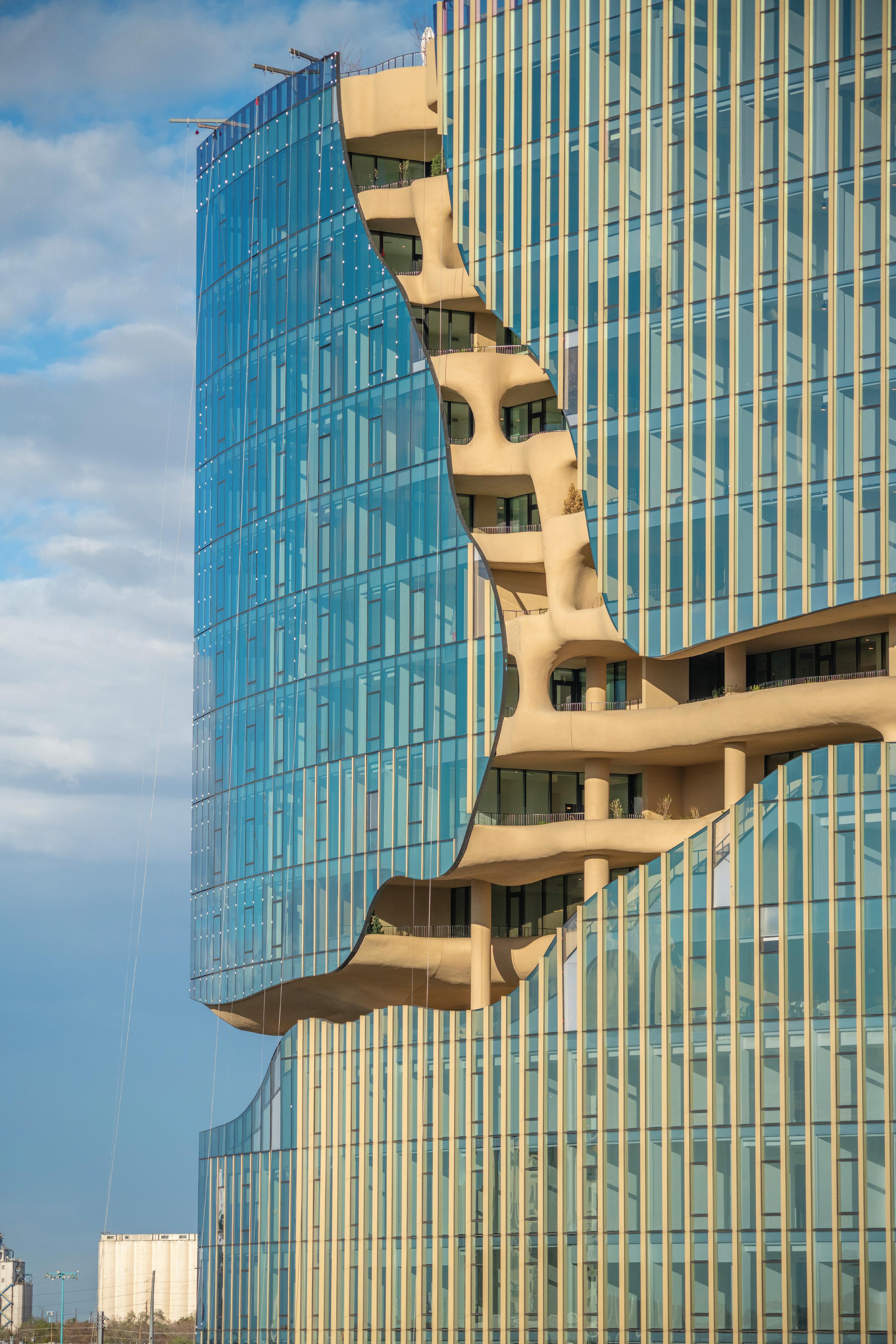
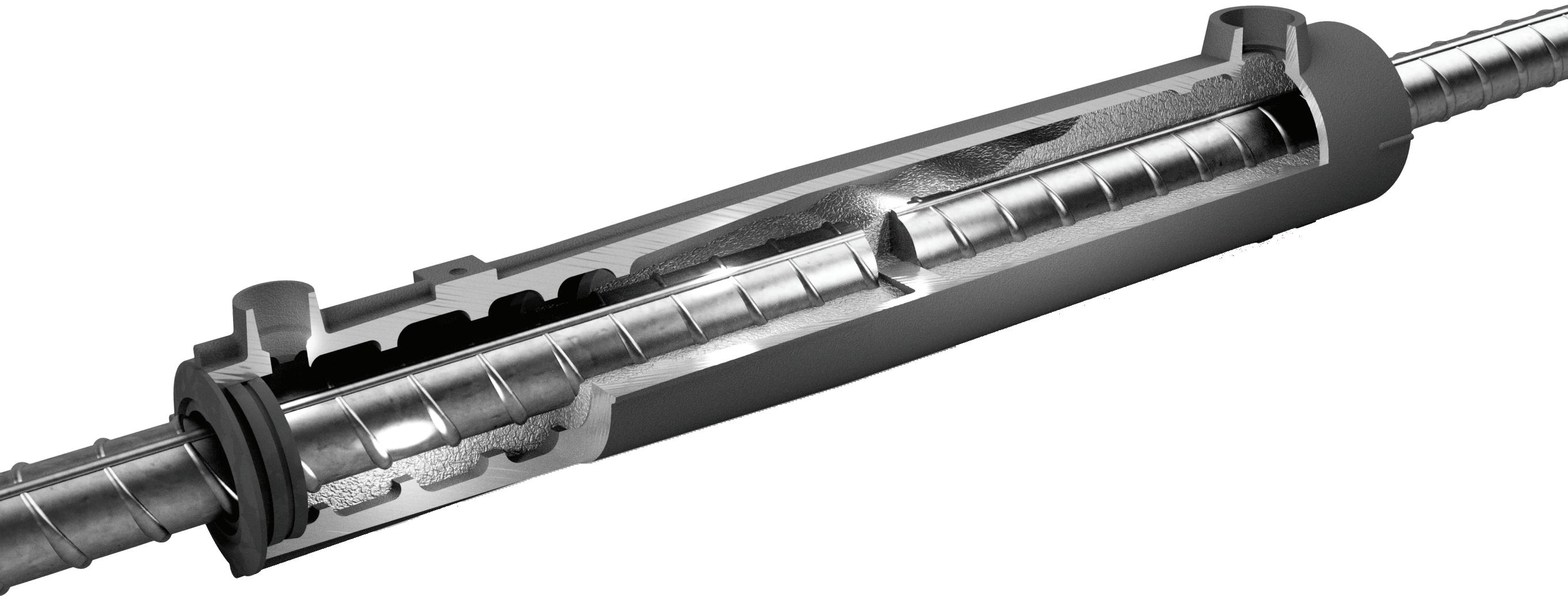
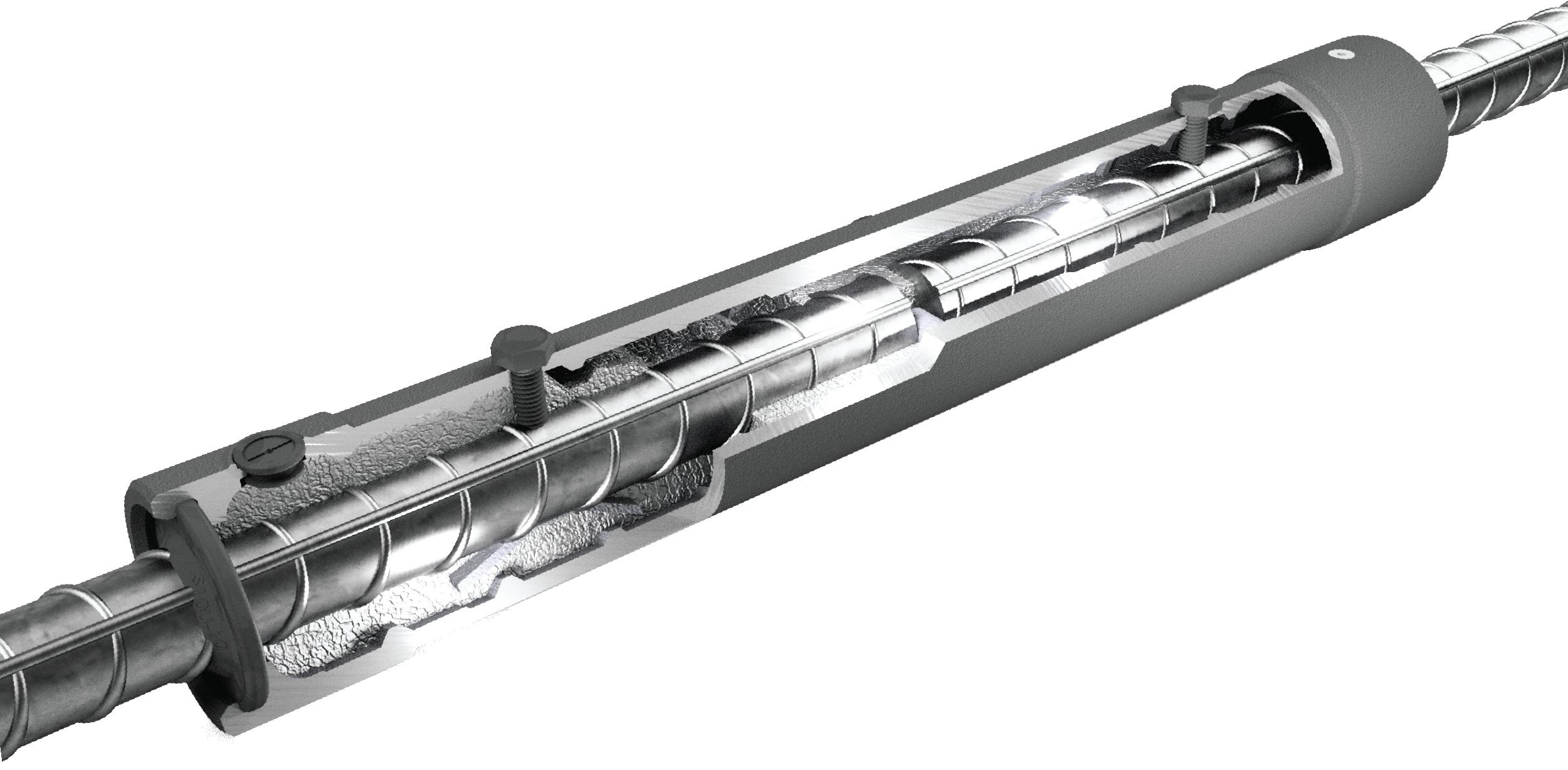

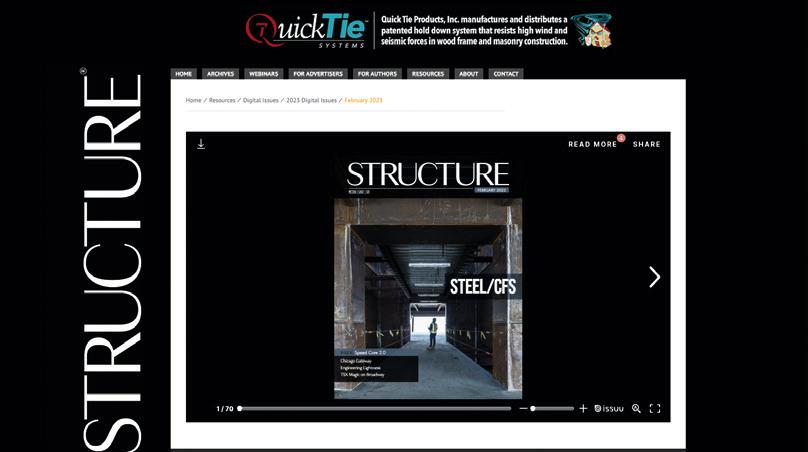
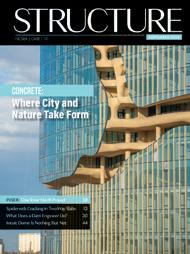
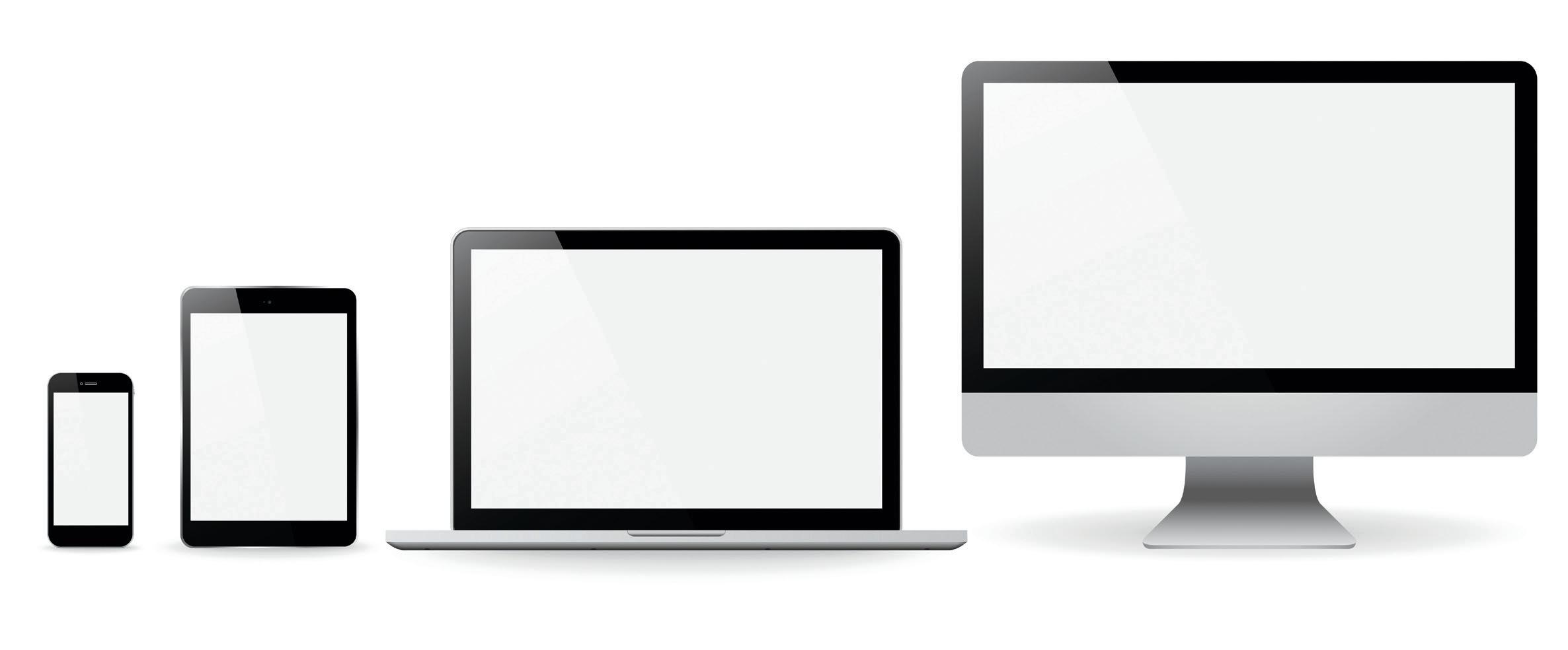
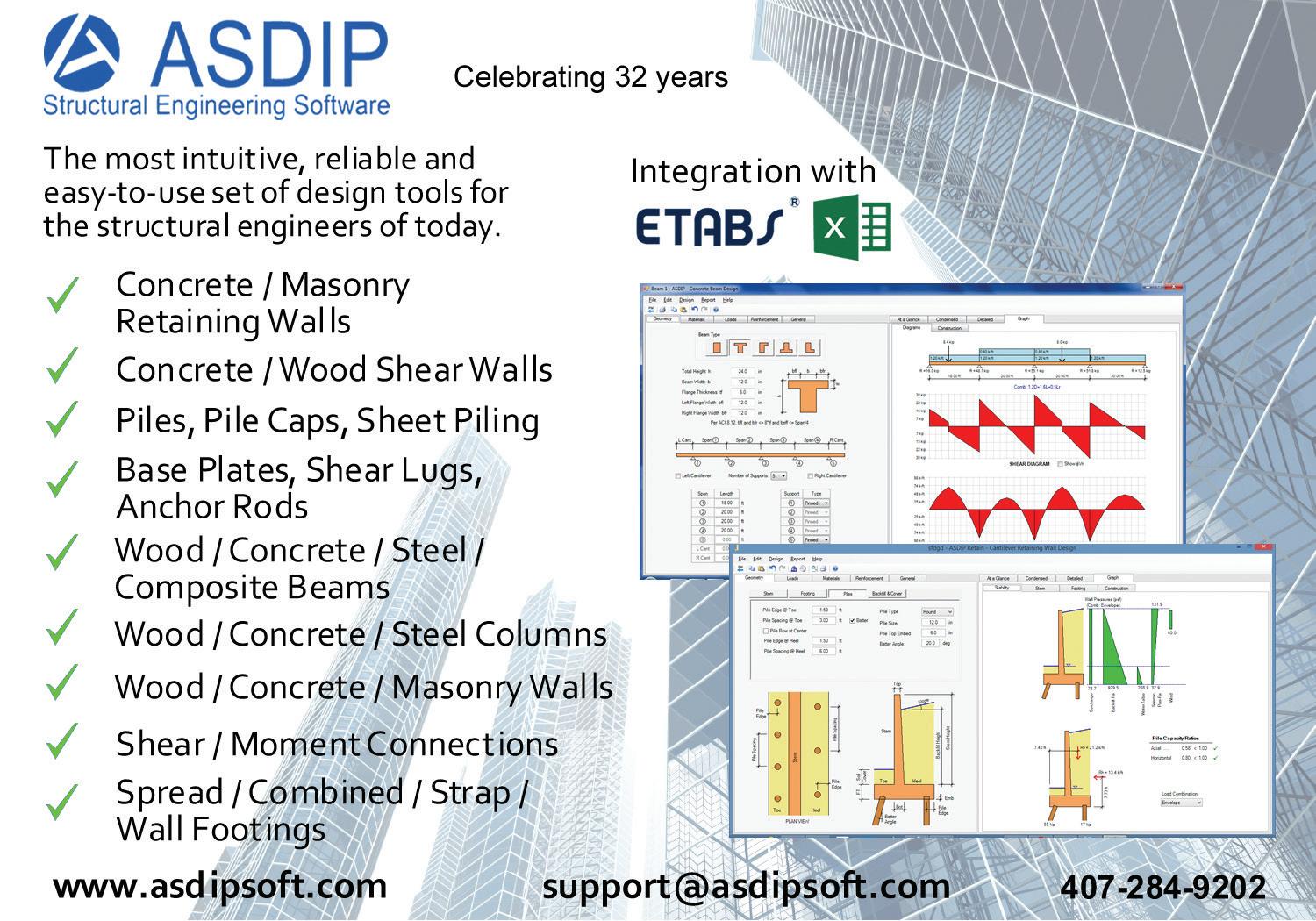
subscriptions@structuremag.org
Chair John A. Dal Pino, SE Claremont Engineers Inc., Oakland, CA chair@STRUCTUREmag.org
Marshall Carman, PE, SE Schaefer, Cincinnati, Ohio
Erin Conaway, PE AISC, Littleton, CO
Sarah Evans, PE Walter P Moore, Houston, TX
Linda M. Kaplan, PE Pennoni, Pittsburgh, PA
Nicholas Lang, PE Vice President Engineering & Advocacy, Masonry Concrete Masonry and Hardscapes Association (CMHA)
Jessica Mandrick, PE, SE, LEED AP Gilsanz Murray Steficek, LLP, New York, NY
Brian W. Miller
Cast Connex Corporation, Davis, CA
Evans Mountzouris, PE Retired, Milford, CT
Kenneth Ogorzalek, PE, SE KPFF Consulting Engineers, San Francisco, CA (WI)
John “Buddy” Showalter, PE
International Code Council, Washington, DC
Eytan Solomon, PE, LEED AP Silman, New York, NY
Jeannette M. Torrents, PE, SE, LEED AP JVA, Inc., Boulder, CO
Executive Editor Alfred Spada aspada@ncsea.com
Managing Editor Shannon Wetzel swetzel@structuremag.org
Production production@structuremag.org
Director for Sales, Marketing & Business Development
Monica Shripka Tel: 773-974-6561 monica.shripka@STRUCTUREmag.org
• Concrete Repair Mortars
• Corrosion Protection
• Construction Grouts
• Waterproofing
• Sealants and Joint Fillers
• Coatings and Sealers
• Epoxy Adhesives
• Cementitious Flooring Systems
• Cure and Seals
• Densifiers
• Structural Strengthening Products
• Precast
• Epoxy Adhesives
Visit www.mapei.com/us for details on all MAPEI products. Your single-source provider for construction
MAPEI offers a full range of products for concrete restoration, waterproofing and structural strengthening. Globally, MAPEI’s system solutions have been utilized for such structures as bridges, dams, tunnels, highways, parking garages, stadiums and high-rises.
By Austin Reese, PE
Coordination, collaboration, and creativity were required to meet the structural engineering challenges created by the building's unique canyon feature.
Geology, hydrology, hydraulics, and more go into the structural engineering puzzle of each dam project.
By Bryan Seamer, SE, and John Wilson, SE
On a tight, urban site, LPA’s West Hollywood Aquatics and Recreation Center (ARC) uses inventive structural engineering to create space for community and well-being.
Simpson Strong-Tie provides comprehensive product solutions for commercial institutional construction. Whether you’re building an educational facility, healthcare center or public office, you can rely on a full array of products that are assembly-tested and proven for strength and versatility. From cold-formed steel and structural steel to adhesive and mechanical anchors, code-listed connectors and fastening systems, we have what you need to build safer, stronger structures. Our nationwide distribution network ensures product availability and timely jobsite delivery. Along the way, our expert field, technical and sales support teams are always ready to assist you. With Simpson Strong-Tie as your partner, you have a total solution designed to elevate performance. To learn more about our complete solutions for institutional construction, visit strongtie.com/institutional or call (800) 999-5099.
