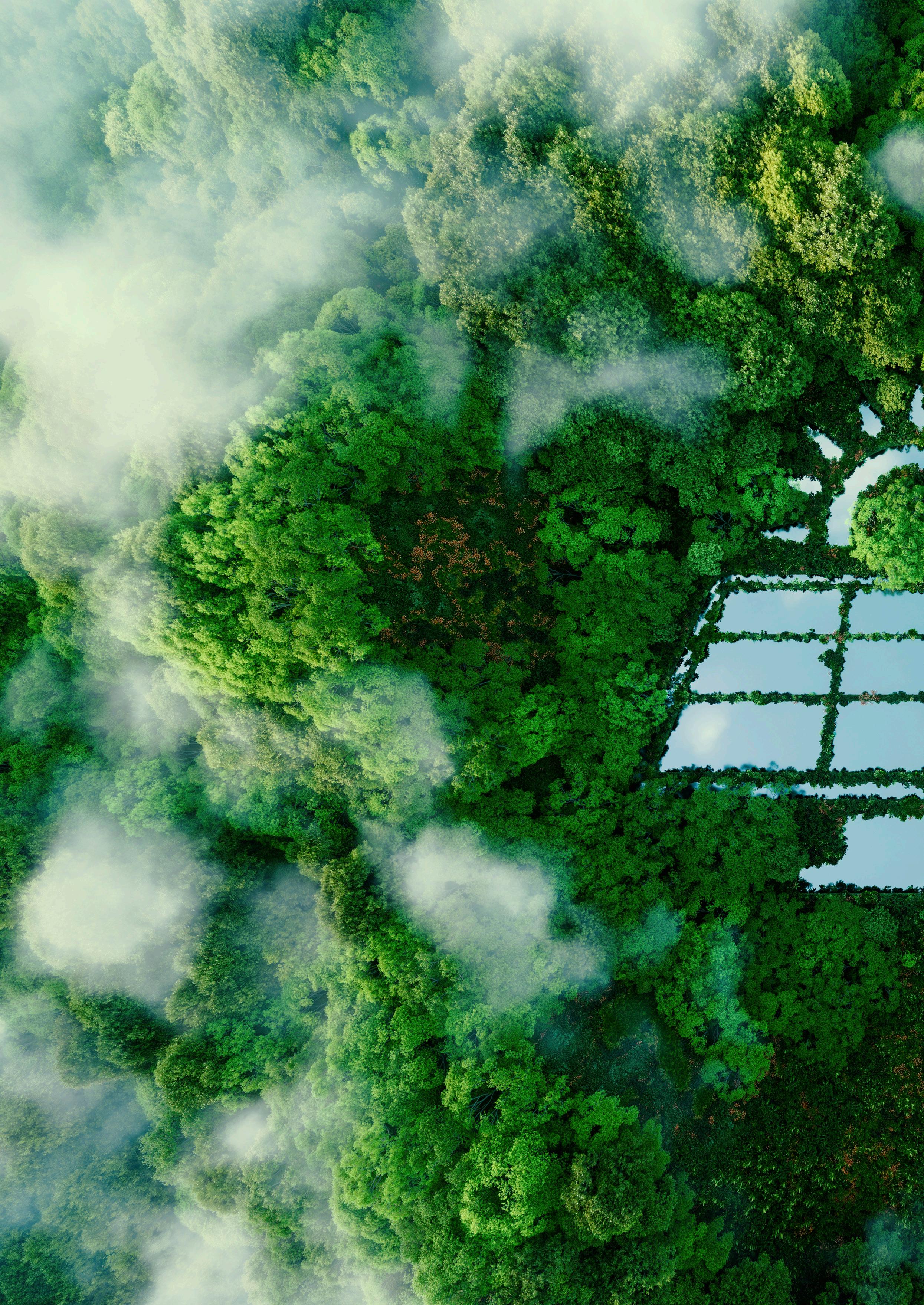
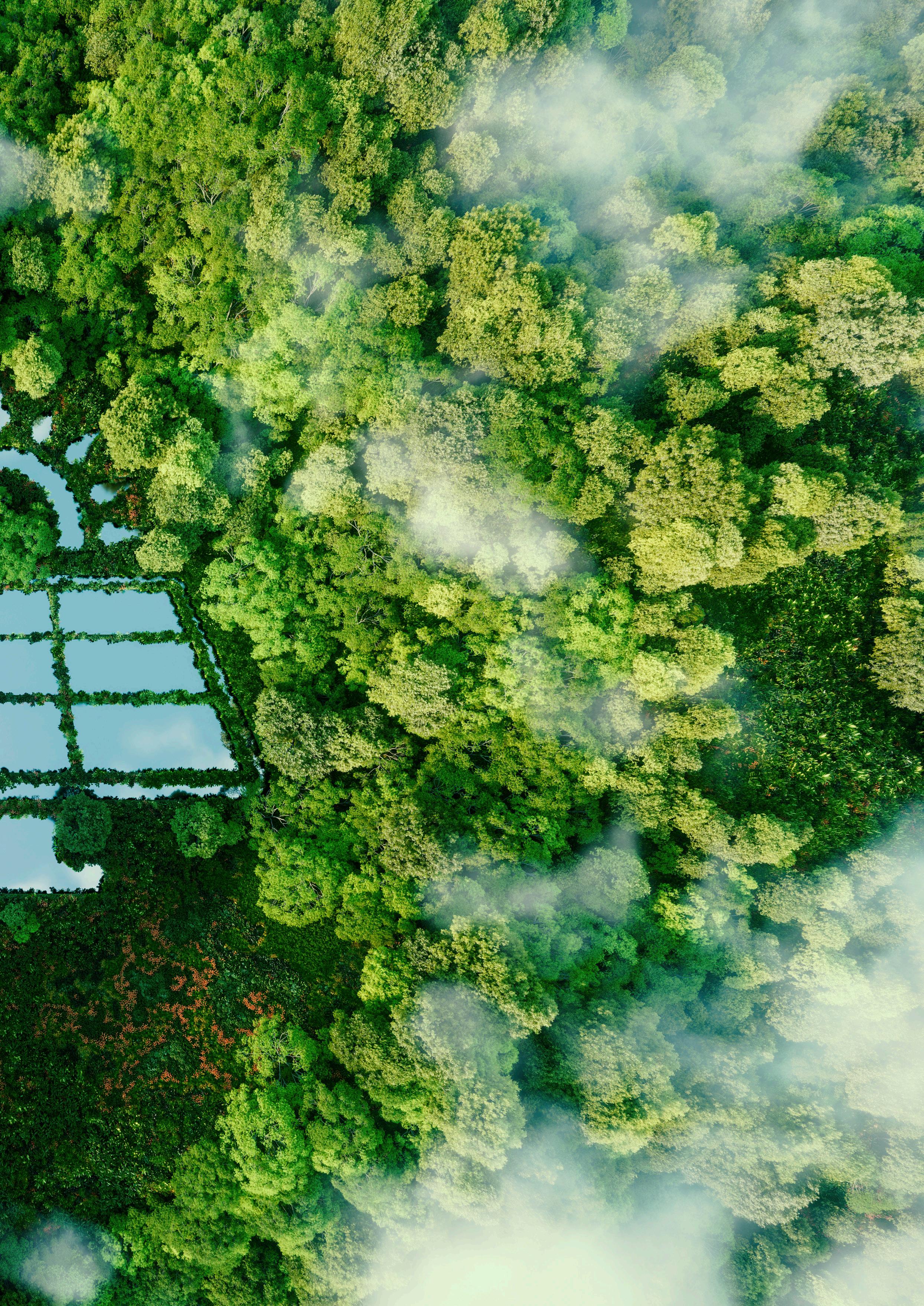
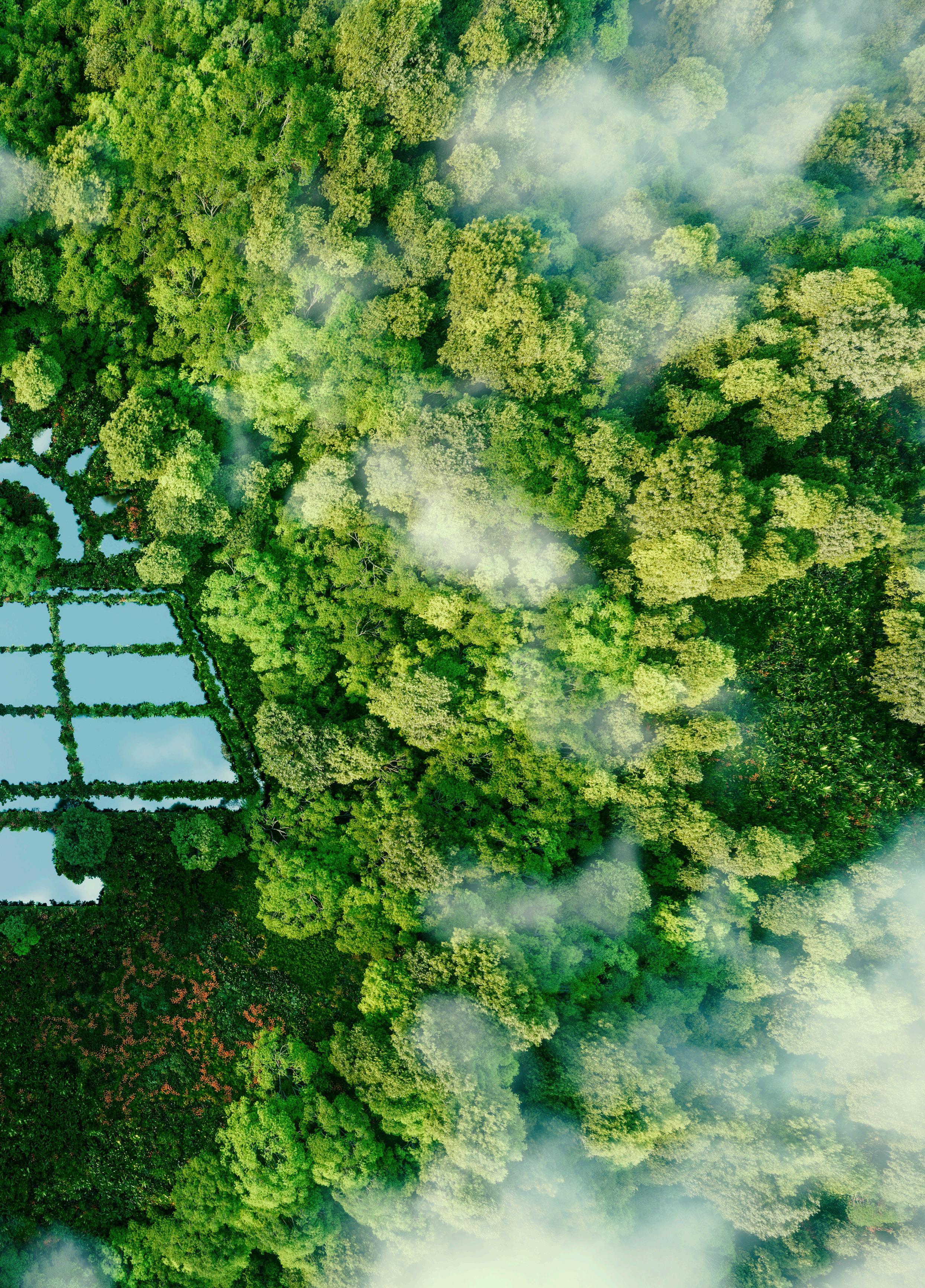
However, there are many good reasons why this issue should start being taken more seriously now. The good news is that many governments have already started introducing frameworks for recycling, reusing and, if neither is possible, disposing of dead solar panels. For example, in the UK and many European countries, collection, recycling, and recovery targets for all types of electrical goods, such as solar panels, are set according to the Waste Electrical and Electronic Equipment Directive (WEEE Directive). Still, it is questionable whether the capacity of the existing recycling facilities dedicated to electrical and electronic waste would be able to cope with the ever-increasing volume of solar panel waste. Moreover, conventional recycling processes for general electrical and electronic waste may not be able to deliver the best material recovery rate for solar panel waste. With the rise of high-efficiency PV cells made of novel semiconductor or organic materials, it is also questionable whether conventional electronic waste recycling and disposal processes, which are designed mainly with silicon electronics in mind, would be able to safely handle novel PV materials, some of which are known to be toxic to human health.
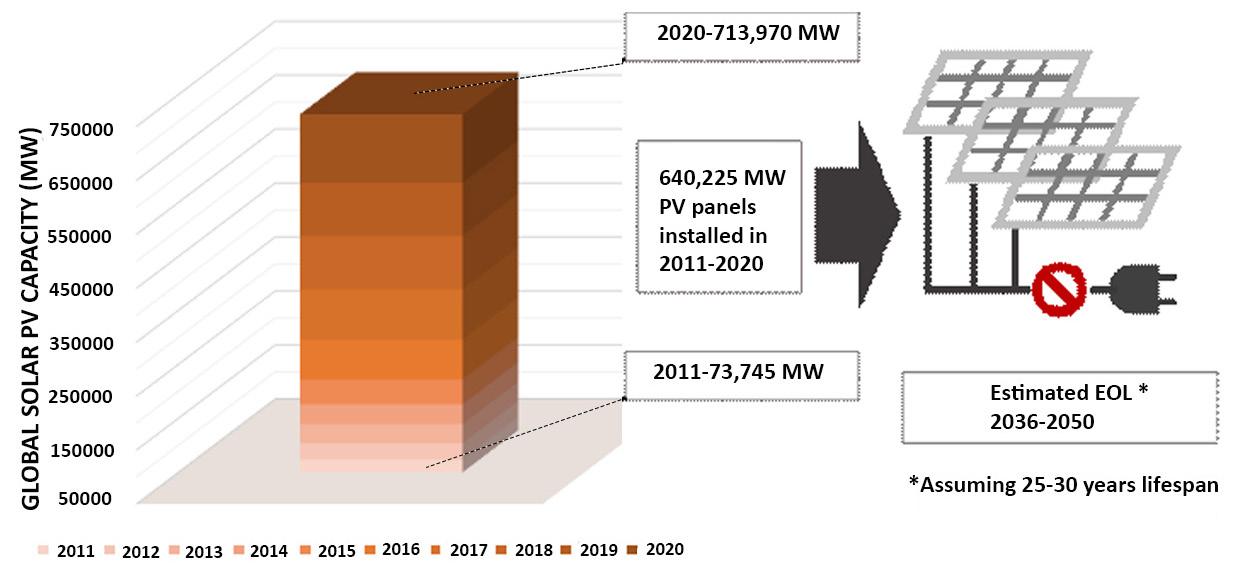
Material recovery
But why is there a need to worry about how to reuse and recycle EOL solar panels and their parts? Although the most obvious answer to this question would be to minimise the environmental impact that such large volumes of solar panel waste can have on the planet, this is not just an environmental issue. Solar panels are made of many components, including PV cells (electricity-producing devices), electrical connections, and support frame, all of which contain materials that can be reused or extracted for further use.
Currently, materials from EOL solar panels are typically recovered at general recycling plants (e.g. MRFs) alongside other types of electrical and electronic equipment.
These material recovery processes typically involve shredding EOL solar panels into small pieces and mechanically separating major components and materials of the panels. Such processes can already achieve high overall material recovery rates that are in line with the existing regulations, such as EU WEEE Directive. However, processes involving shredding typically accompany loss of high-value materials, such as silver, which are dispersed in form of dusts during shredding. So far, due to the relatively low volume of solar panel waste, such losses have been considered to be negligible. Nevertheless, without significant improvements of recycling process, the volume of unrecovered high-value materials would only increase along with the growing volume of solar panel waste.
Environmental impact
