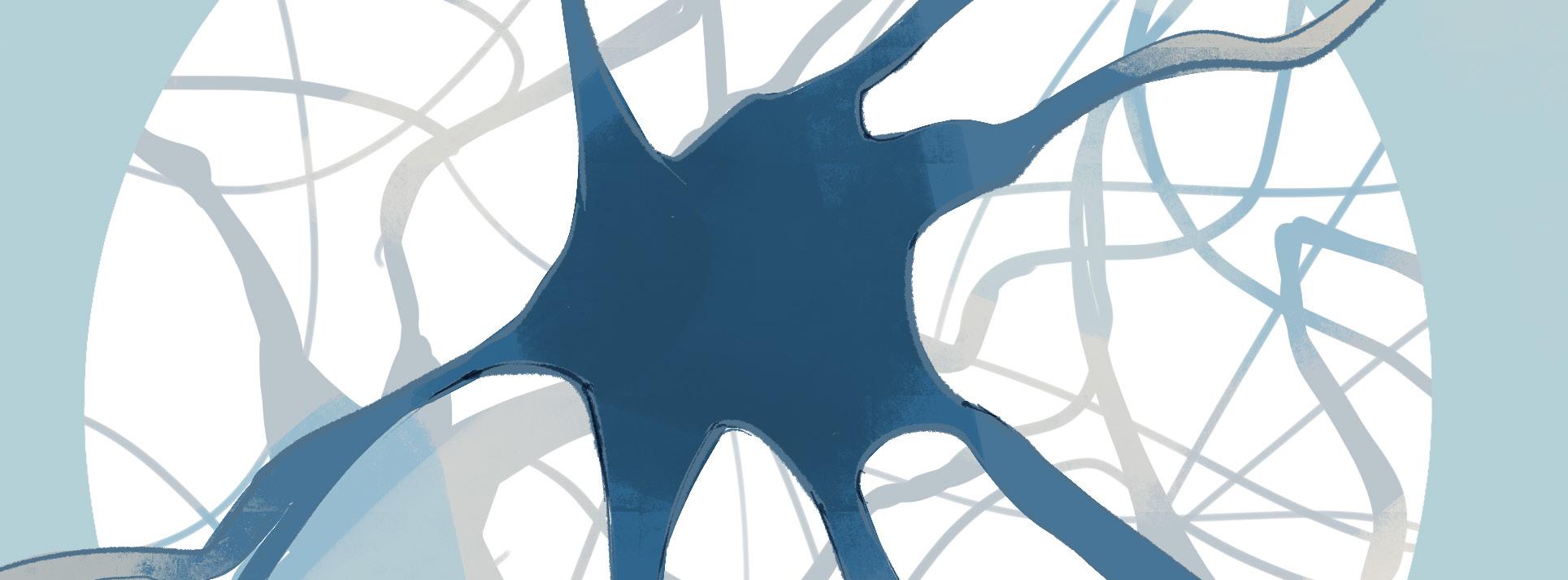
32 minute read
Mechanims of Satellite Glial Cells in Chronic Pain Genesis and Maintenance
from PCR - Fall 2022
Mechanisms of Satellite Glial Cells in Chronic Pain Genesis and Maintenance: A Novel Method for Analgesic Research
by Charles Jiang (VI) Abstract
Chronic pain is a common condition that involves hypersensitivity of neurons. Many treatments currently target the neurons of the nervous system but often result in adequate responses. Located in the peripheral nervous system, satellite glial cells (SGCs) are a type of glial cell that closely envelope the cell bodies of neurons in the sensory ganglia. SGCs have been recently proven to play a direct role in the pathogenesis of pain. Because of their location early in the pain sensation pathway and their close relationship with neurons, they are a possible therapeutic target for chronic pain. The activation cascade of SGCs includes ATP, hypersensitivity of purinergic receptors, release of substance P and cytokine, changes in expression of ion channels, increased gap junction coupling, and a sustained calcium wave. There is evidence that these changes caused by SGCs contribute to chronic pain by altering neuronal activity. In particular, purinergic receptor P2X7R plays a major role in this cascade, promoting further ATP release, increasing ATP sensitivity, and contributing to a pro-inflammatory feedback loop. In this paper, we propose an experiment to block P2X7R with A-740003 as a treatment for chronic pain. Two tests would be conducted on rats. The first test would confirm P2X7R activation, A-740003 blockage, and downstream effects in vitro. The second test would determine the effect of blockage of P2X7R with A-740003 in animals exhibiting hyperalgesia in vivo. Hypothetical results suggest confirmation of the significant role of P2X7R in SGC activation, that A-740003 inhibits this role, and that A-740003 successfully returned animals with hyperalgesia to normal levels of nociception.
Pain perception, nociception, is the process by which noxious stimuli is relayed from the site of stimulation to the central nervous system (CNS), which includes the brain and spinal cord. Different from normal perception where somatic receptors are activated in response to non-painful stimuli, nociception involves different neural pathways (Freudenrich, 2007). Neurons called nociceptors are located in the peripheral nervous system (PNS) and are activated in response to painful stimuli (Freudenrich, 2007). Generally, there are three steps in the pain process: reception of stimuli, transmission, and pain center reception (Yam et al., 2018). Nociceptors are activated after sensing the stimulus. Nociceptors then transmit the pain signal from the PNS to the CNS. Finally, the brain processes the information and responds accordingly. This paper focuses on a novel perspective on treating pathogenesis of the pain sensation pathway, specifically chronic pain, by focusing on the PNS. Sensory ganglia are clusters of nerve cell bodies located in the PNS that have a bifurcating axon. One end goes to the periphery, and the other goes to the spinal cord. The three main types of sensory ganglia include the trigeminal ganglia (TG), which innervates the head, face, and teeth; the nodose ganglia (NG), which innervates most of the visceral organs; and the dorsal root ganglia (DRG), which innervates the rest of the body (Hanani, 2015). Shown in Fig. 1 is the innervation of the GI tract, involving the NG and the DRG; one end of each ganglion goes toward the periphery (i.e. the GI tract) and the other goes toward the spinal cord. Chronic pain usually originates from misfiring nociceptors in sensory ganglia, making it a viable target for pain treatment. Pain serves a protective role as the warning system for the body to react to any possible threats, but it can also develop into pathological pain which is nonprotective and maladaptive (“Classification of Chronic Pain”). Chronic pain is long-lasting pain that is usually caused by an initial injury but continues even after the underlying injury is resolved. Examples of chronic pain include arthritis (joint pain), back pain, neck pain, migraines, etc. As a result, chronic pain interferes with daily activities, affecting mobility and decreasing the general quality of life. It can become so debilitating that psychological problems like depression could arise.
