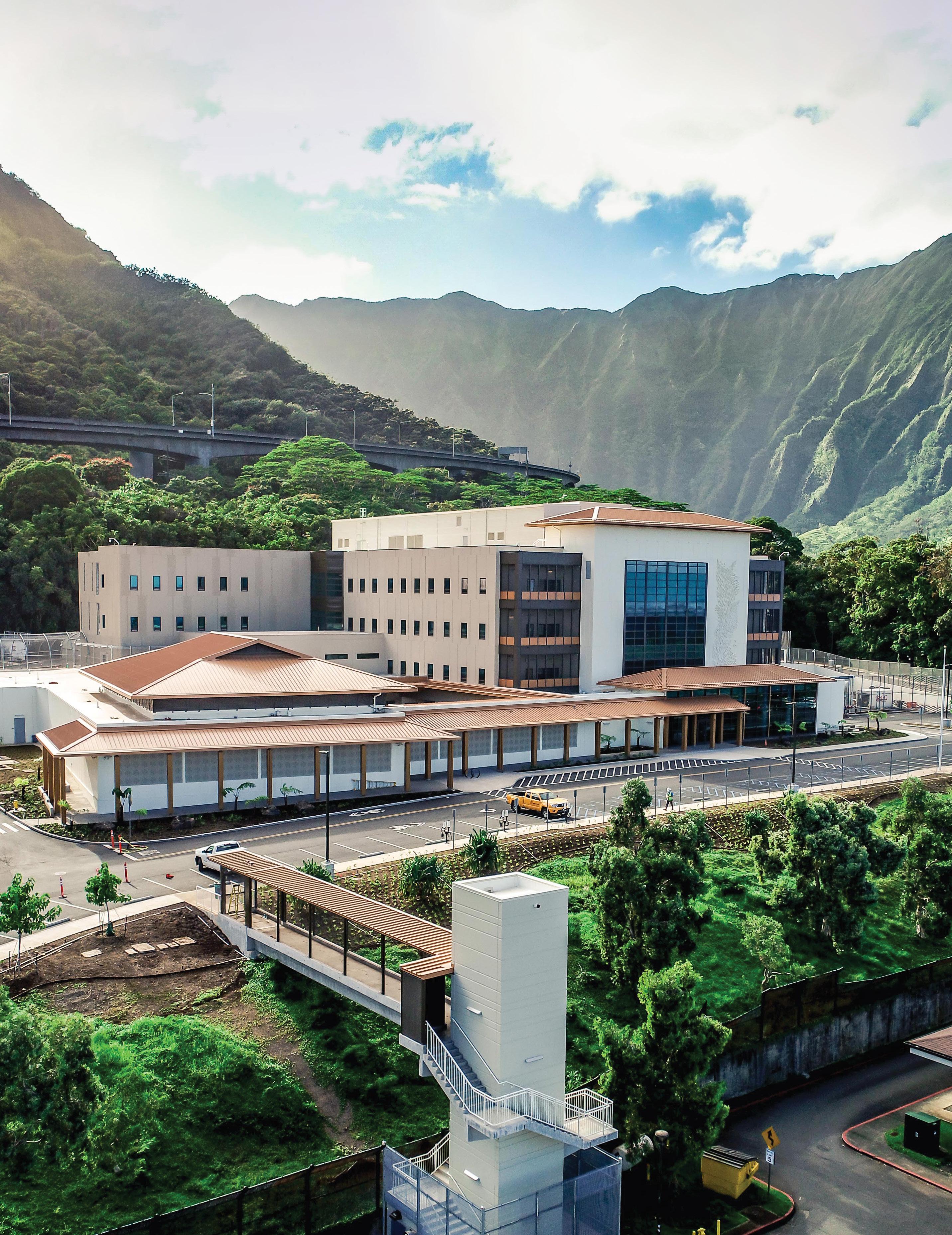
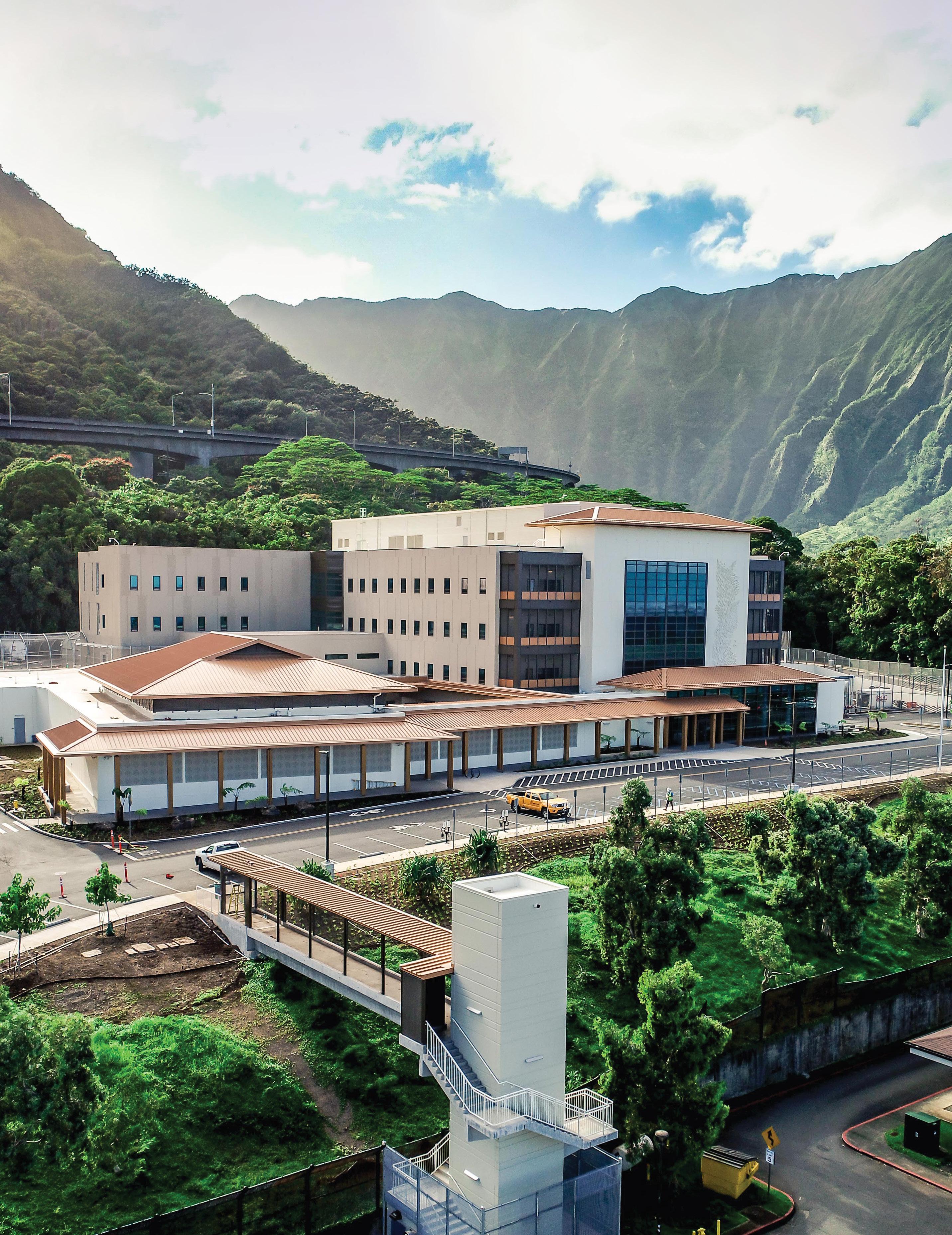
WHERE VISION BECOMES STRUCTURE
®
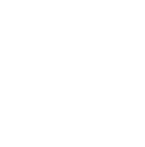
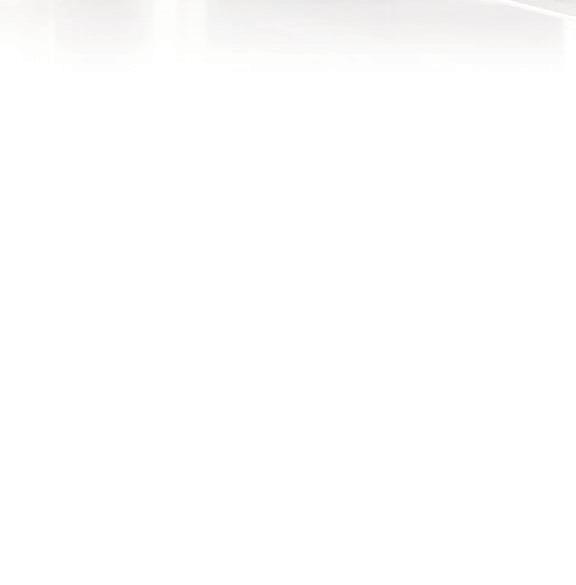
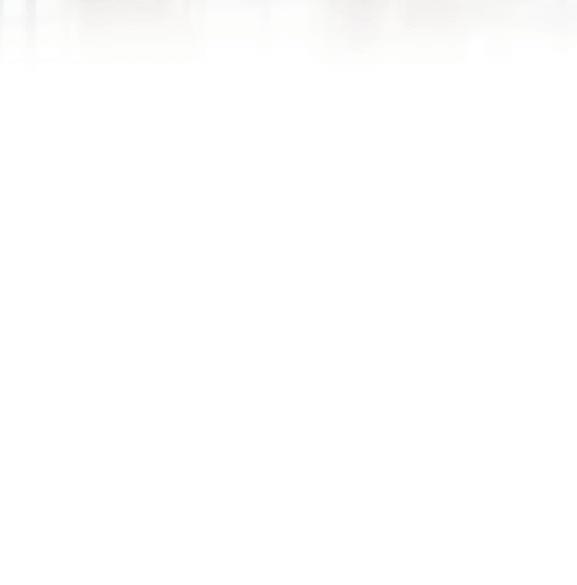

RISA o ers a comprehensive suite of design software that work together to simplify even the most complex projects. As a result, engineers can work e ciently on a variety of structures in a mix of materials including steel, concrete, wood, masonry and aluminum. risa.com
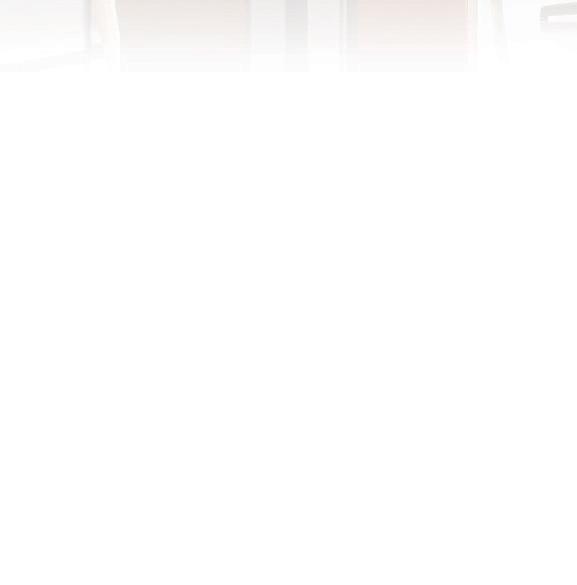
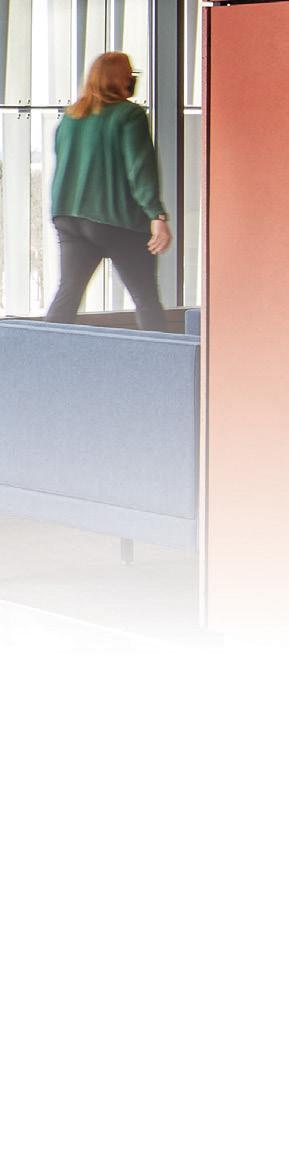
