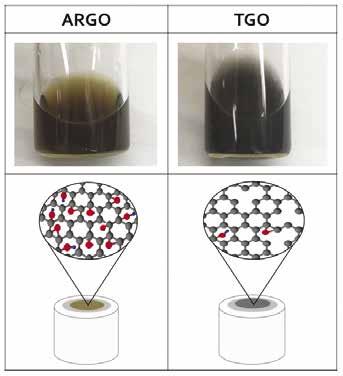
12 minute read
The role of oxygen functional groups in graphene oxide modified glassy carbon
The role of oxygen functional groups in graphene oxide modified glassy carbon electrodes for the electrochemical sensing of nicotinamide adenine dinucleotide hydride
Ananya Mukherjee a , Yan Wang a , and Leanne Gilbertson a, a Environmental Engineering Laboratory, Department of Civil and Environmental Engineering, University of Pittsburgh, PA 15260, USA
Ananya Mukherjee is junior year environmental engineering student at the University of Pittsburgh. Inspired by the wetland ecosystems of her hometown in Princeton, NJ, she hopes to pursue a career related to sustainability and environmental design.
Ananya Mukherjee
Yan Wang is a PhD candidate in the Department of Civil and Environmental Engineering at the University of Pittsburgh. She obtained her bachelor’s degree at Sun Yat-sen University and master’s degree at Zhejiang University in China. Her current research interests are related to sustainable design of graphene-based nanomaterials for advanced energy, environmental, and biological applications.
Yan Wang
Leanne Gilbertson is an Assistant Professor of Environmental and Sustainable Engineering. She joined the faculty at Swanson School in the fall of 2015 after completing her postdoc and PhD, both at Yale University Department of Chemical and Environmental Engineering. Her research group focuses on the sustainable design of emerging materials and products for applications at the nexus of the environment and public health.
Leanne Gilbertson
Significance Statement
Epoxides on graphene oxide (GO) modified glassy carbon electrodes (GCEs) appear to be more electrochemically active than other functional groups in the oxidation of nicotinamide adenine dinucleotide hydride (NADH). This suggests that better NADH sensors can be designed by incorporating GO engineered with high epoxide content.
Category: Device design Keywords: NADH, graphene oxide, glassy carbon electrodes, oxygen functional groups Abbreviations: Nicotinamide adenine dinucleotide hydride (NADH), nicotinamide adenine dinucleotide (NAD+), graphene oxide (GO), as received graphene oxide (ARGO), thermally annealed graphene oxide (TGO), carbon nanotubes (CNTs), cyclic voltammogram (CV)
Abstract
Nicotinamide adenine dinucleotide hydride (NADH) plays an important role in cellular metabolizing processes that generate energy. NADH levels can be indicative of cellular health so researchers are interested in developing better sensors for monitoring NADH. Electrochemical sensors are economically advantageous, but the irreversible oxidation process results in accumulation of nicotinamide adenine dinucleotide (NAD+) at the electrode surface. This leads to electrode fouling that inhibits further oxidation. Graphene oxide (GO) is a popular nanomaterial often used for modifying electrode surfaces to speed up electron transfer and prevent fouling. This research was focused on identifying what oxygen groups on GO are responsible for the enhanced sensing of NADH. Glassy carbon electrodes (GCEs) were modified with thermally annealed GO (TGO) and as received GO (ARGO). ARGO was found to cause faster oxidation in NADH and differed in composition from TGO mainly in its high percentage of epoxide moieties. This indicates that epoxide groups on GO may facilitate electron transfer in NADH. Should further research confirm these findings, more effective sensors could be designed by incorporating GO with high epoxide content.
1. Introduction 1.1 Medical Relevance
The oxidation of NADH to NAD+ plays an important role in cellular metabolizing processes that generate energy in living cells. NADH is essential for mitochondrial respiration to produce ATP, as well as for over 200 other enzymatic reactions. [1] The levels of both NADH and NAD+ can be indicative of cellular health. In a study looking at NAD+ levels in patients with multiple sclerosis (MS) it was found that NAD+ levels declined as the diseases progressed. [2] Healthy controls had the highest NAD+ levels, followed by patients with relapsing remitting MS, secondary progressive MS, and primary progressive MS. The study concluded that reduced NAD+ levels indicate a loss of cellular function and metabolism which culminate in cell death.