Carl Josephson, PE,
Terrence Paret, Hayley Proctor, PE, Gwenyth Searer, PE, SE, and Prateek Shah, PhD
By Tim Lane and Cris Moen, Ph.D, PE
Dr. Ibrahim M. Metwally, Ph.D,
By Carl Josephson, PE, SE
There are eleven member boards in NCEES (National Council of Examiners for Engineering and Surveying) that require an engineer to have a Structural Engineer license to design some or all the buildings or bridges in their jurisdictions. There are four other states in which use of the title Structural Engineer is restricted to engineers licensed as a Structural Engineer. It has become increasingly clear that engineers who practice structural engineering across state lines or who design buildings that are commonly called “significant structures,” need to become licensed as a Structural Engineer. As with all engineering licensing, the minimum requirements involve the “three-legged stool” of education, experience, and examination. The examination for would-be Structural Engineers is now the NCEES PE Structural Exam that is used by all jurisdictions that license SEs.
To improve the exam, make it relevant and more in keeping with typical office practice, NCEES needs licensed practicing structural engineers (including newly licensed engineers) to participate in the process.
The exam went from being a two-part (16hour) exam that tested vertical design one day and lateral design the next, to a four-part (21-hour) exam that takes at least four days to complete. The previous exams had the breadth portion (vertical or lateral) in the morning and the depth portion of the respective section in the afternoon. Previously, the exam was graded with a “hybrid compensatory grading system” which meant that one could not get an acceptable result in either vertical or lateral if they did especially well in the multiple choice portion and bombed the “paper and pencil” portion. Now the four sections (vertical breadth, lateral breadth, vertical depth, and lateral depth) are all graded separately, reported separately, and acceptable results are required for each. The first administration of the new computer-based NCEES PE Structural exam took place this year, with the depth portion of the exam in April. For the first administration of the new SE exam, the pass rates for the breadth (multiple choice) portions of the exam were in the 50% range, while the pass rates for the depth (alternate item type) portions were in the 15% range. The pass rates for the breadth sections were slightly higher than what has been seen in the past for the combined score, and the pass rates for the depth sections were lower.
That said, common concerns that have been raised by examinees who recently took the exam were that the use of the computer-based references were clunky and slow, only one window could be open at a time, some references did not have bookmarks, it was reported that one couldn’t go back to a problem later, and time seemed too short for the exam.
The licensing committees of SEI, NCSEA, and CASE have been monitoring the exam, and fielding concerns and complaints. The committees or their members have discussed these issues with NCEES, who has been open and receptive, and is looking to continually improve the exam. Here is some of the feedback that we received:
For exam security, we will never see personal reference material come into or out of the exam rooms again. The solution is providing all references that the examinee needs in a searchable digital format. Unfortunately, the proprietary software used by Pearson VUE test centers does not allow more than one window open at a time. For the foreseeable future, one will not be able to have two references open simultaneously, and there are no plans by Pearson VUE to rewrite their software or to provide multiple monitors for any of their exams.
It is true that after the break, for security reasons, one is not allowed to go back to work on uncompleted problems. This was true with the “paper and pencil” exams as well and it does not reflect a change in policy. Before or after the break, one can “flag” questions to go back to later.
The depth portions of the structural exam are indeed difficult. With the “Alternate Item Type” questions, which may require fill-in-the-blank, multiple correct responses, drag and drop, etc., there is no partial credit. The answers are either right or wrong. The previous method of grading did not report the relative difficulty of the breadth and depth sections of the exam and it is hard to know if the depth portions of the new exam are truly more difficult.
All would-be examinees should take advantage of reviewing NCEES videos that explain how the new Structural Exam works by watching “P.E. Structural Computer-Based Exams” by NCEES Media on YouTube.com. NCEES has the reference text materials available prior to taking the exam, but the code materials are not available in the same digital format as will be provided at the exam due to copyright protections. The exam writers and standard setters for all the NCEES exams including the PE Structural Exam are volunteers who, after they apply to help on the exam, are vetted and invited to participate. NCEES pays for travel, lodging, and incidentals for the volunteers, who can also receive continuing education credit for the time spent working on the exam. Participants have found the experience of working on the exam to be extremely rewarding, both personally and professionally, and many of us have developed close friendships with colleagues across the country that have served as resources and helped us in our practice. To improve the exam, make it relevant and more in keeping with typical office practice, NCEES needs licensed practicing structural engineers (including newly licensed engineers) to participate in the process. Learn more and get involved at ncees.org/volunteer/. ■
The comments and statements in this article are the author’s alone and do not necessarily reflect the positions or policies of NCEES.
Carl Josephson, PE, SE, is a Senior Principal at JosephsonWerdowatz & Associates, Inc. in San Diego, and Chair of the Structural Engineering Licensure Coalitionwww.selicensure.org.
Janiele Maffei is the California Earthquake Authority's Chief Mitigation Officer and Director of Research. Among several responsibilities at CEA, she manages an incentive program that has helped more than 23,000 California residents com plete code-compliant seismic retrofits. She earned an AB in architecture and an MS in structural engineering at the University of California Berkeley. She is also a past president of the Structural Engineers Association of Northern California.
STRUCTURE: What or who encouraged you to seek a career in structural engineering?
Maffei: My grandfather was a carpenter, and he had a magical workshop in his basement with a box of scrap wood specifically saved for his granddaughters’ special projects. Each of us had our own little tool box and we loved spending time with him building objects with maximum nails and minimal usefulness. My grandfather built a playhouse in our back yard and filled it with creative homemade toys. My Barbie dream house and dream car were made of wood. I loved designing and building things and was the only girl I knew who had a Mattel power shop. I was the only girl in my 8th Grade mechanical drafting class and one of three girls in my high school drafting class. I majored in architecture at the University of California (Cal) but took all the math, science, and undergraduate electives necessary to be accepted into the Structural Engineering Structural Mechanics (SESM) graduate program. While the ratio of men to women was close in architecture, I was one of only six women in the graduate program. While being a female certainly made me stand out I shared the same desire to build great things.
STRUCTURE: If you had to give advice to young people considering a career in structural engineering, what would that be?
Maffei: The typical advice to someone interested in engineering is to take all the math and science you can. However, the people who gravitate towards engineering already tend to already be interested in math. I would encourage them to make sure that they focus on being a well-rounded person. Study music, art, and literature, and be an effective communicator too.
STRUCTURE: Any pitfalls to be avoided?
Maffei: Structural engineers play a hugely important role in society, not only in their contributions to the built environment, but also in providing solutions to the challenges and problems of our world. I read an article once about how many of the significant improvements in health and safety in our communities were advancements in civil engineering: improved sanitation; access to clean drinking water; protection from inclement weather; and building fire protection to name a few. It may feel comfortable to be behind the desk on a computer, but real problems are solved through creativity, collaboration, and communication.
STRUCTURE: Your working career started out in a traditional engineering office. What did you learn there or who did you meet then that proved useful later?
Maffei: To answer your question about who I met as a young(er) engineer that proved useful today, I have a one-word answer: everyone. My current job is to take the best and brightest structural engineering ideas and put them to use to create earthquake resilience in residential
buildings. I really enjoy the notion that everyone in structural engineering, and in the broader earthquake engineering community, has a little piece of the puzzle and I am the puzzle master. The modern term for this is networking but it feels more like making friends to me.
STRUCTURE: Of all of the projects you have worked on, would you describe the one or two that you are most proud of and why?
Maffei: The work I am now doing in research and mitigation for the California Earthquake Authority (CEA) will most definitely be at the top of the list but there are a few projects from my consulting days that I am very proud of too. As a Bay Area native, after I graduated from Cal I remained in the area working in the East Bay and in San Francisco. I still live about 5 miles from the Berkeley campus and I drive by the historic Claremont hotel and numerous campus buildings that I worked on. Being a part of preserving those gorgeous buildings still brings me joy. I remember walking through Le Conte Hall and being told by the architect I was working with on the seismic retrofit of that structure that “Oppenheimer touched these doorknobs.”
STRUCTURE: What lessons did you learn that were valuable for later?
Maffei: The grass is not greener on the other side of the fence. It might taste a bit different, but it is still pretty much grass. When I first started Janiele Maffei SE after my son was born, I had the pleasure of working with a number of structural engineering firms around the Bay Area. I found similar issues at all of them with middle managers, less so with the older guys. I found giant egos and gentle souls. I also discovered the depth and breadth of talent in our industry was enormous.
STRUCTURE: After several years in the industry, you shifted focus and went to the California Earthquake Authority. Why?
Maffei: I was in a semi-retirement state, traveling a lot with my husband. We visited 12 countries in 12 months one year and I was pretty much able to work anywhere with a laptop. Then the job description for the Chief Mitigation Officer with the CEA flashed across my screen. I realized that I wasn’t finished yet, I had more to give. The notion of working on residential earthquake resilience directly through mitigation could be described as being my sweet spot. I also had that collection of friends, otherwise known as my network, who teemed with solutions ready to be put into action.
sexual harassment, and there was barely any maternity leave let alone parental leave. I can honestly say that it was very difficult to compete with my male colleagues who had a wife at home. I took a leap and started my own company and that forced me to take a lot of responsibility and also allowed me to work with so many different engineering firms. From lemons—lemonade.
STRUCTURE: You have demonstrated a long and dedicated commitment to professional activities. What is the most rewarding aspect of your service?
STRUCTURE: Most engineers don’t want to speak, let alone be the voice of an industry. What can we learn from your experience?
Maffei: The common notion is that public speaking is number one on the list of human fears. Seinfeld had a great bit about people preferring to be the guy in the casket rather than the one giving the eulogy. A quick internet search suggests that the fear of heights is actually number one, and contractors I have worked with can attest that I definitely have acrophobia. However, I somehow found my voice somewhere around the time I stepped into that middle school drafting class as the only girl. I stood up at a school assembly and said something into the mic and realized that the world didn’t come to an end. It didn’t rise to the level of “Ich bin ein Berliner” but I think it encouraged me to speak up. In my current job I have on average one public speaking event a week, sometimes more. However, I don’t consider myself to be a fully formed orator. I am continually watching and listening to other speakers, at conferences, on TV, and in movies, noting how they succeed, and fail.
STRUCTURE: There is a lot of talk these days about community resilience. But more than talk about that, you actually did something. Would you tell us a little more about your motivations and goals?
Maffei: My success started with the important decision in 1996 to include continual funding for mitigation in the CEA’s operating budget. While the contributions are small ($5 million maximum per year) we are able to leverage that funding with State and FEMA funding to provide $3,000 grants for what is, on average, a $5,200 retrofit. We also just received news that we have Building Resilient Infrastructure and Communities (BRIC) funding for multi-family soft-story retrofit grants. The decision to ask the California legislature to create the Chief Mitigation Officer position was also an important step towards success. My motivation was to step up and accept the challenge to put that funding to good use.
STRUCTURE: Can you say a little more about what it was like entering an industry where women are few and far between?
Maffei: With a nod to Ruth Gordon, the first woman to become a structural engineer in California, there were definitely challenges to being one of very few female engineers in the profession in the 1970s and 1980s. While there were supposedly around 20 women who got their California SE before me, I knew of only a handful of women who were older than me who were still working in the profession. It was a time when construction calendars and marketing materials featured scantily clad women on their covers. The Anita Hill testimony hadn’t yet socialized the notion of
Maffei: We asked the very first homeowner who retrofitted with one of our grants how he felt about the program and about the retrofit. He said, without prompting, that he slept better at night. I honestly feel that my team is “fighting the good fight.” Our residences are typically the single largest investment we will make, they are where our family sleeps at night, they are our home. Knowing that we are assisting homeowners increase the resilience of their homes is so worth it.
STRUCTURE: What is your approach to mentorship?
Maffei: It still surprises me when I am the oldest person in the room. I laugh at myself because I have become that older person who always has a related story to tell, on any subject. Being around longer does afford one the opportunity to share lessons and wisdom. But it is also an opportunity to assist a mentee find the value in themselves, regardless of what stage they are in their career. The most important part of being a good speaker is being a good listener because everyone learns more from a dialogue than from a monologue.
STRUCTURE: Many people think the structural industry broadly, including risk mitigation and insurance, has changed during the last few decades. Do you agree? Is it for the good or bad?
Maffei: Mitigation and insurance has definitely changed. Mitigation has become enormously important because we have so many buildings that are becoming un-insurable. The confluence of older buildings designed and built before building codes with seismic provisions, the upward financial pressures of inflation and the cost of global capital make catastrophe insurance unaffordable to most people. Many cities in California are moving in the right direction by retrofitting their vulnerable structures. However, life-safety retrofits don’t necessarily improve the insurability of the building. It is safe to say that there is more work to be done.
STRUCTURE: We all have mentors and people who helped us be successful. Who would you like to thank and why?
Maffei: I would like to thank my husband who taught me what torque was when I was an architectural student and he was in civil engineering at Cal. I think my forming a study group with two other female graduate students was absolutely the right thing for me to do for our relationship at the time. However, he has been a sounding board and incredible engineering confidant and mentor. He is, and I believe I am qualified to say this, one of the best structural engineers I know.
STRUCTURE: In terms of your personal legacy, what would you like to be remembered for?
Maffei: Just to prove I can make up a story about anything: a few friends and I pondered the question of whether we would like to be known for our intellect, our beauty, or our kindness. I don’t think anyone is truly intelligent or beautiful if they are not kind. I will go with kindness. ■
This article explores the subject of radial and spiderweb cracking in reinforced concrete two-way flat plates and flat slabs (herein referred to as “two-way slabs”) and the underlying reasons for it. Proper investigation of radial and spiderweb cracking will be addressed in a following issue of STRUCTURE.
By Terrence Paret, Hayley Proctor, PE, and Gwenyth Searer, PE, SE, and Prateek Shah, PhD
Over the years, the writers have observed radial (sometimes called “starburst” or “sunburst”) and spiderweb cracking in dozens of two-way slab structures. These slabs were constructed in different regions of the country, were designed by different engineers in different decades using different editions of ACI-318, support different occupancy types, and were constructed by different contractors. Many of these slabs were found to exhibit radial and spiderweb cracking during construction, i.e., before the addition of finishes and before being put into service, but others were only reported to have radial and spiderweb cracking after years of continuous occupancy, sometimes only discovered after the removal of floor finishes during routine maintenance or remodeling. The relatively common occurrence of such cracking across a breadth of circumstances warrants a technically accurate understanding of its causes and structural significance. While radial and spiderweb cracking sometimes are interpreted to be symptoms of elevated punching shear stress, and therefore are mistakenly interpreted to signify high risk conditions, analysis and research both demonstrate that these crack patterns are actually characteristic of flexural behavior that typically manifests at loads significantly below ultimate punching shear capacity, as documented by Elstner and Hognestad as well as Paret et al. This cracking largely results from a fundamental disparity between the explicit averaging of flexural demands across column strip widths in the ACI 318 strip method of design and the quantifiable distribution of bending moment in real slabs. Before delving into supporting analyses, it is helpful to
explore the conceptual behavior of two-way slabs.
When subjected to service-level gravity load, multi-span, two-way slabs with larger span-to-thickness ratios are generally expected to experience larger midspan deflections, and therefore larger curvatures near the columns, than slabs with smaller span-to-thickness ratios, all other things being equal. These differences can be idealized in three dimensions by thinking of the relatively flatter deformed shape in slabs with smaller span-to-thickness ratios as “doming” and the amplified curvatures in slabs with larger span-to-thickness ratios as “tentpoling” (Fig. 1). Both behaviors are flexural as they are associated with curvature. The larger curvatures associated with larger span-to-thickness ratio slabs necessarily increase the propensity for cracking and local yielding of negative flexural reinforcement close to the columns, both of which would further amplify deflection and tentpoling.
Figure 2 overlays a typical layout of column strip reinforcement onto a plan view of the deformed shape for a typical bay extracted from the tentpoling illustration in Figure 1. Because curvatures vary across the width of the column strip, the distribution of negative moment across the width of the column strips also must vary, with the highest negative moment located near the centerline of the column strip (close to the columns) and significantly diminished negative moment near
Fig. 1. (Above) Notional deformed shape comparison of slabs with varying span-to-thickness ratios. The area outlined in red is shown in plan view in Figure 2.
Fig. 2. (Right) Plan view of overlay of distribution of negative moment reinforcing steel (solid blue lines) and deformed shape (see Figure 1). The edges of the column strip are shown with black, dashed lines. The corners of the plan coincide with the centers of the surrounding bays.
the edges of the column strip. This distribution, whether for the larger or smaller span-to-thickness ratio slabs, is at odds with the ACI 318 strip method of design which assumes an averaged, uniform distribution of moment across the column strip width, thereby leading to designs in which negative moment reinforcement is uniformly distributed across the column strip width.
For typical span-to-thickness ratio slabs, even when the total quantity of column strip reinforcement is adequate to carry the total column strip moment, the concentration of negative bending stresses very close to the column results in a mismatch when compared to the more uniform distribution of steel in the column strip. This mismatch is indicative of what the writers refer to as a “nonuniform utilization” of the negative moment reinforcement: closer to the column, the stresses in the column strip reinforcement are necessarily higher than they are farther away from the column. Intuitively, the greater the degree of nonuniform utilization of the reinforcing steel, the greater the likelihood of developing relatively wide concrete cracking and yielding of reinforcing steel. However, this mismatch must be quantified to objectively assess the consequences of the mismatch.
with the letter of the code – to distinguish it from the “Compliant” model, which met the minimum slab thickness requirements, while the marginally compliant model did not. The flexural reinforcement in all three models was “designed” by ETABS to comply with the ACI 318 strip method.
Finite element software packages commonly used in structural design can readily quantify the severity of any nonuniform utilization, provide insight as to whether this nonuniformity is sufficient to lead to radial and spiderweb cracking and yielding of reinforcement, and predict the midspan deflection and crack pattern that results. Using ETABS, the writers developed three models of an idealized multi-span parking garage slab to isolate and study the influence of span-to-thickness ratio on performance (Table 1). The analyses, described below, demonstrate that characteristic tentpoling behavior due to nonuniform utilization of negative moment reinforcement, including radial and spiderweb cracking and yielding of reinforcement close to columns, predictably occurs even in code-compliant slabs under service loading.
The three models were identical in every respect (e.g., span length, column size, and loading) except for slab thickness, which was used to alter the span-to-thickness ratio. As tabulated, the slabs in all models were compliant in two-way shear (i.e., punching shear), though to different degrees since the slab thickness varied. Models characterized as “Robust” and “Compliant” had 12-inch-thick and 10-inch-thick slabs, respectively; each satisfied the minimum thickness requirements and the deflection limits set forth in ACI 318-19 Table 8.3.1.1 and Table 24.2.2. The third model, which had a 9-inch-thick slab, did not meet ACI 318’s minimum slab thickness requirements but was also ACI-318-compliant because it satisfied the calculated deflection limits. The 9-inch-thick slab model was characterized as “Marginally Compliant” – despite being compliant
For parking garages, which typically have few, if any, deflectionsensitive finishes, the controlling criterion for immediate live load midspan deflection per ACI 318 Table 24.2.2 is ℓ/360, or 1 inch in the case of a 30-foot span. Table 1 shows the predicted immediate live load deflections; all are less than 1 inch, indicating that the designs are code compliant, though notably, the predicted midspan deflection of the “Compliant” and Marginally Compliant” slabs are roughly five and eight times, respectively, the predicted deflection of the “Robust” slab. These values represent the deflection increment due to short-term live load on a slab that may have already experienced some cracking due to dead load.
The dominant slab cracking patterns predicted by ETABS for the three slabs are distinctly radial and concentrated close to the columns (Fig. 3), which is consistent with expectations for the flexural doming and tentpoling behavior illustrated in Figure 1. Although the meshsize dependency of these predictions makes them more reliable as qualitative points of comparison than as explicit predictions of in-field performance, the ETABS prediction that even the “Robust” slab will crack radially suggests that this behavior is inherent to two-way slabs regardless of level of safety and that engineers should not be surprised to see such cracking in buildings they design, even when the slabs are code-compliant in all ways, including punching shear.
The ETABS analyses also illustrate that the underlying mechanics of the radial cracking issue derive from nonuniform utilization of the uniformly distributed negative moment slab reinforcement. Figure 4 graphically depicts the nonuniform utilization from the ETABS analysis for the “Compliant”/10-inch-thick slab. Since the negative moment demand across the width of the column strip is not uniformly distributed while the negative moment reinforcement is, the local moment demand close to either side of the column significantly exceeds the reinforcement provided per the ACI 318 strip method. At the same time, near the outer margins of the column strip width, the reinforcement is more than adequate to resist the local moment
demand. Said another way, while the ACI 318 strip method provides adequate capacity to resist the total column strip moment, the demand significantly exceeds the slab capacity over the portion of the column strip width nearest the column, which leads to radial and spiderweb cracking and yielding within that portion of the column strip. In a true nonlinear analysis package that explicitly accounts for yielding and redistribution, the lateral extent of the overstress would spread farther from the column.
Radial and spiderweb cracking and yielding of reinforcement that results from the described nonuniform utilization may not negatively impact the ability of the slab to support design loads, but it can impact how the slab performance is perceived, and – if exposed to water and chlorides – the slab’s long-term performance. In addition to the possibility that some owners and occupants may consider the cracking to be objectionable and may express alarm due to the presence of radial cracking, tentpoling behavior can lead to a potentially significant increment of deflection that might not always be considered during design especially if it is not relevant to the ACI deflection criteria for that structure; that increment can also impact owner and occupant perception of performance even if it does not impact safety. For example, for the design of parking garage slabs for which immediate live load deflection is the only relevant codified calculated deflection criterion, dead load deflection and incremental dead load deflection due to tentpoling would normally be ignored, even though those together may be several times greater than the immediate live load deflection. In the writers’ experience, when tentpoling and out-oflevelness of two-way slabs become readily visible, the users’ experience and owner satisfaction regarding those slabs may be reported as being diminished (Fig. 5).
Three of the primary considerations often involved in structural design of two-way slabs are code-compliance, structural safety, and serviceability. In part due to the nonuniform utilization of negative reinforcement, all code-compliant two-way slab designs do not attain comparable degrees of safety and serviceability. Given that ACI 318 provides no guidance on this subject, it might be assumed that a design that exactly satisfies minimum code requirements would attain comparable degrees of safety and serviceability. This
assumption, however, does not withstand scrutiny. Not only will common design software predict tentpoling behavior, including cracking and amplified deflections, in code-compliant designs, but slabs that are code-compliant also commonly exhibit such behavior in the field. This phenomenon has sometimes resulted in design engineers, third-party engineers, developers, owners, and occupants characterizing normal predictable radial cracking and measured elevation differences in slabs that exceed code design limits as objectionable or even as safety risks. As such, an objective understanding of two-way slab behavior is urgently needed by the profession.
