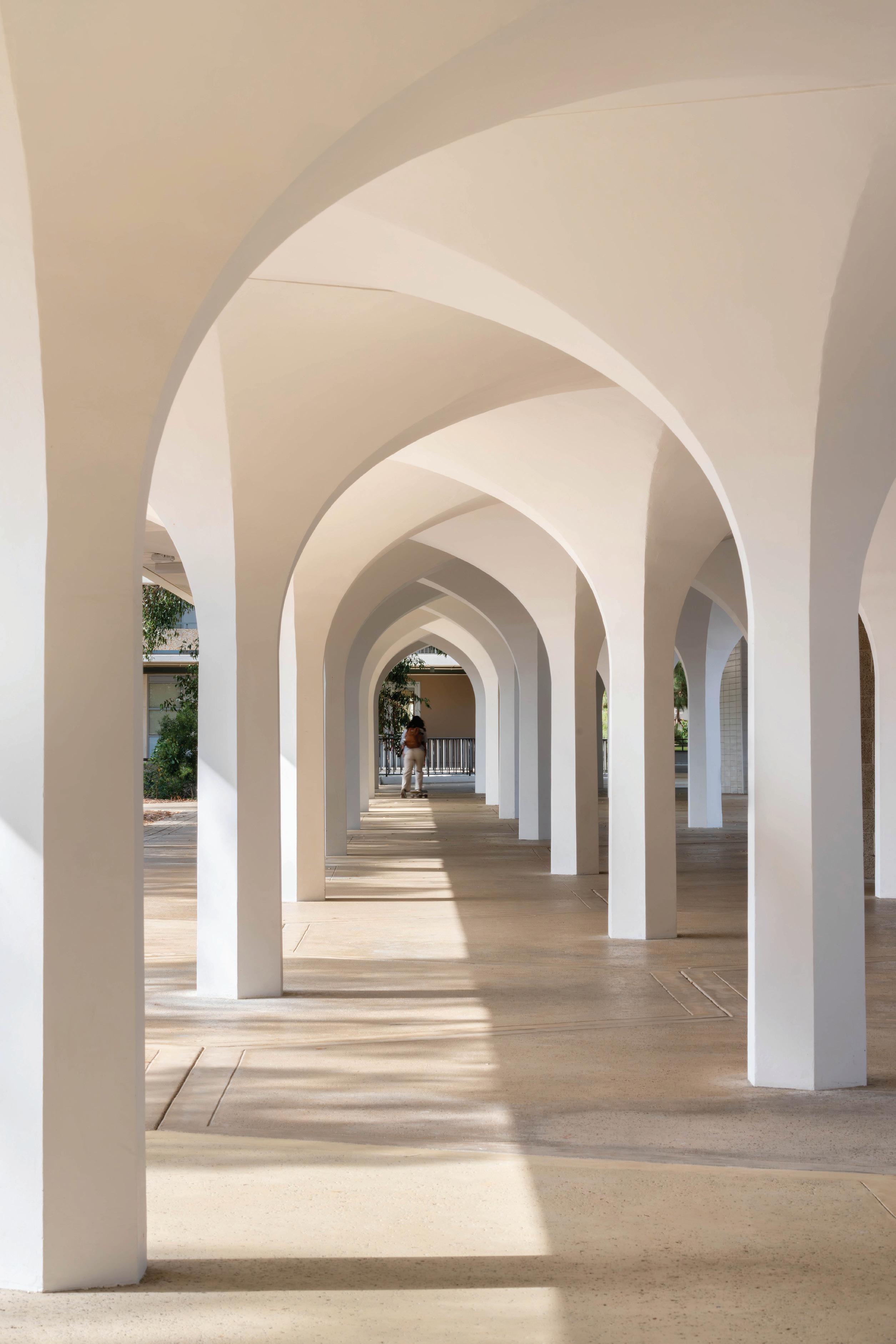
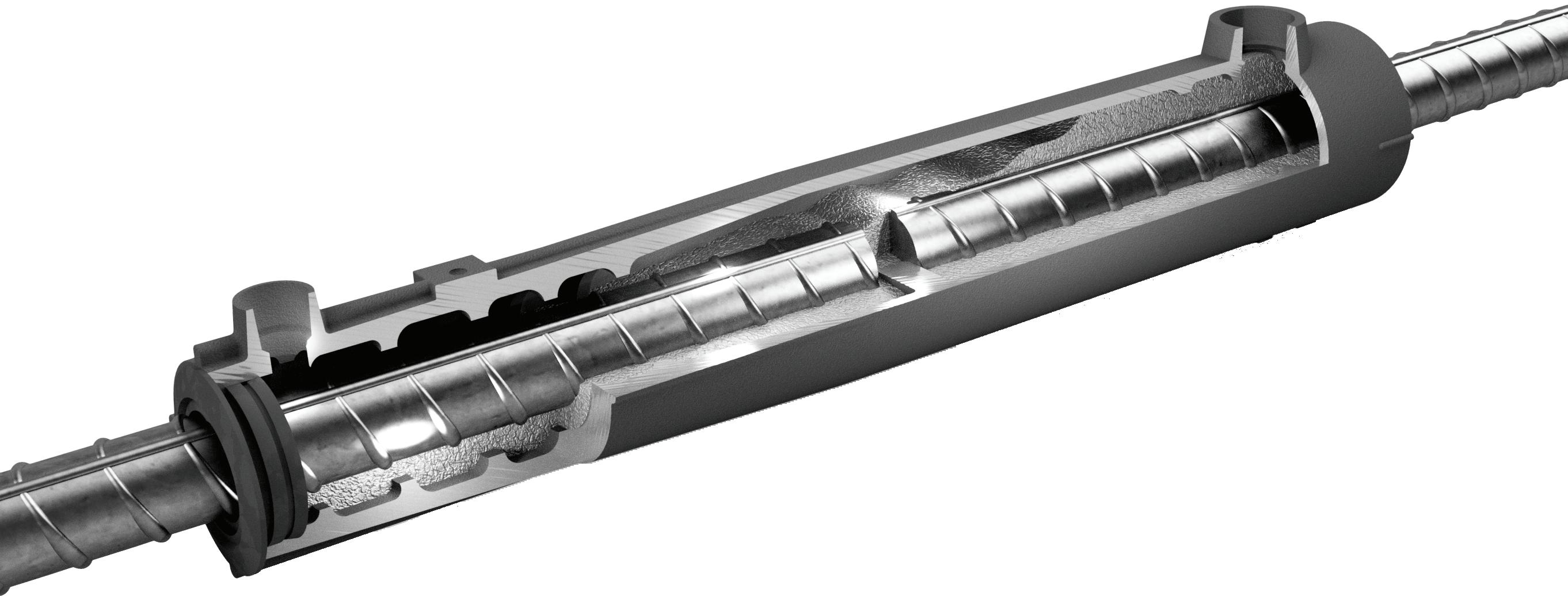
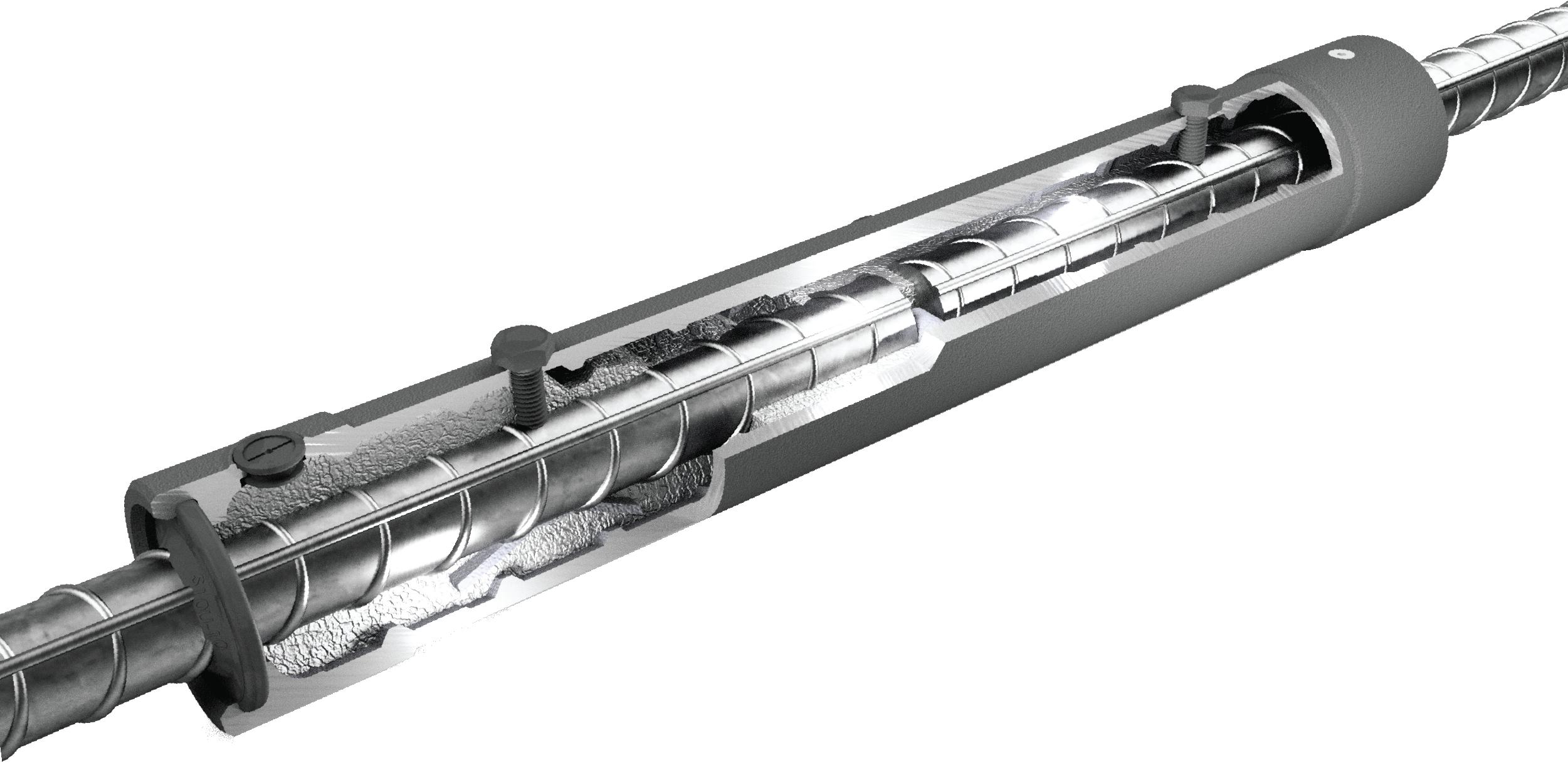

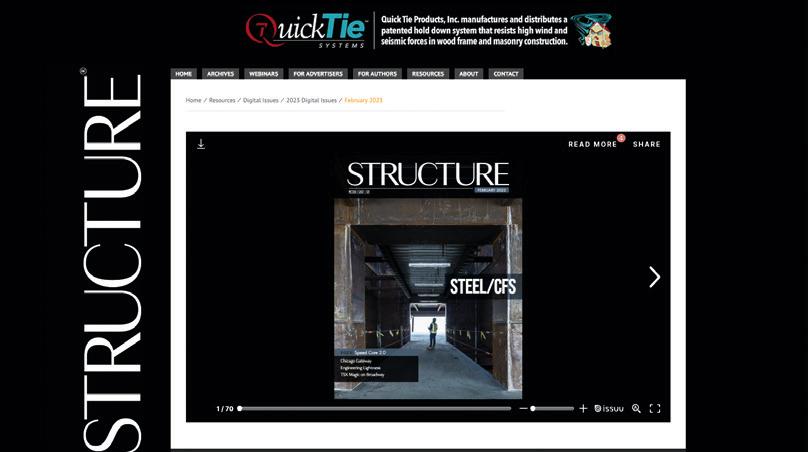
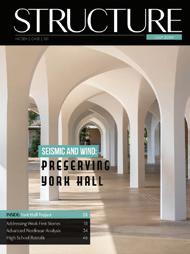
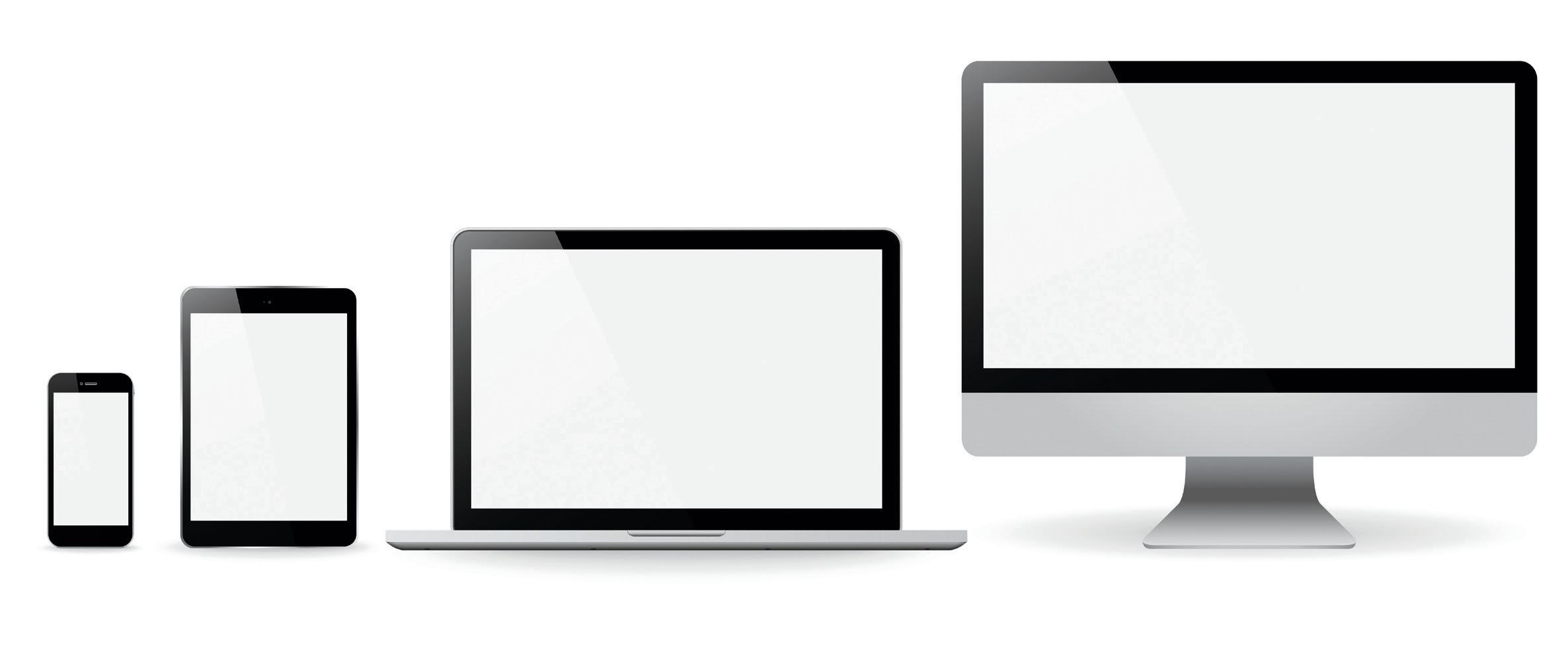
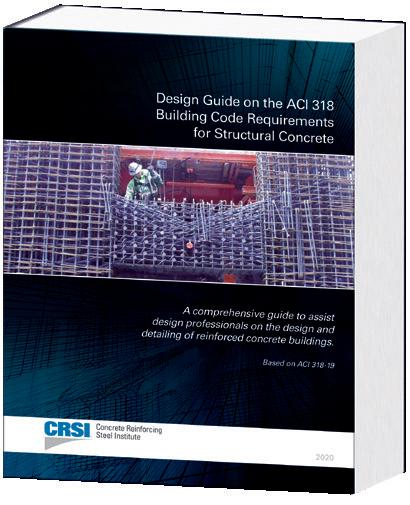
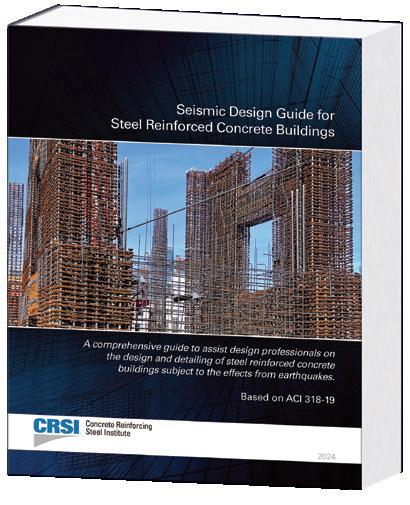
subscriptions@structuremag.org
EDITORIAL BOARD
Chair John A. Dal Pino, SE Claremont Engineers Inc., Oakland, CA chair@STRUCTUREmag.org
Marshall Carman, PE, SE Schaefer, Cincinnati, Ohio
Erin Conaway, PE AISC, Littleton, CO
Sarah Evans, PE Walter P Moore, Houston, TX
Linda M. Kaplan, PE Pennoni, Pittsburgh, PA
Nicholas Lang, PE
Vice President Engineering & Advocacy, Masonry Concrete Masonry and Hardscapes Association (CMHA)
Jessica Mandrick, PE, SE, LEED AP Gilsanz Murray Steficek, LLP, New York, NY
Jason McCool, PE
Cool Country Engineering PLLC,Cabot, AR
Brian W. Miller
Cast Connex Corporation, Davis, CA
Evans Mountzouris, PE Retired, Milford, CT
Kenneth Ogorzalek, PE, SE KPFF Consulting Engineers, San Francisco, CA (WI)
John “Buddy” Showalter, PE
International Code Council, Washington, DC
Eytan Solomon, PE, LEED AP Silman, New York, NY
Jeannette M. Torrents, PE, SE, LEED AP JVA, Inc., Boulder, CO
Executive Editor Alfred Spada aspada@ncsea.com
Managing Editor Shannon Wetzel swetzel@structuremag.org
Production production@structuremag.org
MARKETING & ADVERTISING SALES
Director for Sales, Marketing & Business Development
Monica Shripka Tel: 773-974-6561 monica.shripka@STRUCTUREmag.org
nucor.com/madeforgood
By Bryan Seamer, PE, SE
The award-winning project updated the structural systems of the University of California San Diego building while preserving its architectural heritage.
By Jason Armes, SE; Gina Carlson, SE; and Leo Panian, SE
For many existing buildings, a targeted and more cost effective solution can be found through the use of advanced nonlinear analysis procedures.
By Nik Blanchette, SE; Steve Heyne, SE; and Chris Warner, SE
A charter high school in Northern California planned to make a large commercial building its new permanent home, but first the structure needed to be given a seismic upgrade without disrupting the other tenants.
During the seismic rehab project, a new basement story was created along
Introducing the Simpson Strong-Tie ® Yield-Link ® brace connection ( YLBC).
Now you can add more resilience to structural steel projects. Ideal for new builds or retrofit work, the YLBC connects braced frames in structural steel buildings. It has bolted connections that simplify design. During extreme seismic or high-wind events, the YLBC isolates damage to the connection only — allowing the braced frame to remain intact. In addition, our Yield-Link technology tools make it easy to plan, model and document complete designs according to your unique specifications. Like other Simpson Strong-Tie products, the YLBC is widely available and backed by our industry-leading service and technical support.
Design your next project with all of our structural steel solutions. To learn more, visit go.strongtie.com/yieldlinkbraceconnection or call (800) 999-5099.
a Recruiting Tactic: Find a Purpose By Anthony LoCicero, PE
Considering the Potential Impact of Tsunamis on Structures By Steven Baldridge, PE, SE, LEED AP
Splices (Couplers) in Reinforced Concrete Constructions By Dr. N. Subramanian, Ph.D., FNAE
By Justin Moresco, PE, and David Mar, SE
Jennifer Goupil, PE
By John “Buddy” Showalter, PE, and Sandra Hyde, PE
By John A. Dal Pino, SE
19th Century Mississippi River Bridges: Prairie Du Chien Floating Bridge 1874
By Dr. Frank Griggs, Dist. M. ASCE
By Anthony LoCicero, PE, LEED AP
Recruiting and retaining top engineering talent has never been more challenging. Construction is booming across many markets, leading to strong demand for engineering services. Yet the pool of engineering candidates remains limited across disciplines, including structural.
Retirements are on the rise. Students are choosing among a growing number of technical career pathways. To counteract a potential workforce shortfall, traditional recruitment and retention strategies, such as compensation, advancement opportunities, and work-life flexibility, are important. But often, it’s not enough.
The American Society of Civil Engineers last year partnered with the National Governors Association to identify strategies for counteracting engineering workforce challenges across public and private sectors. I advise my structural colleagues to familiarize themselves with the report’s short-term as well as long-term strategies. While all are important, for this column, I want to highlight one in particular: “Praising and promoting the technical achievements of infrastructure.”
matter our area of focus, we should all find some way to draw connections between our structural designs and these important challenges.
The following examples are just a few of the topic areas where structural design innovations will have far-lasting implications for social, environmental, and economic change.
