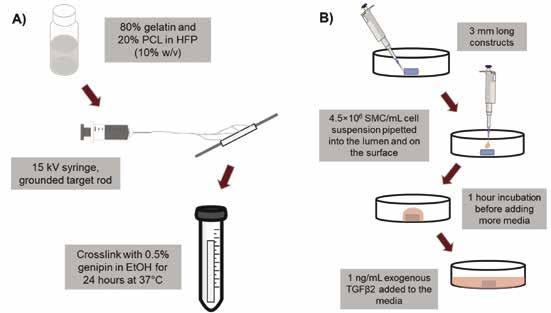
13 minute read
Monitoring the in-vitro extracellular matrix remodeling of tissue engineered vascular grafts
Hannah Schmidt a , Jonathan Vande Geest a, b, c a Department of Bioengineering, b McGowan Institute for Regenerative Medicine, c Vascular Medicine Institute
Hannah Schmidt
Hannah Schmidt is a fourth-year undergraduate studying Bioengineering at the University of Pittsburgh. She conducted research on tissue engineered vascular grafts with Dr. Vande Geest’s lab for two years and, in the future, plans to attend medical school.
Dr. Jonathan Vande Geest is a Professor in the Department of Bioengineering, Department of Mechanical Engineering and Material Science, the Department of Ophthalmology, the McGowan Institute for Regenerative Medicine, the Louis J. Fox Center for Vision Restoration, and the Vascular Medicine Institute at the University of Pittsburgh. He received his BS in Biomedical Engineering from the University of Iowa in 2000 and his PhD in Bioengineering from the University of Pittsburgh in 2005. Dr. Vande Geest began his career at the University of Arizona in the Department of Aerospace and Mechanical Engineering and joined the U of A’s Department of Biomedical Engineering in 2009. Dr. Vande Geest returned to the University of Pittsburgh in January of 2016. Dr. Jonathan Vande Geest
Significance Statement
It can be difficult to visualize longitudinal structural changes in-vivo in biological systems, oftentimes due to the necessity of destructive imaging. This research successfully overcomes this challenge for the study of vascular graft collagen remodeling by developing two-photon imaging parameters which can be generalized to broad in-vivo tissue engineering applications.
Category: Methods Keywords: tissue engineered vascular graft, collagen remodeling, two-photon microscopy
Abstract
Heart disease is the leading cause of death in the United States for both men and women. Coronary artery bypass grafts (CABG) are frequently implanted to restore blood flow to the heart, but these small diameter vascular grafts frequently accumulate clots and become narrow, making them ineffective. The Soft Tissue Biomechanics Laboratory (STBL) is seeking to create a tissue engineered vascular graft (TEVG) to address these issues of compliance mismatch and thrombosis in small diameter grafts. It is particularly important to assess the extracellular matrix remodeling (ECM) capabilities of our TEVGs in order to monitor the in-vivo transition of TEVGs from synthetic graft to host remodeled tissue. This study therefore aims to develop an in-vitro imaging method for quantifying ECM remodeling of TEVGs. We were able to determine optimal imaging parameters and show that two-photon imaging can be used to characterize structural changes of collagen in the ECM, which will be used in the future to evaluate TEVG efficacy.
1. Introduction
Heart disease was responsible for 633,842 deaths in 2015 [1], making it the leading cause of death in the United States for both men and women. Coronary artery disease (CAD) in particular is responsible for nearly half of all heart disease cases [2]. Coronary artery bypass grafting (CABG) from autologous vessels is a common treatment used to restore blood flow to the heart in patients with CAD, but suffers from high rates of thrombosis and restenosis, with reintervention rates reported to be as high as 8.8% [3]. Providing a functional tissue engineered vascular graft (TEVG) for CABG surgeries would therefore result in substantial improvements in patient care.
The Soft Tissue Biomechanics Laboratory is creating a TEVG for small diameter CABG applications to address these issues. Our team aims to create a primarily acellular, biocompatible, and compliance matched graft. This study will focus on evaluation of the overall function of the TEVGs. Because the grafts are primarily acellular when implanted, we must ensure that native cells can migrate and proliferate within the graft to transition TEVGs into living tissue. This transition is accomplished primarily through the production of a collagen extracellular matrix (ECM) by vascular smooth muscle cells as the TEVG degrades. It is therefore necessary to quantify the in-vitro ECM remodeling of TEVGs to understand the graft’s in-vivo transition from polymer-based scaffold to host remodeled tissue.