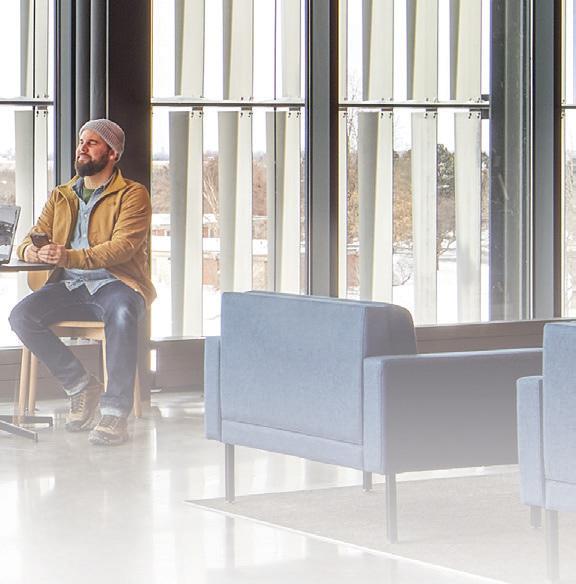
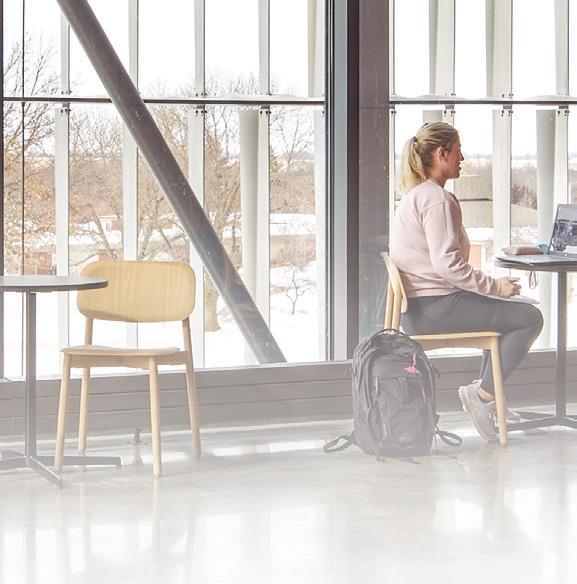
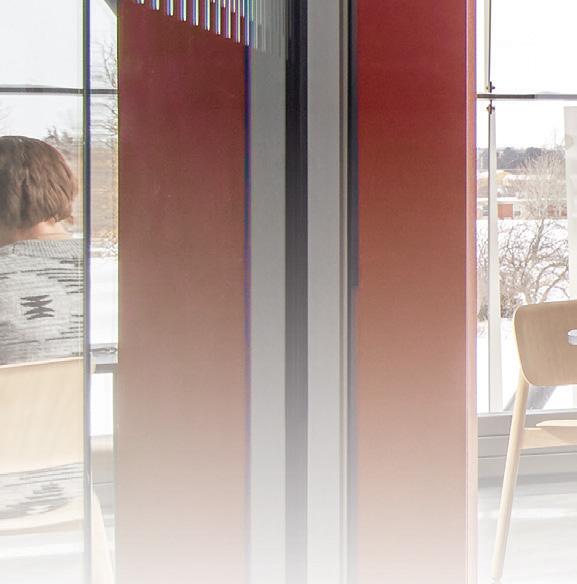
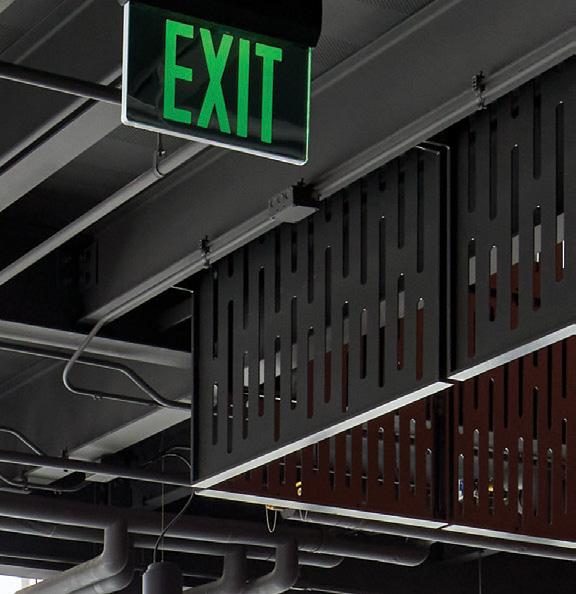
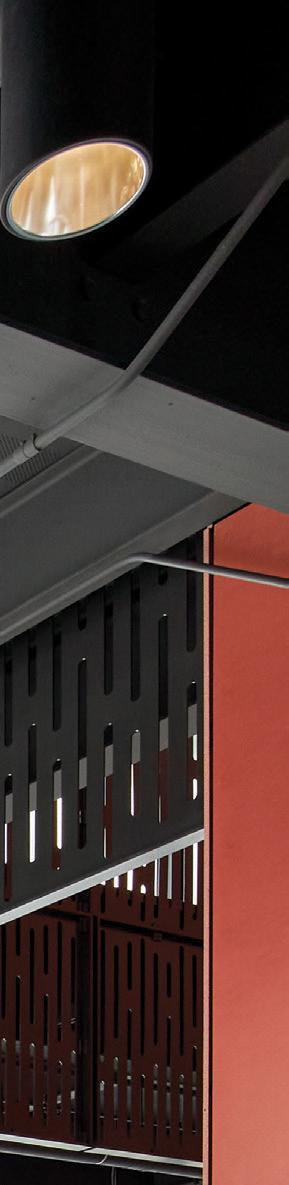
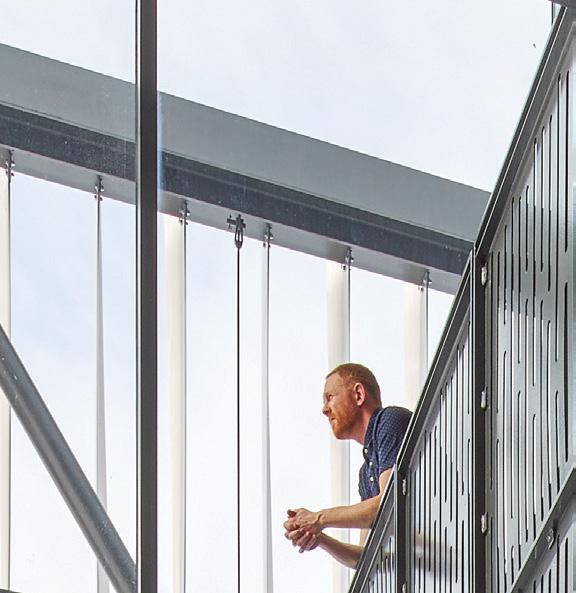
Southeast Community College
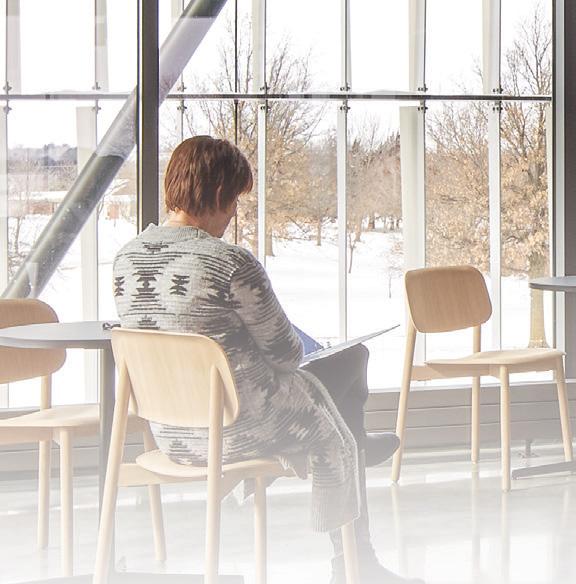
Academic Excellence Center
Composite Steel, Steel Braced Frames, Shear Walls Olsson
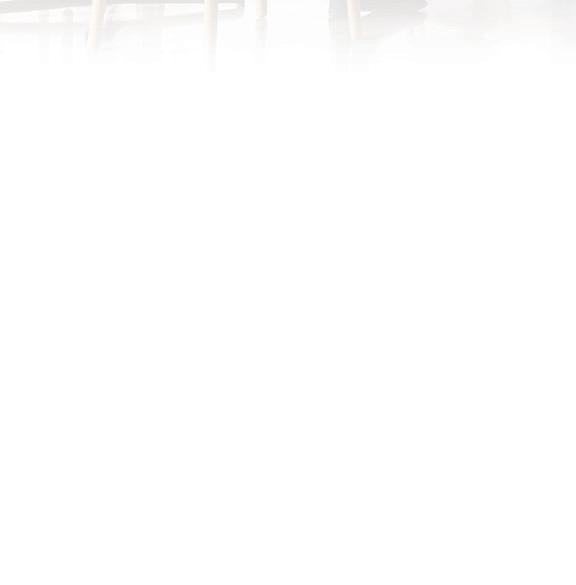
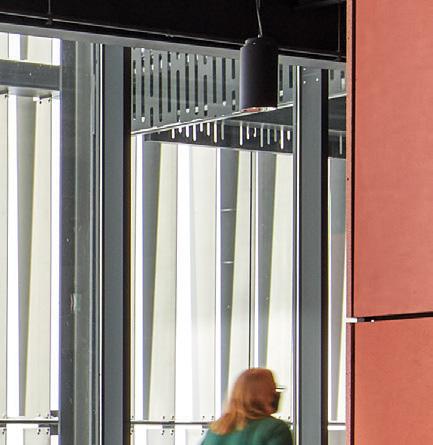
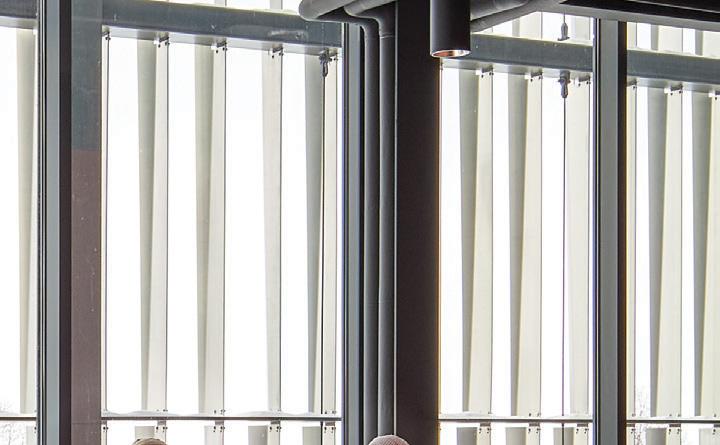
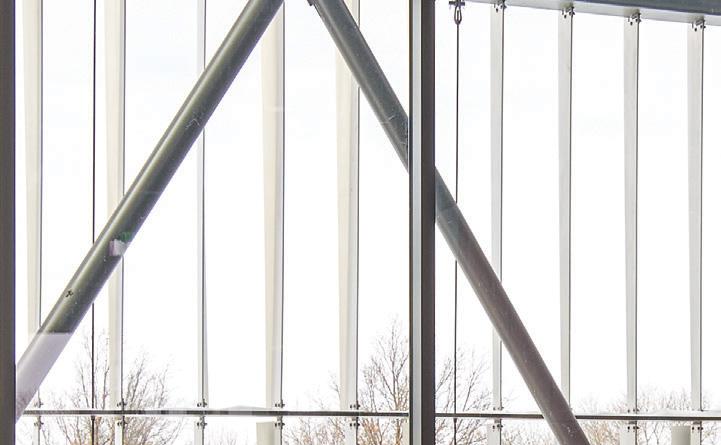