Resilience. Extreme weather is becoming more frequent and costly. Climate change is antici-
transition is cleaning up tailpipe emissions at a time when transportation is becoming the largest contributor to climate change. Structural engineers play a major role in EV enablement. Electric vehicle supply equipment (EVSE) and on-site power generation technologies are being attached to building roofs through canopy configurations. Roofs will need to be strengthened or new pantograph systems created from scratch. Charging systems may also require thoughtful cable management approaches such as trench-based cable reels, overhead conductors, or mounted cables installed on mobile equipment.
New generations of engineers want to be involved in bringing about solutions for society’s most complex challenges: how to adapt to a warmer world, transition to promising new technologies, overcome growing economic disparities, etc.
The ASCE and NGA report makes the correct point that, “too often in engineering and public infrastructure, workers are scorned for public failures and only rarely praised publicly for their success.” As any structural engineer knows well, a successful structural design goes largely unnoticed until something fails. To better recruit and retain talent, the structural engineering field must do better at celebrating individual and collective contributions toward high-profile projects, while drawing connections between structural designs and a project’s social, environmental, and economic benefits.
It is not uncommon for structural engineering recruits to hear how a building’s designs are critically important to occupant safety. Our industry’s high standards of engineering excellence will always be driven by the need to maintain public safety. But when telling the story of the structural profession, safety may no longer be sufficient. New generations of engineers want to be involved in bringing about solutions for society’s most complex challenges: how to adapt to a warmer world, transition to promising new technologies, overcome growing economic disparities, etc. No
pated to increase the intensity of heavy rain and hurricane events, while sea level rise is expected to amplify the impacts of storm surge. Flood risks are disproportionately expected to impact marginalized communities. In areas exposed to new risks, structural enhancements will become necessary to uphold the resilience of critical infrastructure. Tools may include new flood walls and other hardening measures. Depending on the application, these measures may be scaled to protect a single piece of equipment, a building, or for many city blocks.
Sustainability. A structure’s embodied carbon is an accounting of the greenhouse gas emissions associated with construction materials, including material production and installation. Embodied carbon is estimated to make up roughly 11 percent of energy-related carbon emissions worldwide. A growing number of jurisdictions now require life cycle assessments of a development’s embedded carbon, material-specific limits for carbonintensive building materials such as concrete and steel, or declarations of a design package’s carbon footprint. Structural design teams are being called upon to calculate—and lower—the Embodied Carbon Order of Magnitude (ECOM) for a project’s structural materials.
Electrification. The electric vehicle (EV)
Adaptive Conversions. With office vacancies reaching a 30-year high and land available for new construction often limited, developers are reimagining empty office buildings through adaptive reuse. Revitalizing these spaces can help revive downtown neighborhoods and alleviate housing shortages. Conversions have repurposed offices into apartments, hightech laboratories, and educational facilities. In the process, structural design teams are removing walls to access natural light, strengthening floors to hold heavy research equipment, reducing vibrations to accommodate hyper-sensitive lab equipment, and supporting new mechanical, electrical and plumbing systems.
If you ask ChatGPT, a structural engineer’s typical projects include the design of buildings, bridges, and utility structures. But what it means to be a structural engineer is changing. A broader definition is necessary to reflect the increasing complexity—and importance—of structural innovations. In doing so, we can elevate the profession and establish the field as one in which young professionals can pursue both their personal aspirations and technical interests. ■
Full references are included in the online version of the article at STRUCTUREmag.org
Conscientious design of buildings and infrastructure at risk of a tsunami impact can reduce the extent of the destruction. By
Steven Baldridge, PE, SE, LEED AP
With nearly one-third of recorded large tsunamis occurring in Japan, the phenomenon is reflected extensively in the region’s culture and history. Thus, the Japanese word “tsunami,” which means “harbor wave,” is recognized globally for an event that can occur along any coastline.
Tsunamis adjacent to harbors can be very destructive due to several factors, including the presence of ships and containers that can float inland, damaging nearby structures. Many harbors also have a shape both above ground (topography) and below the water’s surface (bathymetry), that can force the incoming tsunami wave to rise higher as it is squeezed into a smaller space.
The risk of the potential devastating effects from tsunamis is not limited to Japan or harbors for that matter. The Pacific coast states and provinces of the U.S. and Canada have both the risk and history of tsunamis. These include deadly tsunamis in Liuya Bay, Alaska, in 1958; Prince William Sound, Alaska, in 1964; and Hilo, Hawaii, in 1946. A deadly tsunami also occurred on the U.S. Atlantic coast in Cape Hatteras, North Carolina, in 1883.
The most common triggering events of tsunamis are earthquakes below or near the ocean floor, but a tsunami can be created by volcanic activity, landslides, undersea slumps, and even meteorite impacts. The Tohoku 9.0 earthquake in March 2011, which is considered one of the most powerful earthquakes ever recorded, occurred on the east coast of Japan and caused a massive tsunami that killed thousands and sent 30-foot waves of water across much of the countryside, destroying entire towns and leveling hundreds of low-rise buildings. The tsunami caused the Fukushima Daiichi nuclear disaster.
Tsunamis are rare but devastating events; as such, strategies to mitigate risk have usually involved horizontal evacuation to areas of naturally occurring high ground outside the tsunami inundation zone. This can be the most efficient and safest way to protect life when there is enough warning. For some coastal communities, additional options
can include a vertical evacuation strategy, utilizing buildings or manmade mounds or hills that have sufficient height to evacuate the level of tsunami inundation and the strength and resiliency needed to resist the effects of tsunami waves.
As coastal populations continue to grow in the U.S. , horizontal evacuation cannot be the only strategy, especially for Risk Category III and IV buildings. Lessons learned from Japan showed that consideration for hardening infrastructure can be critical to recovery. Essential services such as water supply, wastewater treatment, and power facilities, often located near the coasts, can be crippled by the effects of tsunami wave inundation. The Fukushima nuclear disaster, for example, was caused by tsunami damage to the backup generators that were needed for reactor cooling. Emergency facilities and hospitals are additional candidates for hardening.
Tsunamis are categorized by the location of the triggering event and the time it takes the waves to reach a site. A far-source-generated tsunami is one that originates from a source that is far away and takes two hours or longer after the triggering event to arrive. A near-source-generated tsunami originates from a source that is close and could have 30 minutes or less advanced warning. A mid-source-generated tsunami is one that originates from a source that is close to the site of interest but not close enough for the effects of the triggering event to be felt at the site and would be expected to arrive between 30 minutes and two hours after the triggering event.
Wave propagation times from near-source-generated tsunamis can strike suddenly with little to no warning while far-source-generated tsunamis can allow for advanced warning to distant coastal communities. The 2004 Indian Ocean Tsunami, for example, devastated coastal areas both near and far. Coastlines near the tsunami were inundated in as little as 15 minutes, while those far away waited seven hours for the tsunami to reach their shores. On average, 20 tsunamigenic earthquake events occur each year worldwide, with five large enough to generate tsunami waves capable of causing structural damage and loss of life. With the trend toward increased habitation in coastal areas, more populations will be exposed to tsunami hazard.
There is significant uncertainty in the prediction of hydrodynamic characteristics of tsunamis because they are influenced by tsunami waveform and surrounding topography and bathymetry. Although exceptions exist, field research and surveys indicate that tsunamis have the following characteristics:
• The magnitude of the triggering event determines the period of resulting waves, and generally—but not always—the tsunami magnitude and damage potential.
• A tsunami can propagate more than several thousand miles with little loss of energy. This is due to the large wavelengths of tsunamis, which can be more than 100 miles.
• Tsunami energy propagation has strong directivity—it tends to propagate in a direction normal to the major axis of the tsunami force. Direction of approach can affect tsunami characteristics at the shoreline because of the sheltering or amplification effects of other land masses and offshore bathymetry.
• For a locally generated tsunami, the first leading wave is often a receding water level followed by an advancing positive heave or elevation wave. This may not be the case if the coastal ground subsides by co-seismic displacement. For far-source-generated tsunamis, the leading wave is often an elevation wave. This trend may be related to the pattern of seafloor displacement resulting from a subduction-type earthquake.
Tsunami hazard is a measure of the potential for a tsunami to occur at a given site. It also is a measure of the potential magnitude of site-specific tsunami effects, including extent of inundation, height of runup, and velocity of tsunami flow. Tsunami risk is a measure of consequence given the occurrence of a tsunami, which can be characterized in terms of damage, loss of function, injury, and loss of life. Risk depends on many factors including vulnerability and population density.
Evaluation of tsunami risk will depend on several factors including the presence of a tsunami warning system, existence of a local emergency response plan, availability of various evacuation alternatives, the vul nerability of the existing building stock, and location of existing short and long-term shelter.
Evidence points to the ability for multi-story concrete and structural steel structural systems to survive tsunami inundation with little more than nonstructural damage in the lower levels and to continue to support the levels of a building above the inundation depth. This can be attributed in part to these buildings being designed for lateral wind and seismic forces that exceed the force of a tsunami rolling through the lower levels of those buildings. Recent data, including those from the 2004 Indian Ocean Tsunami, 2009 Samoa Tsunami, and 2011 Tohoku Japan Tsunami, where no six-story or higher concrete or steel buildings collapsed due to the tsunami, support these conclusions. Building survivability does, however, vary with construction type. For example, for a given tsunami height, wood or other light-framed construction can experience considerably more damage and frequently be destroyed. In Hawaii, the potential survivability of multi-story buildings is recognized in its tsunami evacuation messaging, informing the public that vertical evacuation in buildings taller than 10 stories is an option if horizontal evacuation is not feasible.
Despite a long history of tsunamis around the world, because they are relatively rare, there has not been good guidance on designing to mitigate their effects. For the U.S., this started to change in 2004 when the Applied Technology Council (ATC) was awarded a contract by the Federal Emergency Management Agency (FEMA) to help develop design guidance for special facilities for vertical evacuation from
tsunamis. That seminal document FEMA P-646 would become the forebearer of a new chapter to ASCE 7-16: Chapter 6 Tsunami Loads and Effects, which includes tsunami design requirements specific to Risk Category III and IV structures.
Several good resources now provide guidance for the design to resist the loads induced by tsunami as noted here:
FEMA P-646 Third Edition—“Guidelines for Design of Structures for Vertical Evacuation from Tsunamis,” 3rd Edition, provides guidance on planning for vertical evacuation from tsunami. This document includes determination of tsunami and earthquake loads along with the structural design criteria necessary to address them, and structural design concepts and other considerations including using existing structures. It provides additional guidance and commentary on the structural design criteria contained in ASCE 7.
ASCE/SEI Standard 7-22—The American Society of Civil Engineers/ Structural Engineering Institute (ASCE/SEI) Standard 7-22 Chapter 6 “Tsunami Loads and Effects” provides minimum requirements for tsunami-resistant design and construction of Risk Category III and IV structures located in a tsunami inundation zone.
ASCE Press—The book “Tsunami Loads and Effects: Guide to the Tsunami Design Provisions of ASCE 7-16” is a good companion document to ASCE 7 and includes detailed commentary and example problems.
While detailed modeling can be performed, the primary method referenced in ASCE 7-22 for determining tsunami flow depth and velocity at a location is Energy Grade Line Analysis (EGLA). This method is based on ASCE Tsunami Design Zone (TDZ) maps which can be found at asce7tsunami.online. The EGLA includes establishing topographic transects, a straight line that cuts through the topography to determine the impact of topography in a given direction of tsunami flow. The EGLA is based on looking at three transects where the center transect is perpendicular to the orientation of the shoreline with the other two located +/22.5 degrees from the principal inflow direction. This initial step is based solely on topography and does not consider the potential impact of adjacent structures on flow velocity. ASCE 7-22 allows the velocity increase that may occur due to adjacent structures to be considered by three possible approaches: two requiring detailed site-specific analysis and one simpler method approximating their impact utilizing a roughness coefficient applied to the EGLA. That coefficient is indicated in ASCE 7-22 Table 6.6-1.
ratio (6.8.7) and in the drag coefficients for individual members in the hydrodynamic calculations. Also included in this section is consideration of potential hydrodynamic forces, including surge uplift on elevated slabs in a structure.
Debris Impact Loads (ASCE 7-22 6.11)—
The force and extent of a tsunami wave not only causes damage to structures, the debris from that damage, along with other floatable objects, can cause additional debris impact forces as those elements continue to move with the wave. These include scenarios such as impact by floating vehicles, tumbling concrete debris, wood logs or utility poles, shipping containers and if near harbors, floating vessels.
Foundation Design (ASCE 7-22 6.12)—
This includes potential scour and slope/ foundation failure. The rushing water can undermine shallow foundations. Deep foundations that have positive connections to the structure they support have a reduced potential for catastrophic impact.
Once the tsunami inundation depth and flow velocity are established, the extensive task of determining structural load criteria can begin. The primary structural impacts that can result in damage to a structure and have specific design requirements include the following:
Hydrostatic Loads (ASCE 7-22 6.9)—These include determining potential loads that might occur from buoyancy, unbalanced lateral hydrostatic forces, residual water surcharge load on walls and floors and hydrostatic surcharge pressure on foundations.
Hydrodynamic Loads (ASCE 7-22 6.10)—These forces are due to moving water and include the global forces on the overall structure along with the local loads on structural components of the structure. Because water-borne debris could potentially accumulate against vertical elements of the structure, consideration is given in both the global forces and individual member loads. This is considered in a minimum closure
CCW Transect
While ASCE 7-22 Chapter 6 is intended specifically for Risk Category III and IV buildings, communities can consider encouraging incorporating tsunami design into conventional buildings in areas with fewer horizontal evacuation options. Unfortunately, this comes with added costs during design and construction. For communities with longer warning times for tsunami, it may not even be necessary to design some low-rise Risk Category III buildings if those buildings do not require design per the Tsunami Risk Category and can’t be used for shelter or there is a well-developed horizontal evacuation plan. That money may be better spent elsewhere.
With recent construction cost inflation conflicting with the growing needs for affordable housing, it is critical that communities look to partner with their builders and designers to offset the costs if additional resiliency measures such as tsunami design are considered for these types of buildings. As all developers of affordable housing will attest to, any increase in regulations or design requirements directly decreases the production of affordable housing units.
Runup Elevation (MWH) 16.75 feet
Include Sea Level Rise Runup Elevation (MWH) 17.02 feet Inundation Depth (MWH) 20.19 feet Flow Velocity 24.25 feet/second
When considering requiring conventional construction to meet design requirements intended for essential facilities, it is critical for those communities to consider ways of keeping added costs to a minimum. FEMA recommends several methods to assist in mitigating the additional costs of this type of construction. These include offering tax incentives, modifying zoning requirements to increase height restrictions and FAR, or applying for federal or state grants.
Since no six-story or higher concrete or steel buildings collapsed in the devastating Tohoku Japan tsunami, it begs the question, “Is it worth using elaborate and time extensive analysis and design for multi-story buildings unless they are essential buildings?” A more cost-effective approach may be to just include tsunami-resistant design concepts in the construction of these buildings.
In performing tsunami design on several projects, Baldridge & Associates Structural Engineering (BASE) found multi-story buildings designed to current codes can meet the intent of ASCE 7-22’s tsunami design requirements without the expense of extensive modeling and additional structural analysis by simply enhancing them with a few structural design concepts, including:
• Provide structural systems that have inherent redundancy and utilize structural integrity concepts. This can include seismically designed cast-in-place concrete systems or structural steel framing, especially those utilizing perimeter moment frames for lateral resistance.
• Incorporate deep foundation systems where possible. For many
coastal locations this may be required anyway.
• Consider making the ground floor as open as possible and detailing non-load bearing walls connections to the structure with little if any overstrength for wind and seismic requirements. The more open the ground floor is, the less load and damage that may occur. A great example of this is podium style construction with open parking at grade level and residential uses starting at the 2nd or 3rd floor levels. With podium construction, the elevated residential levels of a building may not need to consider tsunami requirements at all and can utilize conventional wood or other light framing methods.
• The wild card in tsunami design is the potential for water-borne debris impact loading that might happen on lower-level structural elements. This can be addressed with more robust construction for those structural elements only. This could include increasing column vertical and tie reinforcement at critical concrete columns, concrete encasement of lower-level steel wide flange columns or filling tube columns to make them composite columns.
• Detail slabs to be able to relieve potential buoyancy or uplift forces. This can include areas of slab that can pop out if pressures start to build. ■
Steven Baldridge, SE, PE, LEED AP, is President of BASE, a structural engineering consulting practice. He has participated in natural disaster reconnaissance missions including serving as the EERI team leader in Samoa following its 2009 earthquake and tsunami. He was Project Technical Director for FEMA P646 “Guidelines for Design of Structures for Vertical Evacuation from Tsunamis”, the first U.S. design guide on tsunami loads from which some of this article is based.
Minimize steel tonnage
Minimize seismic detailing
Simplify fabrication
Increase erection speed
Complimentary design assist Resilience through repairability
Complimentary design assist
These best practices will ensure walls are properly reinforced and mechanical splices are staggered in a way to reduce the risk of failure. By
Dr. N. Subramanian, Ph.D., FNAE
Mechanical splicing products (often called couplers) are widely used in the construction industry as an alternative to lap splices when joining two reinforcement bars, especially when there is no room for lap splices, when larger diameter of bars (larger than #11)are used, and in tension members. In addition, the use of mechanical couplers is useful where the reinforcement is congested or space does not allow for the full development length that a splice would require.
Couplers have the following advantages: 1) improved structural integrity thus offering strength and toughness even during seismic events, 2) no reliance on concrete for load transfer, 3) elimination of lap-splice calculations, and 4) reduced material costs. This cost savings can be significant when expensive epoxy coated bars are used, since building codes require up to 50% longer splice laps for these bars than for standard rebars (Hurd, 1998). A few other hidden costs associated with providing lap splices, such as the time spent preparing lap splices and the necessity for additional transverse reinforcement, are also eliminated. (Jacinto, et al., 2023). Additionally, codes such as ACI 318:19, Building Code Requirements for Structural Concrete and Commentary on Building Code Requirements for Structural Concrete(clause 25.5.7.1) require that
Enlarged bar end
Threaded bar area the same as unthreaded bar area
Internally threaded coupler
Internally threaded tapered coupler
Matching tapered bars
Steel sleeve half ontoswaged bars half threaded onto stud
Threaded stud
mechanical splices deliver higher performance than lap splices (typically 125% greater capacity). The use of mechanical couplers results in savings on rebar material and associated costs, including fabrication of lapping rebars. However, this cost advantage may be offset by the cost of couplers.
Several types of mechanical couplers are available-on the market (Table 1). Couplers may be categorized as tension couplers or compression couplers. Unless specified otherwise, tension couplers should always be used. Couplers may also be categorized as in-line couplers, in which the centerline of each spliced bar coincides and offset couplers, where the centerlines have an eccentricity.
Reducer insert-used when bars are of different size
Lock nut
Bar with helical deformations
Internally threaded coupler
Lock nut
Mechanical splices, shown in Figure 1, generally use a threaded annular sleeve, slightly larger than the diameter of the bars, that is placed around the bars at the joint. The sleeves are normally cold pressed against the bars, forcing the ribs of the deformed bars to become embedded into the wall of the sleeve. A sleeve embedment length of only two bar diameters (2db) for each of the two bars may be sufficient to transfer the load of the bar in tension. The connection can also be formed by filling the annular space between the bars and the sleeve with molten metal. Direct threading of bars is avoided to prevent reduction in bar size, and consequently its strength. One solution to this is to increase the size of the ends over a small length by tapering the bars, as shown in Figure 1a. A new type of coupler called a set screw coupler is not threaded, swaged, or metal-filled. Instead, they consist of a steel tube with a series of lock-shear bolts—generally six to eight—and two serrated strips that run the inside length of the coupler, as shown in Figure 1g. The installation of this coupler is carried out as follows: 1) after sliding one length of rebar halfway into the coupler, the bolts are tightened to fit snugly; 2) the second bar is then inserted into the coupler until it butts against the other bar end; and 3) the remaining bolts are tightened to a snug fit. A ratchet or an impact wrench is used to tighten the bolts until their heads shear off, implanting the bolt ends into the rebar and embedding the serrated strips into both the rebar and the interior coupler wall. This type of splicing system does not require any special bar-end preparation and can be used in projects where bars are in place or access is limited. More details about mechanical couplers may be found in ACI 439.3R-2007 Types of Mechanical Splices for Reinforcing Bars, BS 8597:2015 Steels for the reinforcement of concrete - reinforcement couplers
Steel sleeve hydraulically swaged into bars
If seismic or high-wind challenges are important considerations for your next project, Vulcraft and Verco’s steel roof deck and PunchLok II® System is a valuable option to consider. Our team of experts can help you evaluate the variety of value-added sustainable solutions that Vulcraft and Verco offer to ensure that you have the right system in place.
Contact an expert today.
- requirements and test methods, IS 16172:2023
Reinforcement Couplers for Mechanical Splices of Steel Bars in Concrete—Specification, SP 34:1987 Handbook on Concrete Reinforcement and Detailing, and Prakash Rao, 1991. The use of couplers in column reinforcement is shown in Figure 2.