Chronic pain is believed to occur after the nerves become damaged, triggering a process called central sensitization where nociceptors become hypersensitive to stimuli, firing off when they are not supposed to (“Classification of Chronic Pain”). Chronic pain can be classified as nociceptive or neuropathic (“Different Types of Chronic Pain,” 2020). While the differences are not important in this paper, their differentiation lies in the origin of the injury. Nociceptive pain develops in response to a specific stimulus to the body (e.g. fractures) that ultimately leads to long-term changes in the nervous system. Neuropathic pain occurs when the nervous system directly sustains damage, resulting in long-term
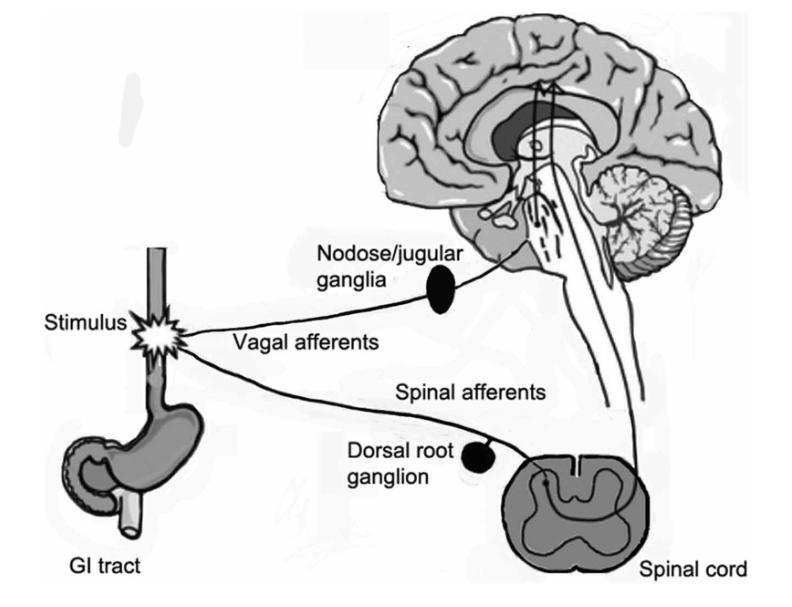
Figure 1. A diagram (Hanani, 2015) showing the sensory innervation of the GI tract
malfunctions in the nociceptors. Both types of chronic pain occur after central sensitization. Chronic pain therapies are complicated and often result in inadequate responses or side effects, so it is essential to improve our understanding of the mechanisms and cells involved in the generation and maintenance of chronic pain (Gazerani, 2021). Pain therapies historically target the neurons themselves that transmit pain signals (Jasmin et al., 2010). However, there is increasing attention on the role of glial cells in pain modulation. Glial cells are part of both the CNS and PNS but do not directly participate in electrical signaling, a role associated specifically with neurons. Rather, they provide a myriad of supportive functions including modulation of the rate of nerve signal propagation, control of neural response to neurotransmitters, and structure for parts of neural development (Purves et al., 2001)). Recently, central glia, especially astrocytes and microglia, have also been identified to participate in the response to neural injury. Even though PNS is where pathogenesis of pain sensation originates, the roles of peripheral glia in pain modulation are significantly less researched and understood (Gazerani, 2021). However, recent evidence shows that activation of satellite glial cells (SGCs) contributes to chronic pain through augmentation of neural activity (Hanani & Spray, 2020). SGCs are poorly understood glia that are exclusively located in the PNS where they envelope ganglionic neurons, including neurons of the DRG, TG, and NG. As a result, their processes are closely tied to the neuron they surround. Considering SGCs are located earlier in the pain pathway than central glial cells and close relationship with sensory ganglia, understanding and targeting their role in pain has the potential for decreasing the likelihood of pain chronification and for hindering the transition from peripheral to central sensitization. Therefore, SGCs and their mechanism in the pain pathway are a promising field of study for pain research and can be a valuable target for therapeutic drugs.
II. LITERATURE REVIEW
A. Satellite Glial Cells
SGCs are mononuclear cells found in the peripheral nervous system. Morphologically, SGCs are similar to Schwann cells in that they wrap around the neuronal cell body, commonly forming a sheath. Typically, a single sheath is made up of multiple SGCs connected together as seen in Fig. 2 and the top diagram of Fig. 3 (Hanani 2020). In neuronal groupings, cell bodies and their sheaths are commonly barely separated by connective tissue space. A small proportion of DRG neurons have been found to share an SGC envelope, forming a cluster with multiple neurons. Neurons inside of a cluster either have a single SGC separating them or have a thin layer of extracellular space as seen in the bottom two diagrams of Fig. 3 (Hanani 2020). SGCs typically maintain a 20 nm gap between themselves and the neuron which often projects into the SGC sheath (Hanani 2020). This closely tied structure allows for bidirectional and complex neuron-SGC and SGC-SGC interactions.