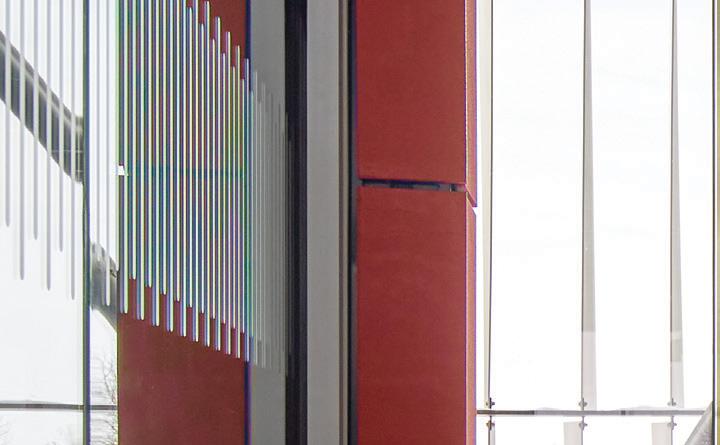
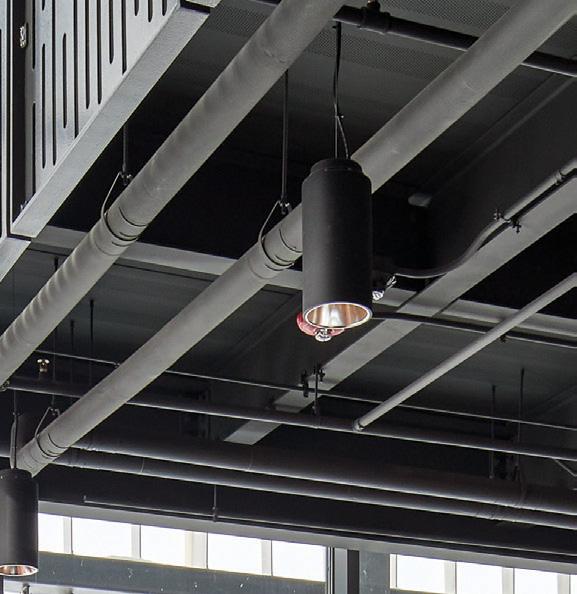
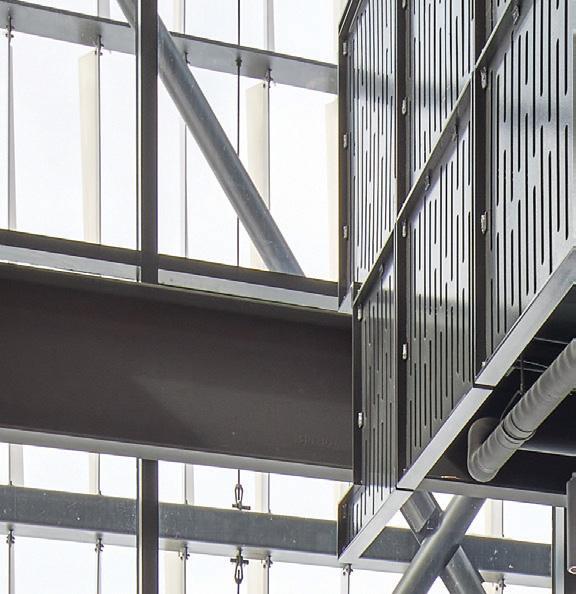
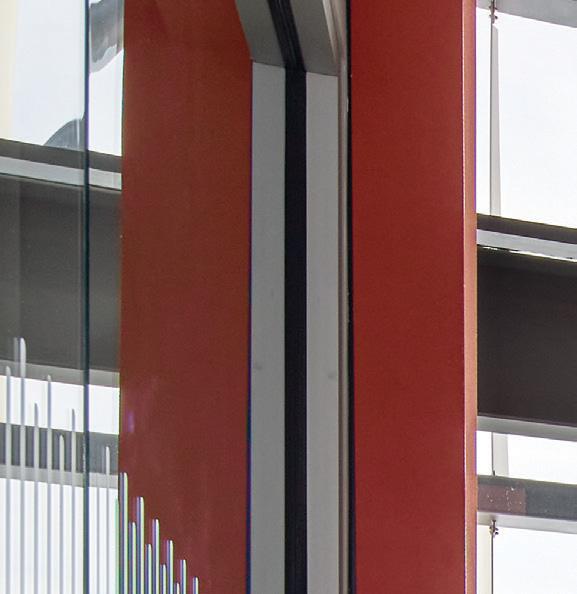
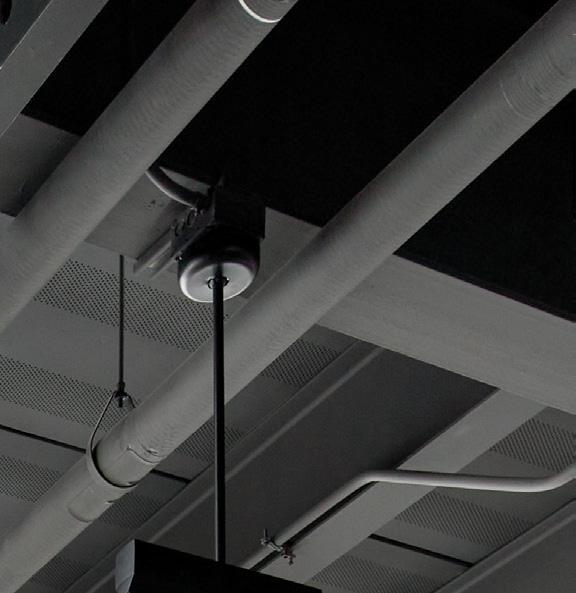
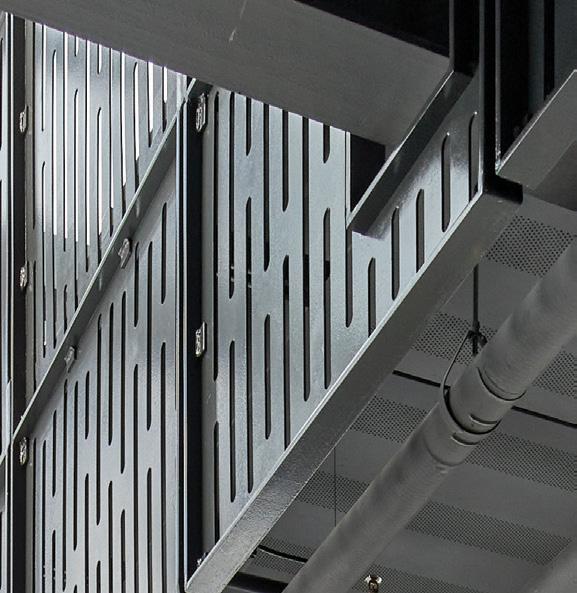
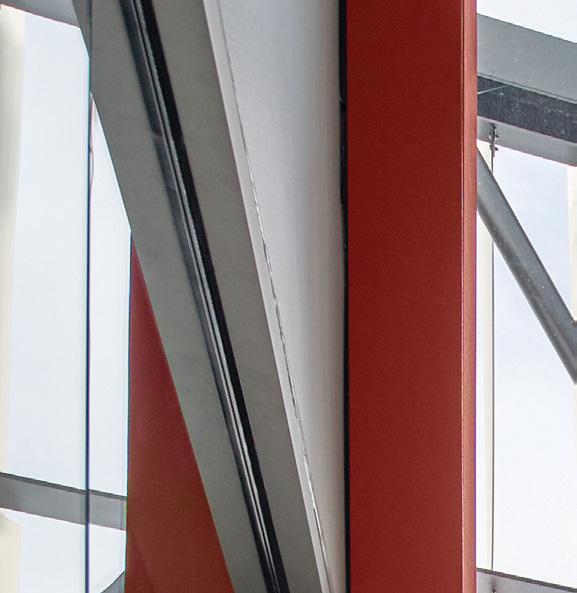
index
American Concrete Institute
Atkinson-Noland & Associates
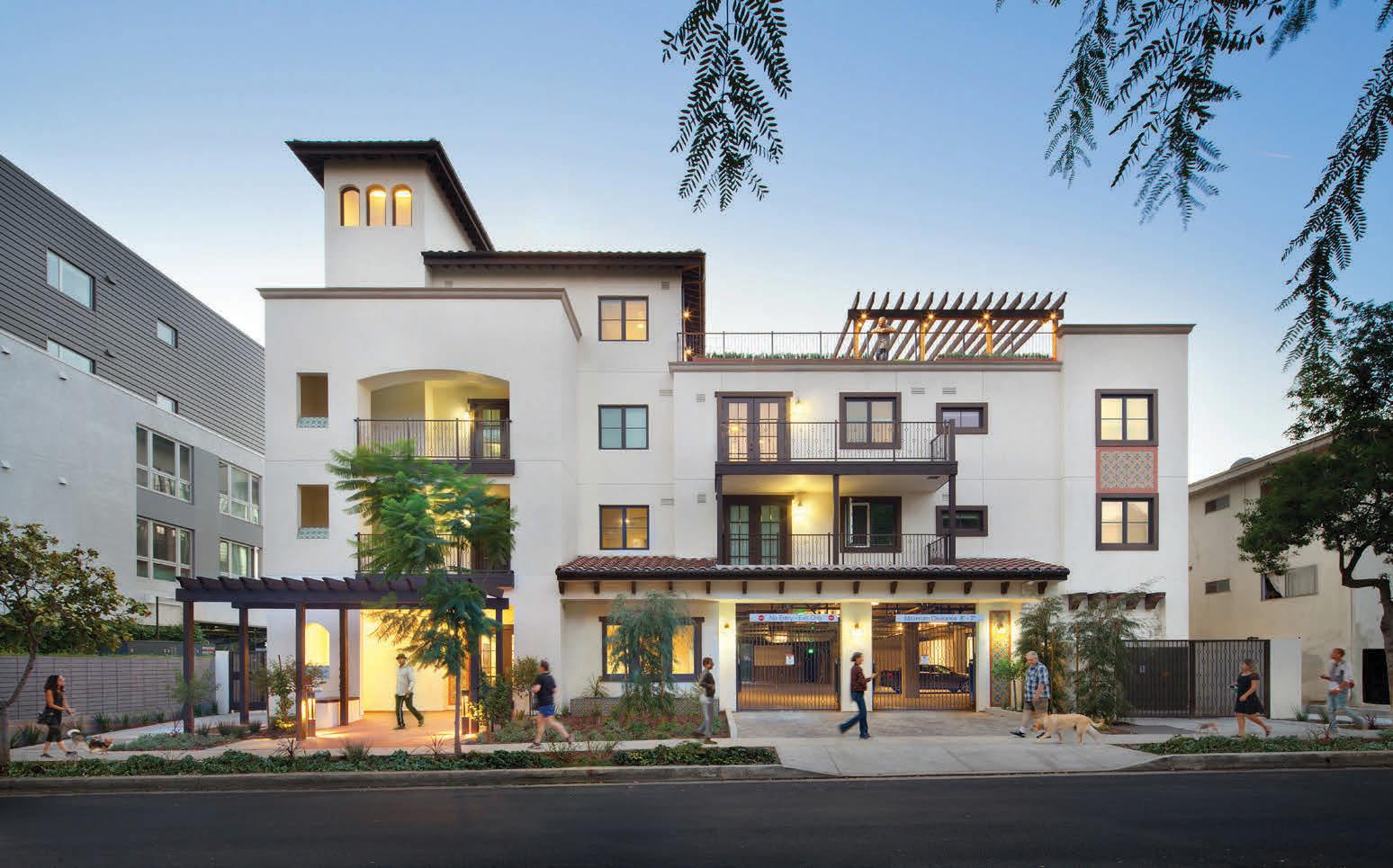
AWI Panels
Computers & Structures, Inc
Coughlin Porter Lundeen (CPL)
CTS Cement Manufacturing Corp
Dewalt
ENERCALC
Euclid Chemical
ICC - Evaluation Service
Integrated Engineering Software, Inc.