Mechanical butt splices provide superior strength during load transfer. Other advantages include superior cyclic performance and greater structural integrity during seismic events. From the structural standpoint, the most important benefit of mechanical splices is that they ensure load path continuity of the structural reinforcement. Using mechanical butt splices allows the use of larger diameter rebar in a smaller column thereby minimizing congestion. Unlike lapsplices which often extend into the plastic hinge regions (thus violating code requirements), mechanical splices can be easily located outside these high stress regions. In addition, the use of mechanical splicing eliminates the tedious calculations needed to determine proper lap lengths and the possible errors associated with the calculations. Also, mechanical splices are fast to install. However, mechanical splices also have a disadvantage compared to lap splices because of their complex process of installation. That is, rebars need to be prepared before installation by cutting the threads into the rebar so that these threads match the threads of the coupler. This process may need skilled labor, and two additional machines: the forging machine and the thread cutting machine (Damsara and Kulathunga, 2018). The installation must be carefully performed to ensure that the threads are well fitted. Also, the threaded ends of the rebars should be protected from corrosion before installation in order to obtain good fixity with the coupler.
Based on experimental results of testing of offset mechanical splices, shown in Figure 3, Coogler et al, 2008,the following was found:
• Offset splices are not recommended for use with bar sizes greater than #5(16 mm), unless tests indicate to satisfy the performance criteria.
• Offset splices should not be used in applications subject to seismic load reversals.
• Offset splices should be considered as a different category of mechanical splices (having a fatigue limit of 80 MPa).
During 1998, Cagley and Apple made a cost comparison between lap splices and mechanical splices and found that the additional cost of using mechanical butt splices was about 0.2 percent of the total cost of the structure. However, since then the cost of mechanical splices have reduced and the cost advantage of using a coupler instead of lapping may increase with the increase in size of rebar (approximately 11% for #5 bars to 140% for #10 bars) (Damsara and Kulathunga, 2018 and Singh, et al., 2013).
Clause 18.2.7.1 of ACI 318-19 Building Code Requirements for Structural Concrete and Commentary on Building Code Requirements for Structural Concrete (ACI 318R-19), defines two types of mechanical bar couplers, Type 1 and Type 2. According to clause 25.5.7.1 of this code, Type 1 coupler is a mechanical or welded splice that can develop 125%
Coldswaged steel coupling sleeve
Coldswaged steel coupler with threaded ends
Extruded steel coupling sleeve
Hot-forged steel coupling sleeve Grout-filled coupling sleeve Coupler for threaddeformed reinforcing Bars*
coupler
Couplers with standard NC threads Bar size range (mm) 3-18 (10-57) 3-18 (10-57) 4-18 (13-57) 5-18 (16-57) 3-18 (10-57) 6-18 (19-57) 4-18 (13-57)
(10-57) 4-11 (13-36)
of the yield strength of the bars (i.e., 1.25Abf y , where Ab is the area of bar and f y is the yield strength of bar) in tension or compression. Type 2 couplers are those that both conform to the requirement of Type 1 couplers and can develop the specified tensile strength of the spliced bars (clause 18.2.7.1). Thus, the requirements for Type 2 mechanical splices are intended to avoid a splice failure when the reinforcement is subjected to expected stress levels in yielding regions. ACI 318-19 requires using Type 1 only in the locations in which the couplers shall not experience yielding. This is because (per commentary R18.2.7, Mechanical splices in special moment frames and special structural walls), in a structure undergoing inelastic deformations during an earthquake, the tensile stresses in reinforcement may approach the tensile strength of the reinforcement. Thus, this requirement ensures that premature failure, bar pullout, or coupler fracture will not impact the ductility of the structural system.
Clause 18.2.7.2 of the code stipulates that except for Type 2 mechanical splices on Grade 420 reinforcement, mechanical splices shall not be located within a distance equal to twice the member depth from the column or beam face for special moment frames or from critical sections, where yielding of the reinforcement is likely to occur as a result of lateral displacements beyond the linear range of behavior. Type 2 mechanical splices on Grade 420 reinforcement shall be permitted at any location, except that clause 18.9.2.1(c) stipulates that they shall be located not closer than h/2(h is the depth of beam) from the joint face of beams. This is because Type 1 mechanical splices on any grade of reinforcement and Type 2 mechanical splices on Grade 550 and Grade 690 reinforcement may not be capable of resisting the stress levels expected in yielding regions. The locations of these mechanical splices are restricted because tensile stresses in reinforcement in yielding regions can exceed the strength requirements of clause 18.2.7.1. These restrictions on all Type 1 mechanical splices and on Type 2 mechanical splices on Grade 550 and Grade 690 reinforcement apply to all reinforcement-resisting earthquake effects, including transverse reinforcement. Although the ACI 318-19 requires mechanical splices should have at least 25% higher design strength than lap splices, clause 26.2.5.2 of IS 456:2000, Plain and Reinforced Concrete - Code of Practice, suggests 100% design strength be assumed for mechanical connections. Note that couplers should be provided with a cover similar to that specified for the reinforcement since there will be a reduction of cover at the splice due to the larger coupler size.
Staggering of mechanical splices in walls is generally recommended, but not required by most codes, except in seismic zones. There are several reasons for this:
• Reduces stress concentration. When mechanical splices are concentrated in one area, it can create a weak point. Staggering the splices helps to distribute the stress evenly.
• Improve concrete placement. Mechanical splices can make it difficult to place concrete properly, especially in congested areas. Staggering the splices gives the concrete more room to flow and helps to prevent voids.
• Reduce the risk of cracking. Cracks are more likely to form at the location of mechanical splices. Staggering the splices helps to reduce the risk of cracking by spreading out the stress concentration.
In seismic zones, ACI 318 Code requires that mechanical splices in special moment frames and special structural walls be staggered at least 30 inches (750 mm) to help improve the performance of these walls during an earthquake.
According to clause 25.5.7.3 of ACI 31819, mechanical or welded splices need not be staggered except as required by clause 25.5.7.4. Clause 25.5.7.4 stipulated that splices in tension tie members shall be made with a mechanical or welded splice provided that the splice develops in tension or compression at least 1.25f y of the bar. It also suggests that splices in adjacent bars shall be staggered at least 30 inches (750 mm). The commentary to clause 25.5.7.3 explains that although mechanical and welded splices need not be staggered, staggering is encouraged and may be necessary for constructability to provide enough space around the splice for installation or to meet the clear spacing requirements.
Mechanical splicing products are widely used in the construction industry to join two reinforcement bars as an alternative to lapping bars. Careful planning will ensure walls are properly reinforced and mechanical splices are properly staggered to reduce the risk of failure. ■
Dr. N. Subramanian, Ph.D., FNAE, FASCE, FIE, is an award-winning author, consultant, Alexander von Humboldt Fellow, and mentor. As a former chief executive of Computer Design Consultants, he has designed 800 projects over a span of 26 years. He has authored 26 books and more than 320 technical papers. He is the recipient of several awards and served as the past National Vice-President of ICI and ACCE(I).
A report published this January provides guidance to advance the understanding of the behavior of SWOF buildings and encourage improved practice in the design of retrofits. By Justin Moresco, PE, and David Mar, SE
Older, multi-unit wood-frame buildings with brittle, weak, and torsionally irregular stories have collapsed in past earthquakes. Often designated as soft, weak, or open-front (SWOF) buildings, many were constructed in the 1950s through 1970s and can be found across the United States, most notably along the West Coast. The lateral systems consist of non-engineered sheathing and architectural finish materials, such as diagonal and 1x lumber sheathing, cement stucco, plaster, and gypsum wallboard. SWOF buildings often house significant numbers of people.
The Federal Emergency Management Agency (FEMA) originally addressed the risk from SWOF buildings by developing and, in May 2012, publishing "FEMA P-807, Seismic Evaluation and Retrofit of Multi-Unit Wood-Frame Buildings With Weak First Stories." This guideline introduced a methodology to focus the retrofit on the first story to protect the building from collapse without transmitting excessive additional seismic forces into the upper stories. This approach accounted for the strength provided by the nonstructural walls and resulted in retrofits that balance performance with economics. Since that time, California municipalities increasingly have enacted mandatory or voluntary seismic retrofit ordinances for SWOF buildings. The ordinances reflect regional differences in their approaches, including the engineering design requirements for retrofits. These ordinances have increased retrofit experience and highlighted regionally based information regarding the configuration and construction materials used in these types of buildings. Many cities in Northern California require that the entire first story be considered and addressed, whereas many cities in Southern California allow retrofits to directly mitigate the open-front (or open-line) vulnerability without considering or strengthening the entire first story.
In 2020, FEMA NEHRP launched a project managed by the Applied Technology Council (ATC) that led to the publication of FEMA P-807-1, Guidance and Recommendations for the Seismic Evaluation and Retrofit of Multi-Unit Wood-Frame Buildings With Weak First Stories. The purpose of this report, which was published this January, is to advance the understanding of the behavior of SWOF buildings and to encourage improved practice in the design of retrofits. The report provides technical information about the expected seismic collapse performance of common
SWOF building configurations, both in their unretrofitted (or original) and retrofitted conditions. It also presents retrofit design examples. The report is intended to be used by jurisdictions and their consultants to inform decisions regarding ordinance scope and retrofit methods. Throughout FEMA P-807-1, both prevalent methods—full story and open-front retrofits—are analyzed and discussed, and much of the content, in particular the retrofit recommendations, is relevant to all types of SWOF building retrofits.
Two basic forms of archetype buildings were studied. They are rectangular in plan, two and three stories tall above ground, and with an open front on either a long or short elevation, designated as LO (long-side-open) and SO (short-side-open), respectively (Figures 1 and 2). The wall materials are cement stucco exterior siding and either gypsum wallboard or lath-and-plaster interior finishes. The diaphragms are either straight or diagonal sheathing. The selection of these materials was informed by an evaluation of common SWOF characteristics using Northern and Southern California datasets. The archetype buildings have two material combinations for the walls— strong wall (SW) and weak wall (WW). The strong wall set, which is representative of buildings constructed from the 1920s through early 1960s, has cement stucco exterior finishes and gypsum plaster interior finishes. The weak wall set, which is representative of buildings constructed from the 1950s through 1970s, has cement stucco exterior finishes and gypsum wallboard interior finishes. Two types of diaphragms were investigated—a strong diaphragm (SD) representing diagonal-lumber sheathing and a weak diaphragm (WD) representing straight-lumber sheathing. These different materials were combined with one another to create the “primary study” archetype building options (Table 1). They consist of long-side-open and short-side-open forms of two and three stories, with both the strong wall/ weak diaphragm (SW-WD) and weak wall/strong diaphragm (WW-SD) material configurations.
Besides the “primary study” archetypes, “variant study” archetypes were developed in FEMA P-807-1 to investigate the impact of wing walls, no open-front vulnerability, and relatively weaker and more brittle diaphragm properties. This article focuses on the “primary study” archetype results due to space limitations.
The buildings were modeled in three dimensions with OpenSees using an assemblage of non-linear
shear springs to represent the walls, diaphragms, and retrofit frames when present. The non-linear springs are placed along a single line in each principal building direction (e.g., Grid Line 3 in Figure 1b or Grid Line C in Figure 2b), and tributary masses are assigned at grid points. The springs were calibrated to physical tests of the representative wall and diaphragm materials. The springs have appropriate non-linear behavior for in-plane shear, high elastic stiffness for in-plane flexural and axial modes, and negligible stiffness for out-of-plane modes. The retrofit frames were modeled as point springs at the second-floor elevation, centered in the open line. The X direction is parallel to the open side and the Y direction is perpendicular.
The archetype buildings were subjected to seismic shaking per the FEMA P-695 protocol. The seismic input for the incremental dynamic analysis (IDA) was 22 bi-directional, far-field records. Each set of records was rotated 90 degrees to expand the set to 44 inputs. The records were scaled with increasing intensities until the models were identified to have collapsed. The peak inputs usually corresponded to walls reaching between 5%-10% drift, while collapse typically resembled an explicitly modeled P-delta collapse, primarily driven by the P-delta effects in the hysteretic wall spring models along with P-delta columns at the open front. Within the IDA results, collapse is seen as an infinite increase in drift without increase in spectral acceleration (Figure 3). As shown in Figure 3, spectral acceleration (Sa) values at each increment of the analysis are taken at a period, T = 0.25 seconds, consistent with the FEMA P-695 protocol.
The seismic resistance of the archetype buildings is limited by multiple vulnerabilities. These are the lateral strengths in each direction, the diaphragm strength, and the torsional imbalance of the structure. A useful analogy is that of a chain with several potential weak links, where the resistance to collapse is controlled by the weakest link. The buildings were found to have multiple vulnerabilities with similar capacities. As such, mitigating one vulnerability without improving the rest often resulted in little improvement to building collapse risk. A seismic retrofit of SWOF buildings usually needs to address several or all the vulnerabilities in the weak story to substantially improve safety.
WW-SD SW-WD
Long Side Open 2-Story LO2-WW-SD LO2-SW-WD
3-Story LO3-WW-SD LO3-SW-WD
Short Side Open 2-Story SO2-WW-SD SO2-SW-WD
3-Story SO3-WW-SD SO3-SW-WD
The most direct way to assess the building’s lateral capacity is to examine the first-story strength-to-weight ratio (V/W). Pushover studies were made of the “primary study” archetypes (Figure 5), and key results are:
• Archetype buildings with weak walls have slightly higher controlling strength-to-weight ratios than buildings with strong walls . This is because the buildings with strong walls are heavier.
• The archetypes are brittle, with very limited ductility. Most pushover curves have a steep strength loss after reaching the peak strength.
• The LO archetypes have similar strength-to-weight ratios in the X and Y directions for both the strong and weak wall conditions. The presence of an open side does not lead to appreciable weakness in the open direction. This is because the walls adjacent to the tuck-under parking are solid, without windows, unlike the typical exterior elevations.
• The SO archetypes are weaker parallel to the open front (X direction) for both wall types. The strength-to-weight ratio difference is greater with the strong walls.
• The controlling strength-to-weight ratios of the two-story buildings (not shown in Figure 5) are typically more than 40%, which is significantly greater than their three-story counterparts. This is because the first-story wall layout is the same, but the two-story building carries significantly less mass because there is one fewer floor.
Three types of retrofits were designed for each archetype: line, optimized line, and FEMA P-807. The line retrofits follow the requirements of the Los Angeles SWOF ordinance and associated city guidelines. The optimized line retrofits also conform to the Los Angeles SWOF ordinance except that the prescribed deflection limits on frames at the open front are ignored, making the frames controlled by strength requirements. The FEMA P-807 retrofits are in accordance with the FEMA P-807 report and are based on output of the Weak Story-Tool assuming default material property values. The Weak-Story Tool is a freely available electronic resource that was developed to help users apply the rules and perform the calculations described in FEMA P-807.
A site in downtown Los Angeles was selected for determining seismic demands, corresponding to a horizontal spectral acceleration of 1.0g for all three retrofits. This seismic demand reflects use of 75% of new building design spectral acceleration, which is permitted by the Los Angeles SWOF ordinance. The ordinance also specifies that acceptable performance for FEMA P-807 retrofits is based on drifts corresponding to onset of strength loss
and that the maximum drift limit probability of exceedance is 20% at the specific hazard. These FEMA P-807 design criteria were input into the Weak Story-Tool.
The line retrofits consist of cantilever columns cast into reinforced concrete grade beams along the open front. This system acts as an inverted moment frame, where the grade beam is strong and stiff enough to develop the capacities of the columns in flexure. The optimized line retrofits are similar to the line retrofits except that the removal of the deflection limits results in lighter and more flexible frames. The FEMA P-807 retrofits also use cantilever columns along the open front, as well as new plywood shear walls in both orthogonal directions applied to the inside of existing wood-frame walls. Table 2 provides selected seismic retrofit parameters for the three-story LO archetype building with weak walls and strong diaphragms, where “L”, “OL”, and “P807” corresponds to line retrofit, optimized line retrofit, and FEMA P-807 retrofit, respectively.
Figure 6 presents results of the IDAs for the “primary study” archetype buildings in terms of probability of collapse (POC) at spectral acceleration of 1.0g. The following trends are noted:
• FEMA P-807 retrofits are effective with results better than 20% POC.
• Line and optimized line retrofits do not consistently improve safety. Three-story LO archetypes show moderate improvements. SO archetypes show limited improvements.
• Line and optimized line retrofits provided similar results for a given archetype.
• Three-story archetypes are more vulnerable than their two-story counterparts.
• SO archetypes are usually more vulnerable than their LO counterparts.
FEMA P-807-1 presents a series of recommendations related to seismic retrofit ordinances that are based on the results of the aforementioned analytical studies. These recommendations include: Importance of Retrofit: In high-seismic-hazard regions, it is recommended that seismic retrofit ordinances be considered for SWOF buildings as part of a program to identify and address seismically vulnerable buildings. Based on the archetypes studied, high POCs were identified for unretrofitted SWOF buildings. This is consistent with observed collapses and near collapses of SWOF buildings in the 1989 Loma Prieta
and 1994 Northridge earthquakes. The POCs can be reduced through seismic retrofits. In moderate and low seismic regions, the need for seismic retrofit ordinances for SWOF buildings is less clear because unretrofitted POCs can be significantly lower.
Type of Retrofit, Part 1: It is recommended that full first-story retrofits be required, where practicable. For the archetypes studied, FEMA P-807 retrofits consistency provided notably better performance than line or optimized line retrofits, with POCs averaging about 10%. Published studies by others suggest that, in general, full-story retrofits in accordance with IEBC Chapter A4 or ASCE/SEI 41 will provide similar or improved performance of the first story relative to the requirements of FEMA P-807 (Buckalew et al., 2015; Burton et al., 2019).
Type of Retrofit, Part 2: Where it is not possible to require a FEMA P-807 or other full-story retrofit, it is recommended that screening occur for open-front wall lines on all exterior walls of the building, including those perpendicular to the evident open-front wall. Where suggested by screening criteria, retrofits should be provided for all applicable exterior walls, including those perpendicular to the evident open front.
Building Prioritization: Where prioritization of SWOF building retrofits is desired, it is recommended that SWOF buildings three stories or more be given higher priority than two-story SWOF buildings. The three-story archetypes generally have higher unretrofitted POCs and greater benefit of retrofit reduction in POC than two-story archetypes.
Local Seismic Hazard: When considering adoption of a seismic retrofit
ordinance, it is recommended that local seismic hazard levels be taken into consideration. Unretrofitted collapse potential of SWOF buildings varies significantly with seismic hazard, thereby varying the need for and benefit of retrofit. For example, the unretrofitted POC is less than 10% at a spectral acceleration of 0.5g for all primary study archetypes and is less than 20% at a spectral acceleration of 0.75g for all but one of the primary study archetypes.
FEMA P-807-1 includes a series of recommendations related to design and construction of SWOF retrofits. The objective of these recommendations is to assist engineering designers and building officials in avoiding common pitfalls and applying proven strategies for strengthening SWOF buildings. The recommendations address the following categories:
New Seismic-Force-Resisting Systems: Common retrofit options include adding new steel moment frames, steel cantilevered columns, wood-structuralpanel shear walls, proprietary systems or, in some cases, a combination of these systems. FEMA P-807-1 discusses each of these options and provides recommendations related to more general topics including redundancy, compatibility, and optimal location of new systems.
Protection of Existing Structural Systems: SWOF buildings do not have engineered lateral systems and are constructed using nonductile materials, making it essential that engineers pay special attention to the impact of their designs on the existing building. For example, local demolition of stucco at the second floor is often necessary to install new retrofit elements, such as collectors, that tie the existing structure directly to new vertical seismic-force-resisting elements. But exterior stucco walls in SWOF buildings often are a major contributor to lateral strength. Several examples are provided in FEMA P-807-1 for how to protect the existing lateral and gravity systems.
Foundations: It is recommended that the foundation system of a new seismic-force-resisting system be tied to the existing foundation system to minimize the possible negative effects of sliding, uplift, and overturning. Other topics covered in FEMA P-807-1 include recommended detailing for fixed-base retrofits, weak-axis implications for fixed-base retrofits, and protecting existing foundations.
Besides the categories noted above, FEMA P-807-1 also provides recommendations related to load paths to new retrofit elements; collectors, moment frame beams, and columns; and quality assurance. Two design examples are presented—an optimized line and a FEMA P-807 retrofit.
The design examples include conceptual construction details and illustrate implementation of the design recommendations.
SWOF buildings can be found across the U.S., and their structural vulnerabilities make them prone to collapse during earthquakes. Municipalities in California increasingly have enacted seismic retrofit ordinances for SWOF buildings, with the ordinances reflecting regional differences in their approaches. The purpose of FEMA P-807-1 is to advance the understanding of the behavior of SWOF buildings and to encourage improved practice in retrofit designs. The report is also intended to be used by jurisdictions and their consultants to inform decisions regarding ordinance scope and retrofit methods.