In view of the environmental impact, finding better ways to reuse and recycle EOL solar panels is an essential step in completing the sustainability cycle of solar PV power generation. Unlike the environmental impacts of manufacturing, installing, and maintaining solar panels and plants, the environmental impacts of dead solar panels have largely been neglected to date. However, according to a study 3, recycling 1000 kg of silicon PV waste produces greenhouse gas emissions of 4.46 x 10 2 kg carbon dioxide (CO 2) equivalent – most of which comes from transporting solar panel waste to recycling facilities, incinerating plastic materials from the panels, and further treatment for metal recovery from the bottom ash. Given that burning 1 l of petrol produces approximately 2.3 kg of CO 2, this is equivalent to burning approximately 193 l of petrol. Such EOL phase emission is not often considered when assessing the environmental impact of solar power generation, but as the volume of solar panel waste grows, the impact of such emission would reach a significant level in coming years.
Future technologies
Thanks to the advances made in the solar energy sector in the past two decades, the cost per kilowatt-hour of solar power generation is now comparable to the cheapest available fossil fuel-based power generation. The increased profitability of solar power means that government subsidy is no longer a requirement for large scale solar projects and will also open up more opportunities for subsidy-free solar projects. Moreover, the rise of hybrid PV systems based on integration of PV technology with other types of energy technologies, such as wind, hydropower, and battery, is likely to lead to further cost reductions through economies of scale.
Although solar energy has come a long way, more work is still needed to make solar power generation more sustainable, both socio-economically and environmentally. In particular, much work is still needed in relation to the EOL phase of solar panels to complete the sustainability cycle of PV power generation.
The problems relating to the EOL phase of solar panels, like other challenges the solar energy industry faced in the past, could be overcome by innovations. For example, developing recycling processes dedicated to PV panels could increase treatment capacities, improve the quality of extracted materials, and increase material recovery rate. More work can also be done to design solar panels to enable easy separation of panels parts or materials without having to use a shredder or thermal treatment. PV cell material research, which currently mainly focuses on improving cell efficiency, may also need to expand towards making cell materials more EOL-friendly, by making them more easily reusable or recyclable. Moreover, improved monitoring methods for analysing ‘gate-to-gate’ impact of EOL solar panels, considering all impacts from transporting solar panel waste to recycling plants up to sorting of the different recyclable materials and disposal of residues, may help identify which aspects of the EOL phase needs more urgent improvement. Such innovations directed to solar waste recycling may not be considered profitable initially, just like the PV cells in their early day. However, given the astronomical number of solar panels that will ‘die’ in the coming decades, solar waste recycling may, with the help of public policy, funding, and continued research, eventually be able to achieve economies of scale like the solar power generation sector did.