NAD+ naturally declines in animals and in humans during normal aging. It has been shown that enhancing NAD+ levels in mammals can improve mitochondrial function under cellular stress. This leads to improved protection against dietary and age-related metabolic complications. There is currently a lot of interest in researching more about NADH/NAD+ for possible therapeutic uses in treating a variety of diseases as well as improving overall health in elderly people. [3] 1.2 Graphene Modified Electrochemical Sensors
Understandably, a lot of interest has been shown in developing sensors to better monitor NADH levels as they can be indicative of cellular health. Electrochemical sensing has been of particular interest to researchers because it is more cost effective, user friendly, and works with smaller sampling volumes than other sensing methods. [4] The problem with using electrochemical sensors is that NADH has sluggish electron transfer rates which leads to build up of the oxidation product NAD+. Fouling then occurs as NAD+ adsorbs onto the electrode surface and blocks electron transfer sites, hindering further oxidation. One method to speed up the reaction and reduce fouling is by modifying the electrode surface. GO is a popular nanomaterial often used for electrode modification because of its unique electrochemical properties. Graphene’s honeycomb lattice of carbon atoms has delocalized pi orbitals that allow electrons to move freely above and below the plane, making it extremely conductive. GO has many oxygen groups that provide abundant electron transfer sites which help to speed up oxidation. Zhang et al. has suggested that NADH oxidation is mediated by abundant oxygen groups at edge planes in GO. [5] However, there has yet to be research focused on identifying which oxygen groups are responsible for the enhanced oxidation at GO modified GCEs. The goal of this research is to inform better sensor design by identifying which functional groups in GO mediate electron transfer in NADH. Once active groups are identified, sensors can be optimized by modifying GO’s material properties to incorporate higher distributions of oxidation mediating moieties. It has been reported that NADH oxidation at ordered carbon nanotube (CNT) modified electrodes is mediated by quinone moieties. [6] Because CNTs are similar to GO in composition, we hypothesize that quinones mediate oxidation in GO as well. 1.3 Approach
In this experiment ARGO and TGO dispersions will be drop casted in order to produce modified GCEs. The electrodes will be placed in solutions of known NADH concentration. CVs will be obtained by monitoring the current in response to an applied potential that steadily increases (anodic cycle) then decreases back to the initial value (cathodic cycle). When NADH is near the modified electrode surface, the current will increase indicating that it is being oxidized. The potential at which the current reaches a maximum value (peak potential) will be used to characterize the speed of the oxidation at either surface. A peak in the CV curve indicates that the majority of NADH near the electrode surface is oxidized. The magnitude of the peak will indicate how many NADH
molecules have oxidized, but what this research is interested in is the voltage at which the peak occurs. A fast reaction will be indicated by a peak that is generated early in the anodic cycle at a low potential i.e. a more negative peak potential indicates better electron transfer (Figure 1). The peak potentials of ARGO and TGO will be analyzed in conjunction with the surface group compositions of both materials (obtained by Wang et al. [7]) in order to determine what functional groups are electrochemically active.
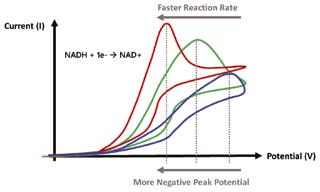
Figure 1: Typical CVs of reactions occurring at different rates and corresponding peak potentials
2. Methods 2.1 Experimental Procedure
Graphene ink dispersions were prepared using 2 mg of either TGO powder annealed at 600°C, or ARGO powder. 1.2 mL of DI water was added to the powder, followed by 792 μL isopropanol to aid with dispersion and 8 μL of nafion perfluorinated resin to act as a binding agent to the electrode surface, giving a concentration of 1 mg/mL. Both dispersions were probe sonicated at 10% power (400 W max power output) three times in 5 minute segments and cooled down in an icebath for 2-3 minutes in between rounds. Afterwards, both samples were placed in a bath sonicator (135 W) for 1 hour, resulting in well dispersed solutions. The ARGO and TGO dispersions are shown below (figure 2). Abundant oxygen groups were responsible for the brown color in the ARGO dispersion. On the other hand, TGO had most of its oxygen groups annealed off, resulting in a gray dispersion.