FEMA P-807 was shown to generate full-story retrofit designs that provide significant benefits in terms of reducing probabilities of collapse for SWOF buildings. A few suggestions for future FEMA P-807 enhancements are given, but no major shortcomings with the method were identified. Both line and optimized line retrofits were shown to provide mixed benefits in terms of reducing probabilities of collapse. For some archetypes, the reductions were moderate, whereas for other archetypes the reductions were negligible. ■
Similar to other ATC-managed projects, the project team included a Project Technical Committee and Working Groups who collectively conducted the technical work and authored FEMA P-807-1. In addition, a Project Review Panel provided technical review at key milestones in the development of the report. The work forming the basis for this publication was conducted pursuant to a contract with FEMA. The substance of such work is dedicated to the public. The authors are solely responsible for the accuracy of statements or interpretations contained in this publication. No warranty is offered with regard to the results, findings and recommendations contained herein, either by FEMA, ATC, its directors, members, or employees. These organizations and individuals do not assume any legal liability or responsibility for the accuracy, completeness, or usefulness of any of the information, products, or processed included in this publication.
Full references are included in the online version of the article at STRUCTUREmag.org
Justin Moresco, PE, is a Director of Projects at the Applied Technology Council in Redwood City, California. He manages multi-disciplinary teams on projects that develop technologies for structural engineering and natural hazards-related applications. (jmoresco@atcouncil.org)
David Mar, PE, SE, is Founder and Principal of Mar Structural Design in Berkeley, California. He was the technical director for the original FEMA P-807 SWOF Retrofit Guidelines, as well as the current follow-up study. (david.mar@marstructuraldesign.com)
By Leslie Jo Hurwitz, PE, Brian Fisher, PE, and Carl Schneeman, PE
Recent events, public perception, and the fact that electric vehicles (EVs) are heavier than gas powered (ICE) vehicles have caused many to question whether the current building code minimum design requirements are adequate to accommodate increasing EV sales. How this will affect minimum required design live loads depends on factors such as electric vehicle sales trends, the weights of the vehicles themselves, and the different loading scenarios.
Today, approximately 260 million vehicles are on the road and roughly 15.6 million passenger vehicles were sold in 2023. Roughly half of 2023 sales were crossover and SUV type vehicles, with the balance largely split between traditional cars and pickups. Trends show that car and van sales are steadily declining while the proportion
of pickup sales have been relatively stable over the past decade. So, how do EVs fit in the mix?
The auto industry is undoubtedly going electric; however, it will take quite a while for EVs to become the typical, much less dominant, passenger vehicle on the road. For comparison, in 2023 approximately 1.2 million electric passenger vehicles were sold, representing 7.9% of annual sales, per Argonne Laboratories data. Please note that the term EV herein includes only fully electric vehicles. Table 1 indicates Walker Consultant’s projections of BASE case (expected trend) and HIGH case (accelerated adoption) for electric vehicle sales and the corresponding percentage of such vehicles on the road. Both projections are based on a consensus of auto industry consultant projections. The on-the-road projections are based on a model developed by Walker that considers industry projections of total passenger vehicle sales through 2030, population growth, as well as historic vehicle scrappage rates to determine total vehicles and EVs on the road.
Sales projections of EVs as a percentage of all vehicles sold is expected to increase as more vehicle choices are offered, prices decrease, and technology improves; however, the actual EVs on the road as a percent of all cars on the road is increasing at a much slower rate. In 2040, the estimated sales range from 55% - 85% but the percentage of EVs on the road is only projected to be 23% - 42%.
Vehicle Weight
Walker has been tabulating vehicle size data since 1983 for cars, with light trucks (crossovers, SUVs, pickups and vans) added in 1996. Adjustments were made in 2016 to account for the percentage of pickups and vans that are classified as heavy duty and used for commercial purposes rather than personal transportation. This annual analysis uses sales data reported by Automotive News for individual makes and models, which is correlated to size, height, and weight data per the manufacturer websites. Historically, the primary focus of this analysis has been to study the footprint of vehicles to determine parking dimensions; however, the weight and height of vehicles have become
items of interest while crossovers and SUVs have become more prevalent on the road. Overall, vehicles today have gotten wider by 3 inches, generally stayed less than 6 feet tall, and still have lengths appropriate (or slightly less) for 18-foot-long stalls as compared to vehicles of the 1980s.
When it comes to the weight of passenger vehicles, 70% to 75% of all cars sold each year in the U.S. since 2001 including EVs, have curb weights between 3,000 pounds and 5,000 pounds. Overall, we have observed a reduction in vehicles of less than 3,000 and 4,000 pounds (predominantly cars), and an increase in vehicles that are 4,000 to 5,000 pounds, reflecting the increase in crossover sales. But, as demonstrated in Figure 1, the percentage of all passenger vehicles that are over 6,000 pounds has remained less than 5% since 1996.
The “Design Vehicle” is commonly used in traffic engineering to design roadways and intersections throughout the world. The concept has been applied to parking to define the composite vehicle on which parking geometrics are based. It is assumed that all cars using a parking structure will be the Design Vehicle and it is defined as the 85th percentile vehicle on the road from smallest (0%) to largest (100%).
By assuming all cars parking in a structure are the Design Vehicle for parking layout purposes, 84% of the vehicles actually parked in the structure will likely be smaller and have a more comfortable experience parking than the 14% of the vehicles that are larger than the design vehicle. In fact, the probability of having a parking space occupied by a vehicle larger than the Design Vehicle parked on both sides of it and across the aisle is 15% multiplied by 15% multiplied by 15%, or 0.33%. Very unlikely!
The percentage of all passenger vehicles that are over 6,000 pounds has remained less than 5% since 1996.
By studying annual sales, not the specific number of vehicles on the road, we are able to annually monitor vehicle size trends. Therefore, the Design Vehicle is not changed each year, but rather the analysis enables identification as to when a trend has been repeated for several years. We can then be reasonably confident that a lasting change has occurred. Since the average age of vehicles on the road is 12 years, the Design Vehicle on the road will not reach revised dimensions for 5 to 7 years. While some may argue that the average vehicle has gotten
larger, the sales data definitively identifies that the 85th percentile vehicle footprint has not changed more than a few inches in length or width in the last decade. This same sales data suggests that the footprint of EVs and conventional vehicles is also similar.
But what about weight? Are EVs heavier? Based on Walker’s tracking of vehicle sales and properties, yes, generally they are. Typical electric vehicles do appear to have a curb weight greater than their traditional counterpart. Over the last decade, the 50th percentile electric vehicle has a weight of approximately 4,400 pounds whereas the same percentile conventional vehicle has a
weight of approximately 3,700 pounds. As an example, the 2023 Volvo XC40 EV has an approximate curb weight of 4,780 pounds compared to 3,970 pounds for its conventionally-powered all-wheel drive variant. But as we look at larger vehicles a different trend emerges from the data.
When considering a design vehicle curb weight, the 85th percentile weight vehicle in 2022 had a curb weight of just over 5,000 pounds. The 85th percentile weight between 1996 and 2018 varied between 4,400 to 4,900 pounds, but since then has been slightly increasing on an annual basis. The 85th percentile curb weight of vehicles over the past five years is roughly 5,000 pounds, whereas over the last 10 years was 4,900 pounds. Therefore, it is reasonable to identify the Design Vehicle curb weight of passenger vehicles is currently 5,000 pounds.
Considering electric vehicles, study of sales data from 2011 through 2023 yields information relative to their specific design vehicle weight. Over the prior 11 years, the 85th percentile electric vehicle weight is 4,861 pounds, represented by the Audi Q4 e-tron. Over the prior 5 years, the 85th percentile electric vehicle weight is 4,877 pounds represented by the VW ID4. Note that these figures are very near that of the Design Vehicle curb weight for all vehicles.
Yes, a small number of EV models with curb weights of 7,000 pounds and up to the 9,063 pound Hummer EV have been introduced, but they sell in small numbers, or exist above the 85th percentile point. In fact, the Hummer is not a “light duty passenger vehicle,” but rather a “heavy duty” truck. In its earlier 1992 to 2006 ICE form, Hummers were never more than a fraction of 1% of cars on the road.
Figure 2 shows the trends in the 85th percentile weight of EVs as well as the total passenger vehicle sales since the first EVs were sold in 2011.
Before the International Building Code (IBC) was first published in 2000, three general building codes were used throughout the U.S. that are considered ‘model’ or ‘legacy’ codes. Those codes were: The BOCA National Building Code primarily used in the northeast, the Standard Building Code (SBC) generally used in
the southeast, and the Uniform Building Code (UBC) that was used in the rest of the of the U.S. Over time, the IBC has been adopted in most, if not all, areas of the country. Much of the design load criteria contained in IBC and in ASCE 7, Minimum Design Loads and Associated Criteria for Buildings and Other Structures, are applicable to the determination of parking garage live loads.
A paper by Y.K. Wen and G.L. Yeo, “Design Live Loads for Passenger Cars Parking Garages” in Journal of Structural Engineering,” published
in March of 2001,provided a better understanding of live loads in parking structures and recommended an appropriate value for design. Looking at the maximum load effects on beams and columns due to vehicle loads over the anticipated life expectancy of a parking structure, Wen and Yeo determined the axial loads and beam midspan moments using the design live load of 50 pounds per square foot (psf) would be two to three times greater than floor members would actually experience. They also concluded that dynamic
amplification resulting from moving vehicles would not increase the prescribed design load unless it is a speed ramp with slope greater than 10-degrees. Their final conclusion recommended a design live load of 40 psf with no allowance for area-based reductions. This recommendation did not apply to special purpose or densely parked structures especially when there would be no variety in the vehicle type parked.
About a year later, the March 2002 Concrete International magazine included an article by Javed Malik who was concerned with the increase in SUVs on the road and argued for point and uniform live loads in parking structure design to be significantly increased suggesting the wheel point loads be increased by 50% and the uniform live loads doubled.
His analysis was subject to an extremely conservative and rare building framing system for parking structure construction. The typical construction materials and framing systems for parking structures at the time was, and still is, precast concrete or cast-inplace post-tensioned concrete systems.
A rebuttal white paper by Mary Smith, PE, and Tony Chrest, PE, of Walker Consultants, reviewed the vehicle trends at the time and analyzed the assumptions made by Malik for maximum wheel loads, axle distribution, vehicle curb weights, and gross vehicle weight ranges (GVWR). The use of the 85th percentile vehicle in the range of lightest to heaviest curb weight was proposed for the first time to be used as the Design Vehicle in the determination of uniform loads since at the time, the practice of using the 85th percentile vehicle was the standard for parking geometrics and commonly used in traffic engineering.
Using the Design Vehicle, Smith and Chrest analyzed loading
Design Vehicle: Volkswagen ID4 Weight
Curb Weight (lbs.)
4877
Passengers (max): 6 adults @ 175 lbs. 1050
Cargo (lbs.) 981
Total Weight when driving (= gross vehicles weight rating) 6908
Total Weight when parked (curb weight + cargo) 5858
conditions for various parking structure concrete and steel framing systems as shown in Figures 3-5.
Next, they considered heavier vehicle density stacked parking such as rental car storage or valet parking.
Consistent with Wen and Yeo, Smith and Chrest’s analysis found that in spans greater than fifteen feet, uniform loads were all less than 40 psf. Shorter span conditions and some uncommon high vehicle density conditions were slightly greater than 40 psf.
Today, the IBC/ASCE 7 still specifies a 40 psf design live load for parking structures.
Walker intentionally checked for the live load when an entire area is parked with EVs due to the local requirements to design 20% or more of the parking spaces to be EV Ready. This is increasingly leading to EVs comprising all the parking stalls in one area. Using the theories of analysis used by Wen and Yo and Smith and Chrest, the uniform load is evaluated over typical bay sizes that are based on framing systems commonly utilized in parking structures in the U.S. today as shown in Figures 4 through 5.
To start, Wen and Yeo’s study found that while dynamic effects from moving vehicles can increase the anticipated load, “It would be reasonable to conclude that dynamic amplification due to moving vehicles would not cause the equivalent uniformly distributed load (EUDL) to be more than the case of fully parked two-way traffic bays with vehicles waiting in the aisles.” In short, it was determined that with moving vehicles in a parking structure, the suggested dynamic amplification factor is independent of vehicle weight since the shallow slopes in which vehicles interact with floors does not transmit appreciable vertical load to the structure. As is consistent with generally accepted practice, when closely spaced concentrated wheel loads are distributed at a 45-degree angle from the direction of span they quickly overlap other wheel loads, allowing the load to be judged as a uniformly distributed load.
Following the Smith and Chrest philosophy of using the Design Vehicle for the determination of uniform loads, Walker used the 85th percentile electric Design Vehicle to establish the anticipated loading on structural members. Following this approach, all parking spaces in a structural bay were assumed to be occupied by electric Design Vehicles at curb weight plus cargo and that a string of electric Design Vehicles loaded to their gross vehicle weight rating (GVWR) are simultaneously placed each way along the drive aisle. This approach is a conservative estimation of uniform live load in a bay since the likelihood that all the vehicles in an area are all an 85th percentile Design Vehicle simultaneously
traversing the drive aisles is extremely small. Additionally, for most public parking facilities serving visitors, commuters, or shoppers the odds of all the vehicles in the drive aisle being loaded to the full GVWR are even less likely.
Let’s now check the uniform load for the electric Design Vehicle created by the scenario in Table 2.
Assumptions:
• Parking Geometrics: 8 ft. 6 in. stalls @ 90 degrees on 60 ft. modules
• Assume Electric DV at 6908 lbs. are driving both ways down the aisle
• Assume Electric DV at 5858 lbs. are parked in every stall
The authors then considered what the equivalent uniform distributed load would be for the framing systems commonly employed in parking structures in the U.S. today:
• Precast Garage.
• Post-tensioned, cast-in-place concrete with one way slabs and beams.
• Conventionally reinforced concrete with 30” x 30” column grid.
• Steel beams and column with P/C double tees.
• Steel beams and columns with a cast-in-place post-tensioned slab.
The calculated equivalent uniform loading with an electric Design Vehicle for each system is shown in Table 3.
As can be seen, none of the typical parking structure framing systems have an equivalent uniform load exceeding 40 psf when considering the electric Design Vehicle. This should be no surprise as the design vehicles weights between conventional and electric vehicles are quite similar.
Despite all the discussion surrounding electric vehicle weights exceeding the weight of their internal combustion engine counterparts, Walker’s data base of vehicle sales and weight indicates that the electric Design Vehicle is of similar curb weight than the overall passenger vehicle mix sold in recent years. As such, the overall 85th percentile weight vehicle is most appropriate for live load calculations. Combined with Wen and Yeo’s finding that maximum load effects on beams and columns using a uniform load of 50 psf would be two to three times greater than what they expected the members to actually experience, the current IBC and
ASCE 7 prescribed live load of 40 psf for passenger vehicle garages is viewed as an accurate representation of expected conditions for the current information available on EV’s and their presence on the road.
Structures that have experienced deterioration represent a very different structural concern, one that is not specific to electric vehicles or internal combustion vehicles. Aged or neglected parking structures that have experienced deterioration may need to be assessed structurally to ensure that they have a sufficient strength for the loads in which they are intended to support. If the available strength is determined to be inadequate, then it is only prudent to repair, strengthen, or restrict loads to such a deteriorated structure. However, this is not due to electric vehicles, but rather the overall shift to SUVs and pickups, as compared to 20 years ago.
How vehicle weight will change remains to be seen as battery technology is still in its infancy and could change to be lighter yet more efficient and achieve longer ranges; but it is viewed by the authors as unlikely that trends of ever-heavier cars, crossovers, and pickups that meet consumers vehicle range expectations will continue. Further, is it premature to consider an increase in design loads on the speculative basis of vehicle weights 25 years in the future if/ when EVs are finally approaching 50% of the vehicles on the road? As engineers, it is important to consider that realistic, rational, and safe loads for the design of facilities that are to be used by the public. However, finding a prudent balance for reasonable design is also necessary so that designs may not be viewed as unreasonably conservative and undermine public trust. Further, judicious designs are needed more than ever to foster sustainable designs and reduction of costly construction materials. Continual monitoring of data will help ensure that the evolution of code load standards reflects actual load scenarios combined with rational safety factors rather than on public perception. ■
Full references are included in the online version of the article at STRUCTUREmag.org .
Leslie Jo Hurwitz, PE, is the Managing Principal of Walker Consultants’ Charlotte, NC office and have worked with Walker for over 20 years in the design and restoration of parking structures.
Brian Fisher, PE is Director of Design in Walker Consultants’ Charlotte, NC office. He has 18 years at Walker and 23 years in the industry designing parking structures.
Carl Schneeman, PE has designed parking structures for over 20 years and is a contributing member of the National Parking Association’s Parking Consultants Council.
The award-winning project updated the structural systems of the University of California San Diego building while preserving its architectural heritage.
By Bryan Seamer, PE, SE
The seismic rehabilitation of York Hall at the University of California San Diego (UCSD) is an excellent example of the challenges faced by seismic engineers in earthquake-prone areas as aging building stock is modernized to prolong its functional life and seismic resilience. Designers must quantitively demonstrate compliance with modern seismic standards while qualitatively preserving critical pieces of social and community heritage.
Built in 1966 and composed of four seismically separate structures, the Donald Neptune and Joseph Thomasdesigned York Hall is remarkable for its “structure as architecture” aesthetic. The 122,000 square-foot midcentury modern complex prominently frames the east side of historic Revelle College, its west wing perched atop a 300-foot-long column arcade and ringed with precast concrete fins that provide shading to exterior walkways at each level. It is one of the original buildings on the campus and has been referred to by administrators as “the heart of our campus.”
Everything that makes York Hall iconic also made it an unacceptable seismic risk for UCSD. The fluted columns, precast fins, and exterior CMU—components listed as “contributing features of historic significance”— all lacked the seismic ductility to meet the University of California’s (UC) seismic performance objective. When upgrading the building, preserving these historic elements was a top priority.
A 300-foot-long fluted concrete colonnade supports the two-story concrete and masonry building above.
LPA Design Studios, San Diego, CA
Owner: University of California, San Diego
Architect of Record: LPA Design Studios
General Contractor: PCL Construction Services, San Diego, CA
Structural Concrete Sub-contractor: Pacific Southwest
Structures, Inc, Lemon Grove, CA
Mechanical, Electrical and Plumbing Engineers: LPA Design Studios
Seismic Peer Reviewer: KPFF Consulting Engineers, San Diego, CA
Historic Consultant: Heritage Architecture, San Diego, CA
The UC Seismic Safety Policy establishes the seismic performance requirements for the retrofit of existing buildings across the University of California system. The standard establishes two performance levels that must be met based on the retrofit methodology described in ASCE Standard 41-17: Seismic Evaluation and Retrofit of Existing Buildings and the building’s Seismic Risk Category. Each of the seismically independent wings of York Hall is classified as Risk Category III based on the number of occupants. The first performance level is Life Safety for seismic demands based on a site-specific response spectrum with a 20% probability of exceedance over 50 years. The second performance level is Collapse Prevention for a site-specific response spectrum with a 5% probability of exceedance over 50 years. The UC Seismic Safety Policy also requires that hazards related to falling building components be abated as part of the seismic retrofit, a requirement that necessitated the assessment, restoration and repair of the hundreds of precast concrete fins that surround each building.
Also high on the university’s priority list was keeping the building operational throughout the renovation. As the main undergraduate biology and chemistry facility at UCSD, York Hall is one of the most heavily used buildings on campus. Classes and grant-funded research going on in the building couldn’t be disrupted or paused during the rehabilitation.
LPA Design Studios, the architect and engineer for the project, proposed an integrated, data-informed approach. Working as a unified team of architects and structural engineers, LPA led UCSD’s project management team through a process that explored multiple seismic retrofit strategies and balanced a broad set of priorities to minimize visible structural interventions, control costs, minimize operational and embodied carbon impacts, and maintain continuous operation of the building complex during construction. Seismic retrofit strategies were the starting point for each iterative design concept, so LPA’s structural engineers led the collaborative coordination sessions with the owner, architect, and contractor from the earliest phases of the project, ensuring that historical
architecture, uninterrupted building systems, and constructability were respected with each subsequent evolution of the seismic retrofit strategy.
The result is an NCSEA-award-winning seismic rehabilitation that seamlessly updated the structural systems while preserving an important piece of regional history. In the process, the project achieved millions in cost savings and created a new model for seismic retrofits on the campus.
York Hall was the first UCSD project to be retrofit under UC’s systemwide seismic risk mitigation program. Understanding the larger goals and providing multiple varied options were key to finding the right approach.
LPA worked closely with the UC Seismic Review Board and developed multiple retrofit options based on six campus priorities: seismic safety, historic stewardship, limiting potential disruption, phasing in coordination with campus user groups, maintaining budget and schedule, and reusing as much of the existing building as possible to reduce embodied carbon impact. Initial concepts ranged from “leaning” the colonnade-supported West Wing on the more structurally regular North and South Wings, to surgically targeting specific vulnerable elements in each of the buildings and many variations in between.
The informed design process helped UCSD mitigate risk at every step. By thoroughly understanding the university’s multi-faceted needs and providing clarity and transparency to the prioritization of those needs, designers were able to add confidence to the bidding process and ultimately save money. The team ultimately took a balanced approach that added capacity to some vulnerable components and fully replaced others to maximize seismic performance while minimizing construction cost and disruption.