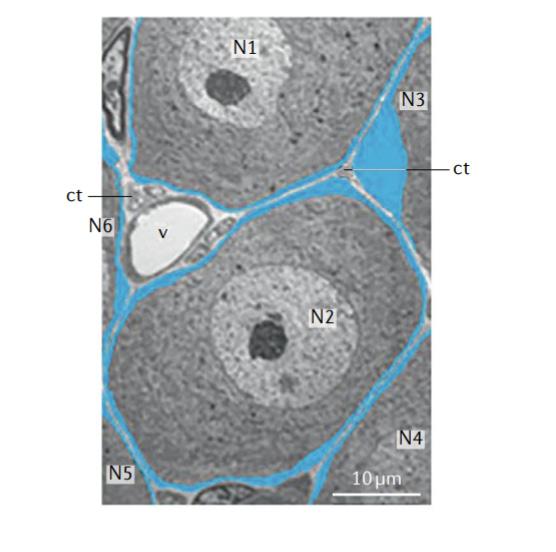
Figure 2. Low-power electron microscope showing SGCs (blue) and neurons (labeled N1-N6). Ct stands for connective tissue, and v stands for blood vessels. (Hanani & Spray, 2020)
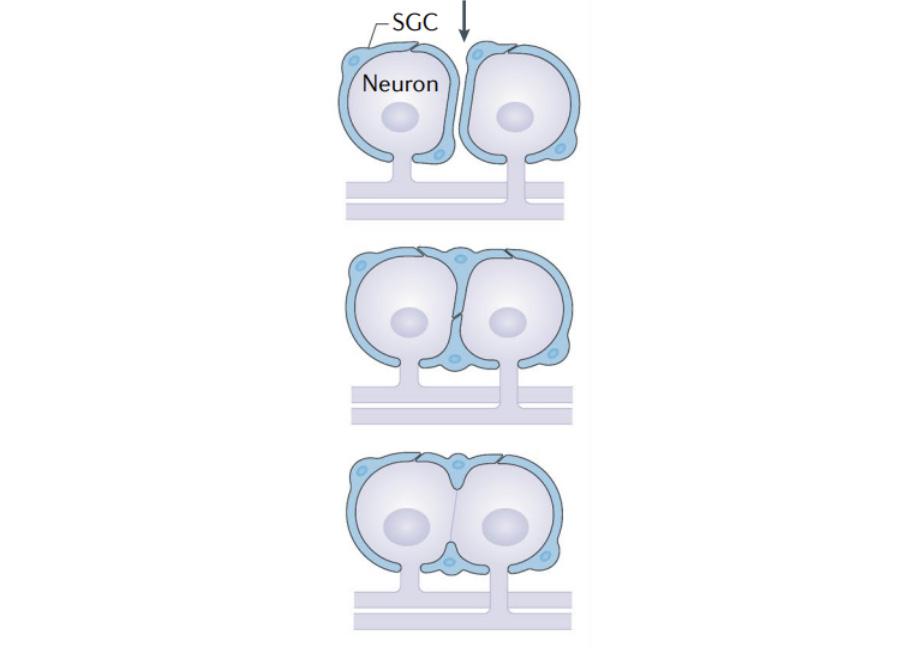
Figure 3. Diagram showing three different ways SGC-neuron clusters can form (Hanani & Spray, 2020)
SGCs are physiologically similar to the astrocytes of the CNS, carrying out functions such as ion concentration regulation in extracellular space and neurotransmitter recycling. To communicate with other SGCs, neurons, and other bodies in extracellular space, SGCs also express numerous receptors and signaling factors, some of which are believed to mediate the generation and maintenance of chronic pain. The most notable include ATP, P2 purinergic receptors, calcitonin gene-related peptide (CGRP), substance P, glutamate, and cytokines. As a result, neuronal activity in sensory ganglia depends to a large degree on neuron-glia interactions, so understanding these interactions is highly valuable in understanding the development of chronic pain. B. SGC and Intercellular Communication
Originally believed to mainly play a passive role in the transmission of information from the periphery to the CNS, there is increasing evidence that SGCs have a much more active role. Neurons in sensory ganglia not only lack synaptic contact with each other, but they are also separated from each other by SGCs and extracellular space, so there should be no interactions between the neurons (Shinder & Devor, 1994). On the other hand, neurotransmitter receptors have been found to be present in the cell bodies of sensory ganglia neurons. A calcium imaging study on DRG neurons in live mice found that the excitation of one DRG neuron sometimes led to the excitation of neighboring neurons. Called cross-excitation, this effect was found to occur more often after nerve injury and was associated with a calcium-dependent release of chemicals and neurotransmitters that alter the physiological characteristics of the neuron, resulting in changes in the excitability of nociceptive neurons. The fact that SGCs completely surround the cell body suggests that SGCs mediate cross-excitation. This idea of enhanced direct neuron-SGC and indirect neuron-neuron communication following injury is consistent with a number of recent studies on rodent pain models. The current suspected participants in this paracrine signaling mechanism include ATP and hypersensitivity of purinergic receptors, release of substance P and cytokine, changes in expression of ion channels, increased gap junction coupling, and a sustained calcium wave. C. ATP, Pannexins, and Enhanced Purinergic Transmission
ATP is the primary messenger between neurons and SGCs in the pain pathway through a process called purinergic transmission. Pannexins (Panx) are homologs of gap junction proteins and form membrane channels that allow ATP to be released into extracellular fluid (Hanani & Spray, 2020). P2 receptors, a type of purinergic receptor, are found on the surface of neurons and are activated by extracellular ATP. There are seven ligand-gated ionotropic receptors (P2X1R-P2X7R) and eight G protein-coupled receptors (P2Y1R, P2Y2R, P2Y4R, P2Y6R, P2Y11R-P2Y14R) (Inoue et al., 2005). Pharmacological and molecular biological analysis has shown that these receptors play a dominant role in mediating nociceptive response to extracellular ATP in the CNS (“Purinergic Receptor”). This relationship between ATP and purinergic receptors remains true in SGC-neuron interaction in response to neuropathic pain. Both
SGCs and neurons can release ATP through Panx1 channels, and both cell types express P2 receptors (Costa & Neto, 2015). Extracellular ATP binds to and activates P2 receptors and is taken in by the cell via endocytosis. Ecto-ATPases then break down ATP to ADP and other purines. In the DRG, ecto-ATPases are found in SGCs but not in neurons, enabling SGCs to regulate the ATP level in sensory ganglia (Hanani & Spray, 2020). Once P2X receptors are activated by ATP, their ion channels open, ultimately leading to further ATP release. Thus, the sensitivity to and level of ATP plays a major role in the level of pain response of sensory ganglia. In several pain models including the DRG of rats following spinal nerve ligation (Zhang et al., 2015), the TG of orofacial pain models (Hanstein et al., 2016), and NG systemic inflammation models (Feldman-Goriachnik et al., 2015), it has been found that Panx1 expression is upregulated during chronic pain. This increased Panx1 expression enables greater amounts of ATP to be released in response to injury to neurons, thus further activating P2 receptors in both the SGCs and neurons. It has also been shown that the sensitivity of SGCs to ATP dramatically increases following injury. Several studies demonstrated that this increased sensitivity is attributed to a shift in the P2 receptor subtype population in SGCs in response to nerve damage or inflammation. One such study of TG pain models recorded an increase in the sensitivity of SGCs to ATP in the TG by 100-fold (Kushnir et al., 2011). It was