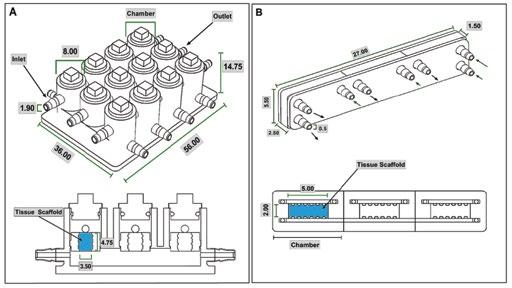
14 minute read
u Fluid flow simulation of microphysiological knee joint-on-a-chip
Fluid flow simulation of microphysiological knee joint-on-a-chip
Eileen N. Lia,b, Zhong Lib, Ryan M. Ronczkab, Hang Lina,b
aDepartment of Bioengineering, bDepartment of Orthopaedic Surgery, University of Pittsburgh School of Medicine
Eileen N. Li Eileen Li is a senior bioengineering student from northern Virginia. She has a passion in tissue engineering geared towards bone regeneration. After graduation she wishes to pursue a PhD and a career in academia.
Dr. Lin is an Assistant Professor in the Department of Orthopaedic Surgery and Department of Bioengineering. His research is focused on investigating the association between aging and OA, establishing an in vitro microphysiological OA model for OA pathogenesis study and drug development, and Hang Lin, Ph.D. testing stem cell-based therapy for the repair of cartilage injury.
Significance Statement
The progression of disease-modifying drugs to combat osteoarthritis (OA) has been limited due to the absence of an appropriate OA model that can effectively simulate the pathologies in humans. Our lab has constructed a 3-dimensional, human cell-derived, multi-tissue microphysiological system (microJoint) and high-throughput microJoint (HTP-microJoint) to mimic the native joint and model OA pathogenesis. Through the utilization of finite element analysis, both microJoints were investigated for characteristics that promote joint tissue development and maintenance.
Category: Computational Research
Keywords: microphysiological system, finite element
analysis, shear stress, joint disease, osteoarthritis, model
Abbreviations: osteoarthritis (OA), high-throughput
microJoint (HTP-microJoint), finite element analysis (FEA)
Abstract
Osteoarthritis (OA) is a degenerative disorder that effects 240 million people globally, leading to inflammation, chronic pain and restricted movement of joints [1]. Current osteoarthritis (OA) drugs are merely palliative. The development of drugs for OA treatment has been limited by the unavailability of a suitable OA model. To address this issue, we have developed a 3-dimensional (3D), human cell-derived, multi-tissue microphysiological system (microJoint); and further, an additional high-throughput microJoint (HTP-microJoint) chip was designed for high-throughput drug testing and convenient real-time imaging analysis. The 3-dimensionality and multi-tissue nature of the microJoint chips establish many similarities to the native joint and can therefore enhance our investigation of OA in comparison to models that are 2-dimensional or do not have multiple joint tissues. However, the fluid flow characteristics of the chips and their effects on the tissue maturation and maintenance are not fully understood, therefore finite element analysis (FEA) was conducted for both chips to quantify the fluid flow-induced shear stress and flow trajectory parameters within the tissue chambers. Volumetric flow rates utilized in the lab for tissue culture within the microJoint chips were applied to the geometry for the analysis. Laminar flow and the continuum hypothesis assumptions were validated through calculations. Fluid velocity analysis indicated no stagnant media areas within the microJoint chips. Quantified fluid-induced shear stress on the tissues within the microJoint chips have been previously reported to enhance osteogenic and chondrogenic tissue differentiation. The analysis suggests that both microJoint chips provide environments that enhance joint tissue development. Therefore, the microJoint chips can closely mimic native joint tissues and will establish a physiologically relevant model of OA progression.