To assist in developing the needed understanding and perspective, Figure 6 sets forth conceptual “scales” of code compliance, structural safety, and serviceability notionally achieved by any given slab design. The scales depict ranges of possible design outcomes from “increasing non-compliance” (solid red) to “marginally compliant” (dashed red to dashed blue) to “increasing robustness” (solid blue) relative to code-compliance; from “decreasing safety” (solid red) to “increasing safety” (solid blue) with intermediate degrees of safety (dashed red to dashed blue); and from “unsatisfactory for most owners” (solid red) to “unsatisfactory for some owners” (dashed red to dashed blue) to “satisfactory to most owners” (solid blue) relative to serviceability. The scales are intended to be read in accordance with their vertical alignment, e.g., reading vertically along Line 1, the dashed red and blue “marginally compliant” portion of the code-compliance scale aligns with the solid blue of the structural safety scale because “marginally compliant” designs are likely to still be structurally safe, but may well perform unsatisfactorily for some owners; this may represent a design that meets only the minimum strength and deflection requirements of ACI 318. At the lower end of “marginal compliance” (i.e., Line 2), structural safety is still likely but is less assured while serviceability tends toward “unsatisfactory for most owners.” At the higher end of “marginal compliance” (Line 3), structural safety becomes more assured while serviceability tends toward “satisfactory for most owners.”
While the ACI 318 strip method of design has proven to be a reliable method for achieving safe two-way slab designs, the mechanics of two-way slab behavior result in nonuniform curvature—and therefore nonuniform utilization of the negative reinforcement— across the width of the column strip in slabs designed by the strip
method. These nonuniformities are underlying causes of commonly observed radial cracking and spiderweb cracking, which are characteristic flexural behaviors of two-way slabs and are often exhibited by code-compliant slabs. Commonly used design software can be used to demonstrate that radial and spiderweb cracking are predicted to occur in slabs that are proportioned and reinforced to just satisfy all code requirements, including for punching shear, as well as in slabs that are substantially more robust than required by code. Localized slab softening that develops in the vicinity of the supports as a result of nonuniform utilization of the negative moment reinforcement will result in greater curvature close to column supports (i.e., tentpoling), which is sometimes noticeable and necessarily amplifies midspan deflection beyond what would otherwise occur.
Engineers designing and assessing twoway slabs should anticipate this behavior, take steps to mitigate it by relying on more robust proportioning and reinforcement than is set forth by code minimum requirements, and be aware that radial and spiderweb cracking and tentpoling behavior are sometimes incorrectly construed as ramifications of punching shear or as otherwise detracting from the safety of the slabs that exhibit them. The performance of highly optimized designs will not be comparable to more robust designs with regard to these behaviors. More robust designs generally tend to experience less noticeable radial and spiderweb cracking and less noticeable tentpoling even if these behaviors will not be completely eliminated. Expectations of behaviors that may be unsatisfactory to some owners (i.e., cracking and out-of-levelness) should be clearly discussed with clients before completion of new designs to ensure that design decisions regarding slab span-tothickness ratios are not driven entirely by code minimum requirements and cost.
“Then and now” structural differences for the purlin lines of an existing metal building roof in Ohio highlight the need for engineering care during a retrofit.
By Tim Lane and Cris Moen, Ph.D, PE
Metal building roof system design has evolved since the 1980s. Design wind and snow loads for buildings with gable roofs are updated every few years. Also, consistent research investment by the Metal Building Manufacturer Association (MBMA) and the American Iron and Steel Institute (AISI) has led to improvements in the strength prediction of thin-walled cold-formed steel purlins with partial bracing from the roof panels. But what about the purlins?
Many existing metal building roofs show significant strength deficits when evaluated with modern building standards which makes it challenging when a roof is slated for a retrofit. And industry practice allows additional dead load without engineering and calculation that can lead to more roof performance uncertainty, e.g. the International Existing Building Code (IEBC) Section 502.4 “5 % rule” which states:
Any existing gravity load-carrying structural element for which an addition and its related alterations cause an increase in design dead, live or snow load, including snow drift effects, of more than 5 percent shall be replaced or altered as needed to carry the gravity loads required by the International Building Code for new structures.
A pre-engineered metal building built in 1989 in Delaware, Ohio, is shown in Figure 1. The overall building dimensions are 144 feet long by 120 feet wide, with varying frame spacings. The roof slope is 2 on 12. The building was designed according to the 1987 Ohio Basic Building Code which references the 1984 Building Officials and Code Administrators National Building Code.
The purlins are 12 inches deep with 2.5 inch wide flanges and 1-inch stiffening lips oriented 45 degrees from the horizontal flange. There are two purlin base metal thicknesses, 0.067 inch (interior spans) and 0.075 inch (end spans). The purlins are spaced at 5 feet on center and they are assumed to lap 1.5 feet on either side of a frame. (Fig. 2). The roof deck is assumed to be a 24 gauge R panel. The purlins are rolled from ASTM A570 steel with a nominal yield stress of 55 ksi. It is assumed that the building was designed for 25 psf live load plus 5 psf defined in the general notes, and this load is applied everywhere on the roof.
It is challenging to know exactly how purlin line strength was determined for the original building. Distortional buckling, i.e., partially restrained flexural-torsional buckling of the purlin compressed flange, had not been incorporated into the AISI standard in 1989, and combined action checks considering flexure, torsion, and shear were also not fully developed yet. Metal building manufacturers typically relied on simply-supported single span pressure box tests (base tests) and extrapolated these results to continuous spans which could miss strength limit states near the frame line purlin laps--for example the common failure mode of bottom flange purlin buckling near a lap (Fig. 3). Now, the AISI S100-16 North American Specification for the Design of Cold-Formed Steel Structural Members provides approaches for considering all the potential strength limit states including combined actions and even partial bracing from attached roof panels, see AISI S100-16 Section I6.1.
In this study the building purlin strength for gravity loads is calculated
with the open-source software PurlinLine.jl (https://www.runtosolve. com/purlinline) utilizing AISI S100-16 Allowable Stress Design (ASD) and structural analysis (https://github.com/runtosolve/ThinWalledBeam. jl) to predict the roof pressure where the first strength limit state develops. This software considers the continuous spans, varying purlin sizes (end spans versus interior spans), and the partial lateral bracing provided by the R panel roof deck.
For gravity loads on this building’s roof, the typical 6 span purlin line allowable pressure is 19.6 psf. (A detailed structural report is available [https://runtosolve.com/purlinline/structures_magazine]).
The failure develops at the end of the lap in the 30 feet end span, consistent with Figure 3. The strength limit state is predicted by AISI S100-16 to be shear + flexure interaction, which makes sense for this 12-inch-deep purlin.
The original demand design load for the roof is 30.0 psf, and the predicted ASD purlin line strength is 19.6 psf, resulting in a demand-to-allowable strength ratio of 1.5 which means the purlins are probably overstressed at ultimate loads (Table 1). This is an example where industry practice of applying the IEBC “5% rule” should be questioned.
What would happen if this building was designed with modern building codes? Consider the unbalanced snow load described in ASCE 7-22 Section 7.6.1 since it will create the most severe conditions for the purlins near the ridge. The ground snow load in Delaware, Ohio, for Risk Category II is 32.0 psf, see ASCE 7-22 Figure 7.2-1B. The unbalanced snow depth is calculated as 2.7 ft., resulting in an
ultimate-level unbalanced snow load on the leeward side of the roof of 42.0 psf extending 18 ft. down from the ridge. The ASD snow load factor is 0.7, resulting in a demand pressure of 29.4 psf and a demand-to-capacity ratio of 1.5. Both the original design and a design check following modern codes shows that the roof purlins are overloaded by about 10 psf.
When a metal building roof system like this one in Ohio is found to be overstressed, common engineered roof retrofit solutions considered are: adding additional purlin lines or reinforcing the existing purlins.
Roof retrofits where additional purlin lines are placed between the existing lines (Fig. 4) are effective and can bring a roof up to
either of which can be time-consuming and costly. An exterior purlin line reinforcement solution like that shown in Figure 5 can be implemented while the building is still in service. A roll-formed pre-punched hat subframe is fastened to the existing purlin lines, adding 10 psf or more capacity to the roof system. A new roof (typically a standing-seam roof) is then attached to the hat subframe top flanges.
The roof purlins in this Ohio metal building were originally designed at or beyond their calculated structural limits. This does not mean that all metal building roofs are about to fail in a snowstorm. The conclusions herein are intended to highlight that: (1) there can be concerning mismatches between existing metal building roof strength and code-specified demand loads; (2) use of the IBEC “5 % rule” should be supported by engineering calculation; and (3) roof retrofit solutions are available to mitigate existing metal building roof system failure risk . ■
Fig. 5. In this purlin line exterior retrofit solution, a roll-formed pre-punched hat subframe is fastened to the existing purlin can provide 10 psf or more additional purlin line roof strength.
With over 45 years of experience in the metal construction industry, Tim Lane is the owner and president of TopHat Framing Systems. TopHat offers patented subframe systems as solutions for metal roof retrofit projects.
Cristopher D. Moen, Ph.D., PE, F.SEI is an engineer, software developer, researcher, and educator. His company RunToSolve LLC creates fast open-source computational tools for predicting structural system performance.
Reducing blast demands is an important aim achieved through reducing deformations.
By Dr. Ibrahim M. Metwally, Ph.D, PE
The main strategy for blast-resistance structures design is to reduce blast demands, which inherently is achieved by reducing the deformations in structural and non-structural building components. This is accomplished through various techniques:
Providing sufficient protection by increasing protected standoff distances against external attacks. The most cost-effective solution for mitigating explosive effects to a building is to ensure the explosions occur as far away from the building as possible (increased standoff). Therefore, the site selection for new construction and site protection in existing structures is important in mitigation blast risk.
Many types of barriers are designed to resist the impact of a vehicle explosive. Among them are massive concrete barriers (Kontek 2008), concrete enclosed with steel plates (Crawford and Lan 2006), and soil filled corrugated metal (Crawford and Lan 2006). Each barrier is designed to absorb the large amounts of energy from an impact or blast with minimal effect on the facilities it is protecting.
effects of blast loading. Re-entrant corners and overhangs are likely to trap shockwaves and amplify blast effects. The reflected pressure on the surface of a circular building is less intense than on a flat building. When curved surfaces are used, convex shapes are preferred over con cave shapes. Figures 1-2 present desirable and undesirable structural
shapes and layouts of buildings when designing for blast loading.
Not many code resources are available when designing for blast loading. However, FEMA 427 does provide guidelines. The general design recommendations are not that different from what has been discussed here, in that blast resistant building designs should use simple geometries without sharp re-entrant corners and be placed on a project site as far from the lot perimeter as practical. The following discusses FEMA 427 recommendations for different types of framing systems:
In frame structures, column spacing should be limited. Large column spacing decreases the likelihood that the structure will be able to redistribute load in the event of column failure (Figure 3).
In frame structures, the exterior columns should be designed to resist the direct effects of the specified blast.
The frame structures system should be designed to resist the likely progressive collapse. In case of occurrence any localized failure must be considered.
The primary loading on the roof is the downward air-blast pressure. The preferred system is cast-in place reinforced concrete with beams in two directions. If this system is used, beams should have continuous top and bottom reinforcement with tension lap splices. Stirrups to develop the bending capacity of the beams closely spaced along the entire span are recommended. Finally, use two-way floor and roof systems.
Cast-in-place reinforced concrete is the structural system preferred for blast-resistant construction. This is the material and structural type used for military bunkers. The military has performed extensive research and testing of its performance. Concrete has significant mass, which improves response to explosions.
Generally, simple geometries and minimal ornamentation (which may become flying debris during an explosion) are recommended. If ornamentation is used, it is preferable to use lightweight materials such as timber or plastic, which are less likely than brick, stone, or metal to become lethal projectiles in the event of an explosion.
Ultra high performance concrete (UHPC) is known for its superior mechanical properties; compressive strength can reach up to 200 MPa (29,000 psi) and tensile strength up to 40 MPa (5800 psi). Also, the crack propagation can be well controlled due to inclusion of steel fibers in its cement matrix, leading to a higher ductility and energy absorbing capacity so as to make it an ideal material for structural members that are exposed to the constant threat of blast attacks. Previous experimental work conducted by Mao et al. and Wu et al., Barnett et al., Ibrahim Metwally, Schleyer et al., and Melançon confirmed the superior blast resistance of UHPC structures under high loading rate conditions such as explosion and impact compared to traditional normal and highstrength concrete.
Concrete-filled steel columns have high ductility and very good blast resistance [Peyman, et al., Ibrahim Metwally and Zhang, et al.). Concrete systems have significant inertia but are susceptible to
Fig. 2. Less than desirable building features for blast loading are multiple levels of stories, complex designs, projecting roofs or floors, and U-shaped buildings.
shear failures. Steel systems have inherent ductility but are locally vulnerable open sections and connections. A combination of steel
Blast-resistant design philosophy allows structural elements to undergo large inelastic (plastic) deformations under blast loading. A ductile structure that undergoes large deformations without failure can absorb much more energy than a brittle structure of the same strength. Tensile reinforcement between 0.5% and 2% of the crosssectional area of the concrete element will usually ensure ductile behavior while providing the required strength.
Compression steel in flexural members serves two purposes. After a structural member is deflected by blast loads, it attempts to spring back or rebound. Dynamic rebound causes load reversal and, under certain conditions, can result in catastrophic failure.
Minor: Non-structural failure of building elements such as windows, doors, and cladding.
Moderate: Structural damage is confined to a localized area and is usually repairable. Structural failure is limited to secondary structural elements, such as beams, slabs, and non-loading bearing walls. However, if the building has been designed for loss of primary members, localized loss of columns may occur without initiating progressive collapse.
Major: Loss of primary structural components such as columns or transfer girders leads to loss of additional elements that are adjacent to or above/below the lost member. In this case, the building is usually not repairable.
Full references are included in the online version of the article at STRUCTUREmag.org
Ibrahim M. Metwally, PhD, PE, is a professor of concrete structures at the Concrete Structures Research Institute at the Housing and Building National Research Center, Giza, Egypt. He is licensed by the Wyoming Board of Professional Engineers in the U.S. and registered as a senior structural consultant at DRSO of the Ministry of Housing of Egypt.
These frequently asked questions (FAQs) about tall mass timber are reprinted with permission of WoodWorks—Wood Products Council. The website address is: www.woodworks.org/learn/mass-timber-clt/tall-mass-timber/.
What is the current status of tall mass timber buildings in the building code?
Follow this link for the status of the 2021 IBC and jurisdictions that have adopted the tall wood code provisions: www.woodworks.org/resources/ status-of-building-code-allowances-for-tall-mass-timber-in-the-ibc/.
Tall Mass Timber Code Adoptions by State No Tall Mass Timber Code Provisions
What are the height limits for the new construction types?
2021 IBC TMT Provisions
2024 IBC TMT Provisions
2021 IBC TMT Provisions adopted on a per-city/county basis: City of Nashville, Tennessess, and Memphis & Shelby Counties, Tennessee
2024 IBC TMT Provisions adopted on a per-city basis in: City of Denver and City of Dallas
What are the timber exposure limits for Types IV-A, IV-B, IV-C and IV-HT construction?
Timber Exposure Allowances
exposed timber permitted Ceilings and integral beams up to an area equaling 20% (per 2021 IBC) or 100% (per 2024 IBC) of fire area or dwelling unit area or Walls and integral columns up to an area equaling 40% of fire area or dwelling unit area or A combination of each, with the sum of ratios (actual exposed divided by allowable exposed) not to exceed 1.0
FRT sheathing or 1/2″ gypsum board or noncombustible material
of Floor (above Mass Timber)
What are the fire-resistance rating requirements for tall mass timber?
Requirements for Tall Mass Timber Structures (hours) *Not required by construction type. Other code requirements may apply. 5/8″ Type X gypsum = 40 minutes
I’ve heard that the 2024 IBC allows 100% timber ceiling exposure in Type IV-B construction, up to 12 stories tall. Is that correct?
Yes, the 2024 IBC includes new code provisions which allow timber ceiling exposure in Type IV-B construction up to 100%. The new code language in the 2024 IBC is available at codes.iccsafe.org. Several jurisdictions such as the City of Denver, City of Dallas, State of Oregon and State of Washington have incorporated these new timber exposure limits in their building codes, and several design teams are looking to utilize the new limits in project-specific discussions with their local building officials.
For more information, contact WoodWorks: info@woodworks.org
Type K shrinkage-compensating concrete optimizes performance and value and minimizes carbon intensity impact.
By Susan Foster
Improving overall structural behavior and long-term performance of critical concrete infrastructure is paramount to supporting today’s carbon neutral initiatives and ensuring good stewardship of both public and private owner investments. More stringent performance requirements combined with the challenging dynamics of change in type, quality, and availability of concreting materials is inspiring innovation, creativity, and collaboration. It is also renewing interest in materials with established, proven performance that offer solutions to today’s challenges. One of those experiencing an inspiring renaissance is Type K cement.
Developed in the late 1950s and brought to market in the early 1960s, Type K cement has proven to be a valuable innovation in cement technology for over 60 years. It is specifically engineered to eliminate negative volume change and improve durability by lower ing permeability, increasing density, improving sulfate and abrasion resistance, and eliminating drying shrinkage cracking. It effectively addresses the fundamental shortcomings of Portland cements by improving the quality of the cement paste and inherently improving long-term performance and structural behavior of vital structures.
From its earliest use in prestressed pipes and pavements to its use in shrinkage-compensated designs for post-tensioned structures, containment structures, dams, spillways, mat slab foundations, bridge decks, pavements, and slabs-on-ground, Type K cement has proven to be a reliable solution for all types of critical structures. Its performance continues to aid designers in simplifying designs and influencing more efficient constructability while minimizing in-service costs and operational downtime for repairs.
Type K cement (American Society of Testing and Materials’ ASTM C845 – Standard Specification for Expansive Hydraulic Cement) is a hydraulic blended cement that combines an expansive calcium sulfoaluminate (CSA) cement-based additive with a Portland cement source (ASTM C150, C595, and C1157). It is proportioned to achieve sufficient expansion to overcome the shrinkage characteristics of concrete and grout mixes. The advanced hydration mechanism of the expansive CSA cement-based additive (marketed primarily as Komponent in the U.S. ) drives the performance of Type K cement-based mixes. During hydration, primary ettringite (the mineral name for calcium
sulfoaluminate) is formed that contributes to design strength, controlled set, and early expansion. The qualified dosage of the expansive cement additive needed to create adequate expansion is determined by ASTM standards designed for use with expansive cements (i.e., ASTM C806 - Standard Test Method for Restrained Expansion of Expansive Cement Mortar and ASTM C878 - Standard Test Method for Restrained Expansion of Shrinkage-Compensating Concrete). The goal is to create sufficient designed expansion to compensate for the shrinkage characteristics of the mix and ensure the concrete is kept in compression for the life of the placement (Figure 1).
By efficiently consuming the excess mix water that is not used by Portland cement during hydration and minimizing bleed water, Type K shrinkage-compensating cement mixes result in dense, low permeability concrete with substantially improved abrasion resistance without the use of admixtures or surface hardeners. The consumption of excess mix water prevents voids and capillaries that allow room for drying shrinkage and results in a more dimensionally stable concrete placement. By eliminating curling and shrinkage stresses, the load capacity of the placement is increased, allowing thinner sections to be placed and reducing the overall volume of concrete required. In addition, its 0% tricalcium aluminate (C3A) content means sulfate resistance is improved proportionately to the percentage replacement used with all Portland cements, making it ideal for containment, marine, and other environmentally exposed structures.
When designing with shrinkage-compensating concrete, industry standards, guidelines, specifications, and other published resources are available to aid in design and constructability. The “Key Resources” sidebar provides a brief overview of commonly referenced standards, methods, codes and resources, from the American Concrete Institute (ACI) and ASTM, when designing with shrinkage-compensating concrete.
As engineers, owners, and project budgets are now more heavily influenced by supply chain dynamics, project and maintenance budgets, global warming potential (GWP) impacts, and commitments to sustainability, shrinkage-compensating concrete maximizes versatility. When designing with Type K shrinkagecompensating concrete, reinforcement options are no different than with other cement types required for concrete design—from traditional steel rebar and steel fibers to FRP, synthetic fibers and hybrids. Whichever is most suitable, available, and cost effective for the project can be used.
Type K cement was originally brought to market regionally as a finished cement. By the 1980s, effective ASTM standards and ACI guidelines had been developed to support consistent quality as a locally blended cement and made the use of the expansive cement additive economical and readily available nationwide. Type K cement is now prequalified per ASTM C878 and added at the local batch plant using normal bulk batching and mixing operations. This is a more practical and cost-effective way to deliver high-performance Type K shrinkage-compensating concrete (K-SCC) and grout (K-SCG), and it paved the way for use in a wide range of applications. Key
critical structures like concrete containment, post-tensioned designs, bridges, and mass elements have been constructed optimizing design, durability, constructability, and sustainability.
For critical concrete containment structures, preventing contamination from external impurities and preventing seepage and leakage of contents into the environment is crucial. Since the early 1970s, K-SCC has been used successfully to create well designed, reliable concrete containment structures with extended joint wall panels, foundations and slabs that help prevent leakage, unscheduled downtime, and costly repairs.
The Bustamante Wastewater Treatment Plant in El Paso, TX, and the J.W. Rogers Water Treatment Plant demonstrate the design possibilities. With tanks including 110 feet (33.5 meters) diameter by 30 feet (9.1 meters) high digesters, 120 feet (36.6 meters) diameter by 20 feet (6 meters) high primary clarifiers, and 140 feet (42.7 meters) diameter by 16 feet (4.9 meters) high secondary clarifiers, all were constructed without joints by using K-SCC. More than ten years later they are still crack free and leak free.