found that this was caused by a shift in the P2 receptor population. In healthy mice, the response of SGCs to ATP was primarily mediated by P2Y receptors. In comparison, in mice with inflammation, response to ATP was mediated by P2XR. Though the exact subtype of P2XR was not determined, the candidates included P2X2R, P2X4R, and P2X5R, but not P2X7R. In a similar study on DRG SGCs, P2X7R was upregulated in patients with chronic pain (Blum et al., 2014). These studies demonstrate that the expression of P2 receptor subtypes in SGCs shifts in response to injury. The exact P2XR subtype that the population shifts towards varies depending on the type of sensory ganglia. In the second study previously mentioned, the primary P2 receptor subtype appeared to shift toward P2X7R in the DRG. In contrast, in the first study, researchers determined that P2X7R did not become prominent in TG following injury. Furthermore, the level of exportable ATP in both SGCs and neurons depends upon the concentration of intracellular Ca2+, as the metabolism of ATP requires Ca2+. Therefore it is not surprising that there is not only an increase in sensitivity to ATP following injury, but there is also an increase in intracellular Ca2+, necessary to keep up with the levels of ATP metabolism. Because of this, the level of Ca2+ can be used as a marker for the activation of P2R. This is especially true for the activation of P2X7R. Focusing specifically on P2X7R, the activation of P2X7R is associated with the maturation and release of interleukin-1β (IL-1β), a potent inflammatory cytokine whose downstream effects are known to lead to the generation and maintenance of pain (Chessell et al., 2005). In a study looking to define the molecular physiology of the P2 receptor subtypes, P2X7R was also found to become more sensitive to ATP in low extracellular Ca2+ concentrations (North, 2002). As the concentration of intracellular Ca2+ increases, the concentration of extracellular Ca2+ decreases, further increasing the sensitivity of P2X7R. These two characteristics place P2X7R as a major target for chronic pain drugs. Ultimately, an excessive response to injury by P2X7R can lead to chronic pain by releasing large amounts of ATP, contributing to sustained P2X7R activation and a pro-inflammatory feedback loop. D. Other Signaling Messengers
In addition to ATP, several other neurotransmitters act as messengers between SGCs and neurons. In particular, active neurons release substance P and calcitonin gene-related peptide (CGRP), which, along with ATP, facilitates the activation of the surrounding SGC sheath through paracrine signaling as shown in Fig. 4 (Costa & Neto, 2015). Active SGCs then re-
lease pro-inflammatory cytokines, which, in turn, act on neurons. Substance P is a neuropeptide whose most well-defined function is modulating pain perception by altering cellular signaling pathways (Graefe & Mohiuddin, 2022). This function is consistent with its role in activating SGCs following stimuli. Its receptor is neurokinin type 1 (NK1) as shown in Fig. 4. Furthermore, in an orofacial inflammation model, scientists found that there is both an increase in substance P release as well as NK1 receptor expression (Hanani, 2015). This increase contributes to a rise in cytokine release from SGCs. Another messenger that contributes to SGC activation is CGRP, which is known to be a major factor in the pathophysiology of migraines (Henson et al., 2020). In summary, neurons use ATP, substance P, and CGRP to communicate with and activate SGCs. As previously mentioned, active SGCs release pro-inflammatory cytokines, including IL-1β and TNF-α as shown in Fig. 4 (Schäfers et al., 2003; Neves et al., 2020). Cytokines recruit leukocytes, including lymphocytes, granulocytes, monocytes, and macrophages, ultimately leading to inflammation around the sensory ganglia (“Cytokine”). The uptake of cytokines by sensory ganglia has also been found to cause hyperalgesia and allodynia in mice, increasing the action potential firing rate in inflamed mice compared to healthy mice (Takeda et al., 2007). These two effects caused by the release of cytokines are believed to contribute to chronic pain. Since SGCs are non-neuronal cells, they cannot conduct action potentials and are therefore lacking voltage-dependent Na+ and Ca2+ channels (Hanani & Spray, 2020). However, they exhibit Kir4.1 potassium channels which favor intaking K+ into cells over releasing them. With Kir4.1, SGCs can regulate the concentration of intracellular K+ in neurons by manipulating the extracellular K+ concentration, thus changing the concentration gradient between the outside and inside of the neuronal cell membrane. This is significant because the level of intracellular K+ in neurons dictates the resting potential and thus the excitability of the neuron (Costa & Neto, 2015). Thus, mediation of extracellular K+ concentrations by SGC is crucial in pain response. Several studies have demonstrated alterations in SGC Kir4.1 channels (Cherkas et al., 2004). In one mouse model, it was found that there was a significant reduction of K+ currents mediated by Kir4.1 in injured ganglia (Vit et al., 2006). Furthermore, the activation threshold for nociceptors decreased in mice with inflammation compared to healthy mice. Additionally, to further determine if these effects were caused by SGC Kir4.1 channels, scientists downregulated the expression of Kir channels in rats without inflammation. They found that rats began exhibiting pain behaviors even without pain stimuli. Therefore, these studies suggest that the downregulation of Kir4.1 channels contributes to chronic pain and is a characteristic of the SGC pain response mechanism. F. Increased Gap Junction Coupling and Calcium Wave