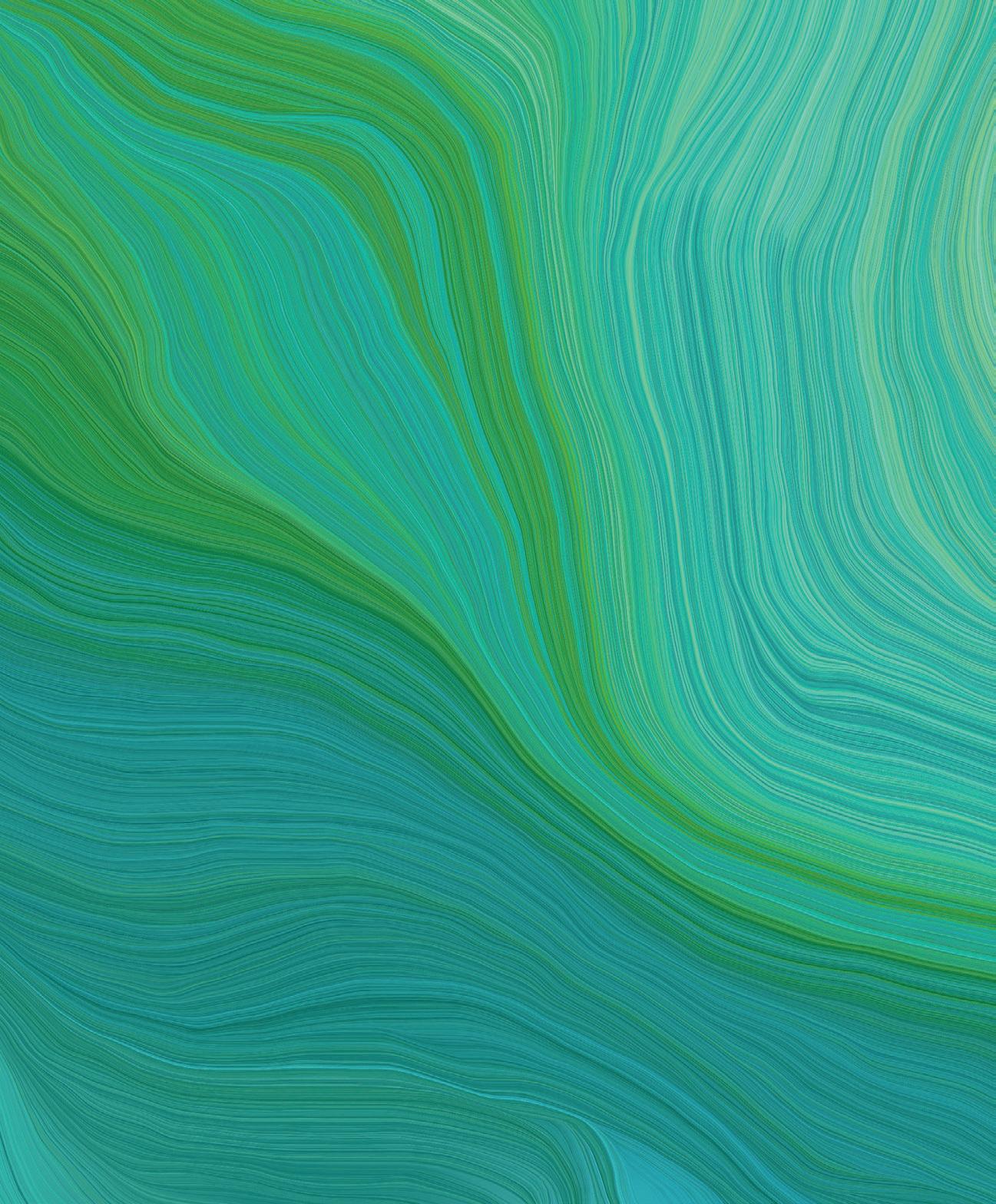
The increasing need for innovations in the non-cell parts of PV systems will be good news for new businesses that wish to step into solar power industry. Perhaps the rapid increase in the number of PV-related patent filings in recent years is not only a reflection of technological advances of PV cells, but also a sign of a wider range of innovations to come.
References
1. ‘Renewable capacity statistics 2021’, IRENA, (2021).
2. ‘Renewable Power Generation Costs in 2020’, IRENA, (2021).
3. LATUNUSSA, C. E. L., ARDENTE, F., BLENGINI, G. A., and MANCINI, L., ‘Life Cycle
Assessment of an innovative re-cycling process for crystalline silicon photovoltaic panels’, Solar Energy Materials and Solar Cells, Vol. 156, pp. 101 – 111, (2016).
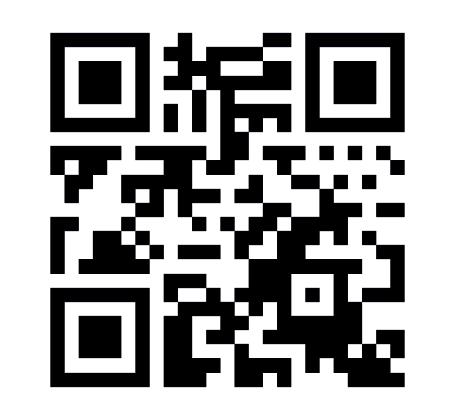
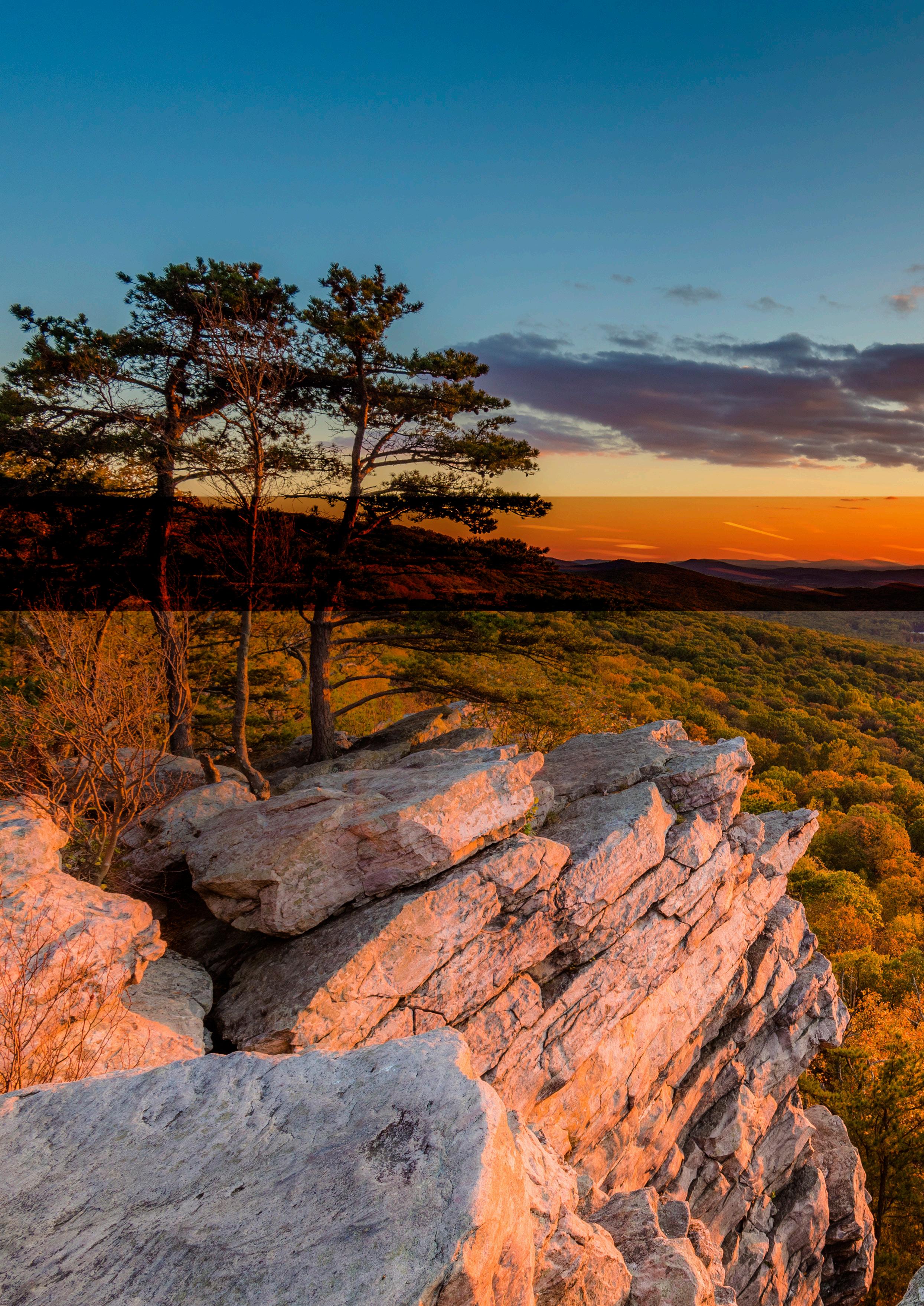
America can adapt to and
Clean energy advocates say to adopt photovoltaics, geothermal, hydropower, or wind. Others urge for the conversion of transport from fossil fuel to electricity or hydrogen fuel cells. Still, more seek forest restoration or no-till farming that locks carbon in the ground.