The $255 million 69th Street Complex Wastewater and Sludge Treatment Plant in Houston, TX, used K-SCC for the construction of foundation slabs for the reactors, clarifiers, pump stations, thickener and digester complex, roof slabs, and reactor train beams. Key drivers for use on this project were eliminating drying shrinkage cracking and preventing leakage in critical areas, reducing shrinkage reinforcement, and placing large sections that minimized construction joints and waterstops. Other advantages included low permeability, higher abrasion and sulfate resistance, and its inherent cohesiveness that allowed for ease of placement of smooth, dense surfaces.
The reduction in steel reinforcement and waterstops combined with the reduction in formwork, mobilizations, man hours and pump days resulted in an overall savings to the project. As reported by the joint venture design team of Environmental Management Consultants (EMC) and the City of Houston, “The use of Type K concrete on the 69th Street Complex was certainly a valuable learning experience for both the consultants and the contractors and has demonstrated that the economies of shrinkage-compensating concrete construction and the resulting long-term, crack-free performance of those structures is worth the extra effort and care.”
The City of New York’s project for eight underground, four-milliongallon combined sewer overflow (CSO) tanks is another example of K-SCC delivering innovation, constructability and value. Hazen and Sawyer were selected to design these critical structures with a focus on minimizing the number of construction joints. With conventional concrete, ACI 350 – Environmental Engineering Concrete Structures recommends a maximum construction joint spacing of 30 feet to control shrinkage cracking. When using shrinkage-compensating concrete, ACI 223 – Shrinkage Compensating Concrete recommends spacing up to 150 feet (45.7m).
By using K-SCC a reduction in construction joints minimized points of water leakage and reduced total construction time. In a comparative analysis between the use of conventional concrete and shrinkage-compensating concrete, the net savings for the project was over two months of construction time saved on the slabs, four months on the walls, and approximately $900,000 in materials and labor. Additional value was realized in-service by minimizing maintenance costs and operational downtime.
In post-tensioned structures, the dimensional stability of K-SCC offers significant advantages. Post-tensioning provides control over flexural cracking while K-SCC eliminates other key challenges related to negative volume change, like drying shrinkage cracking and restraintto-shortening (RTS). It also significantly reduces the effects of long-term creep and shrinkage helping to overcome the effects of differential displacement of supports and minimizing long-term relaxation of steel tendons. This improved dimensional stability eliminates labor intensive and time-consuming details like pour strips, slip joints, wrapped dowels and additional reinforcement for crack control.
Economic studies have shown that in properly designed structures, the savings in design element costs, traditional reinforcement and posttensioned steel more than offset the moderate material cost impact of the cement additive. Of the three key influencers of prestress losses (i.e., prestressing steel relaxation, creep shortening, and shrinkage), shrinkage constitutes approximately 42% of the long-term losses and reduces the initial prestress force by ~7%. When shrinkage is eliminated, this reduction translates into a reduction in the quantity of prestressing steel required. Additionally, a K-SCC structure has less dimensional change resulting in substantial reduction in column moments and affects a reduction in total column steel. Expansion joints can realistically be increased to approximately 500 feet (152.4 meters) without sacrificing performance.
The University of Alabama’s Ridgecrest Community Residence, an eight-story dormitory and parking structure, illustrates the advantages of using K-SCC in post-tensioned structures. Structural Design Group (SDG) of Birmingham, Alabama, used K-SCC to optimize design and improve structural behavior, meet budget requirements, and reduce time to completion. During onsite inspection two years
after placement, critical areas where RTS cracking could be expected revealed the K-SCC placements to be crack-free. The structural designer stated: “The real proof is in the slab itself—there are virtually no cracks in more than 420,000 square feet (39,019 square meters ) of slab. Further, the concrete frame was bid and completed 42 days ahead of a very aggressive schedule.” Net savings by elimination of pour strips were estimated at $250,000 with a total realized savings of the redesign using K-SCC of ~$3 million.
The Dallas Municipal Center is another iconic structure that optimized design and performance of K-SCC. It exemplifies what can be achieved when innovation is combined with collaboration and communication. This structure is a monolith of 60,000 cubic yards (45,873 cubic meters) of buff-colored K-SCC integrated into a unique post-tensioned structure consisting of underground parking and office spaces. The use of K-SCC allowed structural engineers to address cracking concerns related to temperature and shrinkage and reduced the amount of reinforcement needed to address RTS. It also allowed the contractor to cast large individual sections of exposed concrete (up to 70 feet long by 14 feet high (21 meters long by 4 meters high)). This improved project efficiencies and time to completion. Final reports noted that the shrinkage-compensating concrete performed “very satisfactorily,” the contractor “did a commendable job in obtaining the designed architectural finish.”
The use of K-SCC in bridge decks has also been well documented through the years. The Ohio Turnpike Authority has been using it on their bridge decks since the 1980s with great success (Figure 2). In a comparative study conducted from 1983 to 1990, results showed substantial improvement in bridge deck performance, resulting in
ACI 223 – Shrinkage Compensating Concrete is the primary resource for the design and construction of shrinkage-compensating concrete structures. It offers the most comprehensive insight into design considerations like durability, permeability, sulfate resistance, elimination of curling, warping, and drying shrinkage cracking, increased load capacity, prevention of calculated shortening in conventionally reinforced and post-tensioned structures, along with recommended constructability practices.
ACI 301 – Specifications for Structural Concrete includes a section reviewing the use of shrinkage-compensating concrete that addresses requirements and qualifications designers and specifiers should consider.
ACI 302 – Guide to Concrete Floor and Slab Construction, Chapter 5 reviews specifics related to design and construction of joints when using shrinkage-compensating concrete.
ACI 360 – Design of Slabs on Ground, Chapter 9 reviews design of shrinkage-compensating concrete slabs with design examples in Appendix 5 and Chapter 14 highlighting the elimination of saw cut joints when using shrinkage-compensating concrete.
ACI 350 – Environmental Engineering Concrete Structures references performance characteristics like minimizing drying shrinkage and improving both sulfate and abrasion resistance using Type K shrinkage-compensating concrete. It discusses the elimination of bleed water and ultimate lower permeability and reviews key advantages and related engineering regarding thinner walls, elimination of contraction joints, and reduction in reinforcement (maximum 0.3 percent shrinkage steel is common with joint spacing up to 150 ft (45.7m).
ACI Special Publications and the Post-Tensioning Institute’s PTI Journal have published numerous research reports on the use of shrinkage-compensating concrete for innovation and advancement in post-tensioned buildings, bridges, slabs-on-ground, soil anchoring and other infrastructure.
ASTM C150 – Standard Specification for Portland Cement
ASTM C595 – Standard Specification for Blended Hydraulic Cements
ASTM C806 – Standard Test Method for Restrained Expansion of Expansive Cement Mortar
ASTM C845 – Standard Specification for Expansive Hydraulic Cement
ASTM C878 – Standard Test Method for Restrained Expansion of Shrinkage-Compensating Concrete
ASTM C1157 – Standard Performance Specification for Hydraulic Cements
zero instances of drying shrinkage cracking. When asked about maintenance cost impacts, Chief Engineer, Doug Hedrick noted “The answer is quite simple, it is very low cost to maintain the shrinkagecompensating concrete decks – no deck delamination's, spalls, or steel corrosion. We don’t even think about cracks, crack maintenance or spalls on our shrinkage-compensating concrete decks.”
In cooperation with various states throughout the U.S., other studies
of the use of K-SCC in bridge designs have been published demonstrating the performance advantages that can be achieved. The durability attributes that contribute to their extended service life include abrasion resistance, significantly reduced shrinkage cracking, reduced surface capillaries and porosity; superior compressive and splitting tensile strengths ease of placement, consolidation, and finishing, ultra-low permeability, and excellent freeze/thaw results with air entrainment. Grouting is another common application of K-SCG. The Bob Kerrey Pedestrian Bridge in Omaha, Nebraska, is 3,000 linear feet. K-SCG was used for this cable-stayed superstructure to protect the posttensioned (PT) tendons which are essential to the bridge’s long-term durability. Its low permeability, increased density and dimensional stability ensured the PT tendons were effectively protected from moisture, salts, air, and other elements that promote corrosion and deterioration. Adding the Type K expansive cement additive to a bulk shrinkage-compensating grout mix improved production efficiencies at the plant and during pumping operations on-site. It also achieved cost savings over the pre-blended bag material typically used. Success stories continue as many transportation departments across the country embrace a proven solution to building safer, more sustainable infrastructure.
In reinforced pavement designs, eliminating joints in runways
and taxiways is essential. Joints are the location of curling, spalling, and cracking that result in foreign object debris (FOD), creating safety hazards and costly maintenance and repair projects. To identify the most durable pavement solution that would reduce the number of joints required on airport runways and taxiways and significantly reduce the costs associated with maintenance and repair, K-SCC was chosen for use on one of the most innovative concrete slabs ever constructed. In 1993, this post-tensioned, steel fiber reinforced pavement was placed at Rockford International Airport’s Runway Extension project in Rockford, Illinois.
In a side-by-side comparison of two K-SCC designs using steel fiber reinforcement the contractor was able to place two contiguous “Innovative Pavement Slabs” (IP1 and IP2, respectively) of taxiways paralleling a new runway extension. IP1 and IP2 were placed in 75-foot (23 meter) wide pavement sections. The additional flexural strength provided by using steel fibers allowed a reduction in pavement thickness from 15 inches (38.1 centimeters) to 10 inches (25.4 centimeters). Transverse joints were cut in the steel fiber reinforced IP1slab at varying span lengths from 85 feet to 200 feet (26 meters to 61 meters) to test how far apart natural cracking of the material would be with increased joint spacing. IP2 used steel fiber reinforced K-SCC for a 1,200-foot (365.8 meter) long placement with longitudinal post-tensioning and no control joints. The increased flexural strength provided by post-tensioning allowed further reduction in slab thickness to only 7 inches (0.18 meters). The pavement was inspected quarterly for five years. After 10 years of heavy use, the slabs were performing exceptionally well with minimal cracking and virtually no spalling. The FAA’s Pavement Condition Index (PCI) reported the steel fiber reinforced K-SCC (IP1) in Very Good condition (PCI 82), and the post-tensioned K-SCC slab (IP2) in Excellent condition (PCI 98).
Dams and spillways are other critical structures where K-SCC and K-SCG are used. The U.S. Army Corps of Engineers approved the use of K-SCC for their first full-size prototype at the Ririe Dam located about 25 miles (40 kilometers) from Idaho Falls, ID. The goal was to place larger, uniform surface slabs of concrete and minimize cracking. Using K-SCC, the spillway chute invert slab allowed the contractor to increase panel sizes to either 91 feet x 75 feet (27.7 meters x 22.9 meters) or 45.5 feet x 75 feet (13.9 meters x 22.9 meters) in area (vs. the conventional panel size of 22 feet x 25 feet (6.7 meters x 7.6 meters)). This allowed a reduction in total number of invert panels from 104 to either 18 or 9, depending upon the option selected. Minimum thickness was 12 inches (0.3 meters). Total spillway invert slab contained 5,584 cubic yards (4,269 cubic meters) of concrete. The reduction in linear feet of joints, percentage of steel reinforcement, linear f ootage of foundation anchors and foundation drain holes provided economic incentive for this project. Evaluations following the placement noted limited cracking at abrupt foundation irregularities and overbreak in the rock excavation, none deemed detrimental to the slabs, and many slabs that did not exhibit any signs of cracking.
In 2014, the U.S. Army Corps of Engineers, U.S. Department of the Interior, and US Bureau of Reclamation Cooperative used K-SCG for the $900M Folsom Dam Auxiliary Spillway Control Structure project (Folsom, CA). This bulk shrinkage-compensating
grout project was batched on this remote site to achieve 7,000 pounds per square inch (psi) strength. Six submerged steel gate supports were successfully secured and protected using K-SCG with the two-year inspection indicating the grout was meeting dimensional stability design expectations.
With a GWP of 461, K-SCC provides a GWP 56% lower than Portland cements and 37% lower than Type 1L Portland-limestone cements (Fig. 3). The use of K-SCC not only lowers the overall carbon impact of any mix design, it also significantly reduces overall carbon impact throughout the concrete’s service life. By reducing materials and labor requirements during construction, improving durability, and minimizing repair and maintenance, owners, designers and patrons benefit from an asset life two to three times that of a similar Portland cement concrete structure.
With ever increasing demands to build more durable critical infrastructure efficiently, and with more sustainably and added value, Type K shrinkage-compensating cement will continue to deliver. Embracing innovative approaches to design, integration of new products, and the collaborative efforts of professionals throughout the industry in its use will make a difference in the race to carbon neutrality while improving performance.
Full references are included in the online version of the article at STRUCTUREmag.org
Susan Foster is Director – Strategic Initiatives & Komponent for CTS Cement.
The new ACI Center of Excellence is focusing on ways to advance construction productivity.
By Phil Diekemper, Cary Kopczynski, and Michael L. Tholen
In 2017, the McKinsey Global Institute (MGI) published a sobering study showing that construction industry productivity gains have lagged significantly behind those of other industries for many years. In fact, MGI concluded that very little progress has been made in construction productivity for several decades.
In a 2023 report, the National Bureau of Economic Research (NBER) found much the same thing. Their work was published in the paper, “The Strange and Awful Path of Productivity in the U.S. Construction Sector.” The report showed that inflation-adjusted productivity, defined as “value creation per worker,” has made no net progress since the 1950s. It further concluded that the construction sector’s deteriorating efficiency in transforming intermediate construction goods—essentially the raw materials of construction—into finished products is largely responsible.
In the wake of these and other critical reports on construction productivity, many individuals and organizations have scoured the data to try to better understand it. And the news is not uniformly bad. Innovative processes and technology have improved many segments of the industry and will continue to do so. Building information modeling (BIM), for example, is revolutionizing design. Modular assembly is doing the same for construction. However, the aggregated data for the industry at large shows that productivity has been in a neutral to negative trend for many years.
MGI and others disagree on the cause of the problem. Many theories have been proposed, including aversion to innovation due to disproportionate risk sharing, contractual roadblocks that discourage teamwork, the fractured nature of the industry that “silos” its players, and deterioration of
construction document quality. One thing all agreed on, however, was that structural engineers and other designers have enormous power in setting the stage for productivity improvement. By improving the constructability of design, they can change construction.
In response to the productivity challenge, the American Concrete Institute (ACI) formed PRO: An ACI Center of Excellence for Advancing Productivity, in 2023. PRO is ACI’s third Center of Excellence and focuses on leveraging ACI’s global leadership in concrete materials, design, and construction to help advance industry productivity. Like the other two Centers, PRO is a separate 501(c)(3) entity with its own vision and mission, Board of Directors, and bylaws. Being under the ACI umbrella, however, provides the benefit of the Institute’s vast resources. Instrumental in PRO’s efforts are structural engineers, as they can help pave the way for success.
PRO began work by uniting leaders from across the architecture, engineering, and construction (AEC) industry to identify productivity barriers and find solutions. Shortly after its formation, PRO invited a diverse group of structural engineers, contractors, materials suppliers, and others to meet at ACI World Headquarters in Farmington Hills, Michigan. The objective was to identify strategies for attacking the productivity challenge.
Six strategic initiatives resulted from this meeting:
1) Improve the constructability of concrete structures
2) Align contractual agreements of design, construction, and ownership teams
3) Incentivize innovation in design and construction
4) Improve collaboration among all project stakeholders
5) Improve construction document completeness and coordination
6) Expand industry use of new technologies.
PRO defines constructability as “the effective integration of construction knowledge into the planning and design of a project to optimize its construction cost and schedule and maximize its value to the owner.” The following section begins to explore the first initiative in PRO’s Strategic Plan, with future articles diving deeper into the Center’s other five initiatives.
To create maximum benefit, this integration of construction knowledge must begin early in design, which contrasts with the traditional design-bid-build method of project delivery which can provide low initial costs, but rarely the lowest final cost. Nevertheless, owners and others often use bidding as a cost management tool.
When comparing design-bid-build versus design-build methods, it is important to note the various degrees of design assist available. Examples in section one of PRO’s Constructability Blueprint, “Value of Design Collaboration,” include strategic purchasing and proactive problem solving in the design-assist model as a means for increasing value over the project duration. In contrast, adversarial change orders in the design-bid-build model result in decreasing value over the project duration. The design-assist method is a collaborative effort to enhance common project goals, while design-bid-build silos each group in to focusing on their own self-interest. Although designbuild arrangements aren’t always possible, improving trusted design collaboration is key.
Improvements in constructability require changes in this mindset. Maximizing collaboration between designers and contractors is the key to positive change. It improves constructability and, hence, productivity.
Constructable designs ideally include input from owners, materials suppliers, subcontractors, contractors, and others. Solutions are identified early in the design process, which removes roadblocks, reduces later Requests for Information (RFI), and maximizes project value.
At the early stages of design, the design team should include experienced staff who can provide valuable input and insights, even if this
staff doesn’t work on the project to its conclusion. Envision a reverse engineering approach where the contractor and designer establish a construction concept before the design begins. The design can then evolve to achieve the construction plan which elevates the productivity of both design and construction. Ideally, design engineers would have spent significant time in the field seeing issues they create which hampered productivity or caused delays. This is where staff mentoring plays an important role. However, due to increasing distances between project locations and designers’ offices, reduced budgets for field services, and other factors, this first-hand experience seems to be less and less likely to occur.
Engineers who wish to include constructability reviews and ideas early in their design process often struggle to obtain access to trusted individuals that add value and needed knowledge. In most situations, the concrete constructor of the project has yet to be decided. Highly valued contractors may be reluctant to spend time and expense providing suggestions or a construction plan for a project they may never be awarded, or they may skew their bids in either direction by providing a level of knowledge about the project that is not consistent among all bidders.
The Harmon Tower in Las Vegas, Nevada, represents an extreme example of a capable structural design lacking constructability and trusted collaboration amongst the project stakeholders. The tower was designed to be a 49-story building; however, the project was stopped, and litigation revealed construction defects and other issues. The
The Harmon Tower in Las Vegas was never completed, and litigation revealed construction defects and other issues, like this concrete core shear wall link beam detail that was not constructable.
unfinished tower only reached 28 floors and was ultimately demolished in 2015. Collaboration and teamwork were relatively nonexistent between project teams. The design was completed and issued to the contractor as both were entrenched in defensive silos, and lack of trust and blame was prevalent. The structural design documents reflected a design that ultimately could not be constructed, such as link beam reinforcement for shear walls that could not be installed. Instead of contactor-designer collaboration to address constructability issues, and with a determination to meet a demanding schedule, construction proceeded with the installation of reinforcement that did not meet the design requirements. The later investigation of 2,800 different areas of the building found that 1,800 of the locations were grossly deficient in reinforcement. Combining legal, consulting, and demolition fees, the total damages from the project were more than $1 billion.
In contrast, The Bellevue in Bellevue, Washington, is a three-tower project highlighting the benefits of ethical, trusting contractor-designer collaboration toward a constructable design. Consisting of hotel, retail, and parking areas, a major structural challenge was minimizing the amount of transfer beams for the columns connecting the three areas. To achieve this, two structural concepts were presented by the designer, one included the use of a large transfer floor while the second presented slant columns as an option for connection. The final proposal was a combination of the two, which presented a messy, expensive, and nearly non-constructable design. To move forward, the project stakeholders united and collaborated on a path forward. To begin, they established a goal to minimize the number of columns within the towers, agreed to let the structural team control the column layout, and sought the architecture to fit. The designer’s result was a decrease from 20 columns to 12, elimination of two outrigger beams, and reduction from 14 column transfers to eight, resulting in a less expensive project for the owner and a desirable aesthetic for the architect due to a constructable design for the contractor.
PRO envisions a concrete industry that quickly and effectively evaluates, shares, and adopts technology, materials, and processes that improve the constructability and productivity of design and construction for concrete structures of all types. PRO and its members can advance new technologies and processes that improve productivity well beyond historic levels. The Center is seeking involvement from all industry stakeholders, especially structural engineers who wish to be industry leaders in advancing concrete design and construction. Of note to structural engineers: ACI recently launched a new Certificate Program that covers planning, layout, project delivery,
project site drivers, and structural system concept design. ACI Certificate Programs, delivered through ACI University, encourage concrete professionals to gain in-depth knowledge about topics in concrete materials, design, and construction by following a defined online course of study. Once a Certificate Program has been completed, participants receive a certificate and digital badge that can be used on résumés, job board profiles, social media, email signatures, and more. The Concrete Constructability Certificate Program reviews structural system selection, specification of concrete, selection of reinforcement, and testing and inspection. The program serves as a great introduction or refresher to constructability issues for designers with various levels of experience.
PRO also released its Constructability Blueprint, which serves as a starting point for improving construction productivity and project value. The Blueprint will be updated, expanded, and modified to ensure the best and most current guidelines for advancing productivity. It represents the recommendations of PRO’s members, who are collectively committed to continually improving the constructability of design and productivity. A PDF copy of the Constructability Blueprint can be obtained from any PRO member or at concreteproductivity.org. As a catalyst for overcoming barriers to advancing concrete construction productivity, PRO plans to empower and motivate the next generation of structural engineers and constructors to be actively involved in supporting improvements in design and construction methodologies. In addition to the initiatives and resources previously mentioned, the Center’s core functions include:
• Industry outreach and awareness
• Advancing automation
• Interaction with ACI technical committees on constructability
• Technology validation
• Construction productivity resources
• Knowledge transfer and professional development
Several forward-thinking, industry-leading organizations have already joined the Center as members. PRO membership is open to individuals and organizations interested in advancing concrete design and construction. This includes industry leaders, concrete contractors, structural designers, construction companies, concrete producers, manufacturers, federal and state agencies, and more. To learn more about PRO or to become a member, visit concreteproductivity.org.
Phil Diekemper is the Executive Director of PRO: An ACI Center of Excellence for Advancing Productivity. Cary Kopczynski is the CEO of CKC Structural Engineers and is a past ACI President. He chairs the PRO Board of Directors. Mike Tholen is the Senior Managing Director, Technical Operations at the American Concrete Institute. He is a member of the PRO Board of Directors.
Geology, hydrology, hydraulics, and more go into the structural engineering puzzle of each dam project.
By Aimee Corn, PE
If your exposure to the world of dams is limited to flownets or calculating the triangular distribution of water load in one of your undergraduate classes, maybe a childhood trip to the Hoover Dam, or recreation at your local reservoir, you might be wondering what exactly a dam structural engineer does. You are not alone! Many people wind up in this industry by chance.
