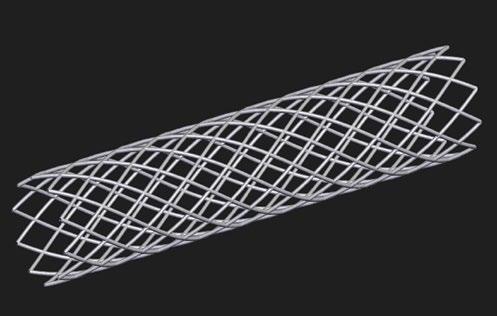
8 minute read
u Finite element analysis of stents under radial compression boundary conditions with different material properties
Finite element analysis of stents under radial compression boundary conditions with different material properties
Yaoyao Xua , Jonathan P. Vande Geestb
aDepartment of Mechanical Engineering and Material Science, bDepartment of Bioengineering and Department of Mechanical Engineering and Material Science
Yaoyao Xu Yaoyao Xu is a mechanical engineering undergraduate at Swanson School of Engineering. Her current interests are control, finite element analysis, and computational modeling. She plans to pursue a master’s degree in control after graduation.
Dr. Jonathan Vande Geest is a Professor in the Department of Bioengineering, Department of Mechanical Engineering and Material Science, the Department of Ophthalmology, the McGowan Institute for Regenerative Medicine, the Louis J. Fox Center for Vision Restoration, and the Vascular Medicine Institute at the Jonathan P. Vande Geest, Ph.D. University of Pittsburgh. He received his BS in Biomedical Engineering from the University of Iowa in 2000 and his PhD in Bioengineering from the University of Pittsburgh in 2005. Dr. Vande Geest began his career at the University of Arizona in the Department of Aerospace and Mechanical Engineering and joined the University of Arizona’s Department of Biomedical Engineering in 2009. Dr. Vande Geest returned to the University of Pittsburgh in January of 2016.
Significance statement
By simulating the reactions of the cardiovascular stents in-vivo with radial compression acts as the boundary conditions, the relationships between the displacements, material stiffness, and the maximum principal stress could be found, which provides references to the stent design when large deformation causes problems to the performance of the stent.
Category: Computational Research
Keywords: Simulation, Stent, Abaqus,
Radial compression, Stiffness
Abstract
The purpose of this work is to begin to establish a computational testing platform to assess the compressive behavior of magnesium alloy stents. This provides references to the stent design when large deformation causes problems to the performance of the stent. According to the results, the sensitivity of peak maximum principal stress to changes in imposed displacement increased as the stiffness of the stent increased. This sensitivity became nearly linear in both stiffness and imposed displacement when these values were near their maximum imposed values.
1. Introduction
The incidence of the cardiovascular and cerebrovascular disease has become higher and higher in recent years and has become the main threat to human life and health [1]. About 80% of patients with the cerebrovascular disease have the ischemic stroke, the main cause of which is the narrowing of blood vessels [2]. A cardiac stent is used to treat narrowed or blocked coronary arteries. Stent restenosis is a major problem resulting in device and treatment failure and often requires subsequent reintervention.
The method of finite elements analyzes the structural characteristics that affect the support strength and safety of vascular stents. Through mechanical simulation and numerical study of the releasing and operating process of vascular stents in the stenosis model, the relevant factors that influence the effect of stent expansion can be identified, which can be provided to the physician when using stent implantation as a valuable reference. Previous research has found that restenosis within balloon-expandable endovascular stents may occur as a result of radial compression [3], and radial compression acts as one of the common boundary conditions for cardiovascular stents in-vivo [4]. Based on Hooke’s law, we assume that the maximum principal stress of the stent is inversely proportional to material stiffness with the same displacement, and as the displacement increases, the stress will also increase. The purpose of this work is to begin to establish a computational testing platform to assess the compressive behavior of magnesium alloy stents. This provides references to the stent design when large deformation causes problems to the performance of the stent.
2. Methods
2.1 Materials and Modeling
The stent geometry showed in Figure 1 was created in SolidWorks using an open-source cardiovascular stent geometry as the starting point. The diameter of the stent was created to be 3.2mm and the length is 16mm [5].
Figure 1. Stent geometry
After importing the stent geometry into Abaqus CAE as a part, several parameters are defined to simulate the radial compression of the stent. The geometry of the stent did not change for any of the simulations. Magnesium represents a very attractive material for biodegradable stents since the process of its natural and gradual dissolution into the human body by a corrosion process would prevent restenosis risks and would allow the progressive transmission of the mechanical load to the surrounding tissues after several months of service [6]. Therefore, the stent material is defined to be mechanical linear elastic material of AZ31-O magnesium alloy. The Young’s Modulus will be changed as the parameter in a specific range. The model uses a second-order tetrahedral element. The approximate global element seeding size was 1. To simulate radial compression, four sets of nodes are imposed different boundary conditions. The first two sets are the left and right ends of the stent. The third set is the bottom part of the stent by selecting the nodes with the minimum Y position. The fourth set is the top part of the stent by selecting the nodes with the maximum Y position. The first two sets have fixed longitudinal displacement (Z direction) to prevent the stent from moving in the longitudinal direction. The third set is fixed in all 3 directions. The fourth set is “compressed” by imposing a negative Y displacement. The output was the peak maximum principal stress in the model. The imposed Y displacement and Young’s Modulus were varied in order to understand how the peak stress nonlinearly changes with these two parameters. A mesh convergency study was performed prior to starting the sensitivity study. 2.2 Data Processing
The negative displacement imposed on the top set is defined by how many percent of the diameter of the stent. There are four groups of displacements, 20%, 40%, 60%, and 80% of the initial diameter. In each group of displacements, four tests with different material stiffness were made. The four tests have the corresponding Young’s modulus to be 0.1MPa, 100MPa, 5000MPa, and 45000 MPa. The Poisson’s ratio was fixed to be 0.3 for all tests. The total sixteen tests give sixteen maximum principal stress as the result of the compression which was visualized using a contour plot. The maximum principal stress for each test was collected and used in this plot.