1. Introduction
Osteoarthritis (OA) is a painful and debilitating disease that affects multiple joint components, including articular cartilage, subchondral bone, synovium, and infrapatellar fat pad. The limited progress in the development of disease-modifying OA drugs is mainly due to the absence of an effective OA model that mimics complex and active tissue crosstalk within the joint.
1.1 Existing models
Animal models represented by mice have a few advantages such as the ability to simulate natural OA progression and the ability to efficiently establish chemically- or trauma-induced OA [2]. However, animal models have limitations as these models are expensive, mimic only part of the features of human OA, and do not accurately predict clinical responses to OA drugs [2]. Along with in vivo models, in vitro models have also been used to investigate OA. Monolayer culture of chondrocytes does not replicate the in vivo 3D tissue niche and often leads to dedifferentiation. Pellet culture of chondrocytes can generate 3D cartilage tissues, but does not replicate tissue crosstalk. Another in vitro model, tissue explants, allows for the investigation cells in their native environment and the study of cartilage as a whole tissue. However, this model has a strong dependence on the donor and tissue harvest site and commonly seen cell death on the tissue edges [2].
To address these shortcomings, we have developed a 3-dimensional (3D), human cell-derived, multi-tissue microphysiological system (microJoint) that can be used to mimic the in vivo joint environment, tissue-specific ECM, interactive physiological biochemical and physical signals, along with modeling OA [3]. In addition, a high-throughput microJoint chip (HTP-microJoint), which significantly reduces cell and material consumption, was also developed to facilitate potential drug testing applications.
Previous studies in the lab have shown the successful maintenance of the tissue phenotypes in the microJoint tissue up to 4 weeks as well as the generation of OA models by inducing synovium inflammation [4]. As we move forward, it is essential to analyze and evaluate the effects of flow trajectory and shear stress induced by medium perfusion on tissue health. 1.2 Finite Element Analysis
To analyze and evaluate flow trajectory and shear stress parameters, finite element analysis (FEA) was used for modeling and simulation. FEA has the benefit of applying and analyzing fluid dynamic parameters on a defined geometry. Through FEA, a fluid can be applied within the microJoint geometry and the software will report computationally simulated data regarding shear stress gradient and fluid flow velocity. An analysis of this data can assist us to understand how these parameters have affected the generation and maintenance of the microtissues.
In this research, we hypothesize that the medium perfusion parameters used for microJoint and HTP-microJoint culture lead to suitable flow rate and shear stress for the maturation and phenotype maintenance of the tissues.
2. Methods
2.1 MicroJoint and HTP-microJoint chip geometry
The microJoint model was developed using Solidworks. The microJoint model is a rectangular prism with the dimensions of 56 mm by 36 mm by 14.75 mm. It consists of 12 cylindrical chambers, which each are 14.75 mm in height and have a diameter of 8 mm, to hold microJoint tissue scaffolds that are 4.75 mm in height and 3.00 mm in diameter, and allow for media flow on the top and bottom of the scaffold (Figure 1A). The HTP-microJoint model was developed utilizing Solidworks and consists of a rectangular prism geometry that has dimensions of 27 mm by 5.5 mm by 1.5 mm, containing 3 combined rectangular prism chambers, with a tissue scaffold placed in the middle of each of these chambers with dimensions of 5 mm by 2 mm by 1.5 mm, allowing for media flow on the top and bottom of the scaffolds (Figure 1B).