The work focused on three main areas: strengthening the fluted colonnade, restoring hundreds
of concrete fins, and mitigating seismic shear forces in critical masonry walls. Crucially, the simplified retrofit process resulted in cost savings of about $3 million, which was later reallocated to other critical campus needs.
York West, a bar-shaped structure that lines Revelle Plaza, a major campus thoroughfare and gathering space, is as remarkable for the beauty of its structural system as it is for its significant seismic irregularities. Bringing it into compliance while preserving its delicate, gravity-defying architecture drew on computational firepower and the ancient craft of shipbuilding.
The 300-foot long, two-story concrete lift slab and CMU shear wall structure is supported in its entirety on an open colonnade that creates a shaded breezeway (Fig. 2). Thirty-five fluted, cast-in-place concrete columns support the heavy upper structure and, along with the cast-in-place second floor slab, create a bi-directional pinned-base space frame as the building’s primary seismic force resisting system. The upper floor and roof lift slabs bear on perimeter CMU piers that, along with several interior concrete shear walls, provide seismic resistance at the upper levels. Because the upper-level shear walls don’t extend to the ground or align with the fluted concrete columns below, the seismic overturning forces and moments on the colonnade are substantial. This unique building configuration resulted in multiple irregularities including soft story, torsion, and in-plane discontinuities in the vertical LFRS.
LPA structural engineers, with oversight from seismic peer reviewer KPFF Consulting Engineers, used non-linear time history analysis and linear dynamic analysis to model seismic performance and determine that four of the fluted concrete columns lacked the capacity to resist the deformation demand caused by the building’s vertical irregularity. A combination of high-axial demands resulting from overturning forces from shear walls in the stories above and bending caused by seismic displacement of the rigid structure above necessitated strengthening. A traditional retrofit approach with FRP or steel column jackets was considered, but due to the historic nature of the vulnerable columns, a more dramatic solution was needed. The four columns would need to be replaced entirely—a “quadruple columnectomy,” as the team dubbed it.
The geometry of the columns made this challenging. The hexagonal columns narrow slightly from base to mid-height before dramatically flaring outward to create a series of cloister-like domes in the exposed concrete soffit.
To match the unique form of the original columns, fiberglass molds were created in situ prior to demolition. To provide structural integrity to the molds, PCL Construction, the design-assist contractor team, borrowed classic maritime engineering techniques learned from the local shipbuilding industry, adding parallel ribs to each fiberglass mold. After shoring the upper levels of the building, the columns were demolished, new rebar cages and structural steel wall-to-column connection hardware installed, and concrete poured into the forms, perfectly preserving the original form of the colonnade (Figs. 3 and 4).
The design of the seismic load path from upper floor shear wall boundaries to the heads of the rebuilt flared columns was equally challenging. A two-piece structural steel connection assembly was custom designed at each column, with the specific arrangement of through-bolts, embedded rebar and steel plate weldments designed to transfer seismic forces without disrupting the existing slab reinforcing (Figs. 4 and 5).
Eight-hundred and five vertical, story-tall precast concrete fins ring the upper floors of three of York Hall’s buildings, providing sun shading for exterior walkways. These critical components of the buildings’ history have been slowly deteriorating through exposure to marine air and direct sunlight for over 50 years. Like with the column arcade, designers used a bespoke approach that relied on technology.
After a comprehensive condition assessment of each fin, designers determined that nearly 75% of them showed signs of deterioration that could lead to failure of the steel connections that tie into the concrete floor plates at top and bottom. Each fin weighs nearly 500 lbs., so ensuring that they could not fall was a life-safety imperative.
The lightly reinforced fins had several types of damage, including spalling of the precast concrete body, corrosion of the steel components at top and bottom connections and cracking at the location where the perimeter guardrail attaches. LPA worked closely with specialty contractors to create a “kit of parts” to efficiently address each required repair and created specific procedures for each unique concrete deterioration and steel corrosion condition (Fig. 6).
The final major piece of the seismic retrofit puzzle was the addition of new shotcrete and masonry shear walls at critical locations throughout each building. This proved challenging because the uniquely colored and textured masonry blocks were identified as a contributing element
to the building’s historical significance. Strengthening these walls without working inside the building—a campus priority—or changing the exterior aesthetic required special attention by the design team.
The solution was to add a combination of shotcrete and multi-wythe CMU directly to the exterior face at key shear wall lines, with new CMU blocks specially fabricated to mimic the color and texture of the historic masonry (Fig. 7). Where seismic demands necessitated new shotcrete walls, the concrete was clad in historic CMU veneer to preserve the original mid-century architectural aesthetic. This approach allowed the building’s interior to remain functional during construction, without sacrificing seismic performance or compromising the historic character of the Revelle College Historic District.
As building owners in California and other seismically active zones work to modernize their buildings and prolong their functional life, York Hall shows how it can be done efficiently and affordably without
losing the architectural heritage that these buildings represent (Fig. 8).
The first big takeaway is integration. By working together as a unified architecture, engineering and ownership team, LPA and UCSD were able to rapidly develop and evaluate multiple design concepts very early to ensure that the project’s goals were optimized.
Secondly, communication within the design team and between the design team, owner, and builder was critical. Each design solution was multi-faceted and relied upon the focused expertise of many individuals across a large project team. In many cases, the willingness and openness of the structural engineering team to lead early iterative ideation was the only way to meet the university’s needs for the project. ■
Bryan Seamer, PE, SE is Director of Structural Engineering at LPA Design Studios. With more than 25 years of experience in new construction, seismic assessment, and rehabilitation of existing buildings, he uses emerging technologies and innovative design strategies to create safe, high-performing, cost-effective structural systems that are long-lasting, durable and resilient. (bseamer@lpadesignstudios.com)
For many existing buildings, a targeted and more cost effective solution can be found through the use of advanced nonlinear analysis procedures.
By Jason Armes, SE; Gina Carlson, SE; and Leo Panian, SE
The poor performance of non-ductile concrete structures typically requires significant structural intervention to meet project seismic goals. Engineers usually take a linear analysis approach to assess building performance and provide a retro-fit, which can lead to high cost and a long, inconvenient renovation period. These extensive drawbacks make it challenging for the structural retrofit to fit within the project budget and broader goals.
To combat this negative outcome, Tipping took advantage of nonlinear modeling and analysis to provide optimized retrofit solutions for Hacienda Apartments in Richmond, California, a senior affordable housing complex. Nonlinear modeling and analysis provided a better understanding of the building’s failure mechanisms and unlocked enhanced solutions that make the best use of the existing structure and add only what was needed to achieve critical performance. By leveraging both a linear and a nonlinear analysis, Tipping could make direct comparisons of the two and see how the project specifically benefited from a rigorous investigation into the behavior of the structural elements to provide improved, low-cost retrofit solutions that satisfied the building owner’s needs.
ASCE-07 & 41 both have two main analysis approaches, linear and nonlinear. Linear analysis is the traditional approach as it is well understood, easy to model, and quick to derive results. The seismic demands for linear analysis are generated with assumed stiffness of the material and then an applied factor to account for ductility. This would be the m-factor in ASCE-41 or the R factor in ASCE-07. These ductility factors are based on testing and real-world observations, but remain based on an archetype structure leading to conservatism in their approach.
Alternatively, non-linear analysis tracks the actual material and element yielding, hardening, and weakening of the materials during a seismic event. This analysis requires more up-front time in the initial model setup, additional time to process and refine modeling, and often a third party peer review. This process can leverage the entirety of the structure’s existing strength, and unique structural solutions can be uncovered. In the right building, such as the Hacienda Apartments, this deeper understanding can lead to a targeted retrofit that has huge benefits for a project.
Both linear and nonlinear analyses were performed and brought through detailed design for the Haciendas Apartments project. Both options provided acceptable solutions, but the nonlinear provided critical project benefits that allowed the retrofit to be less costly and invasive.
The Hacienda Apartments is a 150-unit, 121,000 square-foot complex that served as low income senior housing for decades, but became severely deteriorated due to neglect. The complex consists of four structurally independent six-story, mild steel reinforced non-ductile concrete structures built in 1964, before modern seismic building codes were developed. Each building is rectangular in plan and configured to have an open-air walkway on one side and concrete walls on the other three.
Each building is constructed with 6.5-inch thick, two-way spanning concrete floor slabs supported by beams and columns at walkway edges, concrete columns spaced at 15 feet on center within the building interior, and concrete walls at the building exterior. The foundations consist of grade beams and isolated footings founded six feet deep to avoid expansive clay site soils.
Each building’s lateral force resisting system consists of 8-inch to 10-inch thick concrete walls. In the transverse direction, the buildings have solid continuous walls every 45 feet. The exterior longitudinal walls or perforated walls create a typical pier and spandrel beam system due to the multiple openings.
Given construction standards of the time, these structures present seismic performance issues such as insufficient gaps between buildings, floor slab bearing ledges susceptible to unseating, brittle seismic response at the perforated walls due to lack of confinement, foundation uplift and soil compression failures, and insufficient diaphragm connections to shear walls.
In the Design Development phase, a Linear Dynamic Procedure or LDP analysis (equivalent to an RSA in ASCE 07) was performed in ETABS. All perforated walls and solid walls were modeled using ACI cracked section recommendations, the foundation was pinned per ASCE allowances, and a distributed mass on the typical floors was used.
The results of the analysis indicated that the existing structures’ relative movement was too great, and unseating of the slab at the corbels would occur. Additionally, demand/capacity ratios for the perforated and solid walls showed that they would be severely overstressed and sustain significant damage. Under these loads, the foundations were also overloaded and prone to large movements—suggesting larger spread footings or a deep foundation system would be needed.
Adding enough stiffness to prevent the existing corbel seismic joints between the buildings from failing was not practical. To eliminate the likelihood of this catastrophic failure, the buildings were tied together at each floor and at every joint with steel collectors and a steel shear
link to make the buildings behave as one large building. To limit the stress on the existing walls, six 15-inch by 24-foot planar walls and four 24-inch by 50-foot shotcrete panels were overlaid to the existing wall to reduce the demand capacity ratios (DCRs) on the existing walls to the ASCE-41 acceptance criteria. The new walls were supported by large spread footings to ensure the soil bearing was acceptable.
A detailed set of plans and calculations for the design of seismic improvements, using the LDP was submitted to an independent peer reviewer in order to verify that the project met the necessary criteria. The intent was to meet the basic performance objective for existing (BPOE) buildings, as defined by ASCE 41, which is consistent with a Collapse Prevention (S-5) level of performance under the BSE-2E (~75% of MCE) hazard and the Life-Safety (S-3) level of performance under the BSE-1E (~75% of DBE) hazard. The criteria was established to meet the requirements of insurers and lenders for asset protection
This review showed the design approach was sound and supported the intended path forward. However, as the project proceeded through design, the project’s construction budget for the affordable housing development was outside of the available funding. A large portion of this was the structural retrofit and the added cost to mechanical/ interiors due to the extensive network of collectors. Significant cost savings were needed for the project to remain viable.
It was at this point that a nonlinear dynamic procedure (NDP) was leveraged to explore the possibility using insight from the more detailed approach to optimize the design and reduce the cost of the seismic improvements. A Perform 3D model was set up with all lateral elements modeled inelastically. Pier and spandrel elements represented the perforated walls with shear hinges, flexural hinges at the spandrels, and fiber section at the piers. The walls used inelastic shear and flexural elements. Additionally, inelastic collector elements and gap hook elements at the building joints were used to monitor the seismic joint. Existing and new foundations leverage soil springs to model soil yielding. All element and material properties used ASCE-41 recommendations and 11 ground motions were selected and scaled to match the target spectrum for the site. The analysis showed that the existing perforated walls had significant strength and stiffness, but were prone to a brittle shear failure in the piers at the first floor. It was also
observed that allowing some foundation flexibility showed a ductile rocking and flexural yielding mechanism in the larger planar walls. The perforated wall foundations were also found to be acceptable with soil flexibility. These observations allowed for the elimination of five of the 15-inch planar walls without overstressing the existing perforated or planer walls. Exterior overlays of the one planer and four perforated walls were kept to provide a mode shaping mechanism. This removed the brittle first floor shear failure and allowed flexural yielding throughout the existing wall providing a ductile and reliable mechanism.
The model also monitored the existing shear hinges at all levels. It was observed that collectors at the roof were attracting the most load. A study was performed with collectors only at the roof to see if the existing structure and added overlays were stiff enough to prevent the seismic joints from exhausting their allowable movement. The roof collectors were found to be sufficient, and collectors could be removed at all other floors. MCE ground motions were run to ensure the roof collectors and seismic joint movements were reliable. Through this process, Tipping confirmed that no ground motions exceed the collector design capacities or allowable movement of the joints. The collectors were placed on the roof of the building to separate the retrofit work from the interior work.
The application of advanced nonlinear analyses resulted in a tailored and optimized set of highly effective and cost-efficient seismic improvements for the vulnerable non-ductile concrete structure. The solution significantly reduced the cost of construction by fully harnessing the strength of the existing concrete structure, taking advantage of rocking foundations to reduce foundation demands,
and linking the structures together only at the roof, rather than at each floor level. This solution would not have been possible without detailed shaking simulations and relying on simpler linear-elastic elastic methods.
Ultimately, the structural engineering solution was a key component of making the affordable senior project viable by preserving and making the best use of the existing structure. With the LDP retrofit approach, estimates for the seismic retrofit were at $4.63 million and the targeted improvements and optimized approach were priced at $3.9 million at bid, allowing $730 thousand to be reallocated for other important project elements.
Secondary benefits included simplifying MEP coordination, coordinating architectural finishes, and limiting trade overlaps in interior areas by having the seismic retrofit only on the exterior of the building. This also allowed the seismic work to proceed simultaneously with the interior construction, significantly reducing the construction duration.
The insights of the NDP allowed these benefits to be leveraged and the moderate additional design fees and design time were more than offset with the benefits during construction. NDP made an innovative structural solution possible and brought a deteriorating complex back to life to once again provide safe, affordable housing for local seniors. ■
Structural Engineer: Tipping
Architect: PYATOK
Owner/ Developer: Mercy Housing California
Contractor: Nibbi Brothers
Peer Review: Charles Thiel, Telesis Engineers
Jason Armes, SE, is an Associate at Tipping and the Technical Lead for Hacienda Apartments.
Gina Carlson, SE, is an Associate Principal at Tipping and Project Director for Hacienda Apartments.
Leo Panian, SE, is a Principal at Tipping and Principalin-Charge for Hacienda Apartments.
• Cutting-Edge Equipment: New 48” technologically advanced glulam press and lay-up system.
• Versatile Production: Widths produced range from 2-7/16” to 10-1/2”, while depths range from 3-1/2” to 35-3/4”.
• Expanded Size Options: Finished Glulam Sizes up to 10 ½” x 35 ¾” x 60’.
• Diverse Applications: Available in various appearance classifications and strength grades for Commercial, Industrial, and Residential applications.
• Superior Quality: Manufactured with superior strength southern yellow pine MSR Lumber.
• Sustainability: Sustainable Forestry Initiative (SFI®) is certified for environmentally conscious projects.
During the seismic rehab project, a new basement story was created and the structure was prepared for base isolation.
By Lori Jue, SE, Michaella DeRubeis, PE, and Geoff Bomba, SE
As introduced in Parts 1 and 2 of this three-part series, the Oregon State Capitol Building Renovation project was designed to achieve the goals outlined in a 2009 master plan, which recommended seismic repairs, life-safety improvements, and renovations to improve the Legislature's operational efficiency. Seismic base isolation was chosen as the preferred approach given the historic nature of the 1938 Capitol structure and its associated brittle finishes and non-ductile structural elements. A significant challenge of base isolation is altering the vertical load
path for the building from its existing foundation system onto a new foundation that includes the seismic isolation bearings. Combined with implementing seismic isolation, a new structural level was added below the building to increase the square footage and add the much-needed hearing rooms and meeting spaces. Further, the adjacent 1977 Connector building was structurally tied into the 1938 building to create a single, larger, isolated building to maintain programmatic connections without large seismic joints between them and enhance the performance of the 1977 building.
The historic 1938 Capitol building is a large concrete frame structure with a central drum and rotunda over 350,000 square feet across four stories, including the new concourse level. The Senate and House chambers are two clear-story volumes flanking the rotunda symmetrically. The structure consists of non-ductile detailed cast-in-place concrete framing with limited areas of unreinforced masonry (URM) bearing walls. Floors consist of reinforced concrete pan joist slabs spanning to concrete beams supported by concrete columns. The central drum has a cylindrical shape with a concrete roof slab spanning to steel beams supported on a circular brick bearing wall. The existing lateral system is the non-ductile concrete frame infilled with red brick URM. The existing 1977 Connector building is also a non-ductile concrete building with concrete shear walls. It is a two-story building with a ground floor parking garage, hearing room spaces above, and a roof terrace.
The combined seismically base isolated structure is surrounded by a minimum 2’-0” seismic joint that allows the entire complex to move as a unit up to 2’-0” in any lateral direction. The plane of isolation is below the 1938 building’s new concourse level and courtyard infills, and at the north side of the existing 1977 Connector, transitions up to the top of the parking garage columns.
The owner’s performance goal for the seismic renovation is to have the Capitol building operational within 30 days following a seismic event so that the legislature can convene throughout the response and recovery timeframe. The retrofit uses the 2019 Oregon Structural Specialty Code (OSSC) and ASCE-41-17 – Seismic Evaluation and Retrofit of Existing Buildings as the main code standards used for the project. To meet the owner’s described performance goal and preserve the building’s historic nature, the chosen level of seismic performance is Immediate Occupancy (S-1) at the BSE-1N hazard level (475-year recurrence period) and Life Safety (S-3) at the BSE-2N hazard level (2475-year recurrence period). The chosen level
of non-structural performance is Position Retention (N-B) at the BSE-1N hazard level and Life Safety (N-C) at the BSE-2N hazard level. Combining the structural and non-structural performance levels gives the overall building performance level. These objectives aim to provide a retrofit with less damage to sensitive historic elements and allow quicker re-occupancy of the building. Since the seismic base isolators provide energy dissipation and damping of the seismic movement, the superstructure exhibits significantly lower drifts and ductility demands than fixed-base structures. This results in lower m-factors meeting the more stringent selected performance criteria. For acceptance criteria in ASCE 41-17 when using seismic isolation, there's a cap of 1.5 for m-factors used for Immediate Occupancy and Life Safety.
Although ASCE 41-17 generally does not require vertical seismic loads to be considered in the design of structural elements, there are two elements in this project where vertical seismic accelerations are considered: the post-tensioned gravity transfer system at the underside of Level 1 and the seismic isolators. To account for the vertical seismic component, ±0.2*Sxs*W is added to the gravity component during the post-processing of nonlinear response history analysis (NLRHA) load cases.
While seismic isolation has been used in the United States for roughly 40 years, the code still requires a peer review process that specifically reviews the isolation system and its associated design. This project used a collaborative phased peer review and permitting process. The process was broken into three main parts: isolators and Level 1 transfer system, building superstructure, and non-structural related components. This allowed the construction to begin earlier, as significant time was required to shore the building, and gave the design team additional time to coordinate non-structural elements.
The existing structure for the 1938 building was well documented and included both drawings and specifications. As part of the
initial 2014 investigations about the building in preparation for the seismic retrofit work, a material testing program verified the documentation from the original construction. This information followed ASCE 41-13 guidelines for material testing process and was extremely important to understand the existing strengths of the various elements. Two of the more unique testing types were for the red brick masonry to establish the brick material strength and the brick wall out-of-plane (OOP) strength.
The mortar used for the URM contained cement, which increased the capacity beyond typical mortar materials of that vintage. In addition to lateral resistance, the existing URM infill is relied upon for OOP bracing of the perimeter marble cladding through arching action. In-situ testing on the OOP capacity of the URM infill was performed using an "air bag" test in multiple locations. A reaction wall was built, and air bags were inflated to approximately 1.5 psi that corresponds to 216 psf (Figure 3). Results indicated that the walls had OOP capacity under this static load with no signs of damage, such as cracks, dislocations, or related spalls to the brick infill or marble cladding. Deformations were measured during the testing and determined to be negligible. The isolated building dynamic acceleration estimates were far below the tested values with large factors of safety and URM infill walls satisfied updated ASCE 41-17 prescriptive checks, which were not incorporated into the code at the time of material testing.
URM infill walls provide lateral stiffness and strength to the building through a behavior mode of compression struts and bedjoint sliding. URM was tested to determine the compressive strength (f'm) and the expected shear strength (vme) per ASCE 41-17. Despite the strength of the URM infill, new concrete shear walls were used to take the majority of the design base shear. The stiffness of the URM and its contribution to the building dynamics was considered and explicitly modeled in the analysis to capture its participation as part of the lateral system.