Each answer plays an important role, yet a landmark study by the climate science organisation, Project Drawdown, found the most impactful solution to reducing greenhouse gas emissions is to reduce food waste.1
Slashing food waste eases pressure on habitats, reduces toxic runoff, and avoids up to 102 billion t of emissions.2 That is equivalent to removing 30 000 coal plants or adding 30 million wind turbines. Each week, dump trucks load 1 million t of food waste and haul it off to be incinerated, where it is combusted and emitted as an environmental pollutant,3 or taken to a landfill,
where it decomposes and releases methane, a harmful greenhouse gas.
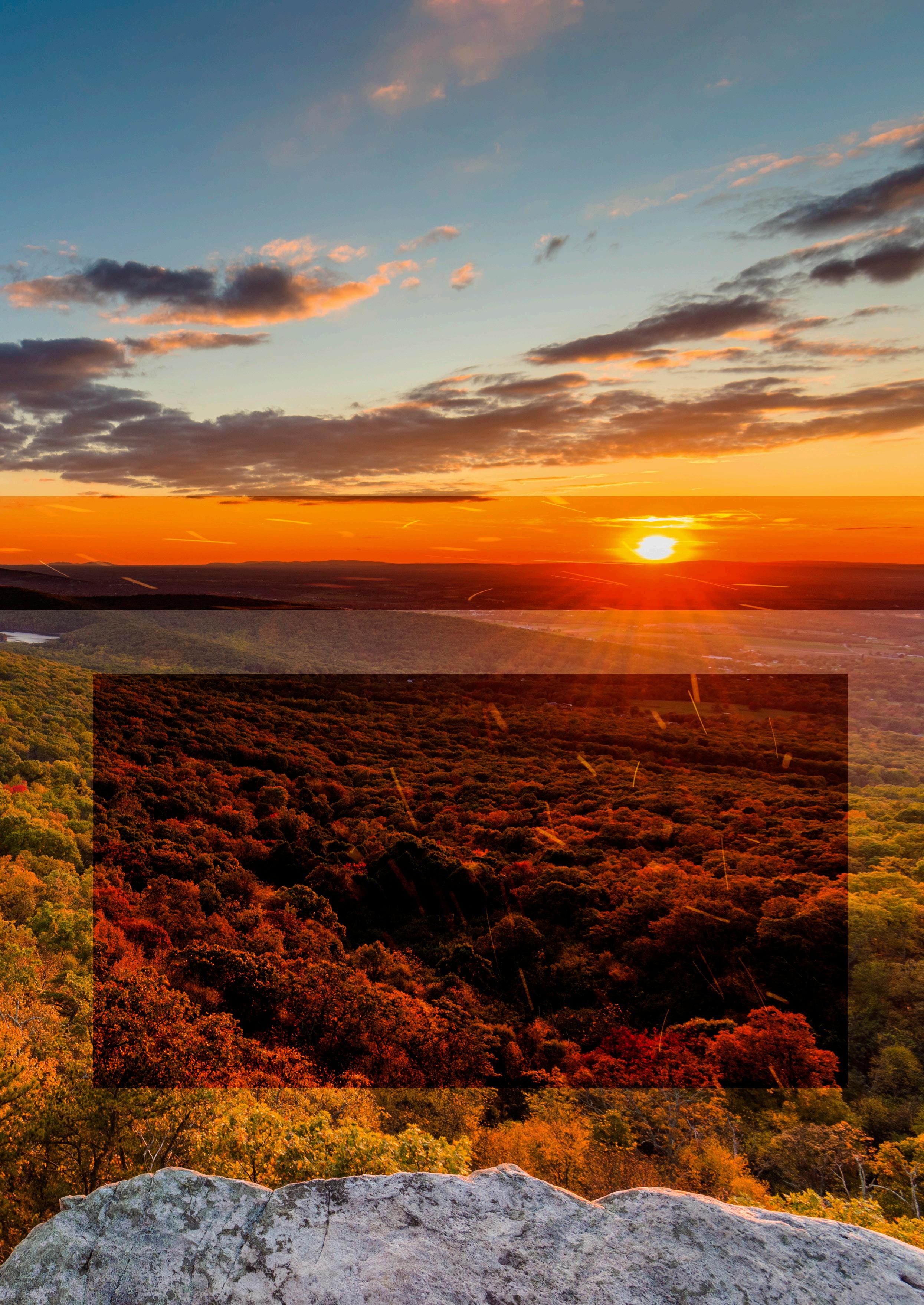
Bagged, spread, or buried in pockets without sunlight or oxygen, organic waste gets broken down by microorganisms through a natural, four-stage process called anaerobic digestion (AD). In a landfill setting, this process releases methane into the atmosphere, compounding negative environmental effects that may have been generated through the food supply chain.
But this natural process of AD can be harnessed, and the biogas it generates can be used as a source of clean, renewable energy to provide heat and electricity to homes or alternative fuels for vehicles.4
The use of anaerobic digestion
AD is both natural and timeless. For billions of years, microbes have broken down dead organic matter into rich muck and swamp gas. But in the past few centuries,
scientists have explored the biochemical processes of AD, and innovators have found ways to utilise its outputs.
Starting in the industrial age, entrepreneurs designed special tanks and systems – anaerobic digesters – that generate methane to fuel streetlights or power homes. Over time, these plants have grown more technologically complex, efficient, and sophisticated, unlocking enough renewable energy to offer a viable alternative to fossil fuels. In Europe and Asia, hundreds of thousands of plants have quite literally empowered cities and farms to manage waste in ways that are affordable, reliable, efficient, productive, odourless, and clean.
AD is a process through which microbes break down organic matter in the absence of oxygen. When this process takes place in a controlled, sealed vessel called a digester, organics (such as animal manure, food waste, grease, and oils) can be used for biogas production,4 utilising the naturally occurring methane for clean energy, rather than
being emitted into the environment. Additionally, this process generates digestate, which can then be used as a safe, nutrient-rich soil amendment, returning organic material back to the very ground from which it grew.
The North American Renaissance
The use of anaerobic digesters to generate renewable energy is a common practice around the globe. Now, North America is poised to catch up and potentially surpass other continents. Governments and industries seek to develop and integrate modular industrial anaerobic digesters that could divert the bulk of indiscriminate food disposal. Rather than bury or incinerate it to pollute our air, this could transform food waste into beneficial outcomes, such as clean energy and soil enrichment that help grow more food.
Far from hypothetical, the North American eco-friendly AD Renaissance is already underway.
Instead of abandoning food to rot in landfills, regional alliances have begun to carefully and responsibly divert organic feedstock into and process it through enclosed AD waste processing and conversion systems. Today’s next-generation anaerobic digesters master every aspect of breaking down food waste: the content, density, consistency, volume, flow, moisture level, temperature, and microbial activity.
In goes discarded waste; out come precious resources. Because the system is sealed airtight, anaerobic digesters refine the generated biogas into clean energy, such as: renewable natural gas (RNG), electricity, or even green hydrogen. Fresh water can flow back into the basin, cleansed of any risks from harmful contaminants, pathogens, or chemicals. And the solid byproduct is a nutrient-rich soil amendment, digestate – much like compost.
Behind this 21st Century AD Renaissance is a convergence of economic, political, and regulatory forces.
Landfills near cities have grown prohibitively expensive to approve, build, and operate. Incineration plants have fallen out of favour due to their negative impacts on local air quality. Food growers, processors, and municipal waste agencies seek ways to reduce operating costs and carbon footprints. Federal agencies now welcome and want to scale up AD systems to reduce methane emissions, displace fossil fuels, recycle organic waste, and replace synthetic fertilizers with the macro and micronutrients already present in waste.