Figure 1. Geometry of MicroJoint Chips. Diagram identifying the size of the microJoint chips and microtissue. (A) MicroJoint chip geometry and (B) HTP-microJoint chip geometry and section view to show inside chambers with tissue scaffold area labeled in blue and measurements in mm. Inlets identified with arrows pointing in and outlets indicated with arrows pointing out. 2.2 FEA parameters
FEA was conducted to simulate fluid flow in the microJoint model using Solidworks. The two volumetric flow rates utilized for microJoint culture, 12 mL/s and 0.0167 mL/s, were used in the simulations as these were medium perfusion parameters shown to have successful tissue phenotype maintenance in our previous lab studies [4]. DMEM with 10% fetal bovine serum (FBS) was simulated to flow in the top inlets, while DMEM without FBS was simulated to flow in the bottom inlets. DMEM with and without 10% FBS dynamic viscosity and density values were obtained from previous literature and used to develop user-defined fluids for the simulation [5]. Outlet conditions were set to be environmental pressure. The temperature was set at 37 °C, the incubator temperature, and force of gravity was applied. Global points for the simulation were set as average shear stress and average velocity. An FEA was also conducted on the HTP-microJoint model to simulate fluid flow. Fast and slow flow simulations had inlet volumetric flows of 3.16 mL/s and 0.004 mL/s, respectively. The user-defined fluids for the microJoint simulation were
used for this simulation as well, along with identical outlet conditions and temperature to the microJoint simulation. Global points for the HTP-microJoint were also set as average shear stress and average velocity. Solidworks was used on both chip geometries to automatically develop a suitable mesh for the geometry. 2.3 Fluid mechanics assumptions
Laminar flow was selected to represent the fluid flow within both microJoints as the flow channel and fluid velocity for both chips in all simulations were relatively small. Thus, giving Reynolds numbers less than 2300 (Table 1), allowing for the assumption of laminar flow. The average fluid velocity v was calculated with the following equation 1:
v = Q/A (Equation 1)
With Q representing volumetric flow rate, A representing the area the fluid is passing through. And the Reynolds number, Re, was calculated with the following equation 2: Re = ρuD/m (Equation 2)
With ρ representing the density of the fluid, u representing the flow velocity, D representing the diameter of the inlet, and m representing dynamic viscosity of the fluid.
MicroJoint Chip Volumetric Flow Rate
(mL/s)
MicroJoint 12
Fluid Velocity
(mm/s)
Inlet Reynolds Number
4.23 Top 8.71
Bottom 10.9
0.0167 0.006 Top 0.01
Bottom 0.01
HTP 3.16 15.8 Top 8.57
Bottom 10.8
0.004 0.02 Top 0.01
Bottom 0.01
Table 1. Calculated Fluid Velocity and Reynolds Number for MicroJoint Chips
The continuum hypothesis is also assumed true for this simulation as the calculated Knudsen number is less than 0.1 [6]. The Knudsen number, kn, was calculated with equation 3:
kn = λ/L (Equation 3)
Where kn represents the Knudsen number, l represents the mean free path of the molecule and L is the characteristic length, which was determined to be the diameter of the inlet. The mean free path of water was used (l = 0.31 nm) [6] as cell culture medium is primarily made of water. Thus, a kn value of 0.0000016 was obtained for the microJoint, allowing for the assumption of the continuum hypothesis along with no-slip boundary conditions [6]. Although DMEM has differing properties from water, with higher dynamic viscosity and density, it is assumed that due to the higher dynamic viscosity, DMEM would have a smaller mean free path than water. Therefore, the real kn value would be less than 0.0000016, which still allows the assumption of the continuum hypothesis and no-slip boundary conditions. Furthermore, although the HTP-microJoint had smaller inlets in comparison to the microJoint, the kn value for the HTP-microJoint is still less than 0.1.
3. Results
The fast media flow for the microJoint led to an average shear stress of 190 mPa for the top scaffold surface and an average shear stress of 115 mPa for the bottom surface (Figure 2A), with a maximum shear stress of 446 mPa and 275 mPa for the top and bottom surfaces, respectively (Figure 3A). The slow media flow for the microJoint led to an average shear stress of 0.1264 mPa, a maximum of 0.619 mPa for the top scaffold, and an average of 0.161 mPa and a maximum of 0.389 mPa for the bottom of the scaffold (Figure 2A, 3B). The HTP-microJoint had a maximum shear stress of 0.05 Pa and an average shear stress of 0.02 Pa during fast flow (Figure 4A, Figure 2B) and a maximum of 55.1 and an average of 25.2 mPa during slow flow (Figure 4B, Figure 2B). Fluid velocity in both microJoint and HTP-microJoint had a minimum fluid velocity of larger than 0 under all flow conditions, indicating no stagnant areas in the chips (Figure 2C).