Figure 4. Diagram depicting messaging between a neuron and SGC following injury (Adapted from Costa & Neto, 2015) SGCs are interconnected via gap junctions. Gap junctions are intercellular channels that connect the cytoplasm of two cells, allowing the direct exchange of ions, messengers, and other small molecules between cells (Goodenough & Paul, 2009). Compared with the transmission of messengers across extracellular fluid, gap junctions provide low-resistance pathways that increase the speed of transmission. Gap junctions are expressed in both CNS and PNS glial cells, including SGCs. Gap
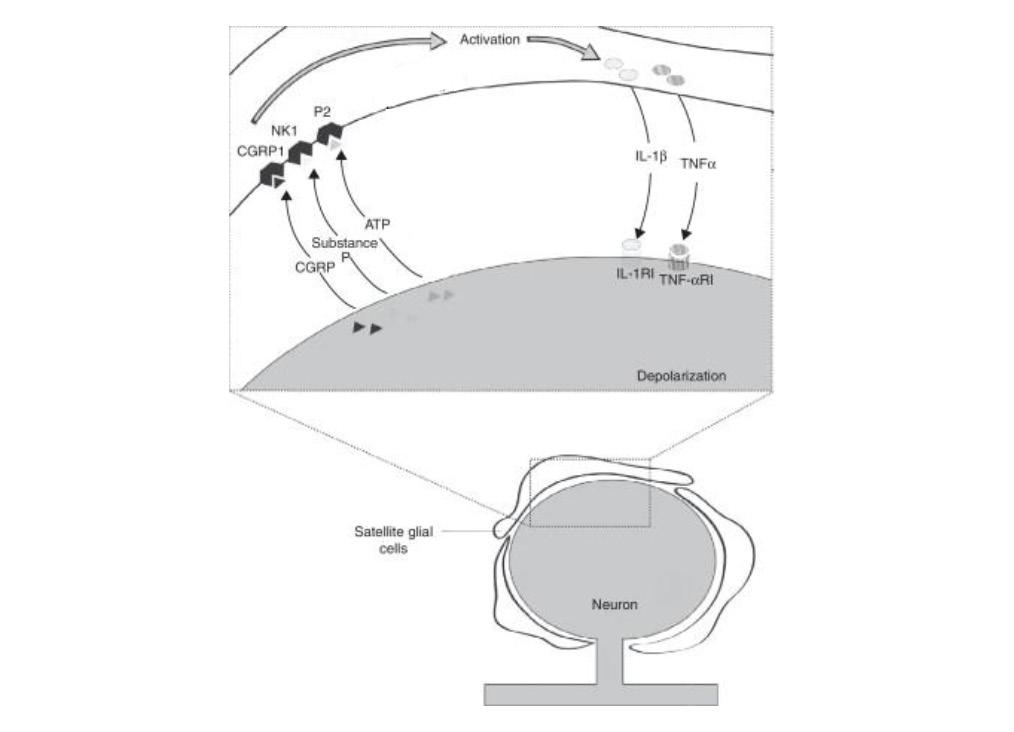
junctions found in SGCs are typically connexins (Cx). The most common Cx in mouse DRG and TG are Cx43 and Cx32 (Manteniotis et al., 2013). Cx30.2, Cx37, Cx26, Cx30, Cx45, and Cx36 have also been identified in SGCs (Manteniotis et al., 2013). This is how SGCs connect to form sheaths around neurons. This connection enables the activation of one SGC to spread to other SGCs in the same sheath as shown in Fig. 5a. Cx43 gap junctions do not occur between the cell body of nerves and SGCs. In animals exhibiting chronic pain, gap junctions appear to connect SGC sheaths of neurons other than the originally activated neuron, enabling signals to be spread to SGCs around adjacent neurons as shown in Fig. 5b. Studies show that this increased gap junction coupling between SGCs occurs following neuropathic injury and results in the hyperexcitability of neurons (Kim et al., 2016). In DRG and TG, Cx43 becomes upregulated following nerve injury or inflammation (Hanani & Spray, 2020). Cx26 expression was also found to have increased in injured TG. Regardless of the type of Cx, evidence indicates that the coupling between SGCs is a vital step in neuropathic pain development and maintenance. In a study on injured spinal ganglia in mice, the mean number of gap junctions for every 100 µm2 of SGC surface was five times greater in injured ganglia than in control ganglia (Pannese et al., 2003), thus demonstrating that new gap junctions form between SGCs following injury. In a different study, Cx43 was inhibited in mice that had chemotherapy-induced chronic neuropathic pain using the drug carbenoxolone (Warwick & Hanani, 2013). Results showed that mice with carbenoxolone exhibited signs of reduced pain, demonstrating that without functional Cx43 coupling, there is reduced pain generation and maintenance.
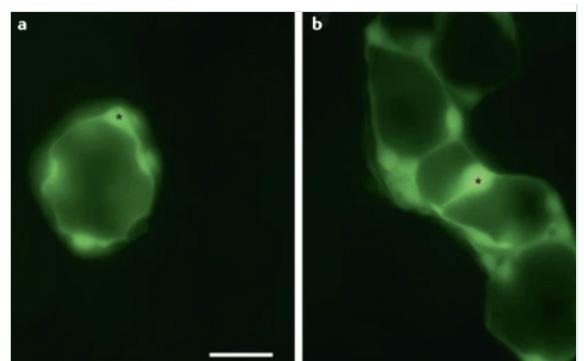
Figure 5. Dye coupling showing the spread of dye across gap junctions. An asterick marks where the SGC was injected with Lucifer yellow fluorescent dye. a) SGCs are dye-coupled to other SGCs in the sheath. b) In the DRG of a mouse with neuropathic pain, the dye spreads to adjacent SGC sheaths. (Hanani & Spray, 2020) G. Mechanism of Hyperexcitability and Cross-excitation
A proposed mechanism for how increased gap junction coupling results in neuronal hyperexcitability is that it not only bolsters existing connections between SGCs thus facilitating the spread of pain signals across a single sheath, but it also connects previously separate sheaths, resulting in the spread of pain signals to adjacent sensory neurons (Hanani, 2015). In this model, the pain signal from one neuron spreads to others, resulting in the cross-excitation of sensory ganglia. It is believed that a pain signal leads to ATP, CGRP, and substance P release from the neuron (Hanani & Spray, 2020). As P2 receptors are activated, SGCs are also activated, releasing more ATP and pro-inflammatory cytokines such as TNF-α and IL1β. These cytokines, in turn, attract leukocytes, induce inflammation, and influence the excitability of the neuron through TNF-α and IL1β receptors. The increased concentration of P2 receptors and shift in P2 receptor subtypes further amplifies this response. For sensory ganglions that have increased expression of P2X7R, the effect of ATP is further increased as extracellular calcium diffuses across the gradient. SGCs enable the maintenance of this pain response in chronic pain through sustained Ca2+ waves. The increased gap junction coupling combined with enhanced P2R transmission enables the spread of signals via Ca2+ wave propagation. Calcium activates other SGCs and sensory neurons, reducing extracellular calcium concentration, and increasing ATP release and sensitivity, thus creating a feedback loop that ultimately leads to allodynia and chronic pain. Summarized in Fig 4 and Fig 6, this mechanism relies on abnormal bidirectional neuron-SGC and SGC-SGC communication.