Figure 2: ARGO/TGO dipersions (top) and ARGO/TGO modified electrodes showing molecular depictions of oxygen content (bottom)
GCEs were polished by rubbing 0.05 μM alumina slurry on microfiber cloth in a circular motion for 1-2 minutes. Electrodes were then cleaned off with isopropanol and then DI water. 10 μL of each dispersion was dropcasted onto a GCE and air dried.
A 0.1 M phosphate buffer solution (PBS) at pH 7 was used for the electrolyte. The PBS was prepared by pouring 34.8 mL of 1 M K2HPO4 and 15.2 mL of KH2PO4 into a 500 mL flask and then filling the rest with DI water. 76 mL of the prepared PBS solution was used in each electrochemical cell and purged with Nitrogen gas for about 20 minutes. Afterwards the cell was cleaned by conducting a CV scan from -0.4 V – 0.9 V.
The electrochemical experiments were conducted using a standard three electrode system with Ag/AgCl reference electrode, Pt wire external electrode, and ARGO or TGO modified GCE working
electrode (Figure 3). The analyte was prepared by dissolving 64 mg of NADH in 4.5 mL PBS. 4 mL of the prepared NADH solution was added to the 76 mL PBS electrolyte to give a 1 mM concentration. CV scans of the 1 mM NADH cell were carried out with a 10 mV/s scan rate and a potential range of -0.4 V – 0.9 V.
3. Results
The peak potential for the ARGO/GCE was found to be 0.492 V, more negative than the TGO modified GCE which had a peak potential of 0.612 V (Figure 4).
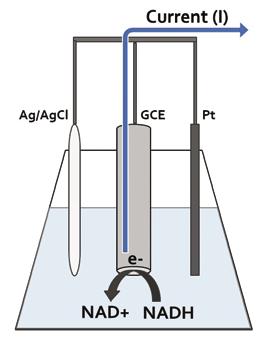
Figure 3: NADH electrochemical cell setup with standard three electrode system
2.2 Quantifying Peak Potential
Three separate overlapping CV scan measurements were used for the analysis after the system stabilized. In each case the CVs showed one nonreversible oxidation reaction resulting in a single current peak during the anodic cycle. The derivative graphs of the anodic scans were analyzed in order to quantify the peak potentials. The graphs were obtained by plotting the change in current (I) divided by the change in potential (V) on the y axis and the corresponding midpoint potential on the x axis. The peak potential was taken as the potential at which the derivative touched or crossed the x-axis after reaching a maximum.
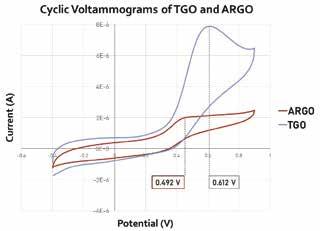
Figure 4: Cyclic voltammograms of ARGO (red) and TGO (blue) modified GCE in the presence of 1 mM NADH in 0.1 M PBS from -0.4 V – 0.9 V with a scan rate of 10 mV/s.
Wang et al. used x-ray photoelectron spectroscopy (XPS) to obtain oxygen group distributions for materials similar to the ones used in this experiment. [7] The XPS results show that ARGO has similar C=O (quinone) and COOH (carboxyl) distributions, but substantially more C-O (epoxide) groups compared to TGO (Figure 5).