For the structural analysis, a 3D model was created that captured the existing building structural components and retrofit elements following ASCE 41 nonlinear response history (NLRHA) procedure. (Figure 4). The 158 base isolators were modeled using native link elements within ETABS analysis software with non-linear properties. As required by the code, both upper- and lower-bound properties of the isolation system are considered to determine the superstructure's
maximum forces and the isolation system's maximum displacements. All other elements in the analysis model are represented as linearelastic and use expected strength properties in accordance with ASCE 41-17. The linear-elastic modeling for the rest of the building is appropriate since the goal of the isolation system is to prevent damage in the structure and historic finishes. Non-structural components were not included as part of the analysis model. For the NLRHA, the geotechnical engineer for the project, GRI, completed a site-specific hazard analysis and provided two suites of 11 spectrally matched ground motions to capture the BSE-1N and BSE-2N hazard levels. These two suites of ground motions were run for both upper and lower bound isolator properties, and all element checks were done for both sets of models. In addition to the inherent damping included in the matched ground motions, modal damping of 3% was used for all modes except the first three isolator modes. The first three modes were assigned negligible damping as they do not have additional damping from the structure. It was determined through various analysis studies that over 15,000 modes were required to fully capture the behavior of the structure due to the inherent degrees of freedom of the nonlinear model.
Even though Salem, Oregon, is situated in a location susceptible to
subduction zone earthquakes, it is 50 miles from a major fault, resulting in lower relative seismicity than other seismically active regions such as coastal California. As a result, lower accelerations are expected at long building periods than for other base isolated projects in more seismically active regions. In 2014, when the initial design work for the retrofit project began, several systems for base isolation were studied, including friction pendulum systems, lead rubber bearings, and a hybrid system composed of lead rubber bearings, low friction slide bearings, and seismic ball bearings. In 2021, when the project's final phase was re-started, isolator systems had been further developed over the 7-year period. New studies were conducted and the Triple Pendulum friction isolator provided by Earthquake Protection Systems Inc. was selected for its bearings performance when considering the variation in seismic displacement demands in the different hazard levels, the high axial loads, and the lower seismic base shear required to protect the building.
Following ASCE 41-17, a bounding analysis captured the potential for variability in the isolation system at the time of installation far into the future. Modifiers to the isolator’s friction factors by decreasing or increasing the stiffness and strength of the isolation system bounded the overall seismic design. Typically, the lower bound isolator properties produce higher displacements (the system is more flexible), whereas the upper bound isolator properties produce higher base shears (the system is stiffer and closer to a fixed base building). However, due to the site seismicity and chosen bearing design, the lower bound isolator properties of the project's bearings resulted in higher displacements and higher base shears. This occurred because the upper bound system had higher damping and a shorter fundamental period than the lower bound system; combined, this produced a higher spectral acceleration at higher periods with low damping. After production testing of the bearings ultimately installed in the Capitol, the bearings were within the tolerances provided and used during design.
No sliding.
Sliding on inner slider only, surfaces Inner_Top and Inner_Bot
Motion stops on bottom inner slider, Inner_Bot. Sliding continues on top inner slider and outer slider surfaces, Inner_Top and Outer_Top
Motion stops on both Inner_Top and Inner_Bot surfaces. Sliding occurs on Outer_Top and Outer_Bot surfaces.
Outer slider limited by Outer_ Bot slider restrainer. Sliding on Inner_Bot and Outer_Top only, no motion on Inner_Top
Inner slider limited by Inner_Top and Inner_Bot slider restrainer. OUtside slider limited by OutTop and Outer_Bot slider restrainer
low enough that many of the existing materials comprising the original lateral system, such as URM infill, met prescriptive ASCE 41-17 checks and would not require extensive modification. The use of friction pendulum isolators achieved this goal, exhibited by both new and retrofit element forces that are within a reasonable threshold for design while also keeping isolator displacements within the project performance goals.
An additional design goal was to keep the isolation system within the limits of triple pendulum bearing behavior with no Phase V behavior (bearing rim contact) for any single ground motion and to keep the average of the maximum displacements for all ground motions within Phase 3 behavior (Figure 7). The Phase 3-4 transition occurs at roughly 16 inches of total displacement, and the Phase 4-5 transition occurs at approximately 19” of total displacement for the isolators used on this project. Even with no Phase V behavior, lower bound stiffness results showed higher base shear. This was further proven through the energy calculation of the hysteresis loop of the triple pendulum bearings, accounting for the effect of the radii of the surfaces and each surface having different coefficients of friction. The nominal coefficients of friction of the isolators used to achieve these design goals are 0.0085 for both the top and bottom inner slider surface, 0.033 for the bottom outer slider surface, and 0.060 for the top of the outer slider surface.
A critical part of achieving the owner’s performance goals for seismic renovation hinged upon overall building accelerations remaining
The nearly 115,000 Kip structure sits atop 158 triple friction pendulum isolators, 63 of which are isolators with a higher bearing capacity located in areas with higher axial load. These isolators have the same dish configuration allowing the two types of isolators to move together. Maximum isolator displacement of 19 inches and average isolator displacement of 13 inches are found using lower bound isolator properties.
Overall building accelerations remained below 0.20 g from the concourse level up to the roof of the main legislative chambers (Table 1). Above the roof, up to the top of the rotunda, where a large 17 Kip bronze statue sits, accelerations were notably higher than the rest of the structure, peaking at approximately 1.15 g due to the dynamic amplification caused by the change in stiffness at the dome. A study comparing fixed base and isolated behavior of the structure found that fixed base periods in the eastwest and north-south direction fell in the range of 0.5 seconds compared to the long period behavior of the isolated structure of approximately 3.5 seconds. The dramatic difference in building response of the fixed base structure results in significantly higher accelerations throughout the structure, which would not have allowed the project team to meet performance goals, thus necessitating the use of isolation.
As with any low friction isolation system, the building behavior should be evaluated for other components that can affect the movement of the building. For the Capitol building, the moat covers and nonstructural components were reviewed so that these elements did not have an unanticipated effect on the planned displacement and base shear. Nonstructural components, such as utilities crossing the isolation plane, are seismically jointed to make the movement of the heavy isolated structure unaffected. Stairs and elevators crossing the isolation plane are entirely separated and, in all cases, hung from the Level 1 isolated structure so that there is no contact with the fixed base portions of the building. For the unique case of the moat covers, the sliding surfaces created friction and “pop-up” forces that proved to be the highest level of force imparted on the movement of the building. While isolation is undoubtedly the cornerstone of this retrofit, seismic isolators alone are not a cure-all for addressing the historic structure's lack of strength and stiffness. It is the use of isolation combined with the additional retrofit elements, such as new reinforced concrete shear walls, critical collector elements, and fiber-reinforced polymer (FRP) strengthening, that allows this retrofit to meet and exceed the performance objectives.
With a target completion date of 2025, the Oregon State Capitol Building Project is currently under construction and supported by temporary shoring with portions of the new lower level and foundation installed. Like most voluntary seismic upgrades, this project was mainly driven by the complex interaction between structural engineering design goals and building upgrades. However, it is essential to consider even a predominantly structural renovation project within the context of the original building’s architecture and functionality to ensure success. The preservation of the existing structure of the building and the thoughtful incorporation of all new elements as part of the architectural design are crucial. The seismic retrofit, along with upgrades to accessibility and mechanical systems, has extended the life of this landmark building far into the future. This project was only made possible through the close collaboration of the architect, the structural engineer, and the general contractor from conception to construction. ■
See Parts 1 and 2 of this series in the March 2024 and April 2024 issues of STRUCTURE magazine.
Michaella DeRubeis, PE (m.derubeis@forell.com) is an Engineer with Forell
Geoff Bomba, PE, SE (g.bomba@forell.com) is a Principal and Director at Forell Elsesser Engineers in San Francisco, CA. He has over 20 years of experience in the seismic isolation of historic structures.
A charter high school in Northern California planned to make a large commercial building its new permanent home, but first the structure needed to be given a seismic upgrade without disrupting the other tenants.
By Nik Blanchette, SE; Steve Heyne, SE; and Chris Warner, SE
How do you navigate the requirement to retrofit an entire building when access is only readily available to a quarter of the floor plan due to the remainder being occupied? A charter high school in Northern California was looking for a permanent home. A great option was found in a large commercial building, however one caveat of using this space was that the introduction of a K-12 education occupancy meant that the entire building required a mandatory seismic upgrade to meet current code requirements. The IEBC notes that any increase in risk category triggers a current code update, though this may be enforced differently per your Authority Having Jurisdiction. The engineering challenge was how to design this seismic retrofit while minimally disturbing the tenants that occupied the other three quarters of the building.
The building is a single-story, steel-framed structure built in the mid-1980s as part of a technology campus. The floorplan includes a sprawling 115,000 square feet of space with some smaller concreteover-metal-deck mezzanines for mechanical systems and maintenance catwalks throughout. The roof is framed with bare metal deck over open web steel joists spanning to steel beams and girders, and solar panels cover a majority of the roof area. Perimeter walls are nonbearing, cold-formed metal stud walls with some non-structural precast concrete cladding panels. Ceiling spaces are full of mechanical ducts and piping.
The lateral force-resisting system is ordinary steel concentric braced frames. The ground floor braced frame connections are often partially embedded in the concrete floor slab (Fig. 1). The foundations consist of grade beams spanning to concrete piers with an interior slab-on-grade.
At the beginning of design of the upgrade project in 2017, the building was divided into multiple tenant spaces, including a food processor subject to frequent health department inspections. Any work outside of the vacant school tenant space needed to be performed on nights and weekends. Work inside of the food processor space was difficult to perform at all, due to the health department inspections and requirement to fully protect the space from construction debris contamination.
The space that the charter school wanted to occupy is approximately 30,000 square feet (Fig. 2), and due to the occupancy type and expected number of students, the introduction of this occupancy type changes the entire building from Risk Category II to Risk Category III, requiring the entire building to be upgraded to current seismic and wind requirements.
Two options for the seismic upgrade were considered: Using ASCE 7 provisions, similar to the design of a new building, or using ASCE 41 to evaluate and justify each existing element of the building individually. A preliminary analysis was performed, and ASCE 41 was selected to most efficiently capture the capacity of existing elements and reduce the work required to meet the performance objective. It was also helpful that no material sampling was required in accordance with ASCE 41 provisions since the original drawings specified all materials of interest. Material sampling can often add significant cost and schedule time when required.
Existing collector lines consist of wide flange beams. Approximately half of the collector lines required new bottom flange braces to increase compression capacity of beams. Existing bolted beam-beam and beamcolumn connections were generally acceptable as-is.
There are six existing braced frames in the building (Fig. 2); four in the east-west direction and two in the north-south direction. The brace members have a relatively high width-to-thickness ratio. To improve ductility of these members, grout was pumped inside. This reduces the “soda can” effect of wall crushing during a cyclic tension-compression seismic event. Only one brace type lacked adequate tension capacity; cross-sectional area was added by welding plate material to increase the cross-section.
Almost all existing braced frame connection details required strengthening. Deficiencies included cross sectional steel area being inadequate, weld strength being inadequate, and plate buckling strength being inadequate. Beveled bars were used to add weld over the top of existing welds without introducing new weld directly to the existing (Fig. 3). This also kept the new weld sizes smaller, allowing fewer passes. Some existing columns at braced frames lacked adequate compression capacity. This was remedied by increasing the radius of gyration with a welded WT section along the weak axis of the existing wide flange column.
Existing shear transfer to the foundation was adequate, however tension capacity of the anchor bolts was not. New anchors were added to existing base plates either by drilling new holes or adding gusseted plate extensions (Fig. 1). Fortunately, the existing foundations worked for the braced frames, precluding disruptive interior slab removal and foundation excavation.
In the east-west direction there are four existing braced frame lines in the building, one on each edge and one at each third point of the diaphragm span. Fortunately, the diaphragm was adequate in this direction, however the diaphragm attachment to the interior collector lines was not. Retrofit would involve removal of an 800-foot-long strip of roofing and insulation to add connections via welds or power actuated fasteners. Retrofit from below was considered, however, due to the tall height of the roof above finish floor as well as the presence of the ceiling and myriad mechanical
obstructions in the ceiling space, it was decided that installation from the top was the best option. Power actuated fasteners (PAFs) were chosen to reinforce the deck-to-collector connection. Due to stiffness compatibility issues between existing welds and new PAFs, the existing welds were neglected, and full load put on the PAFs.
In the north-south direction, the only existing lateral force-resisting lines are located at the edges of the building; there are no interior lines of support. The entire load path from metal deck diaphragm through to base of braced frame was significantly overstressed. Therefore, a new line of resistance was added down the middle of the building in this direction. New braced frames fit the programming of the new school tenant space well. The area was already completely open, allowing for clear construction space to install the long interior steel elements, as well as new foundations, including micropiles. However, the new school tenant space reached only a little less than half the length of the building in this direction. The remaining length was occupied by other tenants, primarily the food processor, for which installing overhead beams in their space was a nonstarter. The existing steel roof framing along the new collector line was a typical open web joist, only designed for gravity loads. The design team opted to install new framing on top of the roof to collect load from the diaphragm over the other tenants’ space and drag the load to the new braced frame (Fig. 4). A tee-shaped beam section was used for this new collector by installing one wide flange in a traditional, vertical orientation, but placing a second wide flange sideways on top of the first. This allowed for fewer top flange bracing connections down through the roofing. The two stacked beams were
placed atop the existing roof diaphragm to continue the collector line across the width of the building.
This solution was not without its own challenges. The building owner lost a number of photovoltaic panels where the new collector was placed. Existing surface-mounted utilities had to be lengthened to jog over top of the new several-feet-tall collector. New roofing penetrations were required to connect the new framing to the existing framing below. However, these tradeoffs were outweighed by the ability to allow the new tenant to occupy the space without disturbing the existing tenants.
To support the new braced frame, new hollow bar micropile foundations were installed. The demands to these foundation elements were high as the frame line resists the force from half of the existing building. Micropiles were a good fit for the soil type and for modular installation within existing building versus a traditional cast-in-place pier foundation that would have required a tall drilling mast and reinforcing cage.
Initially, the issue of the new school tenant invoking a mandatory building-wide seismic retrofit seemed infeasible. However, with some creative engineering and an unconventional solution that included adding a building-wide collector member on top of the roof framing, the school tenant was able to occupy this otherwise great space in an existing building. ■
Chris Warner, SE, is Principal at ZFA Structural Engineers, which is a Northern Californiabased firm that provides structural engineering services for a wide variety of projects including commercial, education, residential, healthcare, industrial, and specialty structures. ZFA has extensive experience with the seismic retrofit of all types of structures, including historic buildings. ZFA is celebrating its 50th anniversary in 2024.
ASCE standards provide technical requirements for promoting safety, reliability, productivity, and efficiency in civil engineering. The new ASCE Referenced Standards: IBC package offers users secure access to all ASCE standards referenced in the 2024, 2021, and 2018 editions of the International Building Code. The subscription includes:
Access to all 14 ASCE standards referenced in the IBC.
Referenced standards include key structural engineering guidelines including minimum design loads for structures, use of steel cables, cold-formed stainless steel design, flood design, structural fire protection, shallow foundations, wind tunnel testing, tensile strength, and seismic analysis.
The suite of interactive tools and feature-rich functionality that come standard with the ASCE AMPLIFY platform.
View the full list of standards at asce.amplify.org/ibc
In addition to the IBC-referenced standards, the AMPLIFY platform includes ASCE’s new Standard Practice for Sustainable Infrastructure, ASCE/COS 73-23, which provides design guidance to infrastructure owners, engineers, and contractors, to minimize the environmental impact and protect natural ecosystems, while simultaneously meeting the diverse needs of communities.
ASCE AMPLIFY is a powerful digital interactive platform designed to revolutionize your experience with ASCE’s engineering content. With ASCE AMPLIFY you receive onestop access to the most in-demand standards. You will experience the ease of working with the robust suite of interactive tools with a wide range of features and functionality.
One-click synchronizing between Provisions and Commentary with side-by-side display.
Redlining feature to quickly spot changes to previous editions.
Two-level search functionality: Site-wide search and product search.
Critical real-time incorporation of supplements and errata with date stamps.
Individual and corporate subscriptions available.
Under the umbrella of the Structural Engineering Institute, the Technical Community serves a vital role in bringing together various technical and standards committees within SEI to shape the future of structural engineering. The Technical Community—which combines the former Codes and Standards Activities Division (CSAD) with the Technical Activities Division (TAD)—was created to lend SEI members the space to contribute and collaborate on technical advancements and standards development, shaping engineering practice and addressing complex problems affecting the profession.
The reorganization has changed the way collaboration has been fostered between Technical and Standards committees. With the appointment of Greg Soules, Ph.D., P.E., S.E., F.SEI, F.ASCE as its inaugural chair, the Technical Community Executive
Committee has been steering toward the goals set out by the reorganization. Specifically, by combining standards and technical committees into one community, the Executive Committee is tasked with organizing and fostering growth with the technical heart and soul of SEI. Executive Committee members are working hard to ensure that resources are allocated to the projects that will best serve the structural engineering field and that this process is open and transparent with members.
Currently, the Technical Community committees are actively working on significant projects like ASCE 7, ASCE 41, and SE 2050 — a committee that is looking towards the future by leading sustainability initiatives and striving towards the global vision of net zero embodied carbon buildings by 2050.
“Reliable, resilient structures that people don’t have to worry about thinking whether
or not this building is safe “ says Technical Community Executive Committee member Greg T. Holbrook, P.E., highlighting the some of the goals of the Technical Community and the importance of sustainability in structural engineering. He emphasizes the need for structural engineers to consider sustainability, resiliency, and embodied carbon reductions in their designs, recognizing the impact they can have on the world and on the welfare of the public.
The Technical Community represents a commitment to collaboration, innovation, and leadership development within the engineering community. Through their collective efforts, engineers are shaping a future where unity and collaboration drive progress in structural engineering. Learn more about the Technical Community: https://go.asce.org/sei_awards.
By contributing to the SEI Futures Fund, you invest in the future of structural engineering, foster student interest, support younger members, and provide crucial professional development opportunities. The SEI Future Fund Board is currently reviewing 2025 proposals to select the best projects to support that align with these goals. Join us in shaping a better, more sustainable future for structural engineering. Donate today and make a lasting difference! Learn more at www.asce.org/SEIFuturesFund.
This award is given to promote the development of new insights and advancements in structural design and construction. Huge congratulations to the awardees for their hard work and dedication to the field. Learn more about the O. H. Ammann Research fellowship recipients and their projects at https://www.linkedin.com/showcase/structuralengineeringinstitute/.
Ever wished for a chance to shape and inspire the next generation of engineers? SEI invites you to apply for a position on the SEI Education and Leadership Committee. As a member, you will influence the direction of structural engineering education by developing state-of-the-art curricula and
programs, leading initiatives that promote leadership skills and professional growth within the SEI community and collaborating with a diverse group of professionals dedicated to advancing the field.
Apply online with QR code! Select “SEI Professional Community” from drop down and then select “SEI Education and Leadership Committee.” Applications are being accepted until September 1, 2024.
Following the publication of ASCE/SEI 41-23 Seismic Evaluation and Retrofit of Existing Buildings, the committee is reconstituting its roster to begin work on the 2029 edition. ASCE/SEI 41 is now accepting new members for the next edition of the standard.
Apply online with QR code! Select “SEI” from the drop down and then select “ASCE 41.” Applications are being accepted until September 1, 2024.
Bill Wallace, with over 40 years of experience, details the impacts of climate change on infrastructure and the necessary changes for resilience. The book covers the importance of infrastructure in economic development, the connection between sustainability and climate resilience, and the need for new engineering standards. It offers practical advice on communicating with clients about climate change, managing climate uncertainty with tools like the Robustness Matrix, and creating sustainable designs. Wallace, an environmental engineering pioneer, brings unparalleled expertise, making this book a blueprint for building a sustainable and resilient future. Print available for preorder.
SEI is currently accepting proposals to modify the 2022 edition of ASCE/SEI 7 Minimum Design Loads Associated Criteria for Buildings and Other Structures, as the committee prepares for 2028 revision cycle. The committee will accept public proposals until December 1, 2024. The Public Proposal Form is available from SEI via sei@asce.org.
Registration is now open for the 2024 NCSEA Structural Engineering Summit, taking place on Nov. 5–8, 2024, at the MGM Grand Hotel in Las Vegas, Nevada. This premier event will bring together structural engineering professionals from across the United States for a comprehensive conference focused on education, networking, and innovation.
The Summit offers attendees the chance to connect with other structural engineers and industry experts through a comprehensive program featuring more than 14 hours of education sessions. These sessions will provide the latest insights and best practices shaping the field today. Additionally,
the expansive exhibit hall will showcase cutting-edge technologies and services, offering innovative solutions for professional practice.
Programming will cover a wide range of topics, including best design practices, new codes and standards, project case studies, advanced analysis techniques, management practices, diversity and inclusion, resilience, and sustainability.
A highlight of the Summit is the celebration of prestigious NCSEA awards, recognizing outstanding achievements in structural engineering. This event—now included with registration—features a reception, awards presentation, and after-party, offering
ample networking opportunities in a festive atmosphere.
“The Structural Engineering Summit is an unparalleled opportunity for professionals to learn, discover new solutions, and celebrate excellence in our field,” said Al Spada, NCSEA Executive Director. “We are excited to bring this dynamic event to Las Vegas and provide a platform for structural engineers to enhance their skills and build their networks.”
The MGM Grand Hotel, in the heart of Las Vegas, offers an exceptional venue for the Summit, with world-class entertainment, award-winning cuisine, and luxurious accommodations. To learn more and to register, visit www.NCSEASummit.com.