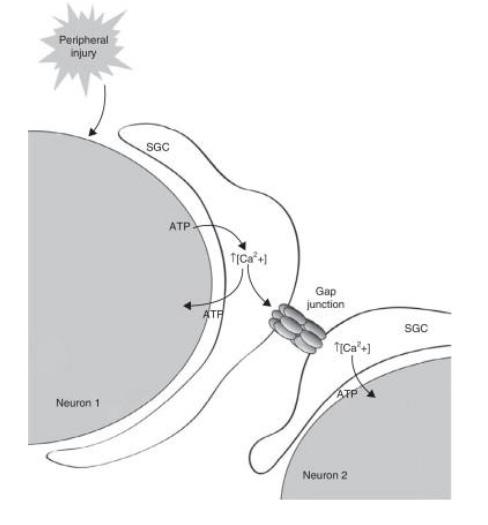
Figure 6. Model of sustained calcium wave across neurons through satellite glial cells and gap junctions (Costa & Neto, 2015) III. PROPOSED HYPOTHETICAL EXPERIMENT
A. Introduction
Given P2X7R’s unique upstream position and its importance in the pain cascade, interruption of it could be a more effective analgesic than current chronic pain treatments. Thus, we present the selective P2X7R antagonist A-740003 to interrupt the ATP release cycle and thus return pathological neuronal behavior to normal. B. P2X7R Antagonist A-740003
A-740003 is a competitive antagonist of P2X7R which means that it binds to the same activation site as the agonist (i.e. ATP) but does not activate it, thus blocking the receptor’s function. It has an IC50 value of 40 nM for a human and 18 nM for a rat, while also showing weak to no activity on other P2 receptors, making it a highly selective drug for targeting P2X7 (Honore et al., 2006). Because of its selective properties, the drug can block P2X7 without disrupting the functions of other P2 receptors in the SGC. C. Experimental Overview
Two tests would be performed to analyze the effect of A-740003 on rats with hyperalgesia. The first test would confirm P2X7R activation, blockage, and downstream effects in vitro, The second test would determine the effect of blockage of P2X7R with A-740003 in live rats exhibiting hyperalgesia. D. Materials and Methods
1) Animals: Experiments would be conducted on live rats in setups according to Neves et al., 2020. Animals would be housed in plastic cages with soft bedding. There would be a 12-hour light/dark cycle with controlled humidity and temperature. Animals would have unlimited access to food and water. All animal handling procedures would be done in accordance with the International Association for the Study of Pain guidelines for the use of animals in pain research in order to ensure that the experiments would be done humanely (“IASP Guidelines for the Use of Animals in Research”). 2) Hyperalgesia Induction Method: Chronic pain often involves hypersensitivity to stimuli that previously would not have yielded as much discomfort, so, to replicate chronic pain, hyperalgesia would be induced by injecting the rat with an inflammatory agent in the sole of the rat’s hind paw, which is part of the L5 DRG (Araldi et al., 2013). 50 µL of Complete Freund’s adjuvant (CFA) would be used as the inflammatory agent, as CFA is a commonly-used antigen that induces autoimmune disease in animal models by enhancing IL‐6 production (Fontes et al., 2017). Mechanical stimuli would then be applied on the same paw of the rat, thus enabling the measurement of hyperalgesia (Neves et al., 2020). 3) A-740003 Drug Delivery Method: In vivo, the P2X7R antagonist A-740003 would be injected following CFA injection. The antagonist would be diluted in a solution of 10% dimethyl sulfoxide (DMSO), 10% propylene glycol, and 80% sterile saline (NaCl 0.9%) as done by Neves et al., 2020. There would be three different doses of differing concentrations, 0.01, 0.10, and 1.00 mM. These concentrations are based on the dose used in an inflammatory pain model by (Neves et al., 2020). The injection technique would be performed by anesthetizing the animal. An ultra-fine needle would then be inserted through the skin
until it hits the L5 DRG (Neves et al., 2020). We would know that the needle has reached the L5 DRG when a paw-flinch reflex is observed (Neves et al., 2020). 5 µL of the solution would then be injected (Neves et al., 2020). The goal of this administration is to isolate the injection to the L5 DRG, ensuring that it does not touch other ganglia such as the spinal cord between the L1-T13 segments (Oliveira et al., 2009). 4) Measurement of Hyperalgesia with Electronic Von Frey Test: Using the electronic Von Frey test, we would be able to measure the level of pain in the rats based on “pain-like” responses to stimuli. Because pain cannot be directly measured in animals, scientists commonly use the Von Frey test. Developed by physiologist Maximilian von Frey in 1894, the test measures pain based on the withdrawal response of the animal following external stimulus (Deuis et al., 2017). In this test, each animal would be placed in a cage with mesh floors, permitting access to the bottom of its paws (Neves et al., 2020). Animals would then be allowed to habituate to the cages until anxiety and exploratory behaviors have calmed down. A filament would then be positioned perpendicularly to the plantar surface of the animal’s hind paw (i.e. same location innervated by the L5 DRG) (Deuis et al., 2017). Then, an increasing force would be applied until the animal voluntarily withdraws its paw. This stimulus would be repeated several times, each time measuring the withdrawal threshold. Animals were tested before and after treatments. To minimize human variation, the same person (blind to the treatments) would conduct all the electronic Von Frey tests (Neves et al., 2020). The results (i.e. the intensity of hyperalgesia) are defined as the difference between the withdrawal threshold before and after treatments. E. Confirming P2X7R Activation, Blockage, and Downstream Effects in vitro
1) Experimental Setup: To check for P2X7R activation and blockage in SGCs, we would first evaluate changes in intracellular Ca2+ concentration in vitro in a DRG culture. We would measure the Ca2+ concentration before and after the application of ATP with calcium imaging (Neves et al., 2020). We would also confirm that A-740003 blocks P2X7R by incubating the DRG culture with A-740003 after ATP is added. We would again measure the Ca2+ concentration before and after the application of ATP. We would also check the downstream effects of P2X7R activation in vitro by stimulating the DRG culture with lipopolysaccharides (LPS) to mimic an injury and induce inflammation. After a given period of time, cultures would then be treated with ATP and A-740003. Finally, an enzyme-linked immunosorbent assay (ELISA) would be used to measure the levels of released cytokines especially TNF-α and IL-1β (Neves et al., 2020). 2) Predicted Results and Discussion: Based on our proposed SGC mechanism, activation of P2X7R should result in increased intracellular calcium concentration in SGCs. Therefore, calcium imaging should show a higher concentration of calcium in SGC sheaths following activation by ATP. In the test where A-740003 is applied, calcium imaging should show little to no change of calcium in SGCs following ATP application. Furthermore, the ELISA should yield high levels of TNF-α and IL-1β in response to injury when no A-740003 is applied in comparison to when A-740003 is applied. If these predicted results are true, it would be confirmed that P2X7R plays a vital role in increasing the intracellular calcium concentration and, thus, facilitating the calcium wave. It would also show that P2X7R activation induces pro-inflammatory cytokines. F. Blockage of P2X7R with A-740003 in Rats Exhibiting Hyperalgesia in vivo
1) Setup: To determine the effect of blocking P2X7R with A-740003 following the development of peripheral inflammatory hyperalgesia, the inflammatory agent CFA would be first administered into the right hind paw of each animal in this test. Split into four groups, three experimental groups would receive three different doses of A-740003 in the same location (Neves et al., 2020). The control group
would be injected with only saline. After a given time, the electronic von Frey test would then be conducted to measure if A-740003 has an effect on hyperalgesia in the animals. 2) Predicted Results and Discussion: Application of A-740003 should decrease the hyperalgesia compared to the control group. The higher the concentration of A-740003, the greater the tolerance the animals should have to the stimuli. For example, the groups that were administered with 1.00 mM of A-740003 should have a greater tolerance to the stimuli than the 0.10 mM and 0.01 mM groups. The control group should have the least tolerance to the stimuli. If these predicted results are true, it would demonstrate that the A-740003 blockage of P2X7R reduces hyperalgesia triggered by the inflammatory agent CFA. The likely explanation for this result is that the blockage of P2X7R inhibits the calcium wave and release of cytokines, thus reducing the hyperactivity of the sensory ganglia. Reduction of hyperactivity in neurons could lead to the reduction of chronic pain because nociceptors would return to their normal action potential threshold.