KPFF
Please support these advertisers
STRUCTURE
MAPEI Corp. Martin/Martin Max USA Corp PCS-Structural PS=Ø PT-Structures
RISA Technologies Simpson Strong-Tie SSFM International Profile Trimble
Wood Products Council
www.kpff.com
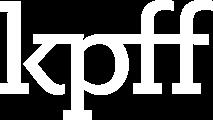
MARKETING & ADVERTISING SALES
Director for Sales, Marketing & Business Development
Monica Shripka Tel: 773-974-6561 monica.shripka@STRUCTUREmag.org
EDITORIAL STAFF
Executive Editor Alfred Spada aspada@ncsea.com
Publisher Christine M. Sloat, P.E. csloat@STRUCTUREmag.org
Associate Publisher Nikki Alger nalger@STRUCTUREmag.org
Creative Director Tara Smith graphics@STRUCTUREmag.org
EDITORIAL BOARD
Chair John A. Dal Pino, S.E. FTF Engineering, Inc., San Francisco, CA chair@STRUCTUREmag.org
Jeremy L. Achter, S.E., LEED AP ARW Engineers, Ogden, UT
Erin Conaway, P.E. AISC, Littleton, CO
Linda M. Kaplan, P.E. Pennoni, Pittsburgh, PA
Charles “Chuck” F. King, P.E. Urban Engineers of New York, New York, NY
Nicholas Lang, P.E. Masonry Industry Representative
Jessica Mandrick, P.E., S.E., LEED AP Gilsanz Murray Steficek, LLP, New York, NY
Jason McCool, P.E. Robbins Engineering Consultants, Little Rock, AR Brian W. Miller Davis, CA
Evans Mountzouris, P.E. Retired, Milford, CT
John “Buddy” Showalter, P.E. International Code Council, Washington, DC Eytan Solomon, P.E., LEED AP Silman, New York, NY
Jeannette M. Torrents, P.E., S.E., LEED AP JVA, Inc., Boulder, CO
STRUCTURE ® magazine (ISSN 1536 4283) is published monthly by The National Council of Structural Engineers Associations (a nonprofit Association), 20 N. Wacker Drive, Suite 750, Chicago, IL 60606 312.649.4600. Periodical postage paid at Chicago, Il, and at additional mailing offices. STRUCTURE magazine, Volume 29, Number 9, © 2022 by The National Council of Structural Engineers Associations, all rights reserved. Subscription services, back issues and subscription information tel: 312-649-4600, or write to STRUCTURE magazine Circulation, 20 N. Wacker Drive, Suite 750, Chicago, IL 60606.The publication is distributed to members of The National Council of Structural Engineers Associations through a resolution to its bylaws, and to members of CASE and SEI paid by each organization as nominal price subscription for its members as a benefit of their membership. Yearly Subscription in USA $75; $40 For Students; Canada $90; $60 for Canadian Students; Foreign $135, $90 for foreign students. Editorial Office: Send editorial mail to: STRUCTURE magazine, Attn: Editorial, 20 N. Wacker Drive, Suite 750, Chicago, IL 60606. POSTMASTER: Send Address changes to STRUCTURE magazine, 20 N. Wacker Drive, Suite 750, Chicago, IL 60606.
STRUCTURE is a registered trademark of the National Council of Structural Engineers Associations (NCSEA). Articles may not be reproduced in whole or in part without the written permission of the publisher.
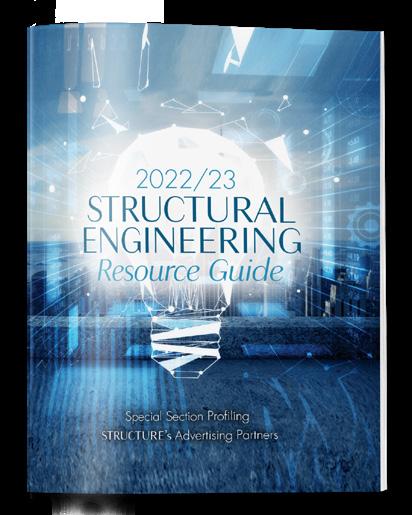
Cover Feature
HAWAII STATE HOSPITAL’S NEW PATIENT FACILITY
By Kevin Galvez, S.E., and Frank K. Humay, Ph.D., S.E. Resiliency was a critical requirement for the New Patient Facility at the foot of the Ko’olau Mountain Range in Kāne‘ohe, O‘ahu. The Design-Build team on this project encountered numerous issues throughout its design and construction. One solution was the use of pre-cast concrete for this remote location. The team learned that it is not always the lowest-cost structural system that produces the most efficient and economical design.
Columns and Departments Feature
LINDQUIST HALL REMODEL AND SEISMIC RENOVATION
By Jeremy L. Achter P.E., S.E.Located within one-half mile of the Wasatch Fault in Northern Utah, Lindquist Hall’s inherent lateral force resisting was insufficient. It was also extremely heavy, resulting in very large seismic forces. One of the solutions for the myriad of seismic issues included de-couple the existing building from the existing shear wall system and replacing it with a buckling-restrained braced frame (BRBF) system.
7 Editorial The Electric Power Grid: The Times They Are A-Changin’
By Wesley J. Oliphant, P.E. 8 Structural Connections Robust Anchorages to Concrete Structures By Christoph Mahrenholtz, Ph.D., Donald F. Meinheit, Ph.D., P.E., S.E., and Nicholas Cewkine III, P.E. 12 Structural Systems
Ductile Coupled Reinforced Concrete Shear Wall System By S. K. Ghosh, Ph.D.
By Mahmut Ekenel, Ph.D, P.E. 20 Building Blocks A Closer Look at Structural Lightweight Concrete
By Ken Harmon, P.E.
By Hee Yang Ng, MIStructE, C.Eng., P.E.
Structural Resilience Adapt and Transform – Part 2
By NCSEA Resilience Committee
InFocus Automation and the Future of Structural Engineering – Installment 4
By Eytan Solomon, P.E., LEED AP
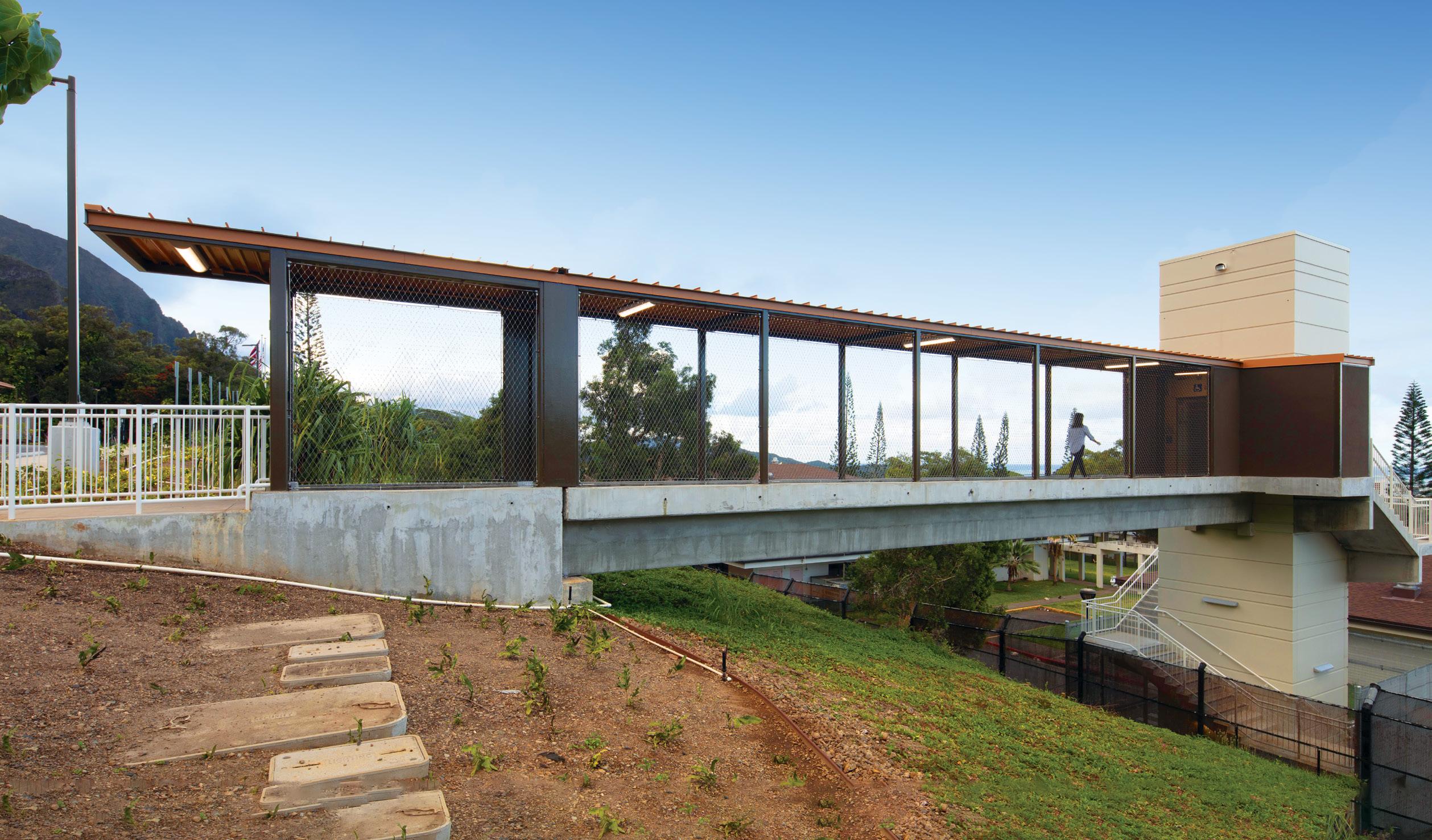
Basic Education NCSEA 2021 Practitioner Sur vey
By Michelle Kam-Biron, P.E., S.E. Brent Perkins, P.E., S.E., and Scott Francis, P.E.