Current approaches to imaging ECM remodeling include the work of Hjortnaes et al. 2009 [4], which showed that TEVG remodeling in-vivo can be monitored using laser scanning fluorescence imaging. The study relied on injection of nondestructive imaging agents which provided signal in response to proteolytic activity. Though they were successfully able to see changes in the enzymatic activity of TEVGs in a mouse model, graft degradation and new ECM formation cannot be directly measured using this method because it does not actually visualize the collagen or the TEVG itself. Rather, it uses enzymatic activity to make inferences about ECM remodeling. A different method is required to visualize degradation and collagen formation first-hand.
Several other studies such as Raub et al. 2007 [5] and Quinn et al. 2016 [6] have developed methods for the assessment of collagen structure using multiphoton microscopy, but they have not sought to differentiate native collagen from synthetic graft materials or cells and compare their changes over time. The present study seeks to develop a method that provides this additional information which is lacking from the current approach, which is important to understanding how native tissue interacts with the graft. Understanding the coupled actions of graft degradation and new collagen formation is critical to evaluating the effectiveness of a TEVG.
This study therefore will aim to develop a method for viewing and quantifying structural ECM changes through two-photon (2P) imaging. First, optimal imaging parameters will be determined, then the imaging method will be applied to study collagen gels. Collagen gels were chosen as a simpler model as they already possess a well-defined collagen matrix. Thus they provide a good model to critically evaluate the efficacy of the proposed ECM remodeling quantification method, toward eventual in-vitro and in-vivo applications.
2. Methods
2.1 TEVG Fabrication and Seeding
TEVGs were fabricated by electrospinning a 10% (w/v) solution of gelatin (Sigma) and polycaprolactone (PCL, Sigma) in 1,1,1,3,3,3-Hexafluoro-2-propanol (HFP, Oakwood Chemical). A ratio of 80:20 (gelatin:PCL) was chosen based on the work of Ardila et al. 2015 [7], which showed that this ratio is favorable for smooth muscle cell growth and migration. The 80G:20P solution was electrospun at 15kV onto a rotating and translating target rod to create cylindrical constructs with an inner diameter of 1.4 mm and a radial thickness of 200 μm. Constructs were then crosslinked in 0.5% genipin (Fisher Scientific) in EtOH for 24 hours at 37°C. After crosslinking, constructs were washed three times in EtOH, then three times in 1X PBS. Porcine aortic smooth muscle cells (SMC), isolated as described by Ardila et al. 2015 [7], were then seeded onto 3 mm axial length constructs by dropwise seeding at a density of 4.5×10 6 cells/mL and incubated for 1 hour to allow cellular attachment. This seeding process was repeated twice. Seeded constructs were cultured in media containing 1 ng/ mL TGFβ2 (R&D Systems), which Ardila et al. 2015 [7] showed encourages SMC proliferation in electrospun constructs. Figure 1 shows a visual representation of this entire process.
Figure 1: Methods flow diagram for fabrication (A) and seeding (B) of TEVGs.
2.2 Creation of Excitation-Emission Spectra
The 80G:20P constructs were excited at a range of wavelengths (750-1000 nm) with a tunable laser at 50 nm increments under a 2P microscope (LaVision BioTec). Excitation laser power at the sample was kept at a constant 40 mW throughout. At each excitation wavelength, the resulting emission signal was filtered from 375 nm to 680 nm. The intensity of each filtered signal was measured. In this way, the emission spectrum of a 80G:20P construct was determined over a range of excitation wavelengths. The same process was repeated for a 40 μm thick cross section of rat aorta, exciting from 750-1250 nm with 50 nm increments. The strength of the emission signal from the adventitial layer of the aorta, which is composed of collagen expressing SHG, was evaluated to determine the emission spectrum of fibrillar collagen. Seeded TEVGs were imaged using optimized 2P parameters based on the excitation-emission spectra of the construct and collagen. 2.3 Collagen Gel Fabrication
After determining the efficacy of the imaging parameters on TEVGs, collagen gels were used to fully develop the method of ECM remodeling quantification. Collagen gels were used because they already have a well-defined fibrillar collagen structure, making them easier to study for the purpose of refining a method. To create the gel, type I collagen from rat tail (Fisher Scientific) was mixed with culture media to a final concentration of 3mg/mL. All materials were kept on ice to prevent premature polymerization. NIH 3T3 fibroblasts expressing red fluorescent protein (RFP) were then suspended within the collagen mixture, and the gels were allowed to polymerize at 37°C. 2.4 Image Analysis and Statistical Testing
Collagen gels (n=9) were imaged using the same optimized 2P parameters, created as described in Section 2.2. Eight images per sample, each 10 μm depth apart, were then converted to binary using ImageJ [8]. The porosity of each binarized image was calculated and the average porosity of each collagen gel sample was determined. This process was repeated at time points of 1, 4, and 6 days to observe variations over time. The change in average porosity over time was evaluated by comparing time points using a student’s t-test (α = 0.05).