IV. CONCLUSION
In this paper, we summarized and discussed the known mechanisms and alterations of SGCs in chronic pain. Based on this mechanism, we also proposed a hypothetical experiment of using A-740003 to target P2X7R, a purinergic receptor whose downstream effects of increased calcium uptake and cytokine release contribute to the pathogenesis of the SGC pain response. Further research needs to be done to determine the potential side effects of A-740003, the efficacy of the drug on humans, and if the drug works on other chronic pain models. Other drugs that target the role of SGCs in pain should also be explored. It would be beneficial to further investigate the chemical mechanisms that induce the changes in SGCs described in this paper in order to better develop future drugs. Due to the location of sensory ganglia and SGCs early on in the pain signaling pathway, the close envelopment of SGCs around neurons, and the lack of the need to directly manipulate neurons, SGCs are a promising therapeutic target for chronic pain.
Works Cited
[1] Araldi, D., Ferrari, L. F., Lotufo, C. M., Vieira, A. S., Athié, M. C. P., Figueiredo, J. G., Duarte, D. B., Tambeli, C. H., Ferreira, S. H., & Parada, C. A. (2013). Peripheral inflammatory hyperalgesia depends on the COX increase in the dorsal root ganglion. Proceedings of the National Academy of Sciences of the United States of America, 110(9), 3603–3608. https://doi.org/10.1073/pnas.1220668110 [2] Blum, E., Procacci, P., Conte, V., & Hanani, M. (2014). Systemic inflammation alters satellite glial cell function and structure. A possible contribution to pain. Neuroscience, 274, 209–217. https://doi.org/10.1016/j.neuroscience.2014.05.029 [3] Cherkas, P. S., Huang, T. Y., Pannicke, T., Tal, M., Reichenbach, A., & Hanani, M. (2004). The effects of axotomy on neurons and satellite glial cells in mouse trigeminal ganglion. Pain, 110(1), 290–298. https://doi.org/10.1016/j. pain.2004.04.007 [4] Chessell, I. P., Hatcher, J. P., Bountra, C., Michel, A. D., Hughes, J. P., Green, P., Egerton, J., Murfin, M., Richardson, J., Peck, W. L., Grahames, C. B. A., Casula, M. A., Yiangou, Y., Birch, R., Anand, P., & Buell, G. N. (2005). Disruption of the P2X7 purinoceptor gene abolishes chronic inflammatory and neuropathic pain. Pain, 114(3), 386–396. https://doi. org/10.1016/j.pain.2005.01.002 [5] Classification of chronic pain, second edition(Revised). (n.d.). International Association for the Study of Pain (IASP). Retrieved September 1, 2022, from https:// www.iasp-pain.org/publications/free-ebooks/classification-of-chronic-pain-second-edition-revised/ [6] Costa, F. A. L., & Neto, F. L. M. (2015). Satellite glial cells in sensory ganglia: Its role in pain. Brazilian Journal of Anesthesiology (English Edition), 65(1), 73–81. https://doi. org/10.1016/j.bjane.2013.07.013 [7] Cytokine | biochemistry | britannica. (n.d.). Retrieved September 1, 2022, from https://www.britannica.com/science/cytokine [8] Deuis, J. R., Dvorakova, L. S., & Vetter, I. (2017). Methods used to evaluate pain behaviors in rodents. Frontiers in Molecular Neuroscience, 10, 284. https://doi.org/10.3389/ fnmol.2017.00284 [9] Different types of chronic pain. (2020, March 26). Southern Pain and Neurological. https://southernpainclinic.com/ blog/different-types-of-chronic-pain/ [10] Feldman-Goriachnik, R., Belzer, V., & Hanani, M. (2015). Systemic inflammation activates satellite glial cells in the mouse nodose ganglion and alters their functions. Glia, 63(11), 2121–2132. https://doi.org/10.1002/glia.22881 [11] Fontes, J. A., Barin, J. G., Talor, M. V., Stickel, N., Schaub, J., Rose, N. R., & Čiháková, D. (2017). Complete Freund’s adjuvant induces experimental autoimmune myocarditis by enhancing IL‐6 production during initiation of the immune response. Immunity, Inflammation and Disease, 5(2), 163–176. https://doi.org/10.1002/iid3.155 [12] Freudenrich, C. (2007). How Pain Works. HowStuffWorks. https://science.howstuffworks.com/life/inside-the-
mind/human-brain/pain.htm [13] Gazerani, P. (2021). Satellite glial cells in pain research: A targeted viewpoint of potential and future directions. Frontiers in Pain Research, 2, 646068. httpsdoi.org/10.3389/ fpain.2021.646068 [14] Goodenough, D. A., & Paul, D. L. (2009). Gap junctions. Cold Spring Harbor Perspectives in Biology, 1(1), a002576. https://doi.org/10.1101/cshperspect.a002576 [15] Graefe, S. B., & Mohiuddin, S. S. (2022). Biochemistry, substance P. In StatPearls. StatPearls Publishing. http:// www.ncbi.nlm.nih.gov/books/NBK554583/ [16] Hanani, M. (2015). Role of satellite glial cells in gastrointestinal pain. Frontiers in Cellular Neuroscience, 9. https:// doi.org/10.3389/fncel.2015.00412 [17] Hanani, M., & Spray, D. C. (2020). Emerging importance of satellite glia in nervous system function and dysfunction. Nature Reviews Neuroscience, 21(9), 485–498. https:// doi.org/10.1038/s41583-020-0333-z [18] Hanstein, R., Hanani, M., Scemes, E., & Spray, D. C. (2016). Glial pannexin1 contributes to tactile hypersensitivity in a mouse model of orofacial pain. Scientific Reports, 6(1), 38266. https://doi.org/10.1038/srep38266 [19] Henson, B., Hollingsworth, H., Nevois, E., & Herndon, C. (2020). Calcitonin gene-related peptide (Cgrp) antagonists and their use in migraines. Journal of Pain & Palliative Care Pharmacotherapy, 34(1), 