Historic Structures Fr. Hennepin Bridge
By Frank Griggs, Jr., D.Eng, P.E.
By ACEC Staff and CASE Executive Committee
By John McNellis
Publication of any article, image, or advertisement in STRUCTURE® magazine does not constitute endorsement by NCSEA, CASE, SEI, the Publisher, or the Editorial Board. Authors, contributors, and advertisers retain sole responsibility for the content of their submissions. STRUCTURE magazine is not a peer-reviewed publication. Readers are encouraged to do their due diligence through personal research on topics.
magazine
Slide a smarter cladding connection into place.
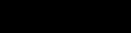

Specify cladding and curtain-wall connections with the new Edge-Tie™ system from Simpson Strong-Tie. Designed with a unique, extruded shape and pour stop, it provides two times the load capacity of traditional bent plates. This innovative solution utilizes bolts that allow installers to easily position and adjust anchors along a continuous slot. By eliminating field welding, the Edge-Tie system speeds up building enclosure while saving time and labor costs. It’s simply a smarter, faster way to get the job done.
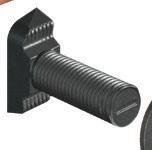
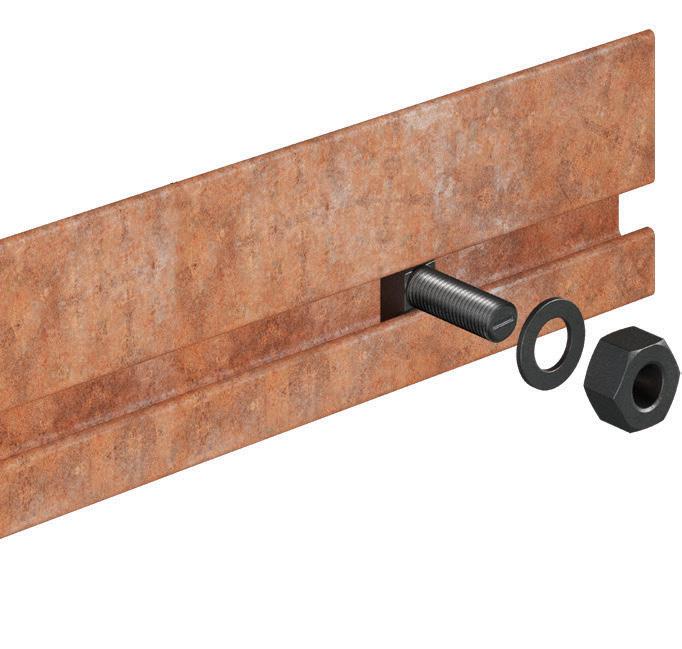
Engineer your next project with all of our structural steel solutions. To learn more, visit go.strongtie.com/edg etiesystem or call (800) 999-50 99.
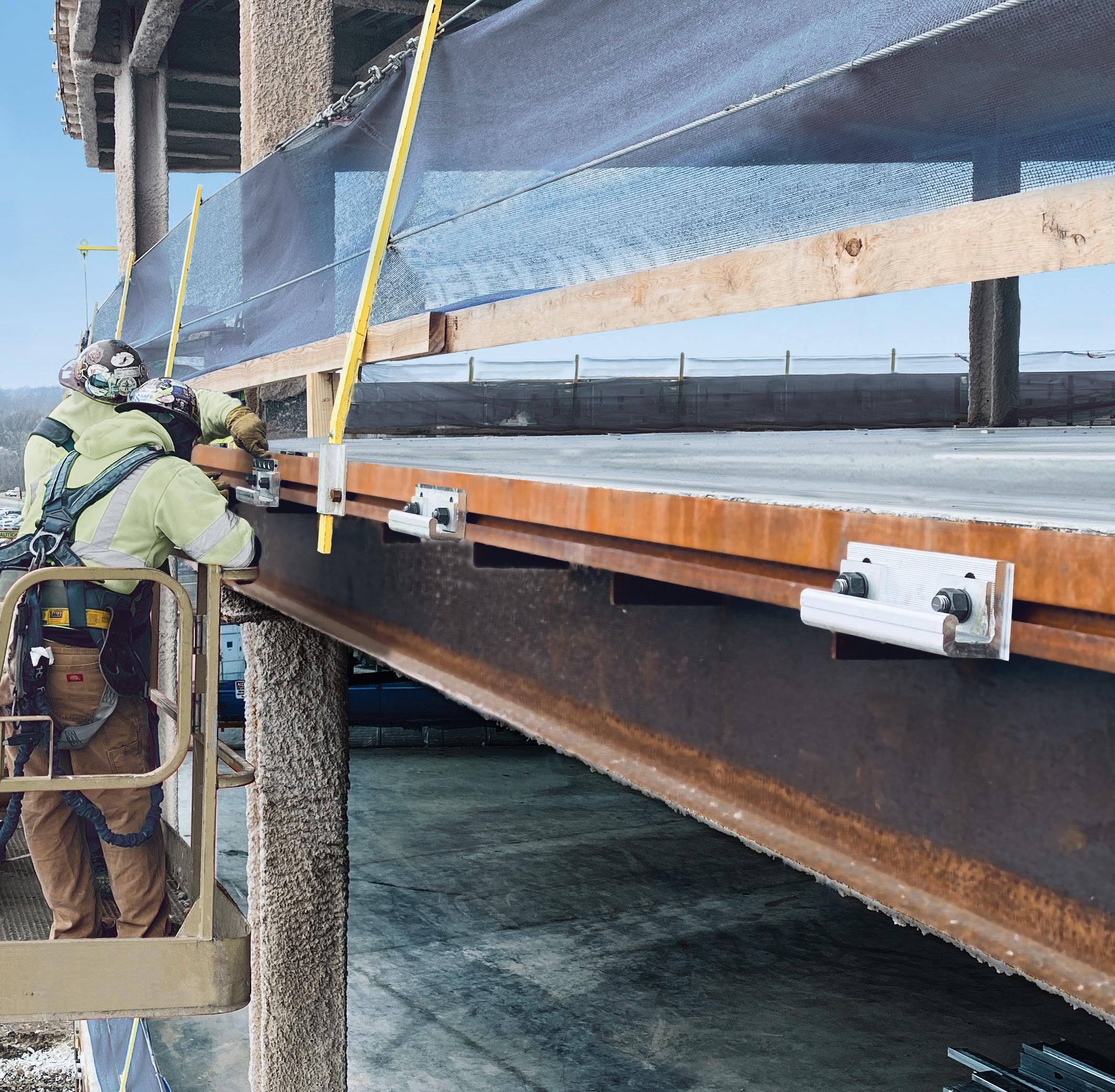
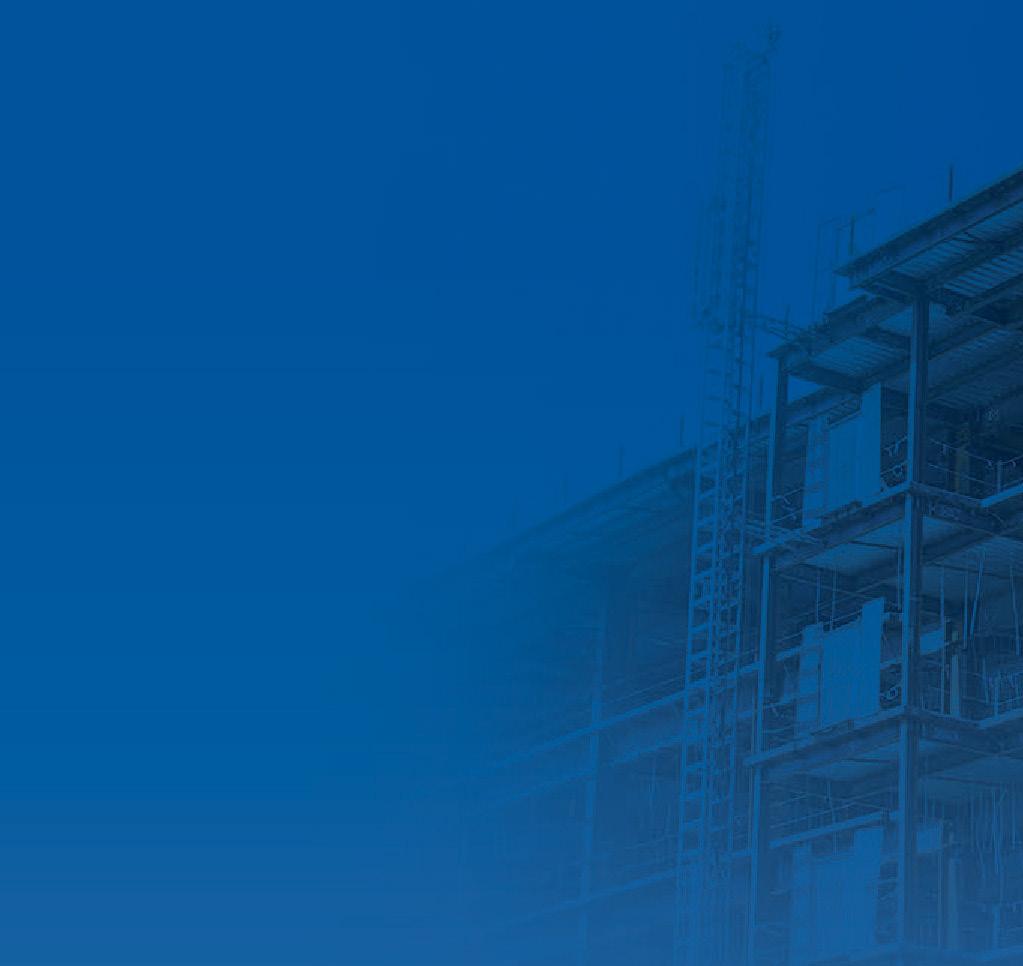
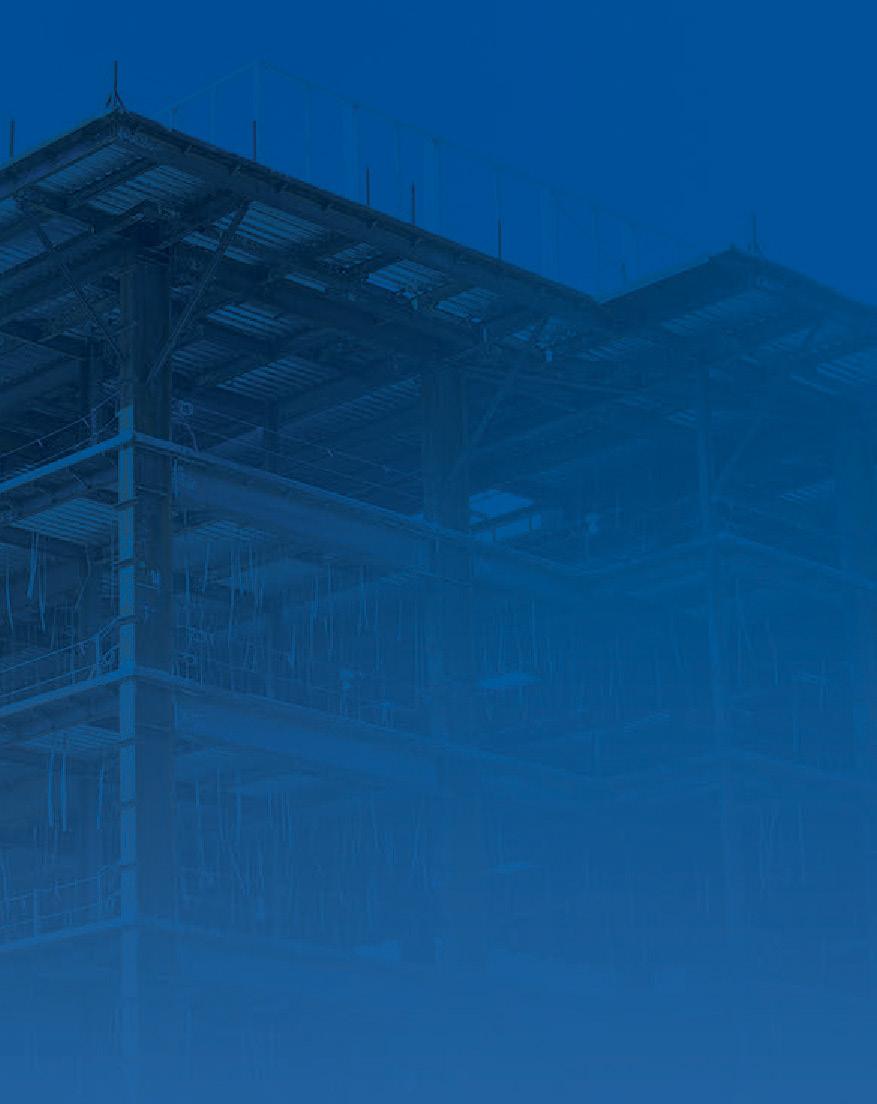
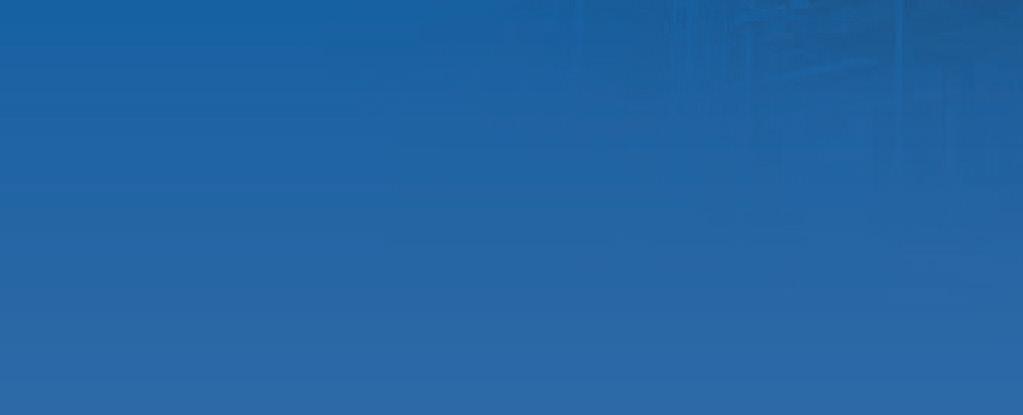
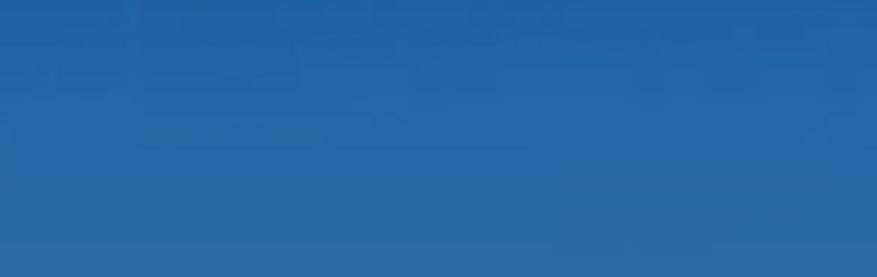

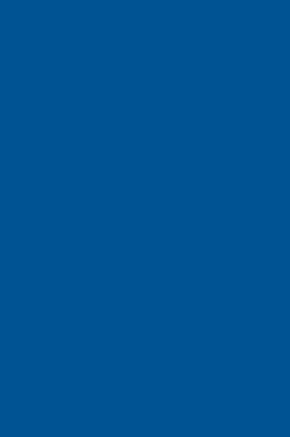
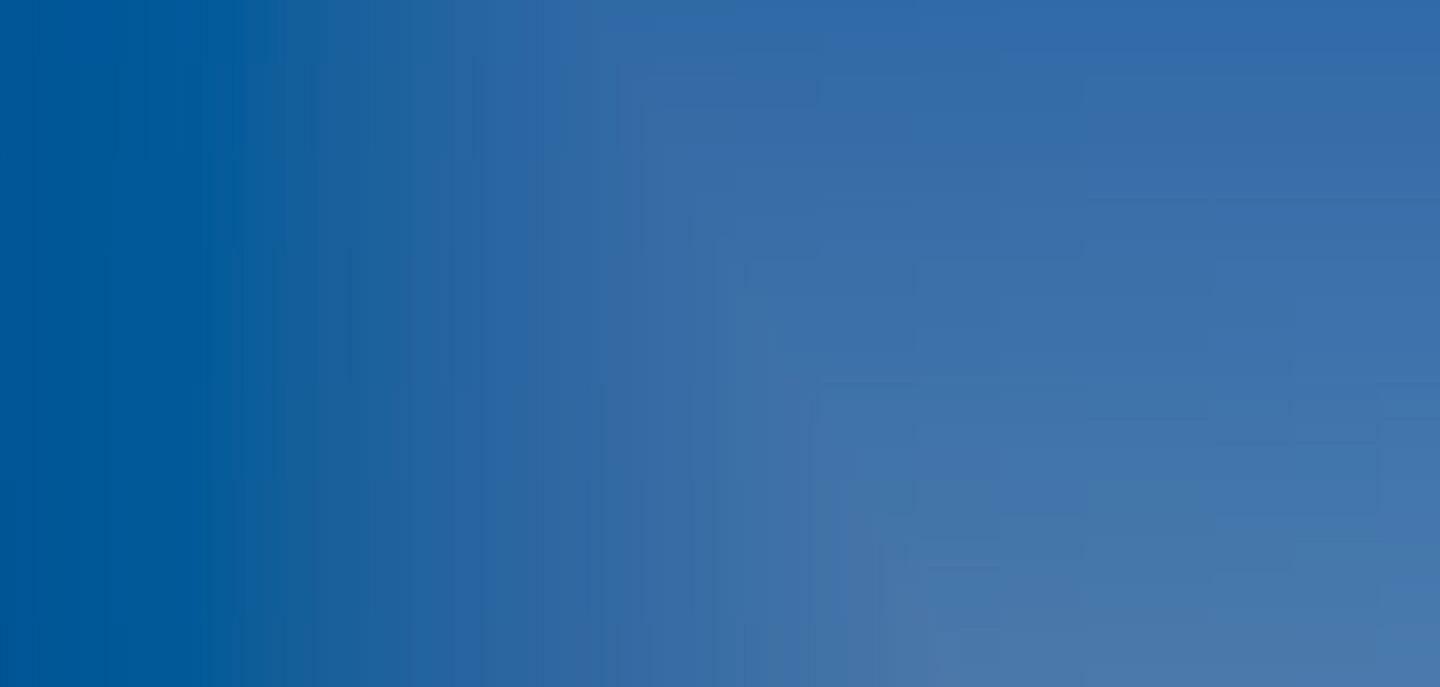
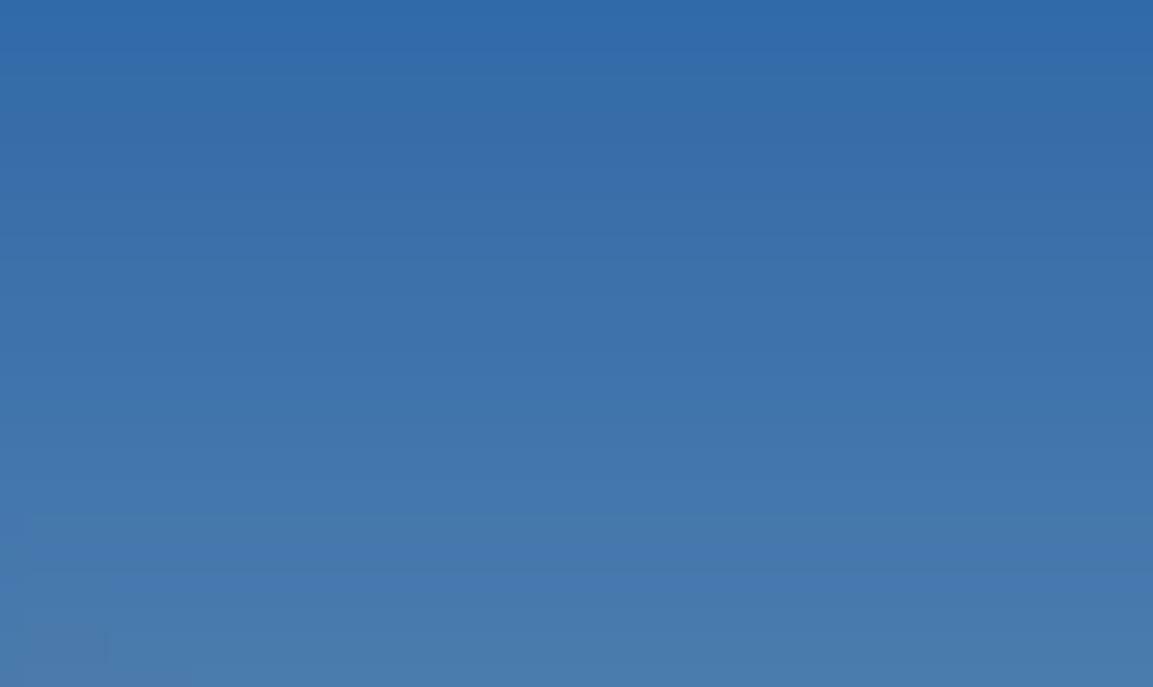
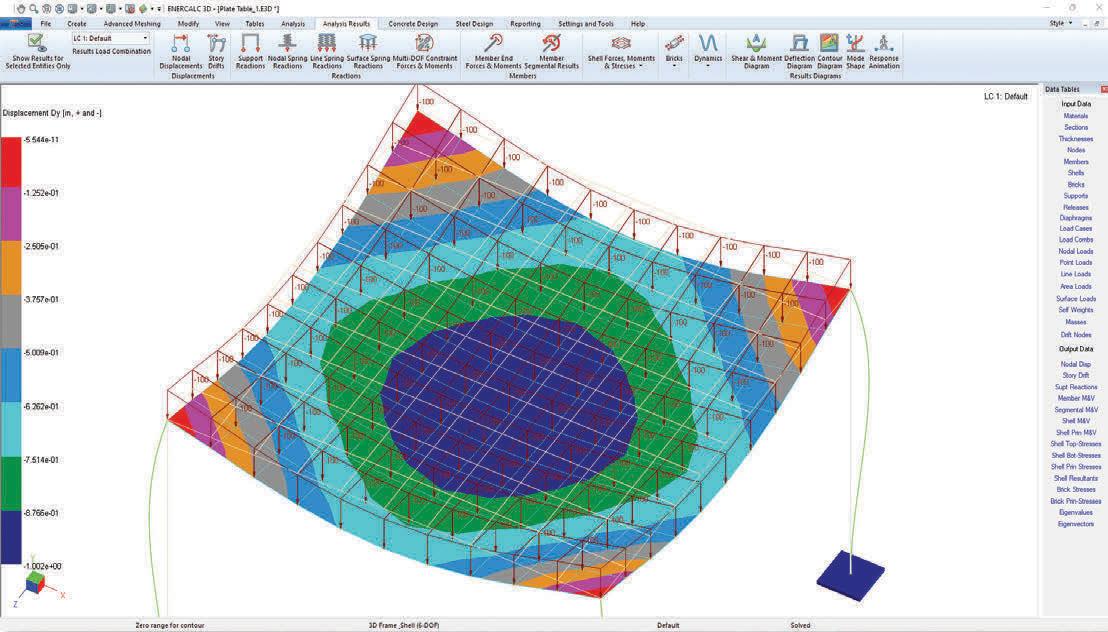
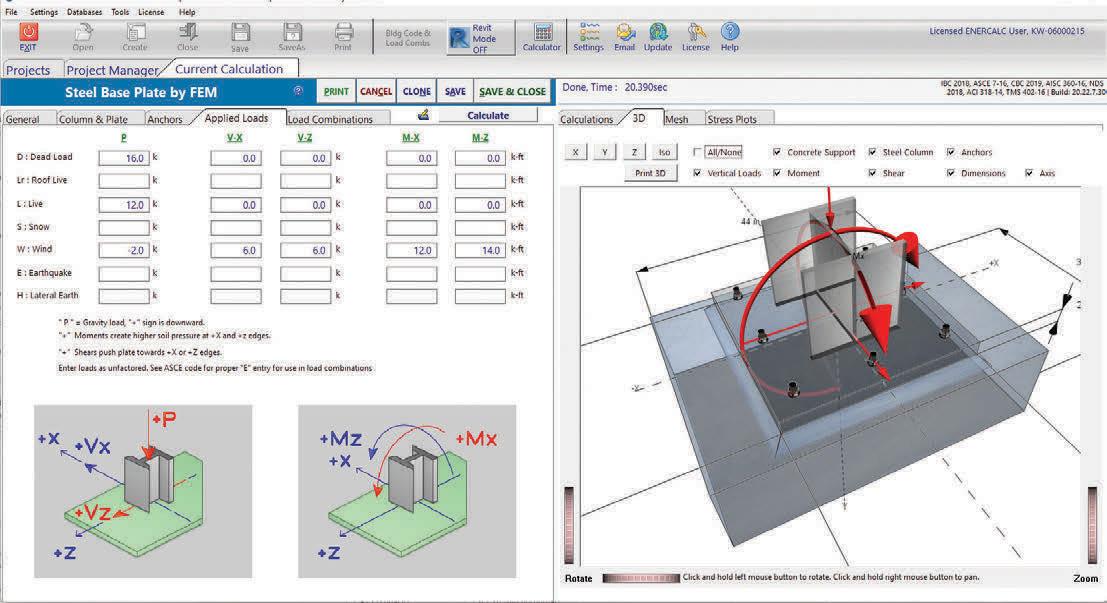
The Electric Power Grid: The Times They Are A-Changin’
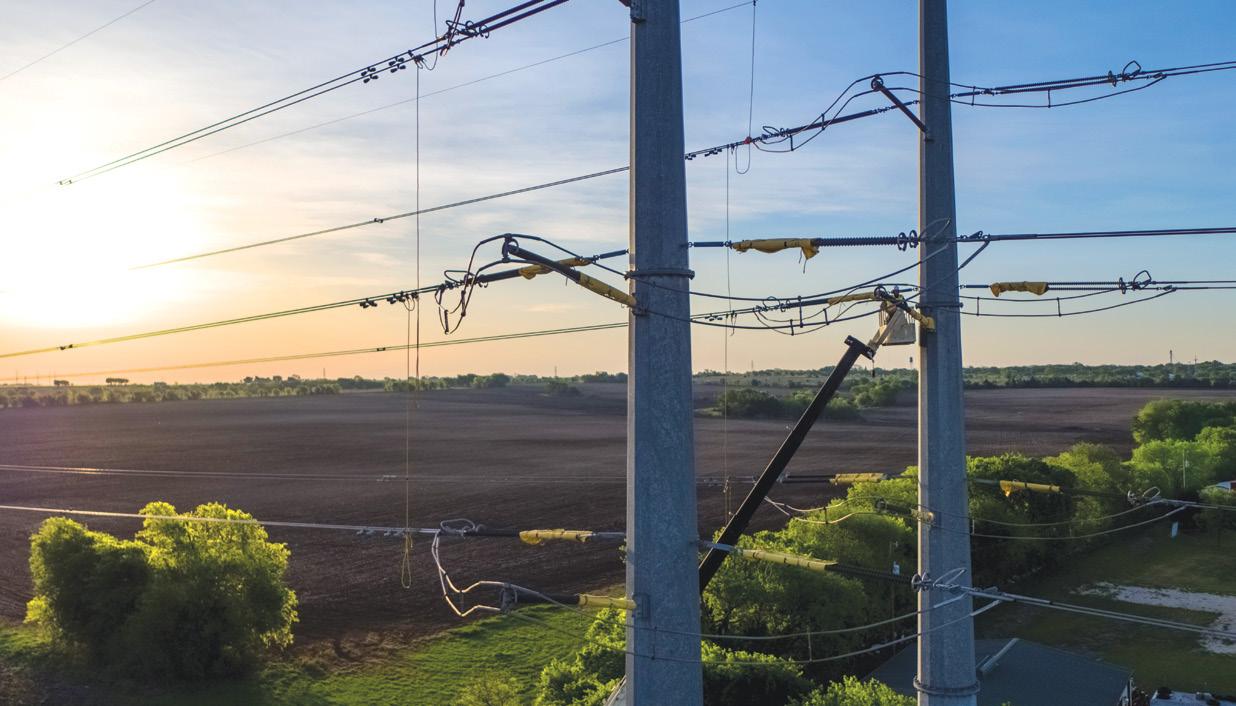
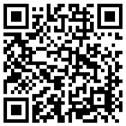