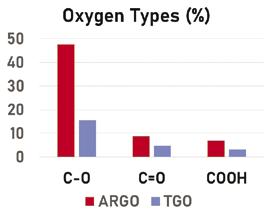
Figure 5: Percent oxygen group distributions in ARGO and TGO obtained by XPS [7]
4. Discussion
The more negative peak potential at ARGO compared to TGO indicates faster electron transfer at ARGO. Since both materials had similar quinone distributions the results counter the initial hypothesis that quinones in GO mediate NADH oxidation in the same way they do in ordered CNTs. Because ARGO and TGO mainly differed only in their epoxide content, it seems logical to conclude that epoxides are more active in NADH mediation on GO. Further research will be needed to confirm that epoxides are truly the mediators in GO dispersions. If this is the case, then sensors should be engineered with high epoxide content GO and tested to see what the optimal material composition is for obtaining the fastest oxidation rate.
Since our results indicated different functional group activity for GO than CNTs it seems as though composition might not be the only factor in determining which groups are most active in the oxidation process. One possible explanation is that the difference in shape between CNTs and GO may impact the activity of certain groups. The curved CNT structure could alter the orientation of moieties that otherwise stick out straight from the flat GO surface. As a consequence, some functional groups might have increased/ decreased exposure to NADH based on whether they are attached to a curved or flat surface. More research is necessary in order to determine if physical structure has any impact on surface group activity.
Although ARGO was found to have a lower peak potential, the current peak was less defined and lower than that of TGO. The low magnitude of the ARGO peak indicates that fewer NADH molecules overall were able to oxidize at the surface, although the reaction proceeded at a faster rate. Additional experiments would be necessary to see if this low peak can be ascribed to adsorption of NAD+ at the electrode surface.
5. Conclusions
This research suggests that NADH oxidation in GO modified GCEs is mediated by epoxide moieties at the GO surface. GO/GCE NADH sensor designs might be improved by maximizing epoxide distributions to increase oxidation rates. Improved sensor designs could greatly aid research into the effects of NADH on human health as well as possible therapeutic uses. Further tests will be needed in order to confirm this study’s results. A diminished current peak was also observed in the ARGO/GCE. Additional research will be necessary in order to determine the cause of the lower current rise.
6. Acknowledgments
This research was conducted under the supervision of Dr. Gilbertson and mentorship of Yan Wang. Funding was provided by Dr. Gilbertson, the Swanson School of Engineering, and the Office of the Provost.
7. References
[1] R.A. Fricker, E.L. Green, S.I. Jenkins, S.M. Griffin, The Influence of Nicotinamide on Health and Disease in the Central Nervous System, Int. J. Tryptophan. Res. 11 (2018), 1-11
[2] N. Braidy, C.K. Lim, R. Grant, B.J. Brew, G.J. Guillemin, Serum nicotinamide adenine dinucleotide levels through disease course in multiple sclerosis, Brain. Res. 1537 (2013), 267-272 [3] C. Canto, K.J. Menzies, J. Auwerx, NAD+ metabolism and the control of energy homeostasis - a balancing act between mitochondria and the nucleus, Cell. Met. 22.1 (2015) 31-53 [4] S.A. Kumar, S. Chen, Electroanalysis of NADH Using Conducting and Redox Active Polymer/Carbon Nanotubes Modified Electrodes-A Review Sensors, 8 (2008), 739-766
[5] L. Zhang, Y. Li, L. Zhang, D. Li, D. Karpuzov, Y. Long, Electrocatalytic Oxidation of NADH on Graphene Oxide and Reduced Graphene Oxide Modified Screen-Printed Electrode, Int. J. Electrochem. Sci. 6 (2011), 819-829
[6] J. Chen, J. Bao, C. Cai, T. Lu, Electrocatalytic oxidation of NADH at an ordered carbon nanotubes modified glassy carbon electrode., Anal. Chim. Acta. 516 (2004) 29-34
[7] Y. Wang, L. M. Gilbertson, Informing rational design of graphene oxide through surface chemistry manipulations: properties governing electrochemical and biological activities, Green. Chem. 19 (2017) 2826-2838