A growing demand
Looking ahead, the American Biogas Council asserts that the construction of 13 500 new AD projects would generate 23 000 long-term jobs, generate enough energy to power 7.5 million homes, and cut emissions equivalent to taking
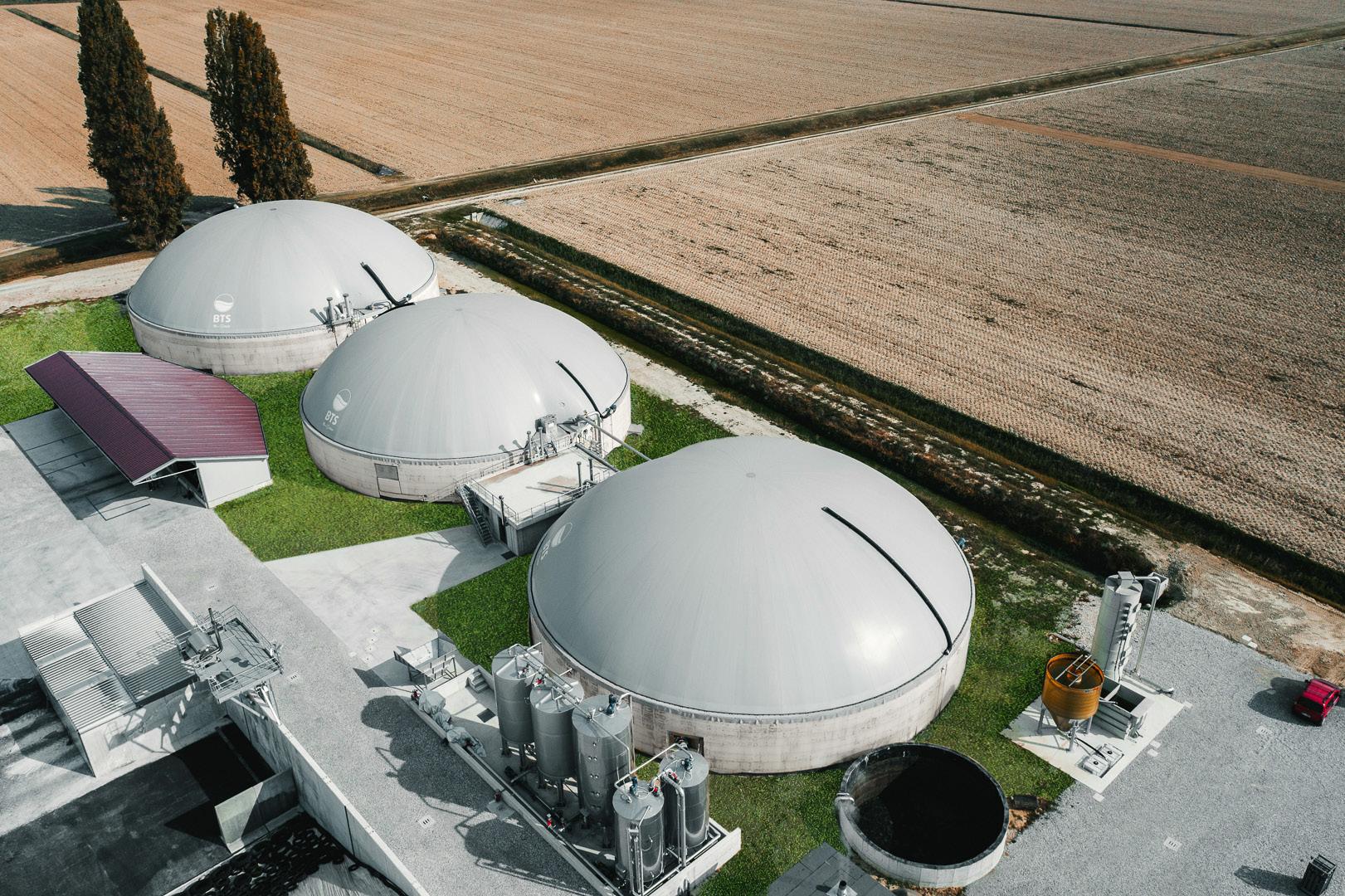
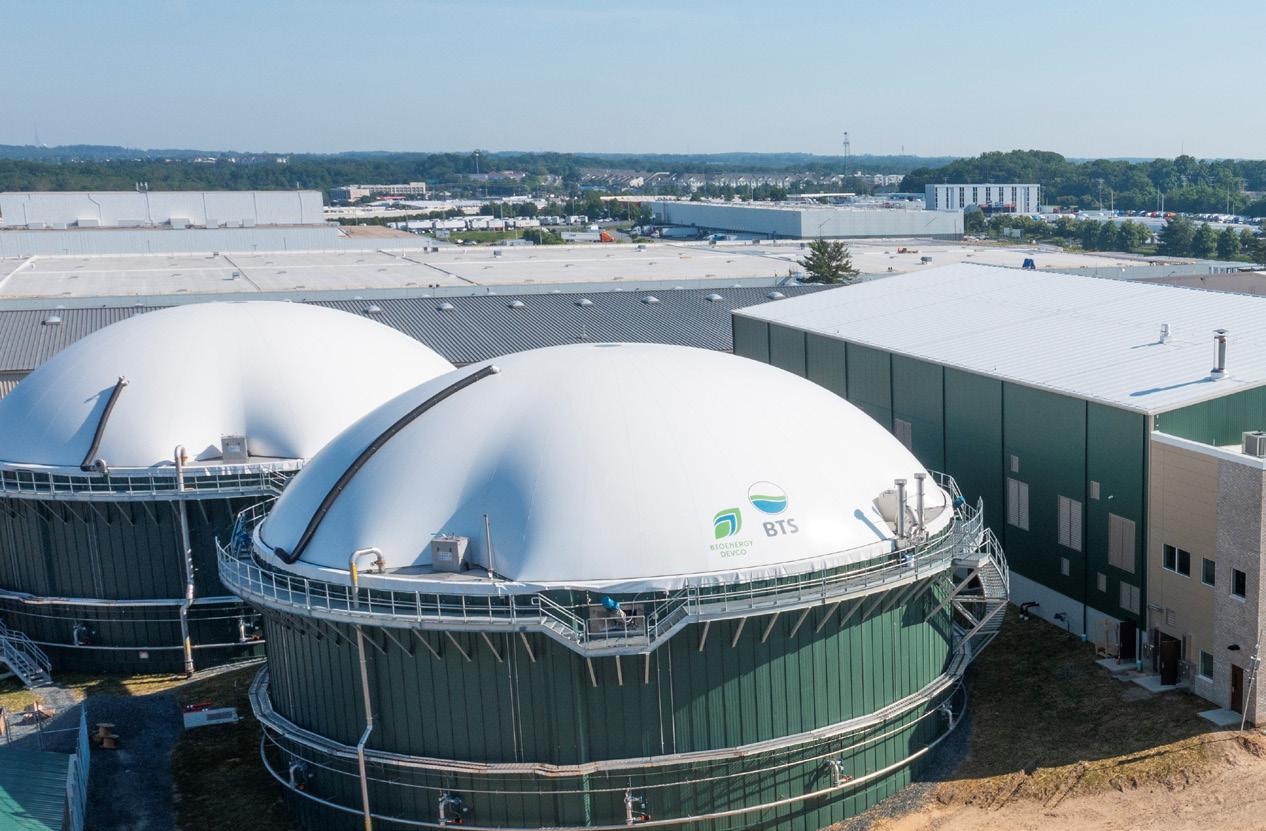
more than 15 million cars off the road. All these lead Acumen research to give North America the dominant share of a growing global market, driven by surging “demand for renewable energy sources, strict government guidelines to reduce greenhouse gas emissions, and expanding volume of solid waste.”5
To meet demand for AD systems in America, Bioenergy Devco has adopted what works so well everywhere else. It has adapted and innovated to meet the contours of local needs, economies, and demographics. Rather than focus only on just one aspect – funding, siting, building, operating, technology, or human resources – the company combines it all together in one seamlessly integrated package. It brings financing to the table; anticipates legal concerns and complies with waste legislation; and integrates food production problems, municipal constraints, and local community demands. Finally, based on stakeholder needs, the company builds to scale and links to the grid or pipeline, applying a seasoned team and proprietary technology to the equation.
Achieving results
Can a billion-year-old biological process really be all that complex? The simple answer is yes.
Running an efficient anaerobic digester is a proven and predictable science. The process requires exactly the right combination of ingredients, broken down to the right size and sequence. The proportions of water, microbes, and organic content (plants, cooking oil, protein, animal fats, etc.) must be precisely and constantly measured and adjusted, while monitoring temperature and gas production. For such a simple living organism, microbes can be astonishingly fussy about what they eat and under which conditions. Too cold or too hot, too dry or too solid, and the whole AD process can be disrupted. But the results are extraordinary on multiple levels.
Case study: Maryland, USA
Bioenergy Devco’s latest commercial scale bioenergy centre in Jessup, Maryland, the US, is designed to ingest 115 000 t of excess waste food scraps, fats, oils, and greases (FOG), along with other organic material. The centre can produce more than 300 000 million Btu of RNG. Injected into the grid, the RNG reduces carbon dioxide (CO2), equivalent to taking more than 12 500 gasoline-powered cars off the road for a year. The fully-enclosed anaerobic digesters eliminate the groundwater pollution often caused by excessive land application and landfill operations, minimising runoff that can poison ecosystems and cause significant human health problems, meaning the facility does not require fresh water to process materials. The resulting greenhouse gasses prevented from being released into the atmosphere have the equivalent environmental impact of approximately 70 000 acres of US forests in one year – 82 times the size of Central Park.
A useful byproduct
These green outcomes are in and of themselves remarkable. But there are other local benefits to consider, from reducing
transport costs to preventing algae blooms and toxic runoff. Additionally, AD yields another byproduct prized by gardeners and farmers alike: digestate.
Digestate, much like compost, is an organic conditioner that traps CO2 as organic matter, feeds the soil microbiome, and returns and cycles waste back into the landscape, reducing the use of chemical, petroleum-based amendments. Like composting, the digestate output from anaerobic digesters optimises the conditions for naturally occurring microbes (i.e., protozoa, microbes, rotifers, fungi) to continue their magic, improving the tilth, life, health, and fertility in soils. Moreover, digestate has even higher concentrations of nutrients than compost, enhancing food production in a closed-loop process that captures the essence of our shift toward a circular economy. The company’s Maryland plant produces more than 16 t of rich, fertile soil amendment for agricultural and other land use.
