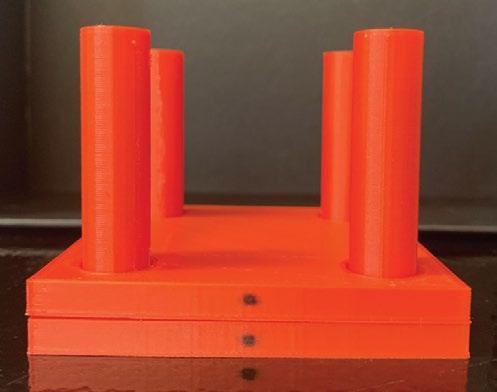
11 minute read
Testing the compressive stiffness of endovascular devices
Lucy Jonesa, Dr. Jonathan Vande Geestb
aSoft Tissue Biomechanics Laboratory, bDepartment of Bioengineering
Lucy Jones Lucy Jones is a senior bioengineering student planning on graduating in the spring. Her interest in biomechanics motivated her to research with Dr. Jonathan Vande Geest at the Soft Tissue Biomechanics Laboratory for nearly two years. She has plans to work for Epic Systems following her undergraduate career.
Dr. Jonathan Vande Geest is a Professor in the Department of Bioengineering, Department of Mechanical Engineering and Material Science, the Department of Ophthalmology, the McGowan Institute for Regenerative Medicine, the Louis J. Fox Center for Vision Restoration, and the Vascular Medicine Institute at the Jonathan Vande Geest, Ph.D. University of Pittsburgh. He received his BS in Biomedical Engineering from the University of Iowa in 2000 and his PhD in Bioengineering from the University of Pittsburgh in 2005. Dr. Vande Geest began his career at the University of Arizona in the Department of Aerospace and Mechanical Engineering and joined the U of A’s Department of Biomedical Engineering in 2009. Dr. Vande Geest returned to the University of Pittsburgh in January of 2016.
Significance Statement
Peripheral artery disease (PAD) is a condition that causes the stenosis of arteries in the limbs. In this work, we used a fabricated 3D printed compression apparatus to assess the compressive resistance of several commercially available stents. Eventually, we will test the compressive resistance of our prototype endovascular device.
Category: Device Design
Keywords: endovascular device, compressive strength,
peripheral artery disease, stent
Abstract
Peripheral Artery Disease (PAD) is a condition characterized by the stenosis of arteries in the limbs. It can lead to negative consequences ranging from numbness, to amputation, or even death. Treatment methods for PAD include surgical intervention, or angioplasty, and stent implantation. However, these treatment methods are known to fail at high rates and could cause infection, vascular injury, or the restenosis of the artery. As a result, our team has created a new endovascular device that can exert force on the artery walls in order to keep it open while also maintaining the flexibility necessary to contend with complex mechanical regions such as the knee. In preparation for the assessment of our novel device, we have fabricated a simple 3D printed apparatus to evaluate the compressive resistance of our device and commercially available stents. In this work, we have assessed the compressive resistance of several commercially available stent devices. Our findings show that stents can generally withstand compressive loads up to 400g before they experience severe buckling. In the future, we intend to evaluate the compressive resistance of our endovascular device and compare it to that of stents.
1. Introduction
Peripheral Artery Disease (PAD) is a disease affecting approximately 202 million people worldwide [1]. This condition causes the stenosis of arteries and reduces blood flow to distal tissues. If left untreated, PAD will lead to stroke, amputation, or death. There are several available methods of treatment for this condition which include angioplasty, medication, and endovascular stent or stent-graft implantation.