Glenn R. Bell, P.E., S.E., a key investigator in the Champlain Towers South collapse, will deliver the closing keynote at the 2024 NCSEA Structural Engineering Summit in Las Vegas. Bell will present “Update on NIST’s National Construction Safety Team Investigation of the Champlain Towers South Partial Collapse” at 11 a.m. PT on Friday, November 8.
The June 24, 2021, partial collapse of Champlain Towers South is one of the most tragic structural failures in U.S. history. The National Institute of Standards and Technology (NIST) formed a National Construction Safety Team (NCST) to
investigate the incident, aiming to determine the collapse’s technical causes and recommend changes to prevent similar disasters.
Bell’s keynote will explore NCST’s systematic approach, including site investigations, evidence testing, document analysis, interviews, laboratory testing and advanced computer simulations. Preliminary results of the investigation will be shared. Find more information here.
Bell has worked on other significant projects including the 1981 Hyatt Regency Hotel walkways collapse and analyses of the World Trade Center Towers 1 and 2 collapses. He
has received numerous awards for his contributions to structural safety and currently serves as Director of Collaborative Reporting for Safer Structures – U.S.
The NCSEA Structural Engineering Summit is Nov. 5–8, 2024, at the MGM Grand Hotel in Las Vegas, Nevada. Registration is open at www.NCSEASummit.com.
The NCSEA SEA Grant Program is now open for applications. Designed to foster growth and innovation within Structural Engineers Associations (SEAs), these grants fund impactful projects that advance the field of structural engineering. For 2024, grants can be requested for any program aligned with the NCSEA
Foundation’s mission. All SEAs or their members are eligible to submit applications, but they must first be reviewed and approved by local SEA leadership before being forwarded to the NCSEA Foundation for consideration. A subcommittee of the NCSEA Foundation Board of Directors will select the recipients.
For detailed application information, submission guidelines, and to apply, visit www. ncsea.com/foundation/advocacy/sea-grants. The deadline is Sept. 13, 2024.
The NCSEA Foundation advances the science and practice of structural engineering by supporting NCSEA, state SEAs, and practicing structural engineers.
Nearly 100 SEA leaders, NCSEA committee chairs, and NCSEA Board Members gathered in suburban Chicago on June 4–6 for NCSEA Leadership Week, a collaborative event aimed at supporting the profession today and tomorrow.
The first two days focused on the NCSEA Leadership Collaboration, where the Board of Directors and Committee Chairs aligned on initiatives and strategic planning. Discussions emphasized enhancing engagement between committees and their communities to develop valuable tools for practicing structural engineers, ensuring unified leadership. Following this, the SEA Leadership Retreat provided SEA leaders and NCSEA Delegates with essential knowledge in association management and practical tools for local applications. The event connected SEA leaders from more than 30 SEAs with NCSEA’s committees, highlighting key resources available year-round and encouraging diverse leadership growth.
“Leadership Week is an exceptional opportunity for the NCSEA board, staff, committee
chairs, and SEA leaders to come together in person to collaborate and to exchange ideas and resources to collectively propel the profes sion forward,” said NCSEA Board Member Michelle Ryland, S.E., R.A., who attended the
2024 event. “Together, we can accomplish so
Sponsored by Atlas Tube and MiTek, Leadership Week also featured an evening reception where attendees celebrated NCSEA’s 30th anniversary.
More than 40 structural engineers from Richmond and Virginia Beach attended the Structural Engineers Association of Virginia’s (SEAVa) annual Demo Days on May 8 and 9. Attendees experienced new products and tried their hand at installing bolts and anchors. The event was sponsored by Hilti, Simpson Strong-Tie, and the Southeast Concrete Masonry Association, with additional presentations from Lindapter, Weyerhaeuser, and MTC Solutions.
Visit www.ncsea.com/education for the latest news on upcoming webinars and other virtual events.
July 18 Codes, Standards, and the Practicing Engineer: Seismic and Wind
July 30
August 1
IBC Structural Changes Part 1: Structural Design Loads
IBC Significant Structural Changes Part 2: Materials and Temporary Structures
Purchase an NCSEA webinar subscription and get access to all the educational content you’ll ever need! Subscribers receive access to a full year’s worth of live NCSEA education webinars (25+) and a recorded library of past webinars (170+) – all developed by leading experts; available whenever, wherever you need them!
CASE has committees that work together to produce specific resources available to members, from contract documents to whitepapers, to help your business succeed.
If you are a member of CASE, all CASE publications are free to you. NCSEA and SEI members receive a discount on publications. Use discount code - NCSEASEI2022 when you check out.
Check out some of the new CASE Publications …
In today’s world, survey after survey has listed flexible work schedules and work location as a top desire of employees at all levels. Many companies are currently struggling through this shift and trying to determine what works best for the company in the long term as there are many pros, cons, and important considerations. In addition, structural engineering firms are having more difficulty filling positions and expanding the candidate pool beyond the local office locations could yield high quality employees. These options do create business, insurance, technical, IT/security, productivity, training/mentoring and cultural challenges. This Tool attempts to provide some guidance in these areas.
A critical component of talent management is succession planning. Training, development, career planning (employee-centered), career management (organization-centered), and replacement planning are all key elements of succession planning. Successful succession planning helps an organization cope with talent scarcity, identify skill gaps and training needs, promote knowledge transfer to retain institutional knowledge, increase morale and retention by investing in employees, and create bench strength for unique and highly specialized skill sets.
This tool provides guidelines and tips for the verification of analysis and design software results. It focuses on linear elastic structures and small deformation/small strain analysis which represent the vast majority of design office work.
An important aspect of a joint project pursuit between a contractor or design professional and a structural engineer is an agreement covering the activities of the parties prior to contract award. This agreement is commonly referred to as a teaming agreement. Teaming agreements are often associated with design-build projects but can be used on any project pursued jointly by two or more parties. Many organizations familiar to structural engineers provide a standard form teaming agreement. This commentary summarizes the contents and typical clauses of the standard form teaming agreements offered by these four organizations:
• American Institute of Architects (AIA): Contract C102-2015
• Engineers Joint Contract Documents Committee (EJCDC): Contracts D-580 and E-580
• Design-Build Institute of America (DBIA): Contract No. 580
• Consensus Docs: Contract Nos. 296 and 498.
You can purchase these and other Risk Management Tools at https://www.acec.org/member-center/get-involved/coalitions/case/resources/
Is there something missing for your business practice? CASE is committed to publishing the right tools for you. Have an idea? We’d love to hear from you!
August 8-9, 2024, Minneapolis, Minnesota
The CASE Summer Meeting will be in Minneapolis, Minnesota this year. The meeting will feature breakout sessions for the CASE Committees, interactive discussions on structural engineering and business resources, education sessions, and more. Registration is open now.
Now more than ever we need to support the upcoming generation of the workforce. Give to the CASE Scholarship today!
Questions you always wanted to ask.
By Jennifer Goupil, PE
This quarterly article addresses some of the questions received about structural standards developed by the Structural Engineering Institute (SEI) of the American Society of Civil Engineers (ASCE). Questions from engineers, building officials, and other design professionals are often considered to develop future editions.
This month includes many topics about the code adoption process for ASCE referenced standards. These topics and more are discussed on the ASCE Peer-to-Peer Standards Exchange Forum. ASCE/SEI members can ask and answer questions in the forum. Visit https://collaborate.asce.org/standardsexchange/home to learn more and read about other topics.
ASCE 7-22 U pdates in the 2024 IBC
Question: The 2024 International Building Code (IBC) is available now and my jurisdiction is starting the process of review and adoption of new I-Codes. How and who decides what updates from ASCE 7-22 will be included in the new IBC?
Answer: This is a great question. The International Code Council defines the process for updating their International Codes, also referred to as the I-Codes, one of which is the International Building Code. Answering the question of how ASCE 7 becomes adopted into the IBC, the process includes several steps, summarized to include participant submittals of code change proposals, followed by several rounds of committee hearings and public comments to adjudicate the change proposals. ASCE/SEI staff, along with ASCE 7 committee members, prepare the proposals and attend the hearings. This process occurs every three years and therefore the publication of ASCE 7 is timed so that it can be adopted by reference into every other edition of the I-Codes. For example, ASCE 7-22 is adopted by reference into the 2024 I-Codes, and ASCE 7-16 was adopted into the 2018 I-Codes. Similarly, the next edition of ASCE 7, which will be 2028, is
planned for publication to be adopted into the 2030 I-Codes.
To clarify what is submitted as a change proposal, the ASCE/SEI proposals primarily are focused on updating the loading and other criteria – including the many environmental hazard maps—within the I-Codes that is defined in ASCE 7.
Changes in ASCE 7 for the IBC
Question: I would like to make a change in ASCE 7 for the International Building Code. How do I submit that?
Answer: The Q/A above briefly summarizes the process for updating the IBC, however, if anyone has an idea for a change or clarification for ASCE 7, the committee accepts public proposals for the first two years of the cycle. Public proposals for changes to ASCE 7-22 are being accepted until Dec. 1, 2024. If you have an idea, contact sei@asce.org and request the ASCE 7-22 Public Proposal Form. All proposals received will be adjudicated by the committee in accordance with ASCE Rules.
Question: I am confused by the new term in the IBC for allowable stress design ground snow loads, p g(asd). When should this be used?
Answer: Great question! Let’s start with the change in ASCE 7-22. The snow maps included in this current standard are reliability-targeted design ground snow load maps. This represents a significant change from ASCE 7-16 and prior editions, which used ground snow loads with a 50-year mean recurrent internal. Why this change was made is detailed in the Chapter 7 Commentary, however it is important to understand that the corresponding load combinations in Chapter 2 have also been modified to reflect this important change and the load factors have been revised to 1.0S for strength design and to 0.7S for allowable stress design
Snow Details
Ground Snow Load, pg 39 lb/ft2
20-year MRI Value, pg 11.49 lb/ft2
Winter Wind Parameter, W2 0.45
Mapped Elevation 4994.5 ft
Data Source ASCE/SEI 7-22, Figures 7.6-1 and 7.6-2 A-D
Values provided are ground snow loads. In areas designated "case study required," extreme local variations in ground snow loads preclude mapping at this scale. Numbers in parenthesis represent the upper elevation limits in feet for the ground snow loads at elevations not covered.
Snow load values are mapped to a 0.5 mile resolution. This resolution can create a mismatch between the mapped elevation and the site-specific elevation in topographically complex areas. Engineers should consult the local authority having jurisdiction in locations where the reported 'elevation' and 'mapped elevation' differ significantly from each other.
Ground Snow Loads for IRC Only, Pg(asd) 27.3 lb/ft2
to appropriately represent the reliability basis of the new ground snow values.
The snow maps in the IBC have been updated to reflect (and match) the new strength-based snow maps in ASCE 7-22. Because of this change, we added a new section in the 2024 IBC, 1608.2.1 Ground snow conversion to provide continuity with conventional construction provisions within the I-Codes. This section states:
Where required, the ground snow loads, p g , of Figures 1608.2(1) through 1608.2(4) and Table 1608.2 shall be converted to allowable stress design ground snow loads, pg(asd), using Equation 16-17.
p g(asd) = 0.7 p g (Equation 16-17)
Where:
p g(asd) = Allowable stress design ground snow load.
p g = Ground snow load determined from Figures 1608.2(1) through 1608.2(4) and Table 1608.2.
This new term was added to provide a simple conversion for existing conventional construction tables and equations in the 2024 International Residential Code (IRC), and therefore the IRC could continue to be used with the existing pointers back to the IBC for the ground snow values. The new term should not be used with ASCE 7-22 Section 2.4 Load Combinations for Allowable Stress Design because those combinations have a factor of 0.7S, which is intended to be used with the pg values from the reliability-targeted ground snow values defined in Chapter 7. Nor should this term be used with Section 1605.2 Alternative
allowable stress design load combinations for the same reasons. In summary, the pg(asd) conversion should only be used with the IRC, and in collaboration with the conventional construction stakeholders, this value has been added to the ASCE Hazard Tool (ascehazardtool.org) and identified as Ground Snow Loads for IRC only, pg(asd). ■
This article’s information is provided for general informational purposes only and is not intended in any fashion to be a substitute for professional consultation. Information provided does not constitute a formal interpretation of the standard. Under no circumstances does ASCE/ SEI, its affiliates, officers, directors, employees, or volunteers warrant the completeness, accuracy, or relevancy of any information or advice provided herein or its usefulness for any particular purpose. ASCE/SEI, its affiliates, officers, directors, employees, and volunteers expressly disclaim any and all responsibility for any liability, loss, or damage that you may cause or incur in reliance on any information or advice provided herein. If you have a question you want to be considered in a future issue, please send it to sei@asce.org with FAQ in the subject line. Visit asce.org/sei to learn more about ASCE/SEI Standards.
Jennifer Goupil, PE, F.SEI, F.ASCE, is the Managing Director of the Structural Engineering Institute and the Chief Resilience Officer for the American Society of Civil Engineers.
Temporary Structures (IBC Chapter 31)—Part 9
By John “Buddy” Showalter, PE, and Sandra Hyde, PE
This multi-part series discusses significant structural changes to the 2024 International Building Code (IBC) by the International Code Council (ICC). This article includes an overview of changes to IBC Chapter 31 on temporary structures. Only a portion of the chapter’s total number of code changes is discussed. More information on the code changes can be found in the 2024 Significant Changes to the International Building Code available from ICC.
Special requirements that apply to temporary structures are included in IBC Section 3103. For applying the applicable code provisions, “temporary” is considered as being erected for a period of less than 180 days. The International Fire Code (IFC) also has a significant number of provisions applicable to such
structures. Additionally, the IBC identifies key criteria that must be applied to temporary structures, including conformity with structural strength, fire safety, means of egress, accessibility, light, ventilation, and sanitary requirements. Permits are typically required, as are construction documents. The scope of IBC Section 3103 includes special event structures, tents, umbrella structures, and other membrane structures.
STRUCTURE. Any building or structure erected for a period of one year or less that serves an assembly occupancy or other public use.
SERVICE LIFE. The period of time that a structure serves its intended purpose. For temporary
structures, this shall be the cumulative time of service for sequential temporary events which may occur in multiple locations. For publicoccupancy temporary structures this is assumed to be a minimum of 10 years.
TEMPORARY EVENT. A single use during the service life of a publicoccupancy temporary structure at a given location which includes its installation, inspection, use and occupancy, and dismantling.
TEMPORARY STRUCTURE. Any building or structure erected for a period of 180 days or less to support temporary events. Temporary structures include a range of structure types (public-occupancy temporary structures, temporary special event structures, tents, umbrella and other membrane structures, relocatable buildings, temporary bleachers, etc.) for a range of purposes (storage, equipment protection, dining, workspace, assembly, etc.).
3103.1 General. The provisions of Sections 3103.1 through 3103.4 3103.8 shall apply to structures erected for a period of less than 180 days. Temporary special event structures, tents, umbrella structures and other membrane structures erected for a period of less than 180 days shall also comply with the International Fire Code. Those Temporary structures erected for a longer period of time and public-occupancy temporary structures shall comply with applicable sections of this code.
Exceptions:
1. Public-occupancy temporary structures complying with Section 3103.1.1 shall be permitted to remain in service for 180 days or more but not more than 1 year where approved by the building official.
2. Public-occupancy temporary structures erected within the confines of an existing structure are not required to comply with Section 3103.6.
3103.1.1 Extended period of service time. Public-occupancy temporary structures shall be permitted to remain in service for 180 days or more without complying with requirements in this code for new buildings or structures where extensions for up to 1 year are granted by the building official in accordance with Section 108.1 and where the following conditions are satisfied:
1. Additional inspections as determined by the building official shall be performed by a qualified person to verify that site conditions and the approved installation comply with the conditions of approval at the time of final inspection.
2. A qualified person shall perform follow up inspections after initial occupancy at intervals not exceeding 180 days to verify the site conditions and the installation conform to the approved site conditions and installation requirements. Inspection records shall be kept and shall be made available for verification by the building official.
3. An examination shall be performed by a registered design professional to determine the adequacy of the temporary structure to resist the structural loads required in Section 3103.6.
4. Relocation of the public occupancy temporary structure shall require a new permit application.
5. The use or occupancy approved at the time of final inspection shall remain unchanged.
6. A request for an extension is submitted to the building official. The request shall include records of the inspections and examination in Items 1 and 3.
3103.1.1 3103.1.2 Conformance. (No changes to text)
3103.1.2 3103.1.3 Permit required. (No changes to text) (No changes to IBC Sections 3103.2 through 3103.4)
3103.5 Bleachers. Temporary bleachers, grandstands and folding and telescopic seating that are not building elements shall comply with ICC 300.
3103.6 Structural requirements. Temporary structures shall comply with the structural requirements of this code. Public-occupancy
temporary structures shall be designed and erected to comply with the structural requirements of this code and Sections 3103.6.1 through 3103.6.4.
Exception: Where approved, live loads less than those prescribed by Table 1607.1 shall be permitted provided a registered design professional demonstrates that a rational approach has been used and that such reductions are warranted.
Temporary non-building structures ancillary to public assemblies or special events structures whose structural failure or collapse would endanger assembled public shall be assigned a risk category corresponding to the risk category of the public assembly. For the purposes of establishing an occupant load for the assembled public endangered by structural failure or collapse, the applicable occupant load determination in Section 1004.5 or 1004.6 shall be applied over the assembly area within a radius equal to 1.5 times the height of the temporary non-building structure.
3103.6.1 Structural loads. Public-occupancy temporary structures shall be designed in accordance with Chapter 16, except as modified by Sections 3103.6.1.1 through 3103.6.1.6
3103.6.1.1 Snow loads. Snow loads on public-occupancy temporary structures shall be determined in accordance with Section 1608. The ground snow loads, pg , in Section 1608 shall be modified according to Table 3103.6.1.1.
Exception: Ground snow loads, pg , for public-occupancy temporary structures that employ controlled-occupancy procedures per Section 3103.8 shall be permitted to be modified using a ground snow load reduction factor of 0.65 instead of the ground snow load reduction factors in Table 3103.6.1.1.
Where the public-occupancy temporary structure is not subject to snow loads or not constructed and occupied during times when snow is to be expected, snow loads need not be considered, provided that where the period of time when the public-occupancy temporary structure is in service shifts to include times when snow is to be expected, one of the following conditions is met:
1. The design is reviewed and modified, as appropriate, to account for snow loads.
2. Controlled-occupancy procedures in accordance with Section 3103.8 are implemented.
3103.6.1.2 Wind loads. The design wind load on public-occupancy temporary structures shall be permitted to be modified in accordance with the wind load reduction factors in Table 3103.6.1.2.
Exceptions:
1. Design wind loads for public-occupancy temporary structures that implement controlled occupancy procedures per Section 3103.8 shall be permitted to be modified using a wind load reduction factor of 0.65.
2. For public-occupancy temporary structures erected in a
hurricane-prone region outside of hurricane season, the basic wind speed, V, shall be permitted to be set as follows, depending on Risk Category:
2.1. For Risk Category II: 115 mph.
2.2. For Risk Category III: 120 mph.
2.3. For Risk Category IV: 125 mph.
3103.6.1.2 Reduction Factors for Wind Loads for Public-Occupancy Temporary Structures
be inspected when purchased or acquired and at least once per year. The inspection shall evaluate individual components, and the fully assembled structure, to determine suitability for use based on the requirements in ESTA ANSI E1.21. Inspection records shall be kept and shall be made available for verification by the building official. Additionally, publicoccupancy temporary structures shall be inspected at regular intervals when in service to ensure that the structure continues to perform as designed and initially erected.
3103.6.4 Durability. Reusable components used in the erection and the installation of public-occupancy temporary structures shall be manufactured of durable materials necessary to withstand environmental conditions at the service location. Components damaged during transportation or installation or due to the effects of weathering shall be replaced or repaired.
3103.7 Serviceability. The effects of structural loads or conditions shall not adversely affect the serviceability or performance of the publicoccupancy temporary structure.
3103.6.1.3 Flood loads. Public-occupancy temporary structures need not be designed for flood loads specified in Section 1612. Controlled occupancy procedures in accordance with Section 3103.8 shall be implemented.
3103.6.1.4 Seismic loads. Seismic loads on public-occupancy temporary structures assigned to Seismic Design Categories C through F shall be permitted to be taken as 75 percent of those determined by Section 1613. Public-occupancy temporary structures assigned to Seismic Design Categories A and B are not required to be designed for seismic loads.
3103.6.1.5 Ice loads. Ice loads on public-occupancy temporary structures shall be permitted to be determined with a largest maximum nominal thickness of 0.5 inch (13mm), for all risk categories. Where the public-occupancy temporary structure is not subject to ice loads or not constructed and occupied during times when ice is to be expected, ice loads need not be considered, provided that where the period of time when the temporary structure is in service shifts to include times when ice is to be expected, one of the following conditions is met:
1. The design is reviewed and modified, as appropriate, to account for ice loads.
2. Controlled occupancy procedures in accordance with Section 3103.8 are implemented.
3103.6.1.6 Tsunami loads. Public-occupancy temporary structures in a tsunami design zone are not required to be designed for tsunami loads specified in Section 1615. Controlled occupancy procedures in accordance with Section 3103.8 shall be implemented.