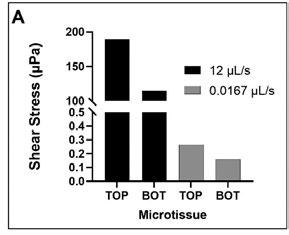
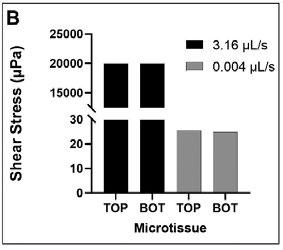
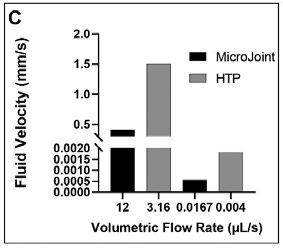
Figure 2. MicroJoint chips characteristics. Values of average shear stresses and flow rates in both microJoint chips have been reported to enhance tissue development (A) Average shear stress in the microJoint chip at 12 mL/s and 0.0167 mL/s. (B) Average shear stress in the HTP-microJoint chip at 3.16 mL/s and 0.004 mL/s. (C) Average fluid velocity in both chips in fast flow mode (12 and 3.16 mL/s) and slow flow mode (0.0167 and 0.004 mL/s 51
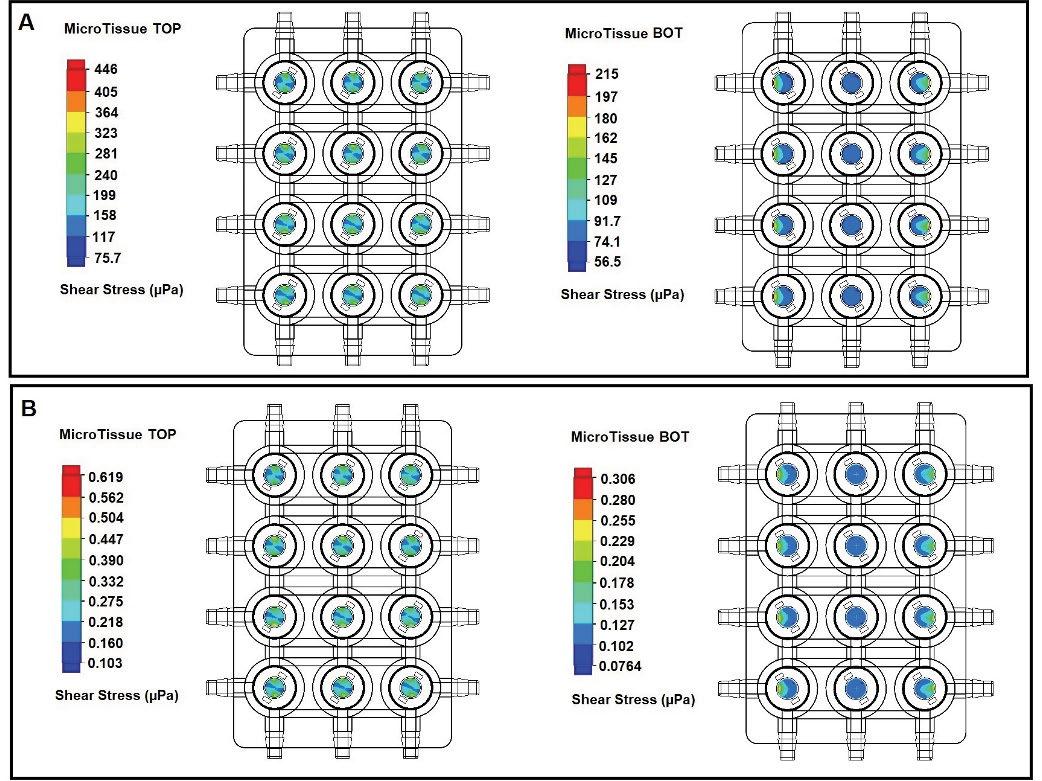
Figure 3. Heat map of microJoint. Fluid induced shear stress undergone by the microJoint microtissue are not large enough to induce cell death and instead assist in tissue maturation. Heat map summarizing shear stress in the microJoint chip at 12 mL/s (A) and 0.0167 mL/s (B) flow.