Stent grafts are endovascular devices made of a metal mesh and covered in fabric that following deployment expand the artery to keep it patent. People who experience PAD in their femoropopliteal artery need a solution that will be able to endure the complex mechanical challenges associated with that region of the leg (located behind the knee). Stent graft implementation has become a common treatment for PAD in extremities that experience loading from joint movement. Yet, stent graft failure is incredibly common, especially in the femoropopliteal artery, with an approximate 50% loss of patency two years following implantation [2]. Given the adverse effects of PAD, stent failure can be detrimental to the patients’ health and potentially fatal. As a result of the frequent failure of current stents, there has been an effort to make stents more adaptable to their mechanical environment. For instance, there has been success with the Misago, Supera, and Absolute stents, but the flaw of current stents still seems to be the rigidity of the design that is required to create a supportive scaffold inside stenosed arteries. Since stents are still not wholly effective in the long-term treatment of PAD in complex mechanical regions, our goal is to design a new device that will create a more flexible solution and allow for blood flow through the artery with a reduced risk of restenosis. For this phase in the development of our device, we
are evaluating the compressive resistance of commercially available stents so that we can compare the results to those of our new endovascular device. In order to test the compressive strength of existing endovascular stents, we 3D printed a device, shown in Figure 1, that would allow for a known mass to compress the stents. We determined the masses used in our study by extrapolating values from the minimum “hoop stress” or the “compressive force acting on the stent” in an artery [3]. Our hypothesis is that if a mass above 400g is added to a stent using the compression apparatus that we have developed; the stent will collapse and no longer have functionality.
2. Methods
We started by obtaining 4 Taxus Liberté monorail stents which are commercially available and widely used in the treatment of PAD as well as coronary artery disease. Three of these stents had an initial diameter of 2.5mm and one had an initial diameter of 2.75mm. We then deployed our stents using a device called an indeflator. The indeflator is essentially a large syringe that fills balloon catheters with water, causing them to expand, and conforms the stent that is cinched around the balloon catheter to its cylindrical shape. Once the balloon is deflated again, the stent can be removed and used for compression testing. A total of 6 masses were added to each of the stents: 50g, 100g, 200g, 400g, 600g, and 800g respectively using the device shown in Figure 1. As each mass was added, a 12-megapixel wide-angle camera was used to capture a digital image showing the compression of the device. We then utilized a Matlab image processing program to quantify the diameter of the stents under each given load. The diameters of the stents were then compiled into a graph so that the reduction in diameter, and therefore the compression of the stents under various loads, could be quantified and evaluated.
Figure 1. 3D Printed Compression Device. This device consists of 2 parts: a square top plate with a hole in each corner and a square base plate with 4 cylindrical prongs extending vertically out of the corners. The stent is placed on the base plate while the top plate is lowered onto it by lining the holes up with each of the prongs. Then, the desired weight is positioned on the top plate and the change in stent diameter (distance between top plate and base plate) is measured.
3. Results
We were able to determine the diameter of the stents under various loads ranging up to 800g. Figure 2 shows that all of the stents could withstand loading up to 400g, but at 600g, most of the stents collapsed. Meaning, when a mass of 600g was added to the top plate of our compression device, the stent in between the top and bottom plates was completely flattened. Only one stent was able to stay open under 600g of weight. It’s also worth noting that none of the stents exhibited any drastic (more than 0.5mm) changes in diameter until weights exceeding 200g were added. Meaning, the changes in diameter under weights ranging from 50g to 200g were very slight for all of the stents. However, once that weight exceeded 200g, there is a noticeable reduction in the diameter of all of the stents, ranging from 0.45mm to 1.14mm.
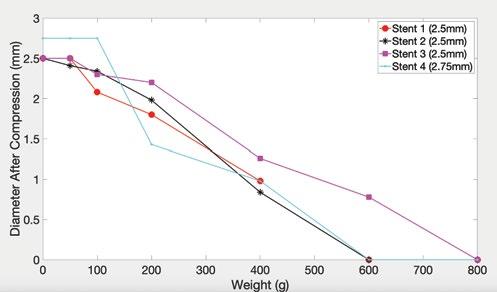
Figure 2. Compression of Stents Under Loading. This graph displays the results of 4 different endovascular stents subjected to varying amounts of compressive loading. It shows that all of them could withstand compressive loading up to 400g. At 600g, all but one stent is fully compressed.
Figure 3a shows Stent 1 prior to compression, while Figure 3b displays that same stent after 600g was added to it using the compression device. These figures demonstrate that this stent has completely lost its cylindrical shape under 600g of loading.
Figure 3a. Stent 1 Initial Diameter. Figure 3b. Stent 1 After 600g Loading. This shows the diameter and initial This displays Stent 1, fully compressed, shape of Stent 1 prior to any loads after 600g of weight was added. being added.