The United States has more than 91,000 dams, with an average age around 62 years old. Dams serve a variety of purposes, including storing drinking water, flood control, recreation, and hydropower. Every four years, the American Society of Civil Engineers releases an infrastructure report card which aims to depict the condition and performance of American infrastructure, assigning letter grades based on the physical condition and needed investments for improvement. While the overall grade for America’s infrastructure was a “C-,” Dams
received a solid “D.” Part of this is due to the fact that over the last 20 years, the number of high-hazard potential dams has more than doubled.
The hazard classification reflects the hazards downstream of the dam: the potential consequences if the dam were to fail, laid out in Figure 1. High hazard dams are dams that have the potential for economic loss as well as the potential for loss of life if the dam were to fail; these dams are held to the most stringent safety standards. The hazard classification can change over the lifetime of the dam. As populations continue to grow downstream, more dams have the potential to become high hazard structures.
The oldest dam in the U.S., Old Oaken Bucket Pond Dam in Massachusetts, was completed in 1640. The early 1900s ushered in an era of “big dam” building in America as demands for water storage and electricity increased. Dam construction increased during the Great Depression and spiked the decade after World War II was over. Dam construction peaked in the 1960s and decreased rapidly after the 1970s.
Gravity dams rely on their self-weight to maintain stability and retain the reservoir they impound. Gravity dams have been around for thousands of years. Many older gravity dams were constructed with large masonry blocks. Current construction practices utilize mass concrete, which relies on the material properties of the concrete; steel reinforcement is generally not used.
Arch dams significantly cut back on concrete material and rely on the compressive strength of concrete. Due to arch action, the arch efficiently transfers loads into the abutments and foundation utilizing its unique shape and geometry. Arch dams can be thin or thick, and some are double curvature, meaning they have curvature in the vertical and horizontal directions.
In a slab and buttress dam, an inclined reinforced concrete deck slab holds back the reservoir load. A series of corbels and buttresses on the downstream side support the upstream sloped slabs. Slab and buttress dams are often referred to as Ambursen dams. Neil Ambursen patented a reinforced concrete design for this system in the early 1900s. This technique was used to construct more than 100 slab and buttress dams in North America.
What state has the most dams?
#1 Texas (7,374)
#2 Kansas (6,427)
#3 Mississippi (6,114)
#4 Georgia (5,457)
#5 Missouri (5,366)
What state has the fewest number of dams?
#50 Delaware (83)
#49 Alaska (110)
#48 Hawaii (128)
#47 Rhode Island (235)
#46 Vermont (371)
What state produces the most hydropower?
#1 Washington
#2 Oregon
#3 New York
#4 California
#5 Tennessee
Embankment dams are typically comprised of compacted earthen material. Most have a central core composed of an impermeable material to stop water from seeping through the dam. This type of dam is well suited for sites with wide valleys and can be built on hard rock or softer soils.
When thinking of structural engineering, the various codes and standards that typically come to mind include ASCE 7, the International Building Code, AASHTO, the AISC steel manual, American Concrete Institute (ACI), as well as wood and timber standards. Concrete dam analysis relies on guidelines developed by the Bureau of Reclamation, Federal Energy Regulatory Commission (FERC), and U.S. Army Corps of Engineers. These guidelines rely on fundamental engineering principles and place a significant emphasis on engineering judgment when evaluating these structures.
The Bureau of Reclamation has Design of Small Dams as well as Best Practices and several engineering monographs. The FERC produces chapters pertaining to several types of dams and associated features and appurtenant structures, such as Chapter 3 which covers Gravity Dams and Chapter 11 which covers Arch Dams.
The U.S. Army Corps of Engineers also publishes a variety of engineer manuals for the design and evaluation of dams. These guidelines lay out recommended factors of safety associated with key failure modes.
The gravity method of analysis is used to analyze concrete dams. This method assumes that the dam is a two-dimensional rigid block, and the sum of the forces and the sum of the moments equal zero. The foundation pressure distribution is assumed to be linear. The gravity method of analysis should be completed before proceeding on to more rigorous studies since it provides good estimates at low computational costs. In most cases, if the gravity method of analysis indicates that the dam is stable, no further analysis is necessary. The drawback to the gravity method of analysis is that the analysis does not consider dynamic behavior characteristics which can magnify the effects of earthquake ground motions in the upper section of the dam. The usual load combination is the normal, everyday load the dam
sees. This includes the weight of the concrete, the load of the reservoir and tailwater, and sediment. Some evaluations also include thermal evaluations that look at how the ambient and reservoir temperatures impact the overall behavior of the concrete. Another usual load combination may include the addition of an ice load for reservoirs in areas where the reservoir freezes.
The unusual load combination evaluates the same conditions as the usual load case, with an increase in the reservoir level to reflect the flood load. For high hazard dams, this is typically the Probable Maximum Flood (PMF). The PMF is the worst-case scenario flood and may be anywhere between a 10,000-year event to a million-year event. For dams having a low hazard potential, the project should be stable for floods up to and including the 100-year flood.
The extreme load combination evaluates the seismic event. For high hazard dams, this is the maximum credible earthquake and is typically around a 10,000-year event. Advancements in earthquake understanding over the last few decades have shown engineers that seismic hazards may be higher than originally thought when many of the dams in the U.S. were designed. Current seismic loads can be double or even triple what the dam was designed for.
The post-earthquake load case is the usual load case accounting for the damage that occurs during the earthquake.
Potential failure modes or PFMs are the hypothetical chain of events that could lead to unsatisfactory performance of the dam and are a crucial step in understanding the behavior of a concrete dam. The analyses structural engineers perform parallel the potential failure modes; think of the failure mode event tree as an outline for the evaluation of the problem.
In concrete dams, concrete overstressing and rotational and sliding stability are the basic failure mechanisms typically evaluated.
In mass concrete, when evaluating for concrete overstressing, the evaluation compares the computed stresses from the analysis
against the allowable strength of the material. If the computed stress exceeds the capacity, then the concrete is assumed to crack or crush.
Rotational and sliding stability evaluates sliding stability of the dam along analysis planes based on minimum allowable factors of safety. Evaluations along the dam/foundation interface are often referred to as global stability. Analysis of sliding planes within the dam section evaluate internal stability. Depending on the foundation material, sliding planes within the foundation may also be evaluated.
and the complexity of the modeling software. These simpler methods can serve as a quality check for more sophisticated studies.
Developing a potential failure mode consists of three specific parts:
1) The first is the initiating event. This can be normal operations, a defect in the material, a flood, or earthquake.
2) The next part of the PFM is the progression. This is the development of steps that define the process leading to failure.
3) For failure, there needs to be a quantitative result. This may be failure of the structure or an uncontrolled release of the reservoir.
During a potential failure modes analysis (PFMA) workshop, a group of people, including the dam owner and subject matter experts, brainstorm and identify all the PFMs for a dam. Once the team identifies the PFMs, the progression is developed for all relevant failure modes. Through this exercise, all participants gain a better understanding of how the dam functions and could fail. Dam owners can use the lessons learned to help shape their dam safety program.
Through the PFMA process, risk reduction measures are identified that can be implemented to help prevent the full progression of the failure mode. The PFMA process is set up to help identify unknown flaws in the dam. Surveillance and monitoring measures should be in place to prevent these failure modes from occurring. There is not a set number of failure modes one should identify on a project. Ideally, enough PFMs are identified to provide a full understanding of the threats to the safety of the project.
Several analysis options are available; the proper method of analysis depends on the problem at hand and the level of complexity needed.
It is good practice to start simple and increase complexity as needed. Typically, the initial step is hand calculations, utilizing spreadsheet or Mathcad analyses, which lend themselves well to the gravity method of analysis. It is important to understand the general behavior of the dam before delving into more sophisticated analysis options. With more complex analyses, it is easy to get lost in the multitude of parameters
The more sophisticated analysis options include two-dimensional and three-dimensional finite element analyses using software such as ANSYS or Abaqus. Finite element models allow for more complex studies, such as thermal and dynamic loading combined with static loads to better simulate actual behavior of the dam and understand the load paths associated with the entire damfoundation-reservoir system. The need for 2D or 3D depends on a variety of things, such as changes in geometry or capturing material changes in the dam or foundation. A two-dimensional finite element model may be sufficient to capture the behavior of a gravity dam with no changes in geometry throughout its length, while a three-dimensional model may be required to accurately represent an arch dam which relies on its shape to achieve stability.
As discussed previously, the hazard classification of a dam can change over its lifetime.
The arch dam in Oklahoma in Figure 2 is located within the boundary of a wildlife refuge. When it was designed and constructed in 1936, there was no population downstream of the dam and it was designed for minimal seismic loading.
The owner completed a hazard classification study of seven dams in the refuge, which involved developing inundation mapping of the flood waters if the dams were to fail. This study showed a population of about 1,000 was at risk directly south of the dam. This raised the hazard classification to high, now requiring the most stringent
safety standards. The understanding of seismicity has also improved in this area, resulting in the need for an updated analysis of record, as the maximum credible earthquake at this site is quite high. The engineering team performed a structural stability analysis of the dam using a 3D finite element model to evaluate the safety of the dam for the increased loads (Fig. 3).
The arch dam in Colorado in Figure 4 was originally constructed as a gravity dam in the late 1930s. An arch dam was constructed on top in 1959, raising the dam to over 200 feet tall. In this example, the foundation is the focus. The geology and the foundation are crucial for the performance of a concrete dam, especially an arch dam. For arch dams, one of the failure modes evaluated is rock block stability. Engineers must evaluate the stability of potential rock blocks in the abutment formed by the intersection of foundation discontinuities. This failure mode has the potential to develop during normal, flood, and earthquake loading conditions. In the development of the PFM, the thrust load from the dam and uplift pressures could dislodge a rock block resulting in a loss of arch support and failure of the dam.
Rock anchors were placed in the foundation in the late 1980s. The role of the structural engineer on this project was to model the dam with a three-dimensional finite element model and evaluate the magnitude and directionality of the thrust loads on the abutment for the various loading conditions to determine if this failure mode was viable or could be ruled out.
For the third example in Figure 5, a slab and buttress dam built in the 1930s had concern of corrosion of the steel reinforcement. Localized deterioration had routinely been observed during regular inspections, so the quality of the concrete was in question. Core samples were taken, and the concrete strengths and reinforcement conditions were evaluated. Several failure modes associated with structural capacity of the reinforced concrete slabs and corbels were evaluated. Hand calculations were used to analyze the loads on the structure and
What year was the oldest dam (still standing) in the U.S. completed?
calculate the nominal capacities of the individual members accounting for various degrees of deterioration. These results were used to determine a proper plan to address deterioration concerns and how to prioritize what regions to focus on first for repair and rehabilitation measures and enhance the service life of the dam.
and rehabilitation measures due to deterioration concerns.
From the geology of the foundation, the hydrology of the reservoir and the hydraulics of the spillway to the mechanics of the dam’s behavior, a structural dam engineer utilizes the full civil engineering degree. Every dam project is a new and exciting puzzle to solve. While the design of new dams is not as common as it once was, extending the life of America’s critical infrastructure and improving dam safety is a crucial need and provides a rewarding career path.
Aimee Corn, PE, M.ASCE, is Sr. Project Structural Engineer at Gannett Fleming specializing in the analysis of existing concrete dams. Corn served on the SEI Board of Governors from 2018 to 2022 and was the 2023 recipient of the ASCE Edmund Friedman Young Engineer Award for Professional Achievement.
REAL-TIME, IN-SITU CONCRETE STRENGTH OPTIMIZE SCHEDULE BY STRIPPING FORMWORK SOONER
AI STRENGTH PREDICTIONS BASED ON SITE LOCATION
TEMPERATURE MONITORING FOR THERMAL
CONTROL PLANS
At the heart of the Signal System is the DEWALT Signal Concrete Sensor: our fully wireless, embeddable sensor that measures in-situ concrete temperature and strength data, uploading to cloud in real time via the DEWALT Signal Live Hub or periodically with the ConcreteDNA software app.
APPLICATIONS
Construction is a vast and diverse industry, with different sectors and project types requiring different applications of the same technology. You'll find some of the main use cases of our technology and how it applies to you.
Temperature measured in real time and location, in conjunction with traditional quality methods, provides more accurate jobsite pour information.
Wireless technology automatically collects temperature from signal sensors. Temperature differentials are calculated and thresholds monitored in real time.
Take action without delay and strip formwork up to 30% faster. Real-time sensor data tells you precisely when concrete has reached critical strength. Set up notifications to alert your device when it's time to remove forms or pull tendons.
Signal Live Hub allows for continuous data collection from embedded sensors.
The smartphone application gives visibility to field teams for efficient decision making.
By combining local weather forecast, historical curing data, and real-time sensor measurements, DeWalt Signal Sensors enable your teams to plan like never before. Thanks to +/- 5% prediction accuracy, sites run smoothly and projects are delivered to plan.
The ConcreteDNA software centralizes and organizes strength data from all pours. Project managers can then make informed decisions on time critical activities such as: tensioning, stripping, and loading.
DeWalt Signal Sensors are Bluetooth enabled and transmit data to the app on your smart device or the cellular hub, all backed up on the cloud. No wires or external collection boxes to risk system damage or water ingress.
Use your data to understand exactly how to create your mix, without overdesigning. Reduce cement and admixtures to optimize pours thus reducing cost and carbon.
Coordination, collaboration, and creativity were required to meet the structural engineering challenges created by the building's unique canyon feature.
By Austin Reese, PE
Denver, Colorado, is known as a city where residents enjoy a connection to the outdoor lifestyle due to the city’s proximity to the Rocky Mountains. One River North, one of Denver’s newest buildings, was designed to reflect that connection and give residents a place to call home with the convenience of the city and feel of the mountains. Worldrenowned architecture firm MAD Architects based in Beijing, China, dreamed up a building design that would provide a mountain-like feel in luxury urban housing.
The building stands out as unique due to the prominent canyon-like features that meander across the building face and are meant to resemble a structure that has been overtaken by nature. The canyon features make the design unlike any other, which forced the construction and design teams to come up with collaborative and creative solutions for the unique geometry. The design team consisted of MAD Architects, who provided the overall architectural design, Davis Partnership Architects who served as local Architect of Record and who also provided the interior and landscape design, Jirsa Hedrick who performed the structural design, KHSS who provided the design of the canyon plaster system, and many others. Saunders Construction Inc. served as the project general contractor and provided pre-construction design assist services.
One River North is 16 stories above grade with three stories of belowgrade parking beneath. The ground level consists of retail space, the main resident entry lobby, resident amenities such as bike storage and pet wash, some mechanical rooms, as well as the porte cochere and
access to the parking garage below. The three below-ground parking levels provide approximately 175 parking spaces for building residents. Levels two through sixteen are residential units, totaling over 180 units. The building boasts thousands of square feet of outdoor amenity spaces, most of which are found on levels six through eight where residents enjoy the sprawling canyon landscape with seating areas, fire pits, a walking path and waterfall. An additional outdoor amenity space on the roof provides residents with a rooftop pool, hot tub, seating areas and views of the mountains to the west.
The building foundations, structural walls, columns, and slabs are cast-in-place concrete. The building foundation system is made up of 76 drilled piers that extend from the basement level into the bedrock below with a typical total length of 35-45 feet. The piers range in diameter from 24 inches to 60 inches at the heaviest loaded columns. The pier cap below the building core walls has nine piers that provide the lateral resistance at the foundation, these piers are 48 inches in diameter with almost 2% vertical reinforcing. Due to the unique geometry, the building has irregular column spacing, but an approximate 28 feet by 28 feet column grid is used where possible. The columns come in 19 different shapes, and some of the largest sections used include 36-inch diameter round columns and 26-inch by 48- inch rectangular columns. Round columns were preferred for aesthetics at outdoor spaces, but square and rectangular columns were preferred at interior applications. The columns that pass vertically through the canyon space had to be coordinated in many locations to
account for the change in shape from rectangular to round and back. Columns were constructed with high strength 8000 psi concrete. The floors of the building are typically two-way, post tensioned slabs that are 8 inches or 9 inches thick in residential areas. The slab thickness is increased in areas with large column spacing or heavy landscape loads, with corresponding increased reinforcing. Slabs were placed with 6000-psi concrete. The building lateral system is provided by the single, box-shaped elevator core at the center of the building. The elevator core walls are 18 inches thick and utilize the 8000-psi concrete mix. The canyons that make the building look so unique are a special panelized plaster and mesh system, referred to as the CHIPS system, that mounts to steel tabs fastened to the structural slabs. The building cladding is a combination of stucco panels in the back and curtainwall system on the front facade.
The construction of the project began in 2021 with excavation of
the existing site down three levels to the basement elevation and erection of the tower crane. Installation of the drilled piers and basement structure took approximately six months, with construction of the ground level slab in spring of 2022. The building was topped out just nine months later in December 2022. The building elevation was visibly different from a typical project during construction, but it wasn’t until the installation of the CHIPS system that the general public realized how unique the building was going to be. Installation of the CHIPS system began in fall of 2022 and wasn’t fully completed until late 2023 at which point the project began attracting increased local media attention. After just three years of fast-paced construction, the building was completed and opened to the public in April of 2024.
The CHIPS system that adorns the building uses the same technology used to build man-made mountains at theme parks and is designed and fabricated by a third-party specialty company. The
system gets its name from its resemblance to a potato chip. Each of the CHIPS segments came out in hundreds of prefabricated mesh panels, typically 48 inches by 48 inches in size. The CHIPS panels were mounted to steel columns and braces that were fixed to embed plates cast into the structural slabs and columns. Once all panels are installed and tied together, they are coated with several applications of plaster and paint. The support system required the placement of thousands of embed plates prior to pouring the concrete slabs and columns, and there were zero missed embed plates through the entire course of construction. Ensuring all embed plates were placed and located correctly was achieved by TEKLA laser scanning technology and integration into the project Navisworks model. Missing or misplaced embed plates were flagged and corrected prior to placement of the concrete. The CHIPS unique geometry was modeled by the MAD Architects team in Rhino 3D software, and the Rhino BIM model was a contract document used by the CHIPS fabricator to produce the desired shapes and curves.
Simply attaching the CHIPS system to a flat-faced structure would not achieve the full desired slot canyon effect. Therefore, in many locations the curves of the canyon CHIPS geometry match up with the structure below to make a gash in the face of the building and create a penetrating reveal into the structure behind the cladding. Many of the final curves were modeled using Rhino splines—curves without definable geometry in 2D documentation. The edges of the structural slabs are defined in the structural drawings for typical locations but the structural team deferred the curved areas to the architecture team to convey due to the complexity. The architectural team plotted the curves on an X-Y grid system to document the unique geometry. The slab edges were then surveyed extensively to ensure the curved formwork aligned with the BIM models before placing the concrete. The general contractor utilized TEKLA’s Augmented Reality goggles which allowed the user to see an overlay of the BIM model
while on site and verify if the curved formwork aligned with the design. Many of the curved areas were heavily reinforced to support the landscape loads, and thus a substantial amount of reinforcing would had to be field-trimmed to fit into the various corners and curves.
Another challenge in construction was the coordination of the concrete floor shoring and reshoring operations. In general, the structural team required a minimum of two floors of reshores in addition to one floor of formwork, requiring three floors total of support for each slab pour. The reveals in various parts of the canyon created conditions at some levels where there may not be a slab below for three levels and required the shoring in these areas be supported by slabs five or more levels below. The shoring coordination added another hurdle for the construction team to coordinate activities in the areas that would be blocked by shoring far longer than typical. In an effort to keep glass installation on schedule the structural team provided a loading allowance for limited construction activities in reshore zones.
A crack running through the structure is usually the last thing a structural team wants to hear about; in this case, the crack in the face of the structure was the defining element and couldn’t be glossed over. The building’s unique gash feature is accomplished by carefully curving the slab edges to mold to the shape of the canyon. The levels with canyon features, levels six through the roof, all have unique geometry and require unique reinforcing. The side of the building facing the alley—referred to as the backbar—was the same for levels three through 17. The building floors were constructed in two separate concrete pours, typically spaced a week apart allowing for a completed level every two weeks. Due to the complexity of the reinforcing and demanding
overall project schedule the structural team was on site to review the reinforcing for almost every concrete pour which included weekend, sunrise, and sunset site visits throughout the course of construction. Floor slabs were designed using RAM ConcePT post-tensioned slab analysis software. The floor geometry was exported from Revit for each level to properly analyze the slab edges and landscaping load geometry. In most instances the landscape loads were located near the edges of the floor slabs in cantilever zones so additional post tensioned reinforcing had to be placed to reduce slab edge deflection and provide the required capacity. While typical interior slab areas may only have 150 psi of precompression, the heavily-loaded landscape areas
to account for anticipated deflections from the cladding, landscape, and CHIPS weight (approximately 15-psf). Locations with extensive heavy landscaping, such as the level 6 waterfall feature, are thickened to 12 inches with additional heavy mild reinforcing.
One of the design challenges for the post-tensioned slab system included a three discontinuous columns that supported several levels above level 1 but did not continue to the basement below and had to be supported by a thickened transfer slab at level 1. The columns stop at level 1 and are supported by the 40-inch-thick PT slab below, with over 100 PT tendons in each direction and reinforcing mats and ties at 9-inches on center. The 24 inch by 36 inch discontinuous column has a design load of over 2000 kips that is transferred via the post tensioned slab to the columns over 10 feet away below.
The construction of the transfer slab was also challenging because it required the rebar installers to crawl fully inside the rebar cage and lay horizontally to place the cross ties. Despite the large balanced load, enough mild reinforcing was provided to resist initial stresses and avoid having to use staged stressing.
One River North is located directly next to a large trainyard and several large parking lots which all contribute to a C exposure classification. The building is classified as Seismic Design Category B, so the wind loading controlled the lateral design over seismic effects. The building was studied with a wind tunnel analysis by local wind tunnel expert Cermak Peterka Peterson (CPP Wind) who provided the design team with MWFRS and components and cladding design loads. The building lateral analysis was performed using RAM Structural System with a lateral model calibrated to correlate with the wind tunnel criteria. The design team considered building drift, backstay effects, and system ultimate capacity with multiple RAM models that were used to envelope the various possible conditions.