“Come gather ’round people wherever you roam and admit that the waters around you have grown and accept it that soon you’ll be drenched to the bone. If your time to you is worth savin’, then you better start swimmin’, or you’ll sink like a stone for the times they are a-changin’.” - Bob Dylan
Times in the electric utility world seem to be more rapidly a-changin’ than ever before. Why you may ask, do I mention the electrical utility industry in STRUCTURE magazine? While it is obvious that Electrical Engineers are the beating heart (at 60 Hz) of the electric power grid, Civil and Structural Engineers add the bones and create the muscle that helps give the grid its strength, durability, and, dare I add, resiliency? The challenges facing the electric power grid are unique and unprecedented. As the Bob Dylan refrain elaborates, the waters of change are growing and we... better start swimmin,’ or you’ll sink like a stone!
Currently, there is much swimming like crazy to keep up with the changing times in this industry. I was honored to serve on ASCE’s Committee on America’s Infrastructure this past cycle. This Committee publishes a detailed progress report on various infrastructure segments every 4 years through its Infrastructure Report Card. The report card grade for the Energy Sector was raised last year from a D+ to a C-. The sector recommendations came with an acute recognition that the electrical power grid (not just in the USA but globally) has rapidly become the most critical of all societal infrastructures due to an increasing interdependency on electricity to function effectively. Disrupt the power for even a few days and see how long it takes before devastating effects are experienced. We only need to look back at the aftermath of recent extreme weather events like Hurricanes Katrina, Ike, Harvey, Ida, Sandy, etc., that significantly impacted the electrical grid to understand what a loss of electrical power means to civil society. Or ask the folks in Puerto Rico following Hurricanes Irma and Maria. Or study and report on the events leading to a near catastrophic grid collapse in Texas due to Winter Storms Uri and Viola as I did along with an incredibly talented group of Texas Section ASCE folks. Electricity ranks right up there with food, water, and shelter as a societal must-have.