3. Results 3.1 Imaging Parameter Optimization
Optimal imaging parameters were chosen based on the two-photon excitation-emission spectra for each graft component. Figure 2 shows the excitation-emission spectra for an 80G:20P construct and Figure 3 shows the spectra for collagen from rat aorta. Parameters to simultaneously image constructs and collagen were established as (excitation/collection) 900/620nm and 900/425nm respectively. These optimized parameters were applied to image TEVGs as shown in Figure 4, which is a representative image of a seeded TEVG after one week of culture in media containing 1 ng/mL TGFβ2. The same parameters were applied to the rest of this study.
94 Undergraduate Research at the Swanson School of Engineering Figure 2: Excitation-emission spectrum for 80G:20P electrospun constructs. Two distinct emission peaks are visible at 460 nm and 620 nm regardless of excitation wavelength.
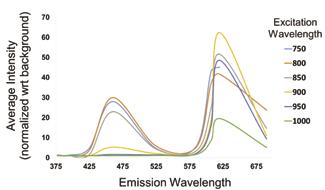
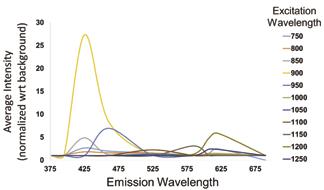
Figure 3: Excitation-emission spectrum for adventitial collagen in rat aorta. Emission peaks vary based on excitation wavelength due to second harmonic generation, corresponding to a maximum emission at roughly half the excitation wavelength.
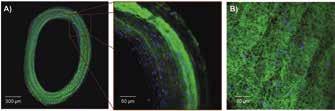
Figure 4: Cross sectional (A) and en face (B) views of an SMC seeded TEVG after one week of culture in media containing 1 ng/mL TGFβ2. The 80G:20P construct is shown in green and DAPI stained cell nuclei are shown in blue.
3.2 Porosity
Images of seeded collagen gels over time, which were used as a model for ECM remodeling, are shown in Figure 5. From image analysis, gels showed slight variations in average porosity of the collagen matrix over time (Figure 6), but no statistically significant differences were found between time points, with p=0.48 from day 1 to 4 and p=0.21 from day 4 to 6.
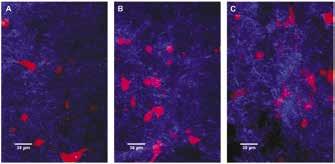
Figure 5: Seeded collagen gels over time at time points of 1(A), 4(B), and 6(C) days. Fibrillar collagen is shown in blue and RFP fibroblasts are shown in red.
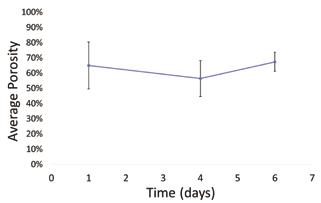
Figure 6: Average porosity of NIH 3T3 seeded collagen gels over time. No statistically significant difference in porosity was observed from day 1 to 4 (p=0.48) or from day 4 to 6 (p=0.21). Error bars show standard deviation.
4. Discussion
The emission spectra of the 80G:20P construct and ECM collagen have very distinct signals which can be used to distinguish them during intravital imaging. This is evident from their excitationemission spectra. The 80G:20P construct displays two distinct emission peaks at 460 nm and 620 nm regardless of excitation wavelength (Figure 2) which are likely due to the autofluorescence of genipin within the graft. The collagen emission signal, on the other hand, varies depending on excitation (Figure 3). Each peak appears at roughly half the excitation wavelength, corresponding to the second harmonic generation (SHG) of collagen. Using these spectra, imaging parameters can be easily tuned to fit future needs by selecting excitation wavelengths and collection filters which preferentially collect TEVG or collagen signal. Thus, parameters for spectrally separate imaging of TEVG remodeling components were established.