3. Results and Discussion
Figure 2 below displays the original stent and the deformed stent following compression.
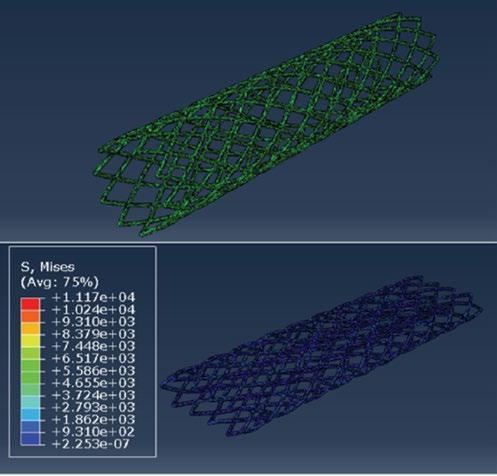
Figure 2. Upper part is the initial stent geometry; the lower part is the deformation of the stent.
Table 1 below displays the maximum principal stress of different material properties and compression levels. Different mesh densities were tested with the same model and boundary conditions to make sure that the results in this table were mesh converged.

Table 1. Maximum Principal Stress of The Whole Stent
Figure 3 is the plot of the relationship between the data in Table 1. The color changes represent the changes in stress with corresponding material and imposed Y displacement, and the values in the plot represent the maximum principal stress of the stent in MPa.
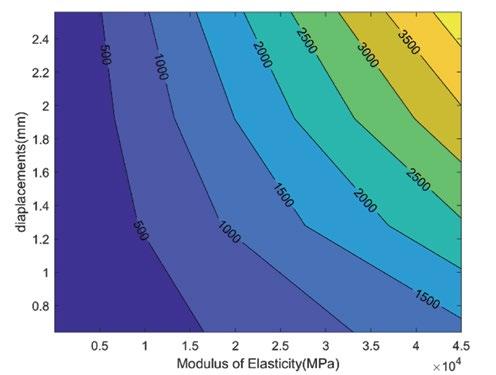
Figure 3. Different color represents different maximum principal stress value in MPa
According to the output from the simulation, the top part and bottom part of the stent will suffer the highest principal stress, but the specific element which has the highest stress could change with the changes of each parameter. The maximum principal stress within the stent under radial compression is proportional to both the modulus of elasticity and the percentage of radial displacement.
The sensitivity of peak maximum principal stress to changes in imposed displacement increased as the stiffness of the stent increased as expected. This sensitivity became nearly linear in both stiffness and imposed displacement when these values were near their maximum imposed values. Our results indicate that stent designs utilizing stiffness values lower than 1 to 1.5 MPa will display low peak maximum stress values. The current work will need to be extended in the future to include different geometries as well as including a model depicting the degradation of the biodegradable magnesium material [7].
4. Conclusion
The methods in this study established a computational testing platform to assess the compressive behavior of magnesium alloy stents, and successfully analyzed the stiffness of materials that affects the reactive stress of vascular stents. Specifically, the results of this study showed that the sensitivity of peak maximum principal stress became nearly linear in both stiffness and imposed displacement when these values were near their maximum imposed values. Chiefly, though, the significance of this study is to find the relationship between the maximum principal stress, the displacement, and the material stiffness for stents under radial compression. Importantly, the results provide references to the stent design when large deformation causes problems to the performance of the stent.
5. Acknowledgments
This research is supported by the Center for Medical Innovation (CMI) (Grant No: F 274), and funded by Swanson School of Engineering, University of Pittsburgh.
6. References
1. Levi F, Lucchini F, Negri E, et al Trends in mortality from cardiovascular and cerebrovascular diseases in Europe and other areas of the world Heart 2002; 88:119-124. 2. ZHOU ZM, YIN Q, XU GL, et al. Influence of vessel size and tortuosity on in-stent restenosis after stent implantation in the vertebral artery ostium[J]. Cardiovascular and
Interventional Radiology, 2011, 34(3): 481-487. 3. Rosenfield, K., Schainfeld, R., Pieczek, A., Haley, L., & Isner, J. (1997). Restenosis of Endovascular Stents From Stent Compression. Journal Of The American College Of Cardiology, 29(2), 328-338. DOI: 10.1016/s07351097(96)00498-6 4. Sullivan, S.J.L., Dreher, M.L., Zheng, J. et al. Effects of Oxide Layer Composition and Radial Compression on Nickel Release in Nitinol Stents. Shap. Mem. Superelasticity 1, 319–327 (2015). https://doi.org/10.1007/s40830-0150028-x 5. LEE, C. H. et al. Differential cutoff points and clinical impact of stent parameters of various drug-eluting stents for predicting major adverse clinical events: An individual patient data pooled analysis of seven stentspecific registries and 17,068 patients. International Journal of Cardiology, [s. l.], v. 282, p. 17–23, 2019. DOI 10.1016/j.ijcard.2019. 6. Farè, Stefano, Ge, Qiang, Vedani, Maurizio, Vimercati, Gianmarco, Gastaldi, Dario, Migliavacca, Francesco, Petrini, Lorenza, & Trasatti, Stefano. (2010). Evaluation of material properties and design requirements for biodegradable magnesium stents. Matéria (Rio de Janeiro), 15(2), 96-103. 7. Moravej, M.; Mantovani, D. Biodegradable Metals for Cardiovascular Stent Application: Interests and New Opportunities. Int. J. Mol. Sci. 2011, 12, 4250-4270