Conclusion
Anaerobic digester systems hold tremendous potential for renewable energy solutions. Yet one of the more novel and exciting prospects is adapting the process to produce hydrogen.
With just one proton and one electron, hydrogen is Earth’s simplest and most abundant element. While it can store and deliver usable energy, hydrogen rarely exists by itself. It must be extracted from other compounds produced from hydrocarbons in emissions-intensive processes that can defeat the purpose. Anaerobic digesters, by contrast, could be adapted to produce green hydrogen using a proven steam upgrading process.
That massive promise lies in hydrogen’s combination of efficiency and versatility of applications. Hydrogen is so clean that, when burned, it produces only water and heat. The energy can be used by trucks, shipping, buses, auxiliary power units, and even aircraft.
Every technological innovation – from electric cars to the Internet – has to overcome initial resistance. The quest to change the world by recycling food waste into clean energy is no different. It can only happen through the convergence of public support, consumer demand, government backing, and private innovation. Once widely embraced, it crosses a tipping point, pushes back old constraints, and opens new directions and possibilities.
In this way, anaerobic digester systems in North America are poised for a 21st Century Renaissance, a transformation that unlocks greener energy, cleaner air, purer water, and richer soils.
References
1. ‘Table of solutions’, Project Drawdown, https://drawdown.org/solutions/ table-of-solutions
2. ‘Reduced food waste’, Project Drawdown, https://drawdown.org/solutions/reducedfood-waste
3. ‘The impact of food waste’, U.S. Department of Agriculture www.usda.gov/foodlossandwaste
4. ‘How Does Anaerobic Digestion Work?’, U.S. Environmental Protection Agency, (2022), www.epa.gov/agstar/how-does-anaerobic-digestion-work
5. ‘Anaerobic Digestion Market – Global Industry Size, Share, Anaylsis, Trends and Forecast 2022 – 2030’, Acumen Research and Consulting, (July 2022), www.acumenresearchandconsulting.com/anaerobic-digestion-market
GLOBAL NEWS
RWE acquires Western Power Offshore Developments
RWE has acquired 100% of Irish company, Western Power Offshore Developments Ltd, which is in the early stages of developing the East Celtic wind farm, located approximately 10 km off the coast of Wexford and Waterford in Ireland. The project will significantly expand RWE’s offshore wind developments in the country and contributes towards its target of expanding its green generation fleet internationally up to 50 GW by 2030.
RWE’s adoption of the project as part of the acquisition reinforces the company’s commitment to offshore wind growth in Ireland, and makes it a key partner to the Irish government in the delivery of its target of connecting 7 GW (7000 MW) of offshore wind capacity to the grid by 2030.
In addition, RWE is already developing the Dublin Array wind farm project in partnership with Saorgus Energy, off the coast of Dublin and Wicklow, which will be capable of generating up to 600 – 900 MW of electricity.
The East Celtic wind farm project is in the very early stages of development, and important decisions have yet to be made, from the overall size of the wind farm to turbine locations, cable routes, and land-based developments. A major public consultation exercise will take place to gather views from local people to help inform the future direction of the project design.
Depending on the final agreed installed capacity, and once fully developed and constructed, it could have the potential to generate up to 900 MW of clean wind energy. It is anticipated that it could also bring significant economic benefits to the South East region of Ireland, during both the construction phase, and across the operational life of the wind farm.
Sumitomo announces participation in onshore wind IPP project
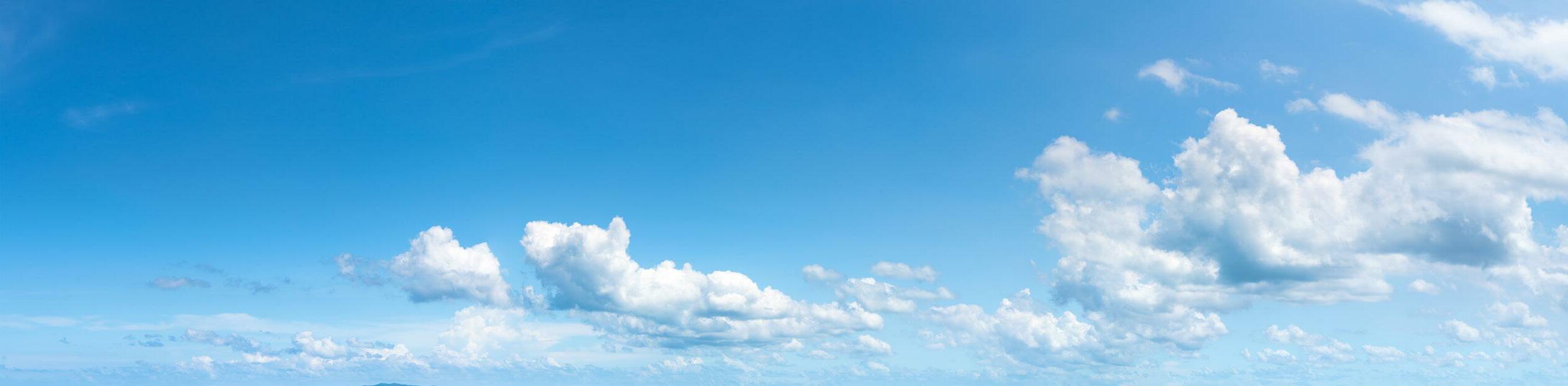
Sumitomo Corporation has acquired an equity stake in Amunet Wind Power Company S.A.E. and signed a loan agreement with a group of banks, including Japan Bank for International Cooperation (JBIC), in relation to its onshore wind independent power producer (IPP) project in Egypt, which Sumitomo Corporation has been developing in partnership with AMEA Power.
The project involves the construction, ownership, and operation of onshore wind farm that can generate approximately 500 MW of electricity, in Ras Ghareb in the Red Sea Governorate along the Gulf of Suez, situated 240 km southeast of Cairo, the capital city of Egypt. When completed, the project is slated to sell electricity to Egypt’s state-owned Egyptian Electricity Transmission Company over approximately 25 years. The construction is scheduled to take approximately 2.5 years until 2025, and the completed facility is expected to become one of the largest onshore wind farms in Egypt. Sumitomo Corporation will engage in the development, construction, and operation of the wind farm by leveraging its expertise acquired through its track record of overseas onshore wind farm projects in the US, the Republic of South Africa, the People’s Republic of China, and other locations.
The project is to be co-financed by JBIC, International Finance Corporation, Sumitomo Mitsui Banking Corporation, Sumitomo Mitsui Trust Bank, Ltd, Standard Chartered Bank, and others through a project financing scheme, for which an agreement-signing ceremony took place on 30 November 2022.
Vestas wins 756 MW order for wind project in Australia
In partnership with renewable energy enterprise, TagEnergy, Vestas will deliver the EPC for the first stage of Golden Plains wind farm, a 756 MW project in Victoria, Australia. Stage one is set to be Vestas’ largest onshore wind farm in Asia Pacific and will feature 122 V162-6.2 MW wind turbines from the EnVentus platform.
Upon completion, Vestas will also deliver a 30-year service and maintenance agreement (AOM5000) to optimise the project’s energy production and long-term business case certainty.
With the highest market share for installed capacity, Vestas currently maintains the most amount of wind turbines in Australia. This project will further expand the company’s leading footprint in the country’s promising clean energy market.
Delivery of Vestas’ wind turbines is expected to occur 4Q23, with commissioning to commence 4Q24. Once operational, the first stage of Golden Plains wind farm will generate enough clean energy to power approximately 450 000 homes a year.
GLOBAL NEWS
Sin Mao Group and Wello to deploy 12 MW wave energy park in Taiwan
Wello and Sin Mao Group have signed a contract to deploy 12 MW of wave energy in Taiwan – the first wave energy power generation project in the country. Furthermore, the two companies have signed an agreement for Sin Mao Group to act as a distributor of Wello’s technology in Taiwan. This collaboration is expected to become the largest offshore power generation developer in Asia in the coming future.
Wello is a leading wave energy technology provider, presently working on several sites with their customers and partners worldwide. Wello’s unique wave energy technology is based on a gyrating asymmetric floating device with the rotational power conversion unit safely constructed inside the steel hull. Wello is co-operating and partnering with established strong offshore technology providers to be able to respond efficiently to the rapidly growing demand at the global market.
Taiwan is aiming to become carbon neutral by 2035. Presently, with 95% of energy being generated by fossil fuels, this is an ambitious goal and requires a totally new energy infrastructure being constructed. All sources of renewables must be taken in use, with several wave energy sites currently being considered and under development in Taiwan.
Wello will provide the technology and the expertise that they have developed during over a decade in wave energy, and Sin Mao Group will be responsible for developing the sites and providing wave energy converters based on Wello’s technology for other site developers.