The building lateral system is provided by the concrete elevator core walls which make up a 30 feet by 30 feet square at the center of the building. The core walls are 18-inches thick and have varying levels of reinforcing. A corridor passes through the elevator lobby and openings for the corridor are required on each side of the core. The link beams above the corridor openings at most levels are typically lightly loaded and easily designed with reinforced concrete sections. However, the lower levels with larger openings have shear stresses exceeding what is allowed by code with normally reinforced sections and required the design team to resort to an embedded W24x131 wide flange beam across the corridor openings at levels three through five. Embedding a steel beam with welded reinforcement inside the link beam formwork required extensive coordination with the construction team to ensure the beam could fit in the wall reinforcing mats. The first beams were placed at level three with minimal issues and the beams at levels four and five went in without any further coordination required.
At one time, One River North seemed like another project that would be doomed by the Covid 19 economic chaos and a prohibitive price tag. The design team was excited to take part in the project, but post-Covid economic uncertainty and fluctuating costs of construction meant a higher risk of project delay or budget constraint. Now the building is complete, and it is an undeniable example of Denver’s special architecture. One River North stands as a source of pride and accomplishment for all who worked on the project. For the structural team at Jirsa Hedrick, the project represented one of the most complicated ever undertaken as this project required a high level of collaboration, coordination, and creativity. By relying on expertise in BIM modeling and structural analysis software, the design team was able to navigate the difficult design and construction journey and successfully produce a design that blends nature with downtown Denver. ■
Los Angeles Clippers’ new carbon neutral arena crosses over into an all-encompassing facility.
By Ryan Anderson, SE, DBIA, and Sam Bass, PE
he new Intuit Dome in Inglewood, California, is more than a basketball arena to the NBA’s Los Angeles Clippers, it is the team’s home away from home. The visually striking 1.1 million-square-foot arena used exclusively for basketball and concerts opened in August 2024 and is the new benchmark for global arena design and engineering.
Intuit Dome’s oblong shape is due to the Clippers’ desire to have an all-encompassing facility—the arena’s seating bowl and basketball court, administrative offices, practice and training facilities as well as the critical infrastructure to ensure the arena is carbonneutral are all located within the structure’s
The LEED Platinum structure is completely powered by 2 MW of photovoltaic solar panels on the roof that provide 11 MW of power to batteries and related equipment housed in a central utility plant inside the structure. There is enough on-site energy storage to power a basketball game or concert while generating no new greenhouse gas emissions.
Because Intuit Dome is designed to exclusively host basketball games and concerts, it provides an intimate seating bowl for more than 18,000 fans and positions them up to 45 feet closer to the action on the court or the stage compared to traditional multi-use—i.e., hockey included—arena design.
Intuit Dome has eight distinct levels: two below grade, five levels above grade, and the main concourse at grade. The arena event level is founded at 32 feet below natural grade, and the top of the shell structure is 121 feet above natural grade.
In total, Intuit Dome is approximately 1,250,000 gross square feet and includes 965,000 square feet of elevated floor structure as well as 277,000 square feet of roof shell surface area, much larger than the typical arenas previously constructed.
Three primary structural construction typologies were instituted for the Intuit Dome—concrete substructure, steel superstructure, and the steel shell.
The concrete substructure contains the cast-in-place structure and shallow and mat foundations at or below grade. This includes the arena’s foundations, perimeter retaining walls, the slab on grade Event Level, and
Club and Plaza Level elevated slabs. The east side of the Plaza Level includes concrete slab over steel deck supported by steel trusses in the loading dock area. The lower bowl also utilizes precast concrete seating units sup ported by steel framing.
The steel superstructure contains the structural steel elevated floor framing above the Plaza Level, which is supported by the concrete substructure below. The Suite, Mezzanine, Mechanical, and Terrace Levels are supported by composite concrete over steel decks slabs with steel beams and columns.
Additionally, steel rakers support precast concrete seating while the concession areas and electrical control rooms have bare steel deck roofs and are supported on top of the Terrace Level. The concession areas are steel frame structures comprised of wide flange beams, HSS columns, and cold formed steel shear walls as the seismic force resisting system.
Finally, the shell structure contains both the typical bare steel deck roof over the top of the arena and a polytetrafluoroethylene and ethylene tetrafluoroethylene (PTFE/ ETFE) shell enveloping the rest of the roof and wraps around the structure on all sides. The shell structure utilizes circular hollow structural steel members in a diagrid shell—formed from a series of diamondshaped panels that reflect the geometry of a basketball net—to support the building enclosure made up of PTFE or ETFE membranes. The shell structure is vertically supported by traditional columns and “tree columns” with horizontal outriggers at the Suite and Terrace Levels. A 12-in. vertical gap was provided between the Plaza Level and the bottom of the shell and is not base supported at any location. It is supported laterally at the gutter—the interface between the arena’s traditional roof and diagrid shell—as well as at the Terrace Level (radial only) and Suite Level (radial only) utilizing toggle braces.
The seating bowl is broken into five different areas—upper bowl, mid bowl, lower bowl, the dugout suite area, and the Wall.
On the east side of the arena and comprised of over 50 rows of uninterrupted seating for
Owner: Los Angeles Clippers
Owner Representative: CAA ICON
Architect of Record: AECOM
Design Architect: AECOM
Landscape Architect: Hood Design
Studio
Structural Engineer: Walter P Moore
Civil Engineer: D & D Engineering
Enclosure Engineer: AECOM
Electrical Engineer: AECOM
Mechanical Engineer: Henderson Engineers
Plumbing Engineer: AECOM
Erection Engineer: Walter P Moore
Construction Manager: AECOM Hunt
& Turner Construction
Facade Contractor: Crown Corr/ETS
Steel Fabricator: Schuff Steel
Steel Erector: Schuff Steel
Concrete Contractor: Largo Concrete
over 4,500 fans is the Wall. This seating bowl builds on what other athletic teams, such as Providence Park, home of the Portland Timbers, have done: putting the loudest and most passionate fans into a designated area. The resulting sound from this area is designed to funnel down to the court.
Due to vibration, and aesthetic considerations, the west side of the seating bowl required a sophisticated and unique structural design due to the massive indoor event space located under the seating area at the Terrace Level.
The west upper concourse is a propped cantilever with the seating bowl serving to counterbalance the cantilevered floor plate. This results in vibrations from the west seating bowl translating to the upper concourse floor. Careful finite element analysis (FEM) of this seating and floor area with many iterations and studies for the west side of the seating bowl was required to ensure patron comfort.
Lightweight concrete precast seating units are used throughout the seating bowl. The west side of the seating bowl is supported by steel rakers. The lateral loads are collected and delivered to the floor diaphragms with under-bowl bracing or buckling-restrained braces at the bottom section of the lower bowl. The rakers are not connected to different floor levels to prevent them from acting like building braces. The lower bowl is connected to the Plaza Level, Club Level, and Event Level to eliminate seismic joints, as discussed later in the article, at the premium suites. Connections to the club level were designed for seismic inelastic drift compatibility forces. The high stiffness of the concrete shear wall system allowed for the design forces to be reasonable.
the arena on the Terrace Level features four basketball hoops with netting on the side, an auto-return system to get the ball back to the shooter, and an electronic scoring system for the team’s Shoot 360 interactive experience where fans can play. Several shade trellises were added for the outdoor concession areas.
On the east side of Intuit Dome, the glazing was designed for blast loads and engineered to ensure the safety of the patrons and the structure. The back up steel for the glazing consists of W24x columns and a cable system behind the glass to prevent any glass from entering too far into the interior space.
Both floors at the Plaza Level and Club Level are in the lower bowl, consisting of concrete flat slabs supported by concrete columns. The floors were designed as two-way slabs and analyzed under gravity loads. Flexural reinforcing was placed as top and bottom base layers of reinforcing bars in both directions with additional reinforcing where flexural demands exceed the capacity of base layers. Punching shear resistance at slab-to-column connection was provided using headed stud-rails.
Concrete beams were added where necessary to provide additional stiffness to the slab at long span conditions or around large openings such as elevator pits. Forty-two-inch deep concrete beams were also used to transfer loads at discontinuous columns from the Club Level and above to the columns below.
Furthermore, the structural design of the seating bowl took a different approach to luxury suites than other arenas to keep fans close to the action. Over 40 suite spaces in a horseshoe formation are located toward the back of the middle section of the bowl—allowing fans to sit in a regular bowl seat that is exclusive to the suite. Finally, 14 court-level suites, including four dugout suites with privacy glass, line the player tunnel. The 10 suites opposite the player benches also include access to seats in the lower bowl.
Intuit Dome has a substantial basement that measures 1,300 feet and includes a 300-foot-long loading dock ramp. Coordination of the transfer trusses over the loading dock was critical because they support
Also on the east side of the arena is a promotional basketball court that has a 60 feet ceiling space from the main deck all the way up to the upper concourse. A series of 95 feet long trusses support the concourse above this area. Structurally, the challenge centered upon the very large façade attached to the HSS cladding girts between the steel columns. The four-story steel columns are supported by concrete beams under the southern section and steel beams and trusses supporting the northern portion, resulting in differential deflections and complications to the glazing design. The steel columns and framing at the concourse are designed for blast loads. Additionally, the trusses in this area were designed to minimize the vibration felt on the terrace level above.
Structural design for the arena’s closure wall above the concession structure also took on special considerations due to the proximity to the roof shell and the differential movements. The walls were cantilevered from the top of concessions to allow for differential movement of the shell. The shape of each concession area is engineered with a curvature along the backside wall so it would avoid contact with the exterior shell but maximize interior space. This curved design of the concession areas is unique compared to traditional arenas with concession areas typically built as masonry boxes. Concession structures utilized sure board, light gauge steel panel shear walls for the lateral system and bare metal deck supported by steel framing for the roof gravity system.
Intuit Dome’s PTFE/ETFE roof shell rides along with the structure; however, it is not the primary structural seismic system. The 2,800-ton
diagrid shell frame roof is supported by seven trusses over the main arena weighing approximately 120 tons apiece and is comparable to a “hoopskirt” where it is rigid in its own way but then tied back to each successive floor with a toggle brace.
Because Intuit Dome is located approximately a mile from the Newport-Inglewood fault, the toggle braces can rotate in two directions in case of a seismic event. The toggle braces allow the shell to be laterally supported by primary structural floors at Levels 4 and 7 so the shell frame roof does not cantilever over 100 feet. However, because the toggle braces can rotate using universal pins, the shell can move freely during a seismic event and not experience high loads due to drift compatibility that would otherwise rip apart the shell. In this way, the roof shell acts as a façade element and not a primary structural element.
Additionally, the PTFE/ETFE diagrid roof shell that makes up the exterior of the arena covers the entire structure. The decision to use both PTFE and ETFE ensured the material could stretch over multiple panels because the diagrid roof shell has several different configurations. The dual roof material is watertight in the critical sections of the structure and a woven mesh in other areas to allow ample natural light and airflow, while also providing a horizontal and vertical decorative aspect.
One unique aspect of the roof is how it is used—or not used— over Intuit Dome’s indoor-outdoor lobby. The partially covered lobby, which includes the main entry and ticketing areas, measures 25,000-square feet and reaches 115 feet upward to the roof shell. The indoor-outdoor lobby is unique in that most venues have lobbies that are completely enclosed or completely outside. Different sections
and portions of the roof are open to allow light and air in to provide the aesthetic design qualities required by the Clippers. The roof is waterproof over the court, seating bowl, upper concourse, and one bay beyond the concession buildings at Level 7. Beyond the enclosure line, the shell acts as a shading and architectural element.
The lobby's indoor/outdoor nature allows the structure to use the Southern California climate for natural heating and cooling. There is no need to heat or cool a massive volume unnecessarily, resulting in a much more sustainable approach to traditional arena heating and cooling.
The team’s 71,000-square-foot, four-floor administrative building houses the team offices and is located above the practice facility and training center. A portion of the administrative building is abovegrade and visible to fans inside and outside of the arena, but the 86,000-square-foot, two-level practice facility and training center that includes two basketball courts, training, medical, and player spaces is primarily located below grade. The areas below-grade required a series of transfer trusses to ensure column-free spaces for the two practice courts and outlying areas. These steel trusses, which span 145 feet, play a critical role because they support the four floors of the administrative building above.
Above-grade, the exterior space around Intuit Dome includes a sunken garden on the west side, which is a private outdoor space for the team that includes a swimming pool, infrared sauna, outdoor track, lounge, and eating area. The sunken garden allows natural light into the training facility and practice courts while also providing privacy for the team.
The shell ties together all the different structures—from the arena to the admin buildings—under the entire roof. As a result, the lateral system of the admin building was tuned to prevent significant force transfer via the overhead shell.
Furthermore, the administrative building has vibration sensitive criteria that is exacerbated by supporting four floors on top of it in a staggered manner. In addition to keeping the floors level and acceptable for vibration, the trusses could not be increased in depth without impacting the practice court below. The discontinuous nature of this area forced the buckling-restrained brace lateral system to the extreme ends of the floor where columns connected all the way to the plaza level. This put significant seismic forces—up to 1,200 kips—on these floors and the buckling-restrained braces but allowed the transfer trusses to be designed without amplified seismic forces that would be required if braces landed on the trusses.
Contrasting the structural system layout used for the braces in the administrative building, within the arena the braces are spaced much closer together—70 to 80 feet apart compared to 140 feet apart within the administrative building.
Unique to Intuit Dome is its double-sided halo 4K scoreboard that features more than 233 million LEDs—the largest ever double-sided halo display in an arena setting—and similar in design to the Infinity Screen at nearby SoFi Stadium.
Most, if not all arenas, have a center hung scoreboard. Walter P Moore provided design and detailing to accommodate the unique shape and design that is well outside the norm for traditional centerhung scoreboards. The halo scoreboard’s scale is significant, with a
continuous perimeter that encircles an area larger than the basketball court and a height that allows the upper-level seating a generous view of the 30-foot-tall continuous screen.
Due to the complexity of size, shape, and weight, the halo scoreboard consists of vertical and horizontal trusses curved in plan to multiple segmented radii. It is a continuous structure with discontinuity only at two ends where there is a retractable segment for use during specialty shows and events. The halo scoreboard is hung from above where it crosses primary roof truss panel points. Because of this, the entirety of the halo was incorporated in the analysis of the roof structure to account for load redistribution and stiffening effects. Walter P Moore was also contracted to work in direct coordination with the erector and fabricator to provide construction and erection engineering services to facilitate speed and erection sequencing of the halo structure.
The halo scoreboard optimizes sightlines from all seats and places a priority on the viewing experience of fans seated in the upper bowls of the arena.
Comparable to the complexity of the structural design for the scoreboard is the internal rigging system used for concerts. The upper echelon rigging capacity is designed to handle the increasingly large loads for modern concert tours. Each individual rigging beam can support 5,000 pounds vertically or in a bridled configuration. From there, several different configurations were evaluated where a series of 5,000-pound loads could be placed throughout the arena, allowing for a maximum of 75,000 pounds on an individual truss but not more than 525,000 pounds using the seven total trusses inside the arena. The significant rigging capacity allows the arena to be a premier concert venue in addition to a tremendous basketball viewing experience.
The seismic base for Intuit Dome is 32 feet below the arena's natural grade. Seismic resistance is provided by a continuous perimeter concrete retaining wall and interior concrete shear walls. Above grade, the steel superstructure is utilized for gravity and seismic support with a buckling-restrained brace lateral system that provides lateral resistance and is transferred to the concrete shear walls below.
Comprising the roof and exterior envelope of the building is the iconic shell structure. It is part of the steel superstructure; however, due to its unique shape and configuration, all shell elements—generally above terrace—were treated as a diaphragm with overstrength loading design forces.
The steel superstructure utilizes a buckling restrained brace lateral system at the occupied floors above grade. The system stacks vertically and uses a single diagonal approach. The arena and administrative offices do not connect to the floor diaphragms from the architectural layout at Levels 5-7 and through a seismic joint at Level 4. However, the arena shell structure is supported above Level 7.
Buckling-restrained brace frames were analyzed with continuous columns and fixed or pinned connections at beam ends corresponding to the details used. Buckling-restrained brace frame column bases were modeled as pinned to maximize the design forces in the braces and detailed accordingly to minimize rotational strength and stiffness at the base.
For the concrete substructure, a special frame concrete shear wall utilizing the basement retaining walls and interior shear walls also support the buckling-restrained brace system above. Special shear wall detailing, and provisions utilize ACI 318-14 (Building Code Requirement for Structural Concrete) with the exception of Grade 80 reinforcement. The diaphragm was cast-in-place reinforced concrete slabs with the east side of the Plaza Level utilizing concrete over steel deck diaphragm over the loading dock. A static linear approach with equivalent lateral force procedure was used for the concrete superstructure design.
The construction of Intuit Dome is a landmark for the Clippers franchise, which has never owned its own arena. The eyes of the world will be on Intuit Dome this fall as a series of concerts will be held prior to the Clippers opening of the 2024-25 NBA season. In addition, the arena is slated to host the 75th NBA All-Star Game in 2026 as well as events for the 2028 Olympic Games in Los Angeles. Intuit Dome’s concept of a fan-first arena that includes ample public spaces and recreational facilities is a design engineering marvel that may jump-start an exciting new era of arena construction around the globe. ■
On a tight, urban site, LPA’s West Hollywood Aquatics and Recreation Center (ARC) uses inventive structural engineering to create space for community and well-being.
By Bryan Seamer, SE and John Wilson, SE
SER: LPA Design Studios, Irvine, CA
Architect: LPA Design Studios
MEP: LPA Design Studios
Landscape Architect: LPA Design Studios
Lighting Designer: LPA Design Studios
Owner: City of West Hollywood, California
Contractors (primary and specialty): Sinanian
Development, Los Angeles, CA
Steel fabricator: ADF Group, Inc., Laval, Canada,
Steel erector: DCCI, Santa Fe Springs, CA
Aquatic consultant: Aquatic Design Group, Carlsbad, CA
Structural software used: ETABS
Situated on a challenging site in a dense, urban area, West Hollywood Aquatics and Recreation Center (ARC) embodies many of the technical and constructability challenges common to urban infill projects. Designers were challenged to create space where there was very little, bending the program around existing structures, over a roadway, onto rooftops, and into a public park. The result is a unique, vertically stacked building that meets the needs of a diverse community and creates a new center for social and recreational life in West Hollywood. Designed by LPA Design Studios and completed in 2021, the project involved a host of innovative structural engineering strategies, including a long-span rooftop pool deck suspended over basketball courts, a new multistory building on top of an existing parking structure, a sky bridge over a public road and a three-story grand stair that cascades into West Hollywood Park.
The ARC comprises three separate structures totaling 138,000 square feet, each with its own set of structural engineering challenges. A four-level Recreation Center—which includes indoor and outdoor community recreation and wellness spaces, a multi-purpose gymnasium, and two pools (competition and recreational)—occupies an area tightly constrained by roadways and an existing building. On its roof, aquatic
facilities are supported above a 100 foot by 160 foot column-free gym by a two-way trussed steel space frame system. Across El Tovar Lane, a public roadway that runs through the project site, a new two-story Community Center sits on top of an existing concrete parking structure. Bridging the two buildings, a multi-level sky bridge spans across El Tovar Lane to connect the new rooftop pools and an existing tennis complex to a raised observation and recreation deck on the roof of the Community Center. These interconnected outdoor environments and the ARC’s main entrance are linked to the open space of West Hollywood Park by a three-story outdoor Grand Stair.
Central to the design was the city’s desire for an inclusive amenity that reflected the diverse West Hollywood community. Designers went to great lengths to create a facility “for everyone.” Community engagement was an impassioned, years-long process with more than 16 user groups and many arts organizations and neighborhood associations engaged. Meeting the diverse, competing needs of the community motivated the design team to explore innovative solutions and do more with less. LPA’s integrated design process was critical throughout, with structural engineers playing a leadership role in the creative process. The firm’s multi-discipline approach enabled the integrated team to develop collaborative solutions that may not have emerged if architecture and engineering had worked independently.
Aquatics have long been a part of West Hollywood’s identity. The previous “aquatic park” was a cultural and recreational hub of the community and an anchor to West Hollywood Park. The need to accommodate aquatics on the tight site led to the most significant structural engineering challenge of the project: suspending a rooftop pool deck above a column-free gymnasium.
Designers envisioned a structural system capable of suspending an Olympic-sized swimming pool and a second, large recreation pool above a 100-foot x 160-foot gym without the aid of columns. Adding to this weighty challenge, the support structure had to fit a strictly limited depth, bounded by the mandatory clear height of the basketball and volleyball courts below and the height of existing adjacent rooftop tennis courts that are accessible from the pool deck.
Every available inch of depth is utilized below and between the pools to support 400,000 gallons of water, a the pool deck amenities and an unreducible, 100 pounds per square foot live load. Two elements make up the support system for the pool: a 100-foot x 100-foot two-way steel trussed space frame, which spans to exterior concrete bearing and shear walls, and a 15-foot-deep x 4-foot-wide box truss tucked into the 100-foot-long slot between the two large pools. The bi-directional space truss creates a 5-foot, 3-inch deep geometric web of steel that minimizes deflection as the pools are filled and drained.
Structural design considerations included vertical deflection and related horizontal deformations associated with varying volumes of pool water as well as the effects of the large volume of liquid mass on the structure during seismic events. The steel rooftop structure supporting the two pools was structurally continuous, with most of the connections of individual members fully welded. To understand the deformation of the structural framing, designers studied various loading conditions representing different combinations of water levels in the pools. This included scenarios where both pools were full, only the Olympic pool was full, only the recreation pool was full, and both pools were empty. Each condition was also checked with and without live loading at the pool deck. The design team used the results of these analyses to determine that strategically cambering the two-way space truss at four control points would ensure that, when fully loaded, the structural steel would not encroach into the clear height required for club, high school and college basketball in the courts below. Designers used ETABS, by Computers and Structures Inc., as the primary analysis tool for both gravity and seismic analysis. LPA’s structural engineers worked closely with Sinanian Development,
the general contractor, Groupe ADF, the steel fabricator, and DCCI, the steel erector, to develop details for the two-way, steel-trussed space frame and optimize the fabrication and erection process. It was important to work closely with the contractor team to find creative solutions to reduce field welding of the two-way truss system. Further complicating the erection was the limited laydown space and tight access to the site. As such, shipping an entire length of a truss line was not feasible, Therefore, two shorter, parallel truss lines of the two-way truss system were shopassembled, including the chord and web members of the perpendicular truss. This allowed for a significant reduction of onsite welding. These truss pairs were then lifted into place and welded together to create one direction of the two-way truss span. The chord and web segments of the perpendicular trusses were then welded in the field, completing the two-way truss system.