4. Discussion
This experiment yielded results that were mostly to be expected. We were expecting the stents to slowly deform as more weight was added, and then to collapse under a mass exceeding 400g. This did happen in 3 out of the 4 stents and therefore allowed us to tentatively confirm our hypothesis. However, there were some idiosyncrasies that we did not expect. For example, the drastic reduction in diameter of the stents that occurred when the load exceeded 200g was not something we anticipated. Considering the fact that stents are being placed in arteries that can sometimes be bent at extreme angles, we expected them to display a resistance to intense changes so that in physiological environments, they would not fail after one harsh bend. Since this was not the case, we believe that stents are only designed to be unyielding under certain small increments of weight. Our conjecture is that stents fail at higher rates in more complex mechanical regions because once they begin to buckle, they lose their integrity entirely. This is shown in the contrast between Figures 3a and 3b. Stent 1 had become completely compromised after a 600g weight was added to it. In an in vivo environment, this would mean that the stent could no longer keep the artery walls open and restenosis would occur. This would align with our knowledge of stent failure in the femoropopliteal artery since sudden drastic movement is to be expected in that region.
This experiment also helped us test the effectiveness of our 3D printed device. Seeing as we were able to conduct the experiment with believable results, we have determined it to be a functional tool in preliminary compressive testing. Our next task will be to quantify the compressive resistance of our novel endovascular device. Eventually, our goal is to compare the compressive resistance of our novel device to existing stents to see whether there are any significant differences.
There are many limitations to our study and how it can be applied in a clinical setting. Firstly, our compression device is a rudimentary tool used to give preliminary results. Stents are usually tested using axial, tensile, and radial compression, as well torsion and bending tests [4]. Our compression device is most similar to the radial compression system, but it is still limited in what it can tell us about the overall mechanical advantages of a stent. For instance, we did not incorporate any torque, internal pressure, or bending to our stents which would all be experienced by a stent in the femoropopliteal artery. Therefore, when we move to testing our endovascular prototype, we will be able to compare its compressive resistance to that of stents, but we will not know how it withstands other forces. As a result, we will not get a comprehensive picture of how effective our prototype is in comparison to stents. This means that after compressive testing, we will need to perform other experiments, preferably in vivo, to determine the efficacy of our novel endovascular device.
5. Conclusions
From our results, we can see that our simple 3D printed device can be utilized to quantify the compression of endovascular devices. We will be using this device to find the compressive resistance of our new prototype endovascular device and comparing the results to those that we have generated from the stents. Our study has clinical implications because once we have the in vitro data to support the claim that our device can withstand more loading than current stents, we will be able to move forward with the in vivo testing of our endovascular device. In vivo testing will yield more realistic results in terms of the actual physiological constraints that endovascular devices face and will help us to modify our design criteria to meet the complex mechanical needs of those arteries. However, given that we are in the preliminary stages of our research, these results are promising and give us a baseline compressive strength that we hope to obtain with our prototype. In conclusion, we can confirm our hypothesis, as our testing has shown that all of the stents can withstand compressive loads up to 400g, beyond which they are prone to collapse.
6. Acknowledgements
This work was completed with the generous funding from the Swanson School of Engineering, the Office of the Provost, the Department of Bioengineering, and the University of Pittsburgh’s Center for Medical Innovation grant number F_274.
7. References
[1] Song P, Rudan D, Zhu Y, Fowkes FJI, Rahimi K, Fowkes FGR, Rudan I. Global, regional, and national prevalence and risk factors for peripheral artery disease in 2015: an updated systematic review and analysis. Lancet Glob Health. 2019 Aug;7(8):e1020-e1030. [2]Strecker EP, Boos IB, Göttmann D. Femoropopliteal artery stent placement: evaluation of long-term success. Radiology. 1997 Nov;205(2):375-83. doi: 10.1148/radiology.205.2.9356617. PMID: 9356617. [3] Cabrera, M. S., Oomens, C. W., & Baaijens, F. P. (2017). Understanding the requirements of self-expandable stents for heart valve replacement: Radial force, hoop force and equilibrium. Journal of the Mechanical Behavior of Biomedical Materials, 68, 252-264. doi:10.1016/j. jmbbm.2017.02.006 [4] Maleckis, Kaspars et al. “Comparison of femoropopliteal artery stents under axial and radial compression, axial tension, bending, and torsion deformations.” Journal of the mechanical behavior of biomedical materials vol. 75 (2017): 160-168. doi:10.1016/j.jmbbm.2017.07.017