To support improvement in structural reliability, ASCE/SEI is leaning in to develop critically essential and needed Standards and Manuals of Practice for the design and use of structures and foundations supporting the electrical power grid. This ensures that technical competence for the design, construction, and ongoing maintenance of these unique structural systems is continuously and professionally updated to meet the growing challenges in these rapidly changing times. One of the
most important activities currently underway is the upgrade of the ASCE Manual of Practice No. 74 to a critically important new ANSI /ASCE Standard that will provide minimum loading criteria for the design of overhead powerline structures. It is a delicate but necessary task to balance current structure design loading practices diligently and effectively with new considerations to anticipate and mitigate the increasing impact of future severe weather events on our electrical grid. And finally, ASCE/SEI has been an active and vocal advocate for nurturing and educating the civil/structural engineering professionals that contribute to making the electrical grid more reliable, durable, and, yes, more resilient well into the future. In October in Orlando, ASCE/SEI’s Committee of Electrical Transmission Structures (ETS) will host a specialty technical conference that has become globally recognized as a go-to event. The ETS Conference facilitates sharing knowledge and innovation for reliable and resilient design and construction of the structures and foundations that are the bone and muscle of the electric power grid – our transmission, distribution, and substation structures. See the SEI Update in this issue (page 54 ) for more information.