Providing pedestrian access from the ARC to the adjacent West Hollywood Park, across a public roadway, was a key programmatic and user-experience challenge of the project. A popular gathering space that has served as home base for many large-scale community events and festivals, including celebrity affairs hosted by Vanity Fair and the Elton John AIDS Foundation, the park was conceived as part of the cohesive recreational experience of the ARC.
Designers wanted community members to be able to walk directly from the newly renovated West Hollywood Park, up the Grand Stair, to the rooftop above the Community Center, across the wide, multi-level skybridge and into the pool deck and sports courts on the fifth-floor roof. This relied on the flawless execution of two distinct structural innovations.
First, the three-story sculptural Grand Stair connects the Park and the Community Center rooftop while creating a new gathering spot and icon for the city. The 70-foot-long structure bears on the top deck of the Community Center but is seismically independent, relying on a large, horizontally cantilevered steel truss to anchor the base of the grand stair to an elevated, structural concrete plinth founded in the park. While the top of the grand stair structure receives vertical support where it connects to the rooftop deck of the Community Center building, it is designed to allow up to 9 inches of horizontal movement in any direction.
Second, a multi-level skybridge over El Tovar Road completes the rise from the park to the pool deck, cantilevering 30 feet from the Recreation
The compact, stacked nature of the multi-level facility provides minimal spatial impact on the tight sight while maximizing the programmed spaces and the creation of the largest continuous piece of open land in the city.
Center structure. Multiple structural levels share the cantilevered load to maintain necessary vehicular clearances below. The upper two framed levels rely on W30 and W40 cantilevered beams to support the skybridge from the ARC building, with no physical connection to the adjacent Community Center building with which it is functionally contiguous. To allow for future flexibility in its use, the Community Center relies on special steel moment frames for seismic resistance, while the recreation center’s main seismic force-resisting system is the special concrete shear walls that surround the gymnasium. To achieve this, the more flexible moment-frame structure needed to be isolated from the rigid concrete shear wall building to ensure regular, predictable behavior in strong earthquakes. This isolation was achieved by adding seismic joints between the two buildings at each floor level. Seismic joints are located at the south side of the Community Center where the ARC building skybridge extends over El Tovar Road. Over 15 inches of seismic drift can be accommodated between the skybridge and the Community Center with seismic drift joints in floors, walls and ceilings. Close collaboration between structural engineers, interior designers, and architects was needed to ensure that the drift joint detailing carried through all building systems.
The ARC is an example of structural design going above and beyond to accommodate a community. By suspending two pools
above a multi-sport gym, the design makes highly efficient use of the limited buildable space in the dense, urban site area. By making use of the rooftop and cantilevering structures to create connections between them, the design creates new spaces for West Hollywood’s vibrant social, arts, and culture scenes to thrive. And by connecting the ARC to the park, the project essentially adds square footage and program area to the only significant public green space in the city. Overall, the project shows what can be accomplished on a tight urban site when structural engineering has a seat at the table as an integral part of the design team. ■
Bryan Seamer is Director of Structural Engineering at LPA Design Studios. With more than 25 years of experience in new construction, seismic assessment and rehabilitation of existing buildings, he uses emerging technologies and innovative design strategies to create safe, high-performing, cost-effective structural systems that are long-lasting, durable and resilient.
John Wilson is a Structural Engineering Managing Director at LPA Design Studios. From the earliest design phases through completion, John works closely with engineers and architects to achieve a unique blend of structure and architecture that will provide safe, efficient and unique designs for clients.
New clarifications focus on repairs when there is substantial structural damage (SSD) or less than SSD due to snow load. By
Michael Fillion, PE
After many roofs were damaged from the 2011 and 2015 New England winters, The Structural Advisory Committee (SAC) to the Massachusetts State Building Code made an emergency amendment to its code to cover repairs to snow damaged roof components when there was less than substantial damage. Also of concern to the committee was when there was snow damage to more than one component in an area of similar construction such as a snow drift area, whether the other undamaged components of the same similar construction should be evaluated for compliance with the International Building Code (IBC). Through the Structural Engineers Association of Massachusetts, the SAC expressed their concerns to the Existing Buildings Sub-Committee (EBC) of the NCSEA Code Advisory Committee. The EBC listened, reviewed, and submitted International Existing Building Code (IEBC) code change proposals to be heard at the International Code Council (ICC) code hearings. The code change proposals introduced snow damaged components to the definition of substantial structural damage (SSD), repairs to snow damaged components when there is SSD as well as less than SSD. The code change proposals were successfully accepted into the 2018 IEBC.
2015 IEBC The definition of SSD is clarified stating that vertically oriented components such as columns and walls apply, not horizontally oriented components such as snow damaged roof framing. Chapter 34, Existing Buildings, is deleted from the IBC.
2018 IEBC Snow damaged components are added to the definition of SSD, repair provisions are added to address SSD snow damage, the repair of less than SSD was modified to address snow damage, and a drifting snow reference was added to Chapter 3.
2021 IEBC Snow load is added for the repair of gravity load-carrying components that have sustained SSD.
2024 IEBC Code confusion is discovered when addressing repairs to components that have sustained SSD from snow loads. Code changes to the 2027 IEBC are being proposed at the next ICC code hearings.
The first edition of the IEBC was issued in 2003 and included the Work Area Method and the Performance Method. In anticipation of Chapter 34 (Existing Buildings) of the IBC being deleted, the Prescriptive method was added in 2006. By 2015, Chapter 34 of the IBC had been deleted. In 2012, Repairs became a stand-alone chapter. Along the way, the NCSEA EBC continuously worked on reconciling the IEBC structural provisions of the Work Area and Prescriptive Methods achieving their goal by 2018.
From 2009-2012, many jurisdictions started adopting the IEBC with the introduction of the term "Substantial Structural Damage." At first, there was confusion about what building elements or components should be considered for SSD. Intuitively, some engineers considered any structural element, component, or member with significant damage to be SSD.
During the 2011 and 2015 New England winters, prolonged cold periods of sub-freezing temperatures and successive snowstorms resulted in near-code level snow loads with hundreds of damaged roofs and some partial and total building collapses. In 2011, snow loads collapsed all the wood roof trusses of a one-story building; the exterior load bearing/shear walls however were all intact. The structural engineer for the project determined that the building had sustained SSD. This interpretation was not unusual then. As a clarifying response to this confusion, the definition of SSD was revised in 2015 to clearly state that damage to elements of the lateral force-resisting system (Item 1) and components carrying gravity load (Item 2) applied only to vertically orientated elements and components such as the columns and walls as highlighted in red of the building model in Figure 1. The newly revised interpretation of the 2011 event is that the collapsed roof trusses did not qualify for SSD because there was no damage to the vertical elements of the lateral force-resisting system nor the vertical components carrying gravity load when using the 2015 IEBC.
Excerpted below is the current 2024 IEBC definition of substantial structural damage. Item 3 was added in 2018. The light blue text was added in 2024 as clarifying language. Clearly when evaluating a building for damage, the amount is determined before repairs are specified. The underlined text in red is clarifying language suggested by the NCSEA EBC for the 2027 IEBC.
SUBSTANTIAL STRUCTURAL DAMAGE. A condition where any of the following apply:
1. The vertical elements of the lateral force-resisting system have suffered damage such that the lateral load-carrying capacity of any story in any horizontal direction has been reduced by more than 33 percent from its predamage condition.
2. The capacity of any vertical component carrying gravity load, or any group of such components, that has a tributary area more than 30 percent of the total area of the structure’s floor(s) and roof(s) has been reduced more than 20 percent from its predamage condition, and the remaining capacity of such affected elements, with respect to all dead and live loads, is less than 75 percent of that required by the International Building Code for new buildings of similar structure, purpose and location.
3. The capacity of any horizontal structural component carrying snow load, or any group of such components, that supports more than 30 percent of the roof area of similar construction has been reduced by or related to snow load effects more than 20 percent from its predamage condition, and the remaining capacity with respect to dead, live and snow loads is less than 75 percent of that required by the International Building Code for new buildings of similar structure, purpose and location.
For purposes of this definition, work done to implement repairs shall not be considered damage that reduces structural capacity. Clarifying Language Reason Statement: For the definition of SSD Item 3, it is clarified that only horizontal roof framing damaged by snow of a certain magnitude will need to comply with section 405.2.5. As written, this third trigger might be interpreted by some to require compliance with 405.2.4 and 405.2.4.1.
During the winter of 2015, The Massachusetts Structural Advisory Committee to the Massachusetts Board of Regulations and Standards reacted to how components damaged by snow were repaired. Since most of the damaged components were horizontally orientated roof rafters and beams, they were considered less than SSD and were permitted to be restored to their pre-damage condition.
Some causes of snow damage were related to deterioration, construction defects, missing stability bracing, added dead load and commonly, the effects of drifting snow, for which the IEBC allowed these components to be replaced without consideration for the load that damaged them. Light framed metal buildings were also vulnerable, in some cases stability bracing was absent and particularly in the cases where taller buildings were constructed adjacent to them creating a drifting snow condition for which they were not designed. As a reminder to design professionals when this condition occurs, section 304.2 was added to the IEBC in 2018.
304.2 Snow loads on adjacent buildings. Where an alteration or addition changes the potential snow drift effects on an adjacent building, the code official is authorized to enforce Section 7.12 of ASCE 7.
Massachusetts made an emergency amendment to its State Building Code requiring framing components damaged by snow, to be repaired to comply with current IBC snow load criteria. Otherwise, damaged components as depicted in Figures 3 and 4 would have been allowed to be restored to their pre-damage condition. Through Massachusetts’s relationship with the NCSEA EBC, code language was proposed at the ICC code hearings resulting in the following 2018 changes for repairs for less than SSD when there is snow damage:
405.2.1 Repairs for less than substantial structural damage. Unless otherwise required by this section, for damage less than substantial structural damage , the damaged elements shall be permitted to be restored to their predamage condition.
405.2.1.1 Snow damage.
Structural components whose damage was caused by or related to snow load effects shall be repaired, replaced or altered to satisfy the requirements of Section 1608 of the International Building Code. When navigating the IEBC, the practitioner should always review Chapter 3, Provisions for all Compliance Methods, to see what may apply. For less than SSD, components not damaged by snow loads are permitted to be restored to their predamage condition in accordance with 302.4 and all new structural members and connections for SSD and less than SSD, shall conform with 302.4.1.
302.4 New and replacement materials.
Except as otherwise required or permitted by this code, materials permitted by the applicable code for new construction shall be used. Like materials shall be permitted for repairs and alterations , provided that unsafe conditions are not created. Hazardous
materials shall not be used where the code for new construction would not permit their use in buildings of similar occupancy, purpose and location.
302.4.1 New structural members and connections.
New structural members and connections shall comply with the detailing provisions of the International Building Code for new buildings of similar structure, purpose, and location.
Exception: Where alternative design criteria are specifically permitted.
Following the 2011 and 2015 New England winters, concern was also placed on when damage occurred to more than one component of similar construction due to snow load. Are the other components of similar construction at risk of damage or collapse if they become similarly loaded? This can occur with drifting or unbalanced snow patterns that can change with wind direction from event to event, year to year. The NCSEA EBC reviewed these concerns and proposed language that was accepted and adopted into the 2018 IEBC. For SSD, Item 3 was added. The roof framing components depicted in red and green in Figure 4 are the ones that apply.
When using the 2018 IEBC, the following repair provision applies to SSD Items 2 and 3.
405.2.4 Substantial structural damage to gravity load-carrying components. (2018 IEBC)
Gravity load-carrying components that have sustained substantial structural damage shall be rehabilitated to comply with the applicable provisions for dead and live loads in the International Building Code . Snow loads shall be considered if the substantial structural damage was caused by or related to snow load effects. Undamaged gravity load-carrying components that receive dead, live or snow loads from rehabilitated components shall also be rehabilitated if required to comply with the design loads of the rehabilitation design.
Moving into the next 2021 code cycle, The Structural Engineers Association of Colorado proposed that snow load be included in the repair of gravity load-carrying components that have sustained SSD, and the change to section 405.2.4 was accepted into the 2021 IEBC. This made good sense for the case of a column that has sustained SSD whose dominant loading condition is snow, otherwise the repair would likely consider the minimum roof live load of 20 psf and perhaps not the controlling effect such as a 40 psf minimum regional roof snow load that may apply.
As a result, section 405.2.4 was modified, and section 405.2.5 was added in the 2021 IEBC (excerpted below). There have been some slight changes to the 2024 IEBC shown in light blue. With the changes to the 2018 & 2021 IEBC, there has been unintended code confusion when addressing SSD. The NCSEA EBC has suggested striking some of the language and adding language in red clarifying the intent for which they were written. The EBC has also included their clarifying language reason statements. These revisions are being developed as proposed changes to the 2027 IEBC.
405.2.4 Substantial structural damage to gravity load-carrying components.
Vertical components carrying gravity load -carrying components that have sustained substantial structural damage shall be retrofitted to comply with the applicable provisions for dead, live
and snow loads in the International Building Code. Undamaged vertical components carrying gravity load -carrying components , including undamaged foundation components , that receive dead, live or snow loads from retrofitted components shall also be retrofitted if required to comply with these design loads.
405.2.4.1 Lateral force-resisting elements.
Regardless of the level of damage to vertical elements of the lateral force-resisting system, if substantial structural damage to vertical components carrying gravity load -carrying components was caused primarily by wind or seismic effects, then the building shall be evaluated in accordance with Section 405.2.3.1 and, if noncompliant, retrofitted in accordance with Section 405.2.3.3 405.2.5 Substantial structural damage to snow load-carrying components.
Where substantial structural damage to any horizontal components carrying snow load -carrying components is caused by or related to snow load effects, any components required to carry snow loads on roof framing of similar construction shall be repaired, replaced or retrofitted to satisfy the requirements of Section 1608 of the International Building Code .
Clarifying Language Reason Statement: For 405.2.4 and 405.2.4.1, reference to vertical components are added to be consistent with the SSD definition. Otherwise, it might be interpreted that this provision applies when there is damage only to horizontal components. For 405.2.5, reference to horizontal components are added to be consistent with the SSD definition. Otherwise, it might be interpreted that this provision applies when there is damage only to vertical components. In the code change process, undamaged components carrying load from retrofitted components was not introduced in section 405.2.5.
Some have questioned the term “of similar construction” and how it applies. Two distinct framing conditions are shown in Figure 5. The area in green is at a drifting snow zone where the rafters are more closely spaced, and the supporting girders are larger than at other roof areas. This would be considered an area of similar construction and within that area, two components of similar construction would be the rafters and the girders. If more than 30% of the green rafters are damaged, the remaining 70% of the undamaged green ones would have to be replaced or strengthened
if they are not IBC compliant, since they would be considered one area of similar construction. If more than 30% of the green girders of similar size are damaged, then they would have to be replaced or strengthened if they are not IBC compliant, since they would be considered other areas of similar construction. The same applies to the rafters shown in red in Figure 5, which support a uniform flat roof snow load. If 30% of the red rafters are damaged, the remaining 70% of the undamaged red ones would have to be replaced or strengthened if they are not IBC compliant since they would be considered another area of similar construction. Similarly, if more than 30% of the red girders of similar size are damaged, then they would have to be replaced or strengthened if they are not IBC compliant since they would be considered yet other areas of similar construction. For the girders in both areas, those carrying loads from two sides could be considered an area of similar construction while those carrying only load from one side would be considered different areas of similar construction. On this basis, the practitioner should use their discretion for determining areas of similar construction based on the simplicity or complexity of the roof being assessed.
The IEBC is a relatively new code and new to many practitioners. The code, written as a national standard, has seen many changes in its short history addressing concerns from local jurisdictions throughout the country. With the changes may come confusion in the short three-year code cycle. Code confusion regarding SSD and less than SSD was brought to the attention of the NCSEA EBC from practicing structural engineers from around the country. The NCSEA EBC who initially introduced SSD for snow damage and how to address repairs when there is snow damage for less than SSD, took a deep dive into reviewing these provisions. This article addresses the code confusion and introduces clarifying language addressing the intent for which these provisions were written.
Michael Fillion, PE, M. ASCE is President of Fillion Group, Inc. Structural Engineers and is Past Chair of NCSEA’s Existing Buildings Sub-Committee, a member of the Structural Advisory Committee to the Massachusetts State Building Code, a participant at the ICC Code hearings and a committee member of ASCE 11-28 Structural Condition Assessment of Existing Buildings. He is also Chair of the SEAMass Structural Engineering Emergency Response Committee and a Cal OES SAP & ICC When Disaster Strikes Trainer. (mrf.structure@verizon.net)
To kick off its 103rd annual meeting in Chicago, NCEES hosted a ceremonial event to cel ebrate the signing of its mutual recognition agreement (MRA) with the Engineering Council–UK.
NCEES President Laura Sievers, PE, and Chief Executive Officer David Cox joined Engineering Council–UK Chair John Chudley and International Affairs Manager Dave Clark to formally sign the agreement, which pro vides a more direct path for licensed engineers to practice in each country without compro mising professional standards. Almost 30 NCEES member licensing boards were present to signify their intent to participate in the agreement.
Built upon the framework of the International Professional Engineers Agreement (IPEA), the MRA upholds disciplinespecific and jurisdictional requirements for those seeking to practice internationally.
in participating states to more easily obtain the CEng title and expanded professional mobility to work in the United Kingdom.
“The world is shrinking every day, and advancements in technology have made it possible for us to communicate
explained NCEES CEO Cox. “The MRA and its increased opportunities for licensed engineers to practice in the United Kingdom, much like the mobility we have between the states, is a great example of that.”
Professional engineers in the
Kingdom through this agreement must be a member of the NCEES International Registry for Professional Engineers (IRPE). This program assists U.S.-based professional engineers who are seeking recognition to practice in another country.
The Ohio Department of Transportation has hired Woolpert for two separate engineering contracts to support bridge maintenance, repair, and replacement projects throughout the state.
The first contract supports ODOT District 9’s bridge replacement projects on State Route 772 in a rural area of Huntington Township in Ross
County and State Route 138 near Clarksburg, Ohio. Woolpert will provide hydrology and hydraulics analyses, bridge structure type studies, conceptual maintenance of traffic (MOT) planning, and floodplain notification services.
Under the second contract, Woolpert will provide field condition surveys, testing, MOT planning, and condition evaluation reports for District 12 to
support maintenance repairs on 11 bridges along U.S. Route 422 in Cuyahoga and Geauga counties.
Woolpert Bridge Engineer and Project Manager Tom Less said these projects are proactive to prevent aging bridges from reaching a hazardous state.
“ODOT is committed to ensuring that Ohio’s infrastructure is safe, maintained, and
well-positioned for the future, and these contracts will help support that mission,” Less said.
“While Woolpert has expanded its presence and services throughout the U.S. and world, we are always incredibly proud to continue our support for such a long-term, valued client and state that many of us here at Woolpert call home.”
The contracts are underway.
Salas O’Brien is excited to announce that its structural engineering team was recognized by the Cold-Formed Steel Engineers Institute (CFSEI) with two honorable mentions at its Design Excellence Awards for the cold-formed steel (CFS) designs of the Colorado Convention Center Expansion and Denver International Airport (DIA) Concourse B & C Expansions, both in Denver, Colorado. Salas O’Brien received the awards during CFSEI’s annual expo and conference in Tucson, Arizona. CFSEI is a national organization comprised of structural engineers and other design professionals. Its annual Design Excellence Awards program recognizes outstanding achievement in creative design, technical innovation, and overall project excellence in the use of CFS, according to the CFSEI website.
Colorado Convention Center Expansion
Already one of the largest buildings in Denver, the rooftop expansion of the Colorado Convention Center added 135,000 square feet to this premier venue. The Salas O’Brien project team, led by Senior Principal Russ Leffler, PE, SE, and Vice President Daniel Stadig, PE, provided exterior structural CFS framing engineering and panelized exterior walls for the 20,000-square-foot outdoor rooftop terrace. They are up to 50 feet tall, including an eight-foot-tall parapet over a 40-foot-long clear span hanging from a new structure above the existing roof. They were also carefully designed to meet the tight restrictions of the construction schedule and downtown site location.
The team also provided interior CFS wall and ceiling
framing engineering for the 80,000-square-foot columnfree Bluebird Ballroom and 35,000-square-foot wraparound pre-function concourse. These areas included challenging elements requiring extensive engineering, including large, sloped ceiling elements in the pre-function concourse area to match the blade roof and soffits that supported 30-foot span ceiling joists.
DIA Concourse B & C Expansions
large expansion project for two of its three concourses, increasing Concourses B and C by 126,000 square feet and 530,000 square feet, respectively. The Salas O’Brien project team, led by Senior Principal Russ Leffler, PE, SE, and Vice President Daniel Stadig, PE, undertook the challenge of designing various CFS and CFS/steel hybrid panels and incorporating the two systems into one panelization process. The hybrid panel design allowed the client to receive the steel members directly from the supplier for insertion into the panels and
off-site due to space constraints at the project location.
The off-site fabrication component also had challenges, including coordinating the structural steel within the CFS panels to allow for reasonable panel sizes and execution and designing the panels with special structural considerations for loads incurred during transportation to and installation at the project site. Salas O’Brien’s expertise, combined with the firm’s extensive DIA project history, minimized the impact on airport operations and allowed
The reconstruction of the heritage-protected House of Culture of the city of Ostrava and the extension of the concert hall continue in the Czech Republic community. The
Lockwood, Andrews & Newnam, Inc. (LAN) announces the completion of the new parking structure at the Galveston Ferry Landing. The ribbon-cutting ceremony took place June 27, marking an important milestone in enhancing transportation infrastructure and community convenience in Galveston, Texas, in partnership with the Texas Department of Transportation (TxDOT).
The need for this new parking structure arose from persistent parking congestion during peak summer months. The influx of visitors enjoying the ferry crossing between Galveston Island and the Bolivar Peninsula increased the demand for parking spaces.
The new facility now accommodates 120 passenger cars and 11 motorcycles and will be fully operational just in time for peak tourist season.
Construction of the parking deck began in October 2022, following the issuance of bid and construction documents in May 2022.
"We are excited to see this project come to fruition," said Steve Gilbreath, PE, chief operating officer and vice president at LAN.