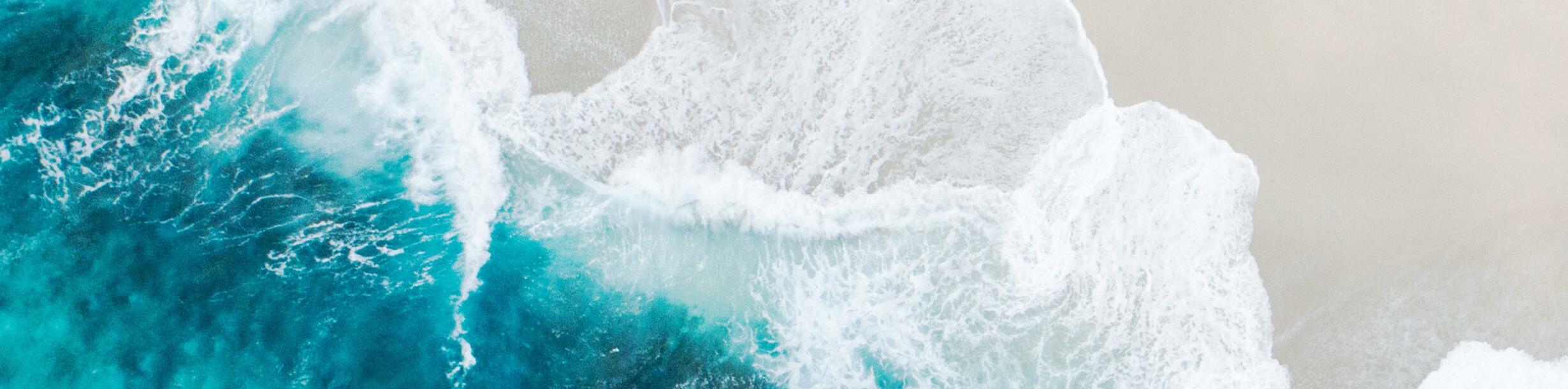
Diary dates
Energy Storage North America |
Intersolar North America 14 – 16 February 2023
California, USA www.esnaexpo.com/
WindEurope 25 – 27 April 2023
Copenhagn, Denmark https://windeurope.org/annual2023/
Global Energy Transition 07 – 08 June 2023
New York, USA
https://events.reutersevents.com/energy-transition/ global-energy-transition-new-york
Minesto signs collaboration agreements for project development in Indonesia
Minesto has signed two collaboration agreements for development in the Nusa Tengara Barat (NTB) region, Indonesia: a memorandum of understanding between Minesto and the regional Eco Regions Indonesia, and a letter of intent between Minesto and the region.
The initiative in Indonesia is part of Minesto’s broader market establishment in Southeast Asia. The NTB region has ambitious sustainability goals and is a forerunner in Indonesia’s energy transition. The regional commitment and availability of ocean currents make NTB a favourable entry market for Minesto in Indonesia.
The collaboration agreements include feasibility studies on the natural resource, infrastructure, and finance, and are based on collaborative work to integrate Minesto’s technology as a part of the 100% renewable energy mix in the region, starting with the special economic zone.
Minesto is a new member of the bilateral programme SISP Energy Alliance, and the collaboration agreements signed is a first step towards build out of several key interest areas in Indonesia. As the fourth largest country by population and one of the largest ocean territories in the world, Indonesia faces a true challenge in the transition from fossils to affordable and suitable renewables.
Global Energy Show
13 – 15 June 2023
Calgary, Canada www.globalenergyshow.com/
Global Offshore Wind 14 – 15 June 2023
London, UK https://events.renewableuk.com/gow23
Offshore Wind North East 08 – 09 November 2023 UK www.nof.co.uk/events/owne-2023/
GLOBAL NEWS
OPAL Fuels, NextEra Energy Marketing, and Republic Services begin operations at Minnesota RNG facility
OPAL Fuels Inc., a leading vertically integrated producer and distributor of renewable natural gas (RNG), and NextEra Energy Marketing, LLC, a subsidiary of NextEra Energy Resources, LLC, has announced the commencement of commercial operations at the first landfill RNG production facility in Minnesota, the US. The Pine Bend RNG project is located at a landfill owned by Republic Services, Inc., a leader in the environmental services industry, and interconnected with a pipeline owned by Xcel Energy, an electric and gas utility in the region.
The new RNG facility captures naturally occurring biogas, made up in part by methane, from Pine Bend Landfill and transforms it into RNG.
This project replaces a landfill gas-to-electricity project, which has now been decommissioned. At full capacity, the facility is expected to process an estimated 3350 ft3/min. of landfill gas, resulting in the production of 6.3 million gasoline gallon equivalent per year of RNG. The low-carbon gas is then injected into Xcel Energy’s gas pipeline.
Shell to acquire Nature Energy
Shell Petroleum NV, a wholly owned subsidiary of Shell plc, has reached an agreement with Davidson Kempner Capital Management LP, Pioneer Point Partners, and Sampension to acquire 100% shareholding of Nature Energy Biogas A/S for nearly US$2 billion (€1.9 billion). The acquisition will be absorbed within Shell’s current capital range, which remains unchanged.
Nature Energy is a producer of renewable natural gas (RNG) from agricultural, industrial, and household wastes. By purchasing the shares in Nature Energy, Shell will acquire the largest RNG producer in Europe, its portfolio of cash generative operating plants, associated feedstock supply and infrastructure, its pipeline of growth projects, and its in-house expertise in the design, construction, and operation of innovative and differentiated RNG plant technology.
The transaction is subject to regulatory approvals and is expected to close in 1Q23. Nature Energy is cash generative, and the acquisition is expected to be both accretive to Shell’s earnings from completion and deliver double digit returns.
Carbon Negative Biofuels from Organic Waste (CARBIOW) is a Research and Innovation Action funded by the EU under the Horizon Europe Programme that addresses green transition and circular economy by proposing novel technologies that cover the whole process of conversion of organic waste to biofuels. The 42-month project held its kick-off meeting virtually on 3 November 2022, and officially started to work together in the planning of the work to be done in the next months.
The goal of CARBIOW is to establish an efficient and scalable process to convert the Organic Fraction of Municipal Solid Waste (OFMSW) and other hard-to-utilise solid organic wastes to biofuels with key targets, such as establishing a new pre-treatment process of OFMSW where a cleaner, denser, carbon-rich, dry, and homogenous solid biofuel is produced and utilising pure oxygen (nitrogen-free gas) in combustion and gasification to produce clean syngas.
The consortium is composed of 12 partners spread around Europe, including companies from the Netherlands, Sweden, Slovenia, Norway, Finland, Germany, Belgium, and Spain.
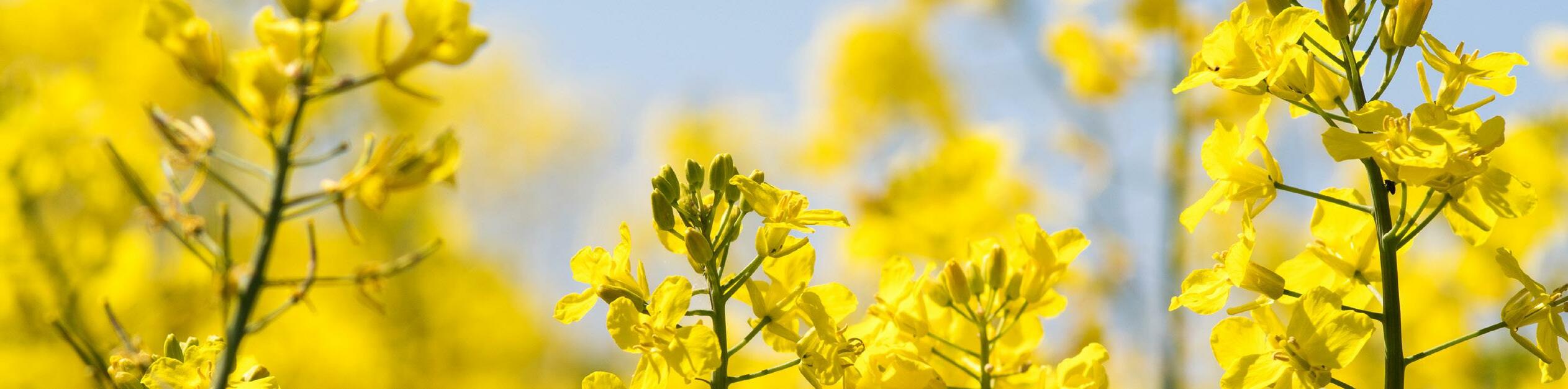
CARBIOW project to help Europe take the lead in energy and transport sector decarbonisation
Renewable
storage venture
Renewable Power Capital (RPC) has announced its entry into the battery storage market in Great Britain, working with Eelpower to acquire, build, and operate utility scale projects.
The venture will target up to 1 GW of storage, with a near-term pipeline of 240 MW.