3103.6.2 Foundations. Public-occupancy temporary structures shall be permitted to be supported on the ground with temporary foundations where approved by the building official. Consideration shall be given for the impacts of differential settlement where foundations do not extend below the ground or foundations supported on compressible materials. The presumptive load-bearing value for public-occupancy temporary structures supported on a pavement, slab on grade or on other collapsible or controlled low-strength substrate soils such as beach sand or grass shall be assumed not to exceed 1,000 pounds per square foot (47.88 kPa) unless determined through testing and evaluation by a registered design professional. The presumptive load-bearing values listed in Table 1806.2 shall be permitted to be used for other supporting soil conditions.
3103.6.3 Installation and maintenance inspections. A qualified person shall inspect public-occupancy temporary structures that are assembled using transportable and reusable materials. Components shall
3103.8 Controlled occupancy procedures. Where controlled occupancy procedures are required to be implemented for public-occupancy temporary structures in Section 3103.6.1, the procedures shall comply with this section and ANSI ES1.7. An operations management plan in accordance with ANSI E1.21 shall be submitted to the building official for approval as a part of the permit documents. In addition, the operations management plan shall include an emergency action plan that documents the following information, where applicable:
1. Surfaces on which snow or ice accumulates shall be monitored before and during occupancy of the public-occupancy temporary structure. Any loads in excess of the design snow or ice load shall be removed prior to its occupancy, or the public-occupancy temporary structure shall be vacated in the event that either the design snow or ice load is exceeded during its occupancy.
2. Wind speeds associated with the design wind loads shall be monitored before and during occupancy of the public-occupancy temporary structure. The public-occupancy temporary structure shall be vacated in the event that the design wind speed is expected to be exceeded during its occupancy.
3. Criteria for initiating occupant evacuation procedures for flood and tsunami events.
4. Occupant evacuation procedures shall be specified for each environmental hazard where the occupant management plan specifies the public-occupancy temporary structure is to be evacuated.
5. Procedures for anchoring or removal of the publicoccupancy temporary structure, or other additional measures or procedures to be implemented to mitigate hazards in snow, wind, flood, ice, or tsunami events.
ANSI E1.21-2020 Entertainment Technology: Temporary Structures
Used for Technical Production of Outdoor Entertainment Event
ANSI ES1.7-2021 Event Safety Requirements - Weather Preparedness Change Significance: Four new definitions have been added to the IBC: temporary structures, public-occupancy temporary structures, service life, and temporary event. Public-occupancy temporary structures are new buildings or structures that are used by the public, or that support public events, where the public expects similar levels of reliability and safety as offered by permanent construction. Publicoccupancy temporary structures are often assembled with re-useable components and designed for a particular purpose and limited period, which is defined as a temporary event when the period is less than one
year. Public-occupancy temporary structures in service for a period that exceeds 1-year must meet IBC requirements for new buildings.
While temporary structures are developed for use up to 180 days, many of these structures are used repeatedly at different locations. Thus, their actual service life may be on the order of 5 to 10 years. Such structures are typically subjected to repeated assembly and dismantling with associated wear and tear. Therefore, service life for temporary structures is defined to provide a consistent basis of reliability relative to that of new buildings, and a service life of 10 years is assumed for public-occupancy temporary structures when determining snow and wind load reductions in IBC Section 3103.6.
IBC Section 3103.1.1 deals with extension of a public-occupancy temporary structure’s service time. The owner and their "qualified person" are to perform any additional inspections required by the building official, and ongoing inspections every 6 months. For the ongoing inspections, the qualified person must keep records should the building official wish to review them. These provisions are comparable to the process for special inspections in IBC Chapter 17, where a building official relies on a special inspector or agency for many of the details of construction. Relocation of a public-occupancy temporary structure (not all temporary structures) requires the owner to apply for a new permit. A request for an extension must be submitted to the building official, and reports resulting from inspections by the qualified person and the registered design professional's examination must be submitted along with the request.
New IBC Section 3103.6 differentiates between temporary structures and its subcategory public-occupancy temporary structures. The latter has new specific provisions for structural loads, foundations, installation and maintenance inspection, durability and maintenance of reusable components, serviceability, and controlled occupancy procedures.
Temporary non-building structures (such as stands supporting lights, audio equipment, cameras, etc.) which are associated with either public assemblies or special event structures need to be assigned a risk category consistent with the risk category of the public assembly. Applicable occupant load determination per IBC Section 1004.5 or 1004.6 is to be applied over the assembly area within a radius equal to 1.5 times the
height of the temporary non-building structure. This is consistent with recommendations from the California Building Officials Association for the “fall zone” around buildings damaged in earthquakes when conducting building safety evaluations. Those recommendations suggest that building safety evaluators cordon off or barricade for a distance of 1.5 times the height of a damaged building in danger of collapsing to protect the public from building materials that can also shatter and bounce. Determination of the risk category for each temporary non-building structure in a public assembly area is to be done for each structure individually, not cumulatively, as it is unlikely that simultaneous failure of multiple structures will occur during an event. See the risk category determination example shown in Figure 1 containing cases of minimum areas and heights assigned to pole-type temporary non-building structures.
Public-occupancy temporary structures should not pose more risk to occupants than permanent structures, but because the code's design-level environmental loads are far less likely during a temporary event, these new provisions reduce certain structural load requirements for a consistent level of risk. Minimum uniformly distributed live loads and concentrated loads can be reduced where a registered design professional calls for such reductions based on a rational approach.
Recognizing the relatively short service life of temporary structures, the ground snow load can be reduced to reflect the comparatively low probability that the ASCE/SEI 7 Minimum Design Loads and Associated Criteria for Buildings and Other Structures ground snow loads will occur during the shorter service life of a temporary structure. If controlled occupancy procedures are implemented, an even larger reduction of ground snow loads is permitted. If the service life of the temporary structure will not occur during months when snow is to be expected, snow loads need not be considered.
In hurricane-prone regions outside of hurricane season, the basic wind speed can be prescriptively set at 115 mph for RC II, 120 mph for RC III and 125 mph for RC IV structures. For nonhurricane areas, the wind load reduction factors shown in Table 3103.6.1.2 are believed to provide a reasonable level of reliability, given the ability to evacuate or modify temporary structures for strong wind events. If controlled occupancy procedures are
implemented, an even larger reduction of wind loads is permitted.
Seismic loads on public-occupancy temporary structures assigned to Seismic Design Categories C through F can be taken as 75 percent of those required by IBC Section 1613 which results in reduced seismic performance relative to permanent structures. This is consistent with the reduction for the evaluation and upgrade of existing buildings and will result in a similar seismic risk to occupants.
Public-occupancy temporary structures can be supported on the ground with temporary foundations where approved by the building official. Consideration is needed for the impacts of differential settlement. Presumptive load-bearing values for structures supported on pavement, slab on grade, beach sand or grass cannot be assumed to exceed 1,000 psf unless otherwise determined by a registered design professional. IBC Table 1806.2 soil bearing values are applicable for other typical supporting soil conditions.
Provisions for controlled occupancy in IBC Section 3103.8 address cases where an environmental loading hazard cannot be reasonably mitigated and allow for actions based on a preapproved action plan that a building official may use to permit installations that cannot resist code prescribed loads. For example, public-occupancy temporary structures subject to high wind loads may be evacuated and sections of the structure can be removed to reduce the wind load and prevent components from becoming projectiles. Where such plans are required, they must comply with ANSI ES1.7 and an operations management plan in accordance with ANSI E1.21 shall be submitted to the building official for approval as a part of the permit documents. ANSI E1.21 includes requirements for monitoring weather forecasts for high winds, tornadoes, thunderstorms, lightning, and other severe conditions, as well as a requirement for mitigating actions for ice and snow to be specified in the operations management plan.
Temporary structures are designed to be assembled and disassembled and transported to different locations as components or as modules. They may be in service during varying weather conditions. Components may be damaged during transportation or installation. Components also may have been manufactured more than a decade prior to the latest use. Therefore, and unlike a new structure that is typically constructed with new building materials and components that were not previously used, components for temporary structures need to be inspected regularly and their suitability for reuse needs to be assessed. This is typically done by the installation crews, and this is similar to bleachers regulated by ICC 300 Standard on Bleachers, Folding and Telescopic Seating, and Grandstands. The qualified person assigned to perform the regular inspections is identified by the owner.
Additional related technical and editorial changes throughout the IBC including IBC Section 108.1 and multiple sections of IBC Chapter 16 are not shown or discussed here. See code change S116-22 with committee modifications on the ICC website for more details.
Structural engineers should be aware of significant structural changes in the 2024 IBC Chapter 31 on temporary structures. New definitions, reduced environmental loads, as well as installation and maintenance inspections are now included for temporary structures. ■
John “Buddy” Showalter, PE, M. ASCE, M. NCSEA (bshowalter@iccsafe.org) is a Senior Staff Engineer and Sandra Hyde, PE, M. ASCE, M. NCSEA (shyde@iccsafe.org) is Managing Director of ICC’s Consulting Group.
By John A. Dal Pino
The nation is half-way through the 2024 election year. I am already looking forward to 2025!
I recently listened to a podcast regarding free speech and open debate. The message was that the public needs to be actively involved and help shape outcomes, basically play defense in the face of a powerful offense. Otherwise, eventually, advocacy groups, through their use of language to shape an issue, and with the support of the media, will establish the “truth” and define acceptable outcomes. Speaking out or taking alternative positions “once everything is settled” will make the speaker an outlier, a kook, a flat earther, etc. This got me to thinking about structural engineering.
I know that many people, particularly engineering and technical types, would rather avoid the political fray, finding anything of a political nature uncomfortable. I suspect the politics they prefer to avoid are the petty, vindictive, clique-centered high school B.S. type. I agree. Better to just keep your head down, avoid bad memories and do your work. But politics is defined (Merriman Webster Dictionary), as “the total complex of relations between people living in society.” This means that politics is everywhere,
24/7, 365. Basically, part of the human experience. Basically unavoidable, whether at home or at the office. Since the “world is run by those who show up” as the saying goes, get ready for politics.
Let’s look at how debate and politics impacts structural engineering and more broadly the construction industry. Individuals and groups are constantly advocating for their views, services, and products, hoping to establish what the rest of us ought to believe, how we ought to act, how we ought to spend our money, etc. The unfortunate reality is that once the battle over the “truth” is settled by the unrelenting pressure of the dominant narrative, or because there is no effective response (people throw up their arms and surrender), it is not easy to express alternative opinions. People with non-conforming opinions will be accused of spouting misinformation or even worse, being a counterrevolutionary tool. If you have spent any time studying history, you know where being characterized as a counterrevolutionary is likely to get you.
Following is a list of topics impacting the AEC industry that I believe need to be examined more thoroughly and more actively
debated before there is no going back. This is just my list. Each reader will likely have his or her own.
Although there is substantial literature on the char resistance of mass timber and the safety of such construction based on tests, the predominate long-term trend has been toward more fire-resistive construction, not less. Code provisions for tall wood buildings are still relatively new and changing. This process will continue as the AEC industry and regulators learns more from actual fire experience.
Each reader will likely have his or her own list of issues. We would love to hear from you.
There is no shortage of opportunities for the construction of low and mid-rise wood buildings of all types, using stick framed and mass timber designs. For taller buildings, however, I argue the focus should be on using steel and concrete for taller buildings. In some cases, it can be quite smart to be skeptical instead of an early adopter. For example, it took a long time for those who objected to the code-mandated design of welded flange (not bolted) steel moment frame connections to be proven right.
It could be argued that the discovery of natural pozzolan cement by the Romans and the invention of Portland cement has changed the course of history. What would the world look like without cement, or cement mortar? Buildings would be constructed from wood, mud bricks, sticks and mud and stone. A vast majority of the world would still be living in the stone age.
We as structural engineers ought to help society move on from the notion that phasing out Portland cement is inevitable. It is a low-cost option and plentily available. An alternative is to factor all costs of production and anynegative effects into the price. A realistic goal would be to make improvements at the margins and use alternatives supported by the free market.
Housing is almost always the largest household expense and is almost never easily affordable, particularly as judged by first-time home buyers. I remember signing the paperwork along with my wife for our first home and thinking “How are we going to afford this?” Then baby #1 came along and panic set in, since all of our family’s savings went into the down payment.
The dominant narrative today is that there is an affordability crisis primarily due to a shortage of new housing construction. However, like many issues, affordability is influenced by many factors, including what people can earn based on their skillsets.
The unavoidable truth is that developers want to maximize the value of their land and are constrained in terms of what they build by local land-use regulations and the building code. Many government entities directly subsidize the cost of housing but that is not a long-term solution, since eventually, someone pays. One of the questions for architects and engineers to ponder is whether, after excluding what they can’t control (like the price of land), building code provisions also exacerbates the affordability issue.. The long-term trend is for more bells and whistles in construction, improved performance in all sorts of hazards, better occupant comfort, and improved energy efficiency, etc. The result is more expensive structures. For example, the cost of new housing in San Francsico runs about $1,000 per square foot. If the purchase price (or the rent) can’t cover the construction cost and a profit, the unit isn’t built.
Should we consider building code provisions aimed at shorter anticipated lifespans, more damage in extreme but rare events, fewer required amenities, etc.? A lower total cost would go a long way toward improving affordability.
I am concerned that the change from long, single-day and two consecutive-day exams to half-day, multiple-day, multiple-part exams could come back to haunt us. If the exam writers are allowed to break a subject up into enough small parts taken over an extended period, arguably almost everyone will eventually be able to “pass” the test given enough studying and re-take opportunities. Do we want to lower our standards of the engineering professional talent pool?
Personally, I want design professionals to be the absolute best, based on their knowledge, performance and ability to make good decisions, and I argue that the single or two consecutive day exam is a key indicator of ability.
As I mentioned above, this is just my opinions on certain issues for further discussion. You may or may not agree. Each reader will likely have his or her own list of issues. We would love to hear from you. Don’t be bashful, jump right in. Offer an opinion.
By Dr. Frank Griggs, Dist. M. ASCE
The Milwaukee and Mississippi Railroad built a 20-milelong line from Milwaukee to Waukesha, Wisconsin, as early as 1851 and extended its line through Helena to Potosi on the Mississippi River in 1857. Later they built a connection from Helena to Prairie De Chien. The line fell on hard financial times and was resurrected as the Milwaukee & Prairie De Chein Railroad. The Minnesota Central Railroad laid track from Minneapolis south across the Minnesota River to Mendota and thence southerly towards Iowa, where it connected with the McGregor Western Railway that had its origin in McGregor (now Marquette) Iowa and built a line from Owatonna, Minnesota, to LeRoy, Minnesota. That line was in turn sold to the Milwaukee & St. Paul Railroad in late 1867. When this line ran a branch south to Chicago, it became known as the Chicago, Milwaukee and St. Paul Railroad in 1874. So in 1874, the same company had a network of tracks east and west of the Mississippi. They made use of car ferries and tracks laid on the ice in the winter time but a bridge was needed to speed up crossing the river.
Section 6 of the July 25, 1866 Chapter 246 An Act to authorize the Construction of certain Bridges, and to establish them as Post Roads stated,
Sec. 6. And be it further enacted, That a bridge may be constructed across the Mississippi River between Prairie du Chien, in the State of Wisconsin, and North McGregor, in the State of Iowa, with the consent of the legislatures of Wisconsin and Iowa, on the same terms and subject to the same restrictions as are contained in this act for the construction of the bridge at Quincy, Illinois.
At the site, the river was about 7,000 feet shore to shore and divided by an island. John Lawler had a contract with the railroad to transport cars across the river and to charge tolls for use of his ferry. He had initially developed a system to carry the rail cars across the river using barges with tracks lashed to each side of a steamer, each barge carrying four cars. When the steamer and barges reached the other side, the cars would be transferred to the main line of track. This method was only available during periods when the river was not frozen over with ice. On October 10, 1872, Lawler was granted the franchise to carry cars across the river and formed the Prairie Du Chien & McGregor Railway Company. After running the cars onto the barge for the 2,000 feet distance to the island, he then used the same barges pulled by cable into a slip where the cars rolled on to a track to cross the island to the next barge that would take them across the westerly branch to Iowa over the McGregor Channel for another 1,500 feet. The barges were pulled across both branches by cables hooked to steam engines. Both branches of the river were navigable. This was still unsatisfactory, and Lawler was looking for another solution.
Up to this time, the solution to the bridging problem was either to build a high-level bridge with a 50-foot vertical clearance and 300-feet and 250-feet spans, or a low-level bridge with swing spans and a clearance on both sides of the swing span pier of 160 feet. Either of these solutions would have been very expensive for a man of limited means.
Lawler told an old friend D. J. Whittemore about his new plan, “I ought to do this work a great deal cheaper and safer than I am now doing it by steamboat and barges, and the idea has occurred to me that I should accomplish the desired end by the agency of a pontoon.” Whittemore told him about the 303-foot-long bridge that had been built at Rouse’s Point, New York, across an outlet of Lake Champlain by Henry Campbell in 1851. It had low level pile bridges flanking a movable pontoon span that was moved by steam power. Lawler, with that information, retained Michael Spettel to design a movable pontoon bridge for the Mississippi. One of the main problems was that the water level of the Mississippi could vary as much as 22 feet between high and low water. The pontoon would float at an elevation dictated by the level of the river but the spans on either side of the pontoon would be fixed. This required a form of ramp at each end to allow the locomotives and cars to run up to or down to the pontoon. What he arrived at on the western channel was a low-level trestle out to the 409-foot movable pontoon bridge followed by more trestle to the island. From the island, another lowlevel trestle was built out to another 409-foot movable pontoon bridge followed by more trestle to the eastern bank of the river. Spettel, with little help from Lawler, experimented with models until coming up with a solution to the problem of variable levels of the river. He designed the spans to cross the river at a sharp angle rather than perpendicular in order to have the current help swing the span open. To close the span, he connected the end of the movable span with a chain back to a steam engine. His plans were submitted to the War Department, and Gen. A. A. Humphreys said in transmitting a report he had prepared for the Senate Commerce Committee, “I now beg leave to present his report upon the subject, by which it appears that while the bridge in question is exceptionally free from objection, as an obstruction to the navigation, it only conforms to existing laws regulating the bridging of the Mississippi River, in that it affords excellent facilities for steamers and rafts to pass through the draw-openings. In view of this fact, it is suggested that in legalizing the construction of the bridge it should be required that the draw-openings be maintained without any reduction of their existing widths.”
In a report dated May 6, 1874, Col. J. N. Holcomb wrote:
draw have not only complied with the requirement but even increased the lengths of each of the draws, and that the bridge as constructed seems to be in accordance with the requirements of the act approved 4th of June, 1872, ‘further regulating the construction of bridges across the Mississippi River,’ as far as concerns the ready passing of boats and rafts through said bridge. At the same time it should be remarked that the draw-openings alone afford the way of passing through, and that such a bridge as the above-described combination of pile-work and pontoons can only be authorized across the Mississippi River by the enactment of a special law as proposed, and the act should be entitled an act to legalize and establish a pile bridge with pontoon-draws upon which to locate a railway across the Mississippi River and in view of the fact that the draw-openings at each channel are single, the width which said draws respectively have, as described in this report, shall not be diminished. In relation to the navigation, I have to state that the captains and pilots unanimously opine this bridge to be the easiest to pass on the whole river, and consider it not to be obstructive to navigation.”
The act to legalize the bridge was Chapter 224, June 6, 1874: “An Act to legalize and establish a Pontoon-Railway – Bridge across the Mississippi River at Prairie De Chien, and to authorize the construction of a similar bridge at or near Clinton, Iowa.” The bridge opened in late 1874.
Lawler gave a presentation of his bridge to an American Society of Civil Engineers Convention in St. Paul on June 30, 1883, that was followed by a long discussion between himself and other civil engineers. In it he noted, “The structure was built at about one - sixth of the estimated cost of an ordinary pivot bridge at the same point.”
“In reference to navigating the draws, I state that both are located in good, deep water, but the direction of the current strikes the eastern draw at an angle of 75° 30’, and the western channel at an angle of 55°. It will be therefore seen that the benefit of the length of the 396 feet in the eastern draw and the length of 408 feet in the western draw practically is reduced to 383 feet and 334 feet, viz, 396 feet x sin 75° 30'=383 feet and 408 x sin 55o=334 feet. The bill pending in Congress in relation to this bridge demands not less than 250 feet for the eastern draw, and not less than 320 for the western draw. It will be therefore seen that the builders of the bridge and
What Lawler and Spettel had done was to design and build a bridge across the Mississippi with little precedent and had succeeded on a first try in adopting an old but little used style of bridge. He received a patent, with no recognition of Spettel, on his bridge on August 11, 1874, #154,055. The pontoons were updated in 1914 and 1916 and served until 1960 when the rail company failed, after which the bridge was dismantled. ■
Dr. Frank Griggs, Dist.M. ASCE, specializes in the restoration of historic bridges, having restored many 19th Century cast and wrought iron bridges. He is now an Independent Consulting Engineer (fgriggsjr@verizon.net).