"The new parking structure addresses parking issues and demonstrates our commitment to supporting Galveston’s infrastructure development."
G
lobal engineering services leader DeSimone Consulting Engineering announced that a team led by Associate Dr. Jeffrey Dragovich, PhD, PE, SE, F.ACI, Director of the company’s Applied Technology and Research group, has won the Belgium-based UCLouvain 2023 Blind Prediction Competition.
The team received recognition for their winning entry at the 18th World Conference on Earthquake Engineering in Milan, Italy, where 4,000 members of the world’s international scientific and engineering communities gathered last month to share research, technology, and risk mitigation commitments. The DeSimone-led team presented their modeling and analysis approach during the session, “Reinforced Concrete (RC) Structural Walls: Advances and Future Challenges for Design, Modelling, Testing, and Construction.”
“As we strive towards progressively more reliable analytical methods to predict earthquake response of structures, our work shows clear progress is being made,” said Dr. Dragovich. “In exploring new materials and methods that can improve wall performance and advance the state-of-practice, competitions such as this help us better understand and simulate the behavior of concrete walls using large-scale testing.”
The UCLouvain 2023 Blind Prediction Competition was open to three categories of participants: practicing engineers, researchers, and students. The results of the blind prediction competition will promote good seismic design practices for RC walls. The shake table tests and participant predictions can help improve the assessment of RC wall buildings and provide directions for future building code revisions.
NRMCA Selected for EPA Grant Aimed at Reducing Embodied Greenhouse Gas Emissions
The National Ready Mixed Concrete Association (NRMCA) announce that the U.S. Environmental Protection Agency (EPA) has selected the association for a $9.63 million grant aimed at “Reducing Embodied Greenhouse Gas Emissions in Construction Materials and Products.” The grant will fund a five-year project managed by NRMCA and supported by its Build With Strength initiative which includes a team of concrete experts to educate the architectural and engineering communities on designing and specifying low carbon concrete.
To support the federal government’s goal
More broadly, this competition helps promote a more robust building stock and strengthen community resilience internationally.
Dr. Dragovich led a team that included Dr. Beyazit Aydin, Dr. Bulent N. Alemdar, and Seth Guthrie from software development company Bentley Systems Inc.; Prof. Kristijan Kolozvari from California State University at Fullerton; Prof. Andrés Lepage from The University of Kansas, and; Dr. Saman Abdullah from University of Sulaimani, Kurdistan Region, Iraq. The engineers used two different software programs to predict how a 40-ton reinforced concrete (RC) U-shaped wall would respond in a simulated earthquake. The tests were conducted at LNEC using a dynamic shake table, also known as an earthquake simulator.
“We are thrilled to see our team’s work in the field of seismic engineering and software development gain international recognition,” said Stephen DeSimone, President and CEO of DeSimone Consulting Engineering. “Over the past decade, significant advancements have been made in modeling approaches that predict how concrete walls will respond under different conditions. The work of our applied technology and research group is ensuring that DeSimone continues to stand at the forefront of designing structures that can better withstand extreme wind forces and earthquakes.”
Team DeSimone used the ADINA and OpenSees nonlinear finite element analysis programs for their analytical predictions. While both models were effective at predicting the response, the ADINA model prepared by their team partners at Bentley Systems was the winning entry in the competition.
of reaching net zero emissions in procurement by the year 2050, the EPA initiated this new grant program through the Inflation Reduction Act of 2022. This project will help NRMCA accelerate its goal to cut the carbon footprint of concrete in half by 2028, using 2014 as a baseline, and to attain carbon neutrality by 2045, five years ahead of its original goal.
Rudolph P. Frizzi, PE, G.E., BC.GE, managing principal, LANGAN, is the recipient of DFI’s highest award bestowed to an individual, the Distinguished Service Award (DSA). This award recognizes individuals who have made exceptionally valuable contributions
to the advancement of the deep foundations industry. The award is being presented at the DFI 49th Annual Conference on Deep Foundations, being held October 7–10 in Aurora, Colorado.
With nearly 40 years in the industry, Frizzi has interacted on projects located in practically every continent worldwide, and has performed geotechnical investigations and designs, prepared plans and technical specifications, and observed field testing and construction as geotechnical “engineer of record” on countless projects. In addition, Frizzi has provided expert geotechnical consultation and trial testimony on over 100 excavation and foundation litigation cases. He has also prepared and presented over 50 technical presentations at universities, conferences and seminars.
The SE 2050 initiative is advancing structural engineering towards sustainability through three essential components: a comprehensive database, proactive policy advocacy, and the ECaps framework —Embodied Carbon Action Plans. At the heart of this effort is the SE 2050 database, currently in its beta phase, where industry signatories contribute project data. This repository serves as a foundational resource for understanding and advancing embodied carbon reduction strategies. Accessible exclusively to SE 2050 signatories, the database facilitates collective learning and informed decision-making, enabling stakeholders to benchmark performance and drive continuous improvement. Vice chair Frances Yang, S.E., emphasizes the significance of this resource, stating, “With the additional feature of collecting structural material quantities, we’ll start to evaluate projects for not just the total embodied carbon, but also the efficient use of materials.”
Policy advocacy forms another cornerstone of SE 2050’s strategy, influencing regulatory frameworks to embed embodied carbon considerations into global building codes and standards. By collaborating with policymakers, industry groups, and advocacy organizations, SE 2050 seeks to create an environment that incentivizes sustainable design practices and accelerates their adoption across the industry.
Luke Lombardi, A.M. ASCE highlights the importance of such efforts, noting, “In order to drive a shift in policy, we need industry leaders to show that we can already do this.”
The ECaps framework guides signatories in setting ambitious carbon reduction targets informed by data insights from the SE 2050 database. These plans empower participants to optimize material use and lifecycle assessments, fostering transparency and accountability in their sustainability efforts. SE 2050’s influence is exemplified in initiatives like California’s Green Embodied Carbon Policy, the first statewide policy tackling embodied carbon with holistic building lifecycle analysis. Such policies validate and accelerate SE 2050’s mission, demonstrating the feasibility and impact of integrating sustainability into structural engineering practices.
With multitudes of projects logged and growing industry engagement, SE 2050 sets a precedent for sustainable structural engineering practices that ensure a resilient and environmentally responsible built environment for future generations. Through pioneering data-driven solutions and advocating for transformative policy changes, SE 2050 is driving a systemic shift towards net-zero structures by 2050, ensuring a sustainable legacy for generations to come.
Learn more at SE2050.org
The errata is in effect as of July 2024. Visit ascelibrary.org to review changes to the standard and Supplement 1 for accurate interpretation and application.
The American National Standards Institute recently announced Jennifer Goupil, SEI managing director, as the recipient of the 2024 Leadership and Service Award for her tireless efforts and leadership on standards including ASCE 7 and ASCE 41. Congratulations to Goupil on this well-deserved recognition!
• Advance and be recognized as an SEI Fellow - Must be current SEI member, actively involved in SEI, 10 years responsible charge (typically post P.E./S.E.) www.asce.org/ SEIMembership
• O.H. Ammann Research Fellowship in Structural Design and Construction
• SEI and ASCE Structural Awards www.asce.org/SEIAwards.
• April 10—The Future of Design: Panel on innovative approaches to reduce carbon emissions from the built environment. Moderated by Marc Hoit, with industry leaders Dave Bennink, Don Davies, and Nancy Novak.
• April 11—Building Community: Putting People First in an Era of Environmental Extremes. Professor Lori Peek on focusing on the human impact of disasters and the importance of designing for resilience.
SEI welcomes the recently elected governors, shown in italics. Terms begin on October 1. SEI also thanks two board members who will finish their terms on September 30: Donald R. Scott, P.E., S.E., F.SEI, F.ASCE, SEI Past President, and J. Greg Soules, Ph.D., P.E., P.Eng, S.E., F.SEI, F.ASCE.
Hamid R. Adib, Ph.D., P.E., F.SEI, F.ASCE, SEI Treasurer
Michael J. Bolduc, P.E., S.E., M.ASCE
Maria M. Garlock, Ph.D., P.E., F.SEI, M.ASCE
Edwin T. Huston, P.E., S.E., F.SEI, M.ASCE, SEI President-elect
Robin A. Kemper, P.E., LEED AP, ENV SP, F.SEI, Pres.19.ASCE
Chad M. Schrand, P.E., F.SEI, M.ASCE
Kenneth L. Sharpless, P.E., F.SEI, F.ASCE
Stephanie L. Slocum, P.E., M.ASCE, SEI President
Elaina J. Sutley, Ph.D., P.E., M.ASCE
James P. Wacker, P.E., M.ASCE
Shuxian (Susanne) Wassenius, P.E., F.SEI, M.ASCE
The 2024 CASE Summer Meeting at ACEC Minnesota brought together structural engineers for two days of focused sessions, committee meetings, and valuable networking opportunities. This event emphasized the importance of collaboration and knowledge-sharing in advancing the profession.
Day one featured sub-committee meetings for the Toolkit, Programs & Communications, Contracts, and Guidelines Committees, where members worked on refining tools and strategies crucial to the industry’s future. These sessions culminated in a collective report-out during lunch, fostering transparency and collaboration across different focus areas.
A highlight of the event was the presentation on the Minnesota Zoo Treetop Trail, an innovative project transforming a decommissioned monorail into an elevated pedestrian walkway. Led by Murphy Curran of MBJ Engineers and Thomas Root of the Minnesota Zoo, the session showcased the challenges and solutions involved in repurposing infrastructure while keeping zoo operations uninterrupted.
The CASE Executive Committee (ExCom) meeting followed, open to all committee members, providing a platform to discuss strategic initiatives that ensure CASE remains at the forefront of professional standards.
The event also included a networking cocktail reception, where attendees connected with peers and industry experts. This informal setting was an ideal opportunity to exchange insights, discuss projects, and build relationships that could lead to future collaborations.
The second day began with a session on Ownership and Compensation Philosophies, offering strategies for aligning business goals with ownership and compensation structures. The final session on the Engineering Joint Contracts Documents Committee (EJCDC) provided practical tips for customizing engineering agreements, emphasizing the role of standardized practices in improving project outcomes.
The 2024 CASE Summer Meeting provided participants with new insights, stronger professional connections, and a renewed commitment to advancing the structural engineering profession. The event highlighted the benefits of collaboration and networking in driving innovation and maintaining high industry standards.
ACEC’s Coalition of American Structural Engineers (CASE) is home to four active committees, each dedicated to advancing the interests and expertise of structural engineers. These committees are responsible for developing education, publications, and other valuable content that supports ACEC’s Structural Engineering firm members. The four committees are:
1. Contracts Committee: Focuses on developing and updating standardized contract documents tailored to the needs of structural engineers.
2. Guidelines Committee: Works on creating and refining practice guidelines that enhance the quality and consistency of structural engineering services.
3. Risk Management Committee: Concentrates on strategies and tools that help structural engineers manage and mitigate professional risks.
4. Programs and Publications Committee: Oversees the creation of educational programs, publications, and other resources that contribute to the professional development of structural engineers. Committee members commit to a term of 2-4 years, with an option for renewal. Meetings are held monthly online, with in-person gatherings twice a year, for which travel expenses are covered. Serving on a committee offers a unique opportunity to expand your expertise, gain recognition as a thought leader, and network with esteemed professionals in the field.
If you are a CASE member interested in contributing to the advancement of the structural engineering profession, consider joining one of these committees. Your participation will not only benefit your career but also provide valuable resources to the broader ACEC community.
The CASE Coalition is committed to fostering the next generation of engineering leaders, which is why we are proud to announce the awarding of a $6,500 scholarship to Allyson McGuire, a second year Master’s student from George Mason University. This scholarship, provided in partnership with the ACEC Research Institute, is a testament to our dedication to supporting the academic and
professional development of future engineers.
McGuire will be recognized for this achievement at ACEC’s Fall Conference in New Orleans this October. With the contributions of students like McGuire on the horizon, CASE is confident that the future of the engineering industry is exceptionally bright. Congrats to McGuire!
Recently, Tricia Ruby and Ruby+Associates, a Degenkolb Company, presented the NCSEA Foundation with a $50,000 donation to establish the David I. Ruby Endowed Scholarship, a permanent endowed scholarship to financially support a historically underrepresented student in structural engineering who is interested in structural steel. This scholarship will provide recipients with funds to help with tuition, fees, and other mandatory education costs.
“It’s a privilege to honor my father’s legacy in structural engineering and passion for steel construction through this endowed scholarship. He has been a leader in the profession throughout his career with a particular love for mentoring the next generation. To be able to align this gift with a personal mission of supporting underrepresented students is a dream realized,” said Tricia Ruby, NCSEA Foundation Board of Directors President.
The scholarship honors the 60-year career of David I. Ruby, PE, SE founder of Ruby+Associates, a structural engineering firm dedicated to serving the steel construction industry. Dave dedicated much of his time to improving the profession. He is a past Director of NCSEA, a founding member and past President of Structural Engineering
Association of Michigan (SEAMi), former Chairman of Coalition of American Structural Engineers (CASE), former member of the AISC Code of Standard Practice, Committee on Research, and Co-Chair of the Blast & Impact Resistant Design Task Group. He received the 2011 AISC Lifetime Achievement Award, and the 2022 AISC J. Lloyd Kimbrough Award. He is also the author of AISC Design Guide #23, “Constructability of Structural Steel Buildings.”
The NCSEA Diversity in Structural Engineering Scholarship program was established by the NCSEA Foundation in 2021 to award funding to students who have been historically underrecognized in structural engineering (including but not limited to Black/African American, Native/Indigenous American, Hispanic/Latino or Spanish, Asian, Native Hawaiian or Pacific Islander, and other people of color, those with disabilities, veterans, and LGBTQIA+). Multiple scholarships are presented annually to junior college students, undergraduate students, and/or graduate students pursuing degrees in structural engineering.
To learn more about the NCSEA Foundation or to donate, please visit www.ncsea.com/about/foundation/ or contact Al Spada, Executive Director, at (312) 649-4600 Ext. 201 or aspada@ncsea.com.
NCSEA is pleased to announce a strategic partnership with WTW Architects & Engineers (WTW A&E) to provide the structural engineering profession with tailored risk management education and training. This initiative aims to mitigate risks related to costly claims and client disputes, enhancing the professional practice of structural engineers across the United States.
WTW A&E is dedicated exclusively to providing insurance and risk management solutions to design professionals across North America. Recognized for its leadership in delivering high-quality risk management education, WTW A&E will collaborate closely with NCSEA to develop customized risk management programs for structural engineers, including on-demand education programs
and special webinars. WTW A&E will also present at upcoming structural engineering conferences, including the NCSEA Structural Engineering Summit.
The goal of this partnership is to improve structural engineering practices by sharing lessons learned from actual claims and nearmiss experiences. The education program will feature interactive webinars, live presentations, and articles in STRUCTURE magazine.
Alfred Spada, Executive Director of NCSEA, stated, “We are proud to partner with WTW A&E to provide our members with invaluable resources aimed at reducing professional liability claims. This collaboration underscores our commitment to supporting structural engineers in their professional development and success.”
Ever wanted to get more involved with NCSEA? Now’s your chance! Participation of SEA members in NCSEA committees is highly valued. Committee chairs are always looking for additional passionate volunteers to get involved.
SEA members can apply for committee positions throughout the year using our Volunteer Application, available online at www.ncsea. com/engage/committees. Most committees welcome new members on a rolling basis, while others add members once per year. Once
submitted, your application will be reviewed to confirm Member Organization/SEA membership and forwarded to the committee chair(s) for consideration. You can expect a response within 30 days.
Being an NCSEA Committee Member is a fantastic opportunity to collaborate with fellow professionals, share your knowledge, and make a meaningful impact in the structural engineering community. To qualify, you must be a member of an SEA or an NCSEA Corporate Member company.
In an era of rapid technological advancements and reduced human interaction, learning to take smart risks is more crucial than ever. At this year’s NCSEA Structural Engineering Summit, Henna Pryor will guide structural engineers in harnessing these essential skills.
Pryor, a renowned workplace performance expert and award-winning 2x TEDx speaker, will present her keynote, “Awkward and Upward! Your Surprising Secret Weapon for Taking More Professional Risks,” on Thursday, November 7, 2024, at the MGM Grand Hotel in Las Vegas.
Pryor’s keynote will delve into how our reliance on digital conveniences is weakening our social muscles, making it harder to build trust, collaborate, and take calculated risks at work. She will share strategies for overcoming the discomfort associated with risk-taking and using deliberate discomfort to strengthen our social and professional skills. Attendees will leave with a customized plan to speak up, share ideas, and embrace courageous actions in their careers.
Known for her dynamic, no-nonsense approach, Pryor has helped high achievers reach new levels of success. She has worked with top organizations such as Google, Workday, FIS Global, and Johnson &
Johnson, and her insights have been featured in Forbes, Real Simple, and Fast Company. Pryor founded Pryority Group to extend her transformational coaching and performance growth workshops beyond the C-Suite.
Join Henna Pryor and other thought-leaders at the NCSEA Structural Engineering Summit, taking place November 5-8, 2024. This is a prime opportunity to gain invaluable insights into the latest trends and innovations in structural engineering. Register now to secure your spot and take advantage of educational sessions, insightful keynotes, engaging exhibits, valuable networking opportunities, and more—all specifically tailored for structural engineers.
The Structural Engineers Association of Colorado (SEAC) Wind Committee is working on a revised wind speed study to accurately define the Colorado Special Wind Region (SWR). The existing data is based on an outdated wind study, and it lacks coverage for areas south of Castle Rock, Colo. The new study aims to update the Front Range Wind Speed map with modern design approaches and extend coverage to major population centers like Colorado Springs.
The estimated cost of the large and impactful study is around $200,000. SEAC has applied for FEMA Building Resilient
Infrastructure and Communities (BRIC) funding to cover up to 75% of this amount. SEAC has pledged to raise the remaining $50,000, with an initial $5,000 pledge from the SEAC board and the NCSEA Foundation’s SEA Grant Program.
“Fundraising is a challenging endeavor and having the financial support from NCSEA at start the fundraising helped show the importance of this effort and has increased the likelihood of getting this study fully funded,” said SEAC Vice President and Treasurer Jordan Jarrett, Ph.D, PE.
www.ncsea.com/education for the latest news on upcoming webinars and other virtual events.
ever need! Subscribers receive access to a full year’s worth of live NCSEA education webinars (25+) and a recorded library of past webinars (170+) – all developed by leading experts; available whenever, wherever you need them!
While some Member Organizations (MOs) have longstanding relationships with the Colleges and Universities within their territories, others have yet to make strong connections.
By Scott Francis, PE, and Chad Mitchell, PE, SE
Engineering firms are the ultimate consumer of the product that academia produces—new graduates—and as such have a vested interest in the effectiveness of their academic training. NCSEA Member Organizations (MOs) throughout the country are in a unique position to team with educators to enhance the education of structural engineering and architectural engineering students. Educators are often looking for opportunities to expose students to the practice of structural engineering and to bring “real world” engineering projects into the classroom. What are ways that MOs can be involved with the programs that are producing these new graduates? As members of the Academia-Practice Partnerships (APP) Committee, we are often asked how MOs can become more involved with the colleges and universities located within their regions. Both academia and practitioners have a vested interest in the outcome of new graduates as they transition from students to EITs and beyond. One of the APP Committee’s initiatives is to foster college student and practitioner engagement. This has included efforts to learn more about what successful interactions between academia and practitioners look like. While many of these ideas are conventional, hopefully one or two of them may spark an interest in you and/or your MO that can lead to increased connectivity.
During a recent poll of Member Organizations, we documented the following ways that MOs throughout the country have engaged with students and colleges/universities:
Time/Expertise
• Host MO-wide career fairs
• Hold Joint Design Competitions
• Serve as student advisors on capstone projects
• Develop specific programs targeting students and early career professionals. One such program is SEAOC’s SE Pathways to the Profession which focuses on engagement, retention, and equity for students & early career professionals.
• Provide speakers (and often food) for Student Chapter Meetings
• Host panel discussions on various topics in engineering
• Lead discussions on licensure for practicing engineers
• Provide opportunities to shadow practitioners
• Host speed mentoring sessions with students
• Participate in resume workshops for students
• Host an Industry Day
• Serve as guest speakers at colleges/universities
• Host jobsite visits for classes
Financial
• Sponsor NCSEA student chapters on campus
• Pay for students to attend state/national conferences
• Cover dues for students and faculty to join their local MO and/ or NCSEA
• Provide internships
If any of these ideas look like something that you would like to pursue, your first question may be how do we go about setting something like this up? First, you can reach out directly to the college or university. If you don’t have an established point of contact with one already, we suggest checking out that school’s website. Often with a little digging, it is possible to find a list of professors along with their areas of interest. At the APP, we usually look for professors who teach primarily structural courses to serve as our first point of contact. If this approach isn’t working, we note that MOs can reach out to the APP. As part of our curriculum survey, we have collected points of contact with most of the over 300 ABET accredited institutions in the U.S., and we are happy to share this information with MOs looking to make connections. Once contact is made, discussions can begin by asking your point of contact what support they need from practitioners to enhance their students' education experience. Remember, while you may have suggestions and ideas that you are excited about, this is about developing a long-term relationship with these programs and the needs of the program may necessitate putting your interests on hold while these first needs are addressed. Another path to connection with colleges and universities is to start a student chapter. Additional resources for this can be found on the Young Member Support Page of the NCSEA website www.ncsea.com/ engage/committees/young-member-support/. At the bottom of the webpage is a link to the Student Chapter Outreach Guide. Ultimately, it is in all our best interests to foster strong relationships with the colleges and universities within our territories in order to have a positive impact on the education of future structural/architectural engineers. One of the best ways to do this is to engage with schools to find out how we can enhance students’ experience and learning outcomes. These interactions are often very rewarding for the students as well as the practitioners. Investing time and resources into today’s students (and sometimes pizza money) will lead to better student outcomes and strengthen the future of our profession.
Scott M. Francis, PE, is a Vice President at Lynch Mykins Structural Engineers, PC, a structural firm located in the Southeast (sfrancis@lynchmykins.com)
Chad S. Mitchell, PE, SE, is an Associate Principal and Department Manager with S.A. Miro Inc a civil and structural firm located in the Rocky Mountain Region. (cmitchell@samiro.com)