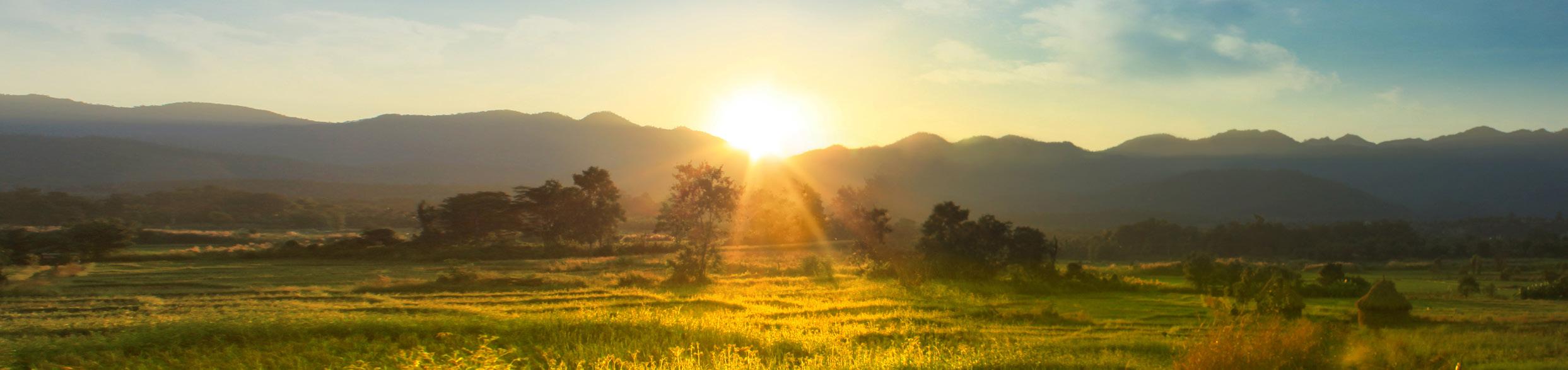
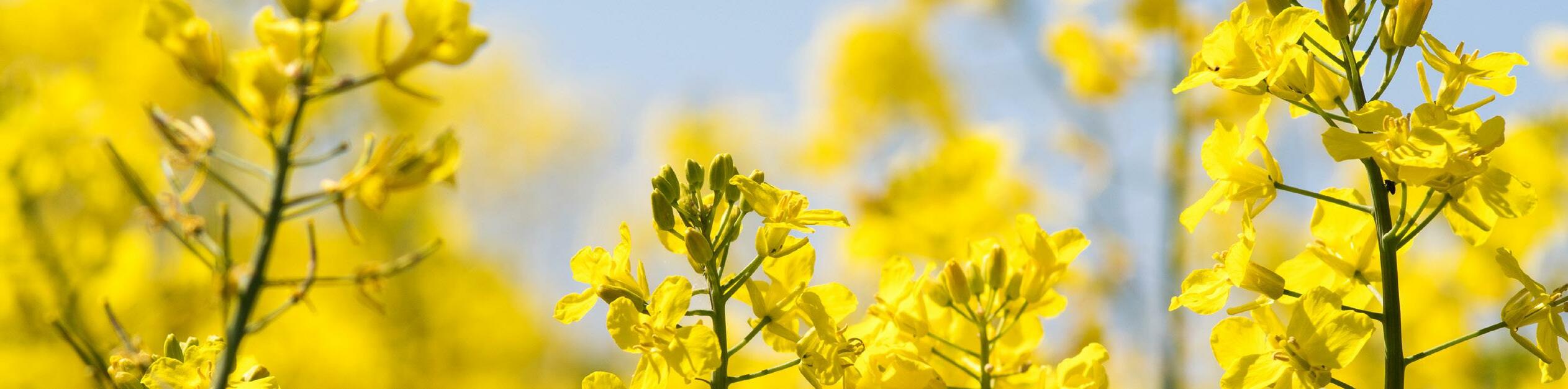
The new RPC-Eelpower joint venture will participate in wholesale electricity markets and provide ancillary services to National Grid, making an expanded contribution to the Great Britain electricity system. Battery storage projects are unsubsidised, underscoring RPC’s approach to investing in projects without government subsidy as a long-term project owner. The joint venture cements RPC’s position as a key investor in the fast-growing storage sector.
This storage debut is also the first investment by RPC in the UK and follows RPC’s recent acquisition of a 528 MW ready-to-build onshore wind portfolio in Sweden; a deal which was executed on an unlevered and unhedged basis. Late in 2021, RPC also secured 10-year power purchase agreements for three Finnish onshore wind projects that are expected to begin operation within the next two months.
Waratah Super Battery starts execution in Australia
Powin LLC will deliver a 1.9 GWh battery energy storage system (BESS) for Akaysha Energy, a BlackRock company, to power the New South Wales (NSW) Waratah Super Battery (WSB) project. Akaysha Energy has been appointed by the Energy Corporation of NSW to develop the WSB to provide a service of at least 700 MW capacity as part of a system integrity protection scheme (SIPS). Powin will supply 2592 CentipedeTM Energy Segments and 288 power conversion systems from their wholly owned subsidiary, EKS Energy, for a total project capacity of 909 MW/1915 MWh. Powin will also provide a 20-year long-term service agreement, which will enhance the reliability, efficiency, and availability of power supplied by the mega battery.
This flagship SIPS project will unlock latent transfer capacity in the existing transmission system, help integrate renewable energy, and maintain grid reliability by acting as a ‘shock absorber’ if disruptions such as lightning strikes or bushfires interrupt the flow of electricity.
The WSB is proposed to be located 100 km north of Sydney at the former 1400 MW Munmorah coal-fired power station. Pending approval, construction will begin in 2023 and is expected to be completed by mid-2025.
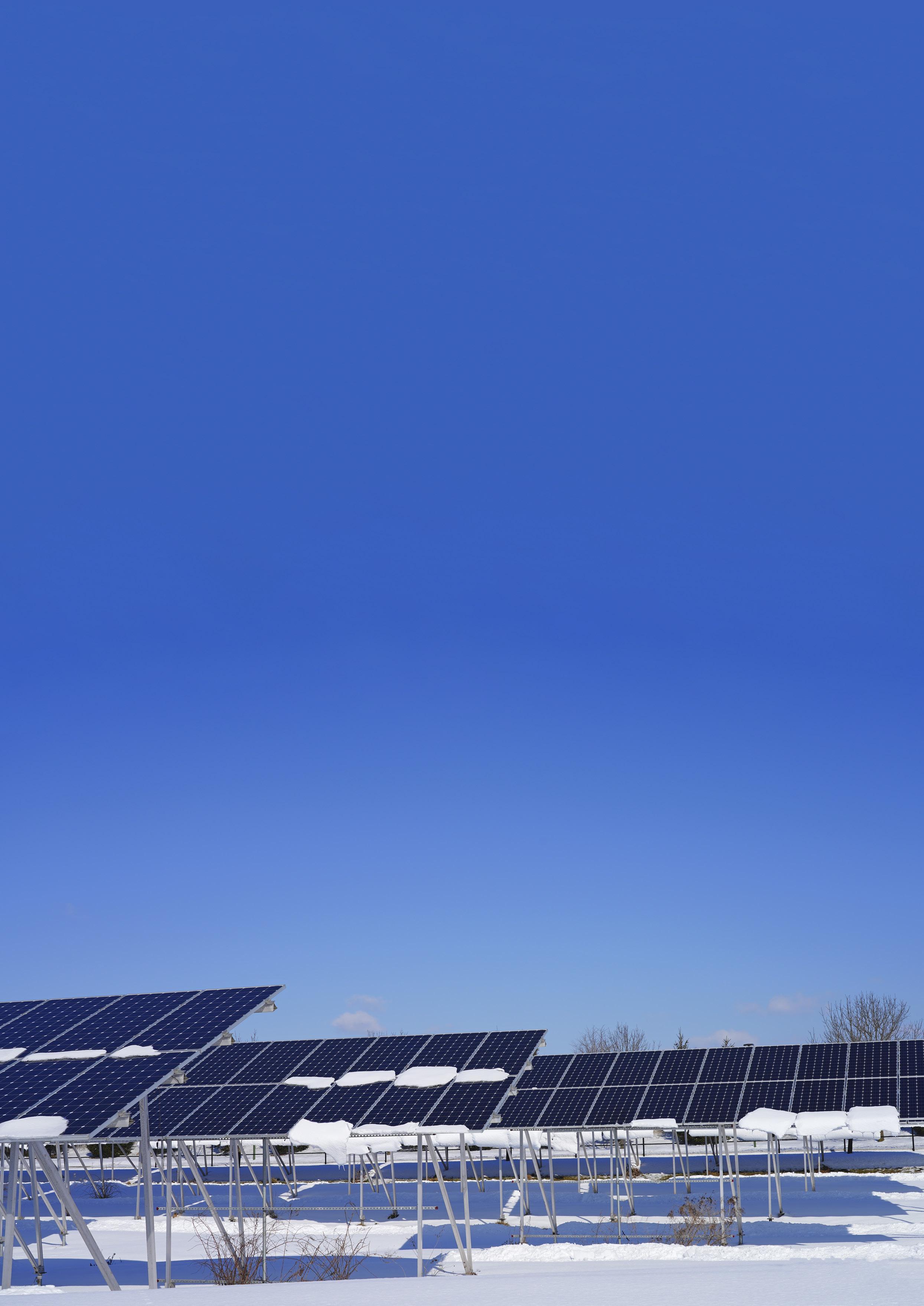