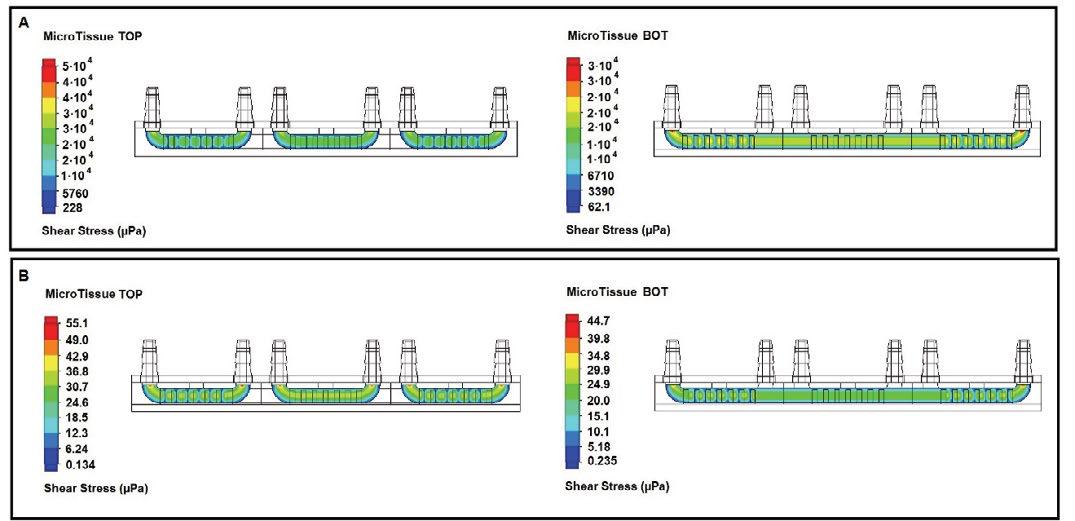
Figure 4. Heat map of HTP-microJoint. Fluid induced shear stress undergone by the HTP-microJoint microtissue are not large enough to induce cell death and instead assist in tissue maturation. Heat map summarizing shear stress in the HTP-microJoint chip at 3.16 mL/s (A) and 0.004 mL/s (B) flow.
4. Discussion
It has been reported that fluid induced shear stresses of over 1 Pa could induce cell apoptosis [6]. Both microJoint chips applied a fluid induced shear stress of less than 1 Pa onto the tissues, indicating that the chips would not cause cell death [7]. Instead, it may enhance osteogenic and chondrogenic properties. For example, the average shear stress for the top scaffold surface during 0.012 mL/s flow for the microJoint has been reported to be within the range that was shown to increase the expression of osteogenic marker genes, such as alkaline phosphatase (ALP), osteocalcin (OCN), collagen I (COL1), and runt-related transcription factor 2 (RUNX2) [7]. Osteogenic proteins ALP, fibronectin (FN), fibronectin receptor (FNR) and transforming growth factor beta-1 (TGF-β 1) are also seen to be upregulated for human osteoblastic cells that undergo fluid induced shear stresses of 1 to 63 mPa [8], a range that includes the average shear stress in the HTP-microJoint during 0.004 mL/s flow. Furthermore, the maximum shear stress of 0.05 Pa in the HTP-microJoint during 3.16 mL/s flow has been shown to increase cytosolic calcium ion concentration in osteoblasts [8]. Finally, a fluid induced shear stress of 0.02 Pa, which is the average shear stress of the HTP-microJoint during 3.16 mL/s flow, is within the range of fluid induced shear stress that has been reported to increase the expression of collagen, glycosaminoglycan (GAG), and proteoglycan, all of which are essential components in the extracellular matrix (ECM) of cartilage [9].