Porosity of collagen gel models, which was used as a metric for quantifying ECM changes, did not significantly change over time (Figure 6). This is primarily due to the high standard deviation of porosity between gels at the same time point and even between different areas of the same gel. Other measures of remodeling, such as cell count, had similarly high standard deviation and no statistically significant differences over time. This high variability makes it difficult to draw conclusions about ECM changes. Therefore this method could be improved by non-destructive imaging over several weeks with an emphasis on imaging the same location in both the x-y plane and in z. The next steps in this research will be to create a repeatable method of locating exact positions within 3D systems over long periods of time to more effectively monitor structural changes.
This study was, as mentioned, limited by the high variability of collagen gels. It is also notable that collagen gels do not provide a perfect model for translating this work to the in-vivo study of TEVGs over time. They are, however, an effective and useful tool for continuing to develop this intravital imaging method. Overall, this imaging method improved upon current methods in the literature by seeking to visualize the interplay between synthetic materials and collagen formation in TEVGs. It can be used to improve the current methods of evaluating TEVG function as it relates to host integration.
5. Conclusion
The imaging parameters established here (900/620nm and 900/425nm in excitation/collection for constructs and collagen respectively) can be used to effectively image a TEVG as it is remodeled by creating spectrally separate signals for each component. This method of image binarization and porosity calculation is also a valid strategy for quantifying structural changes such as porosity, which did not significantly change over time in this study with p=0.48 and p=0.21. It will require further work to locate exact 3D positions repeatedly for more accurate observations of changes over time. This work will be used to evaluate the overall function of TEVGs, ensuring that the acellular grafts are populated and remodeled in-vivo, and has the potential to be applied to broader tissue engineering applications for in-vivo imaging.
6. Acknowledgements
This work was funded by NIH 1R56HL136517-01 to JPVG and made possible, in part, by the 2018 American Heart Association SURP award to Hannah Schmidt.
7. References
[1] C.J. Rothwell, T.E. Price, A. Schuchat, Health, United States Report 2016. Hyattsville, MD: National Center for Health Statistics, Centers for Disease Control and Prevention, 2017.
[2] D. Mozaffarian et al., Heart disease and stroke statistics. Circulation. 2015;131:e29-e322
[3] P.W. Serruys, A.T. Ong, L.A. van Herwerden, et al., Fiveyear outcomes after coronary stenting versus bypass surgery for the treatment of multivessel disease: the final analysis of the Arterial Revascularization Therapies Study (ARTS) randomized trial. J Am Coll Cardiol, 2005. 46(4): p. 575-81.
[4] J. Hjortnaes, D. Gottlied, J.L. Figueirdo, et al., Intravital Molecular Imaging of Small-Diameter Tissue-Engineered Vascular Grafts in Mice: A Feasibility Study. Tissue Engineering Part C: Methods. 16.4 (Aug. 2010) p597
[5] C.B. Raub, V. Suresh, T. Krasieva, et al., Noninvasive assessment of collagen gel microstructure and mechanics using multiphoton microscopy. Biophys J. 2007;92(6):2212–2222. doi:10.1529/biophysj.106.097998
[6] K.P. Quinn, K.E. Sullivan, Z. Liu, et al., Optical metrics of the extracellular matrix predict compositional and mechanical changes after myocardial infarction. Sci Rep. 2016;6:35823. Published 2016 Nov 7. doi:10.1038/srep35823
[7] D.C. Ardila, E. Tamimi, F.L. Danford, et al., TGF 2 Differentially Modulates Smooth Muscle Cell Proliferation and Migration in Electrospun Gelatin-Fibrinogen constructs. Biomaterials. 2015 January; 37:164–173. doi:10.1016/j. biomaterials.2014.10.021.
[8] C.T. Rueden, J. Schindelin, M.C. Hiner, et al. (2017), “ImageJ2: ImageJ for the next generation of scientific image data”, BMC Bioinformatics 18:529, doi:10.1186/s12859-017-1934-z