22–31. https://doi.org/10.1080/1 5360288.2019.1690616 [20] Honore, P., Donnelly-Roberts, D., Namovic, M. T., Hsieh, G., Zhu, C. Z., Mikusa, J. P., Hernandez, G., Zhong, C., Gauvin, D. M., Chandran, P., Harris, R., Medrano, A. P., Carroll, W., Marsh, K., Sullivan, J. P., Faltynek, C. R., & Jarvis, M. F. (2006). A-740003 [N-(1-{[(cyanoimino)(5-quinolinylamino) methyl]amino}-2,2-dimethylpropyl)-2-(3,4-dimethoxyphenyl)acetamide], a novel and selective P2X7 receptor antagonist, dose-dependently reduces neuropathic pain in the rat. The Journal of Pharmacology and Experimental Therapeutics, 319(3), 1376–1385. https://doi. org/10.1124/jpet.106.111559 [21] IASP guidelines for the use of animals in research. (n.d.). International Association for the Study of Pain (IASP). Retrieved September 1, 2022, from https://www. iasp-pain.org/resources/guidelines/iasp-guidelines-forthe-use-of-animals-in-research/ [22] Inoue, K., Tsuda, M., & Koizumi, S. (2005). ATP receptors in pain sensation: Involvement of spinal microglia and P2X4 receptors. Purinergic Signalling, 1(2), 95–100. https:// doi.org/10.1007/s11302-005-6210-4 [23] Jasmin, L., Vit, J. P., Bhargava, A., & Ohara, P. (2010). Can satellite glial cells be therapeutic targets for pain control? Neuron Glia Biology, 6(1), 63–71. https://doi.org/10.1017/ S1740925X10000098 [24] Kim, Y. S., Anderson, M., Park, K., Zheng, Q., Agarwal, A., Gong, C., Saijilafu, Young, L., He, S., LaVinka, P. C., Zhou, F., Bergles, D., Hanani, M., Guan, Y., Spray, D. C., & Dong, X. (2016). Coupled activation of primary sensory neurons contributes to chronic pain. Neuron, 91(5), 1085–1096. https://doi.org/10.1016/j.neuron.2016.07.044 [25] Kushnir, R., Cherkas, P. S., & Hanani, M. (2011). Peripheral inflammation upregulates P2X receptor expression in satellite glial cells of mouse trigeminal ganglia: A calcium imaging study. Neuropharmacology, 61(4), 739–746. https:// doi.org/10.1016/j.neuropharm.2011.05.019 [26] Manteniotis, S., Lehmann, R., Flegel, C., Vogel, F., Hofreuter, A., Schreiner, B. S. P., Altmüller, J., Becker, C., Schöbel, N., Hatt, H., & Gisselmann, G. (2013). Comprehensive rna-seq expression analysis of sensory ganglia with a focus on ion channels and gpcrs in trigeminal ganglia. PLOS ONE, 8(11), e79523. https://doi.org/10.1371/journal. pone.0079523 [27] Neves, A. F., Farias, F. H., de Magalhães, S. F., Araldi, D., Pagliusi, M., Tambeli, C. H., Sartori, C. R., Lotufo, C. M. da C., & Parada, C. A. (2020). Peripheral inflammatory hyperalgesia depends on P2X7 receptors in satellite glial cells. Frontiers in Physiology, 11, 473. https://doi.org/10.3389/ fphys.2020.00473 [28] North, R. A. (2002). Molecular physiology of p2x receptors. Physiological Reviews, 82(4), 1013–1067. https://doi. org/10.1152/physrev.00015.2002 [29] Pannese, E., Ledda, M., Cherkas, P. S., Huang, T. Y., & Hanani, M. (2003). Satellite cell reactions to axon injury of sensory ganglion neurons: Increase in number of gap junctions and formation of bridges connecting previously separate perineuronal sheaths. Anatomy and Embryology, 206(5), 337–347. https://doi.org/10.1007/s00429-002-0301-6 [30] Purinergic receptor—An overview | sciencedirect topics. (n.d.). Retrieved September 1, 2022, from https://www. sciencedirect.com/topics/neuroscience/purinergic-receptor [31] Purves, D., Augustine, G. J., Fitzpatrick, D., Katz, L. C., LaMantia, A.-S., McNamara, J. O., & Williams, S. M. (2001). Neuroglial cells. Neuroscience. 2nd Edition. https://www. ncbi.nlm.nih.gov/books/NBK10869/ [32] Schäfers, M., Lee, D. H., Brors, D., Yaksh, T. L., & Sorkin, L. S. (2003). Increased sensitivity of injured and adjacent uninjured rat primary sensory neurons to exogenous tumor necrosis factor-alpha after spinal nerve ligation. The Journal of Neuroscience: The Official Journal of the Society for Neuroscience, 23(7), 3028–3038. [33] Shinder, V., & Devor, M. (1994). Structural basis of neuron-to-neuron cross-excitation in dorsal root ganglia. Journal of Neurocytology, 23(9), 515–531. https://doi.org/10.1007/ BF01262054 [34] Takeda, M., Tanimoto, T., Kadoi, J., Nasu, M., Takahashi, M., Kitagawa, J., & Matsumoto, S. (2007). Enhanced excitability of nociceptive trigeminal ganglion neurons by satellite glial cytokine following peripheral inflammation. Pain, 129(1), 155–166. https://doi.org/10.1016/j.pain.2006.10.007 [35] Vit, J. P., Jasmin, L., Bhargava, A., & Ohara, P. T. (2006). Satellite glial cells in the trigeminal ganglion as a determinant of orofacial neuropathic pain. Neuron Glia Biology, 2(4), 247–257. https://doi.org/10.1017/s1740925x07000427 [36] Warwick, R. A., & Hanani, M. (2013). The contribution of satellite glial cells to chemotherapy-induced neuropathic pain. European Journal of Pain (London, England), 17(4), 571–580. https://doi.org/10.1002/j.1532-2149.2012.00219.x [37] Yam, M., Loh, Y., Tan, C., Adam, S., Manan, N., & Basir, R. (2018). General pathways of pain sensation and the major neurotransmitters involved in pain regulation. International Journal of Molecular Sciences, 19(8), 2164. https://doi. org/10.3390/ijms19082164 [38] Zhang, Y., Laumet, G., Chen, S. R., Hittelman, W. N., & Pan, H. L. (2015). Pannexin-1 up-regulation in the dorsal root ganglion contributes to neuropathic pain development. Journal of Biological Chemistry, 290(23), 14647–14655. https://doi.org/10.1074/jbc.M115.650218