5. Conclusions
Both microJoints were shown to have shear stresses on the scaffold surface that would increase or assist the maturation of tissues in microJoint. The average fluid velocities in both microJoints show no areas of stagnant media in the microJoint chamber, allowing for sufficient growth factor and nutrient diffusion while enabling tissue crosstalk via the shared bottom flow. Lastly, the flow trajectory within the microJoint enables tissue crosstalk, mimicking tissue interactions seen in the native joint.
There are some limitations of this study. First, this study was based on the simulation and results will have to be verified in the actual microJoint in future research. Secondly, we have not considered how the loading that joint must bear will affect the results.
In the future, we will continue to develop this platform to enhance its clinical relevance. First, we will introduce a new component into the current model to study the influence of mechanical loading on tissue health and will simulate the flow with the presence of mechanics. Second, we will test some known drugs in microJoint to investigate if this novel system is able to precisely predict toxicity and efficacy in humans. Finally, we will use patients-derived cells to fabricate to microJoint, addressing the need of precision medicine in treating OA.
6. Acknowledgements
Summer Research Internship (E.L.) funded by the Swanson School of Engineering and the Office of the Provost. This research is in part supported by the National Institutes of Health (UH3TR002136)
7. References
[1] Nelson A. E. (2018). Osteoarthritis year in review 2017: clinical. Osteoarthritis and cartilage, 26(3), 319–325. [2] He, Y., Li, Z., Alexander, P. G., Ocasio-Nieves, B. D., Yocum, L., Lin, H., & Tuan, R. S. (2020). Pathogenesis of Osteoarthritis: Risk Factors, Regulatory Pathways in Chondrocytes, and Experimental Models. Biology, 9(8), 194.
[3] Makarczyk M, Gao Q, He Y, Li Z, Gold M, Hochberg M, Bunnell B, Tuan R, Goodman S, Lin H. Current Models for the Development of Disease-Modifying Osteoarthritis Drugs. Tissue Engineering. [4] Li Z, Lin Z, Lopez MR, O’Donnell B, Li X, Ian J. Moran IJ, Alexander PG, Goodman SB, Bunnell BA, Lin H, Tuan RS. Organ-on-a-chip System for the Modeling of Synovial Joint Pathologies. ORS 2019 Annual Meeting Paper No. 0148 [5] Poon C. (2005) Measuring the density and viscosity of culture media for optimized computational fluid dynamic analysis of in vitro devices. [6] Rapp B, (2017) Chapter 9 – Fluids. In Micro and Nano Technologies, Microfluidics: Modeling, Mechanics and Mathematics. (pp 243-263). Elsevier. [7] P. Wang, P. P. Guan, C. Guo, F. Zhu, K. Konstantopoulos, Z. Y. Wang, Fluid shear stress-induced osteoarthritis: roles of cyclooxygenase-2 and its metabolic products in inducing the expression of proinflammatory cytokines and matrix metalloproteinases, FASEB J. 27(2013) 4664-4677. [8] C. Wittkowske, G.C. Reilly, D. Lacroix, C.M. Perrault, In Vitro Bone Cell Models: Impact of Fluid Shear Stress on Bone Formation, Front. Bioeng.
Biotechnol. 4(2016) 87. [9] N. Sharifi, A.M. Gharravi, Shear bioreactors stimulating chondrocyte regeneration, a systematic review. Inflamm. Regener. 16 (2019) 39.