The pace of change we will witness over the next few years will simultaneously be impressive and terrifying. Are we preparing for the changes on the horizon, and are we ready to lead the way to ensure the strength, durability, and resiliency of our critical electric power grid? The answer is YES! However, bring plenty of towels! We might be doing a lot of swimming as well!■
Wesley J. Oliphant is Chief Technical Officer and Co-Founder of Exo Group, LLC. He is a Fellow and Life Member of ASCE, a charter member of SEI, and a Professional Engineer in Texas. Wesley serves as Chairman of ASCE/ SEI’s Committee of Electrical Transmission Structures, is a member of ASCE’s Committee of America’s Infrastructure, and recently served on Subcommittee 5 (Strengths and Loadings) of the National Electric Safety Code. Infrastructure
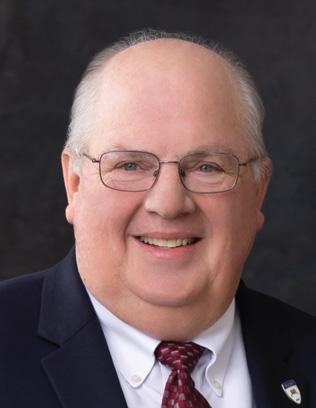
structural CONNECTIONS
Robust Anchorages to Concrete Structures
Anchor Channels in Industrial Structures, Bridges, and Tunnels
By Christoph Mahrenholtz, Ph.D., Donald F. Meinheit, Ph.D., P.E., S.E., and Nicholas Cekine III, P.E.Anchor channels with channel bolts enable the robust anchorage of components to reinforced concrete structures. State-of-the-art systems comprised of anchor channels and channel bolts, also commonly known as anchor channels, handle static, seismic, and fatigue loads in any direction. Installing components with channel bolts is fast and easy even under adverse conditions, where wind, water, and confined space entry restrict work activities, particularly with electrical equipment needed for welding, or when overhead installation may be too exhaustive for the installer due to long installation times.
History of Anchor Channels
Anchor channels are a success story that began over 100 years ago, triggered by the global spread of reinforced concrete in building structures. Originally, anchor channels were invented to fasten components to concrete in industrial structures, such as transmission belts suspended from the ceilings. Therefore, they were known as a means for suspending objects from reinforced concrete structures (Figure 1). Anchor channels consist of steel anchors (for example, round anchors, I-anchors, V-anchors, etc.) and C-channels, fabricated as one unit mostly by forging or welding. Anchor channels are cast flush in reinforced concrete elements and, after the concrete has reached its design strength, become a robust anchorage when utilized with matching channel bolts, known as T-bolts.
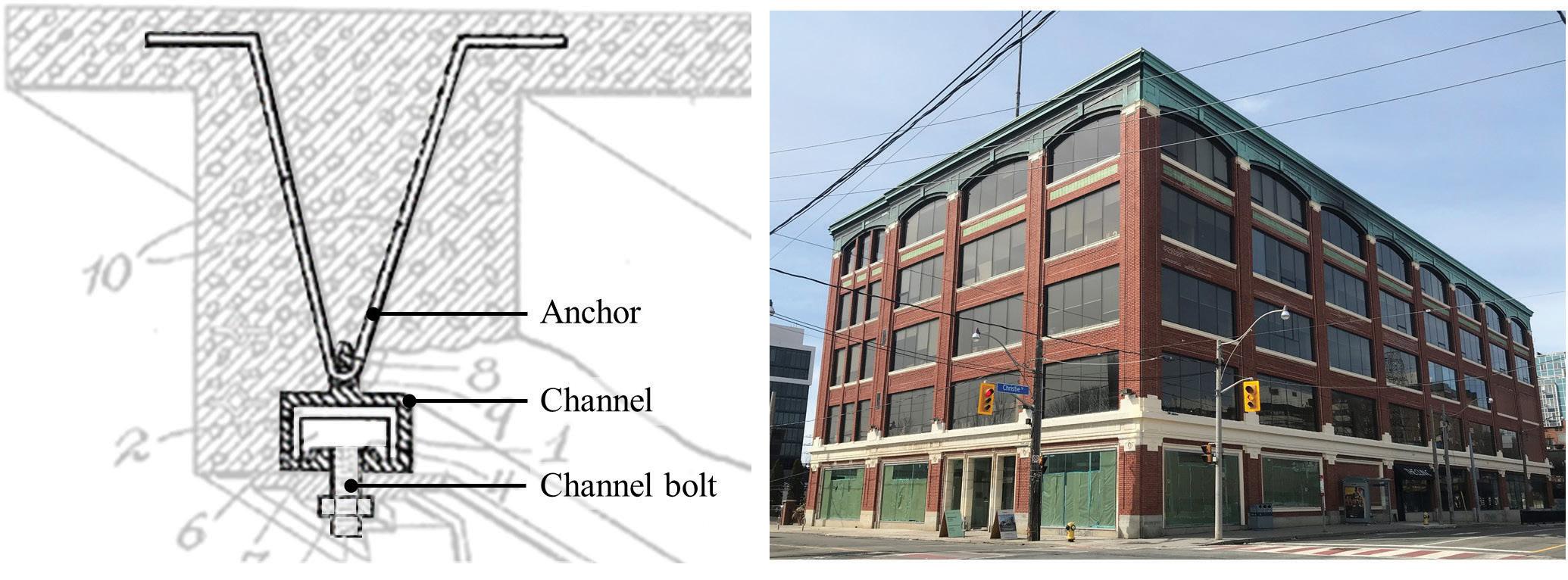
Installation
The installation of anchor channels and channel bolts is a simple procedure (Figure 2). First, the anchor channel is attached with nails or screws to the wood formwork before concrete placement. The anchor
channels contain a removable filler material that prevents concrete from entering the channel. After the concrete has hardened and the formwork is stripped, the filler material is easily removed. Next, channel bolts are inserted into the slot of the anchor channel and turned until they stop – one edge of the channel bolt head is slightly larger than the internal clear spacing between the channel walls, preventing the bolt from spinning inside the channel. The head of the channel bolt is then 90° to the direction of the anchor channel. The channel bolt can be positioned anywhere along the slot, enabling the installation of components at the exact point where needed.
When steel formwork is used, often in bridge and tunnel construction, blind rivets (also known as pop rivets) with special installation cones are used to attach the anchor channel. This attachment method ensures that the anchor channels do not shift during placement and consolidation of the concrete. The anchor channel may be connected to an electrical grounding system before the concrete placement. Thus, any component mounted using channel bolts can be electrically grounded. Anchor channels with channel bolts have further benefits:
• Load transfer in any direction due to mechanical interlock (between bolt and channel as well as between anchor and concrete).
• Tolerance compensation by adjusting the position of the channel bolt along the anchor channel.
• Minimal training is required for installation of the anchor channel and channel bolt.
• A simple mechanical connection option avoids costly field welding, which often comes with quality and safety risks.
• No inaccurate and time-consuming drilling for anchoring, and thus, no silica dust and cutting of reinforcing bars.
• No external power sources or ventilation equipment is needed for installation.
• Provides immediate load-carrying capability.
• A long-established system allows for timely scheduling of anchor channels and channel bolts to the job site.
• Easy positional adjustment, maintenance, and future upgrades of attached components are straightforward.
• A sustainable construction methodology without expiration dates, yet 100% recyclable when the structure is demolished.
Applications
Over time, the vertical layout of early industrial reinforced concrete structures, characterized by a limited height of the individual story (Figure 1), morphed into a horizontal layout with a single, high story. Consequently, some equipment was best mounted in reinforced concrete columns and walls, increasing the application of anchor channels and channel bolts. The use of anchor channels has spread to all types of concrete structures. Today anchor channels are used in wastewater plants, water plants, dams, and power plants, as well as (supertall) high-rise buildings for the connection of curtain wall facade elements. Another important field of application is bridges and tunnels.
bolt in 1985. These bolts have teeth that ‘bite’ into the channel lip when installed and torqued as specified, generating notches and thus mechanical interlock. However, notching channel bolts have a high installation torque sensitivity: the required installation torque, T inst, for example, 130 pounds force-foot for a 5⁄8-inch channel bolt, is considerable. An installation torque accidentally reduced by 50% may almost cut the longitudinal load capacity by half (Figure 4 ). For this reason, channel bolts with serrated heads and anchor channels with matching serrated lips were developed in the 1990s. This connection system of serrated anchor channels with matching serrated channel bolts allows the transfer of significantly higher longitudinal loads by mechanical interlock because of their low installation torque sensitivity (Figure 4 ). The longitudinal load capacity is reduced by only about 5% if the applied installation torque was erroneously only 50%. Even if the bolt is just snug tightened, serrated channel bolts transfer significantly more than half of the original longitudinal load. Notching channel bolts, on the contrary, transfer virtually no longitudinal load without installation torque applied. Moreover, longitudinal displacements under longitudinal load are generally smaller for serrated channel bolts compared with notching channel bolts.
Design
Figure 3 shows example applications used for bridge and tunnel projects past and present. Anchor channels are produced at any length up to twenty feet and curved to any radius needed. Anchor channels and channel bolts are available in hot-dipped galvanized (HDG) carbon steel and stainless steel for installations exposed to highly corrosive environments. Some examples are bridges and tunnels exposed to de-icing salts or high ambient salinity along coastal areas. The application possibilities are innumerable – for example, installations in prestressed concrete spun catenary poles used for railway power lines or precast segments used for lining of bored tunnels. Solutions are available to ensure that tunnel precast segments with cast-in anchor channels can be handled with vacuum lifters which are devices to hoist using suction cups.
Longitudinal Load Transfer
Initially, anchor channels were designed to be loaded in pure tension, N, only. However, most connections are also loaded in shear perpendicular to the channel axis, Vy. In many applications, the connection is also loaded in shear along the axis of the channel, Vx (Figure 4, page 10). By tightening a conventional channel bolt against the anchor channel, a frictional force from the clamping allows limited longitudinal loads. The need for a robust axial load capability initiated the invention of the notching channel
Anchor channels and channel bolts are designed like concrete anchors using design requirements codified in the American Concrete Institute (ACI) 318, Building Code Requirements for Structural Concrete and Commentary. Additional design provisions are given in AC232, Acceptance Criteria for Anchor Channels in Concrete Elements, published by the International Code Council Evaluation Service (ICC-ES). ACI 533.5, Guide for Precast Concrete Tunnel Segments, provides further advice for the use of anchor channels in tunnels. The design of anchor channels and channel bolts is complex and requires product-specific data from a qualification testing protocol. The design procedure involves the calculation of twenty possible failure mode capacities and their interaction. Any failure mode can control the design; that is, the lowest calculated capacity determines the design capacity.

Figure 2. Easy and reliable installation procedure. continued on next page
Figure 3. Applications in bridges (left) and tunnels (right), where tension and shear loads have to be safely transferred into the concrete structure for a design life of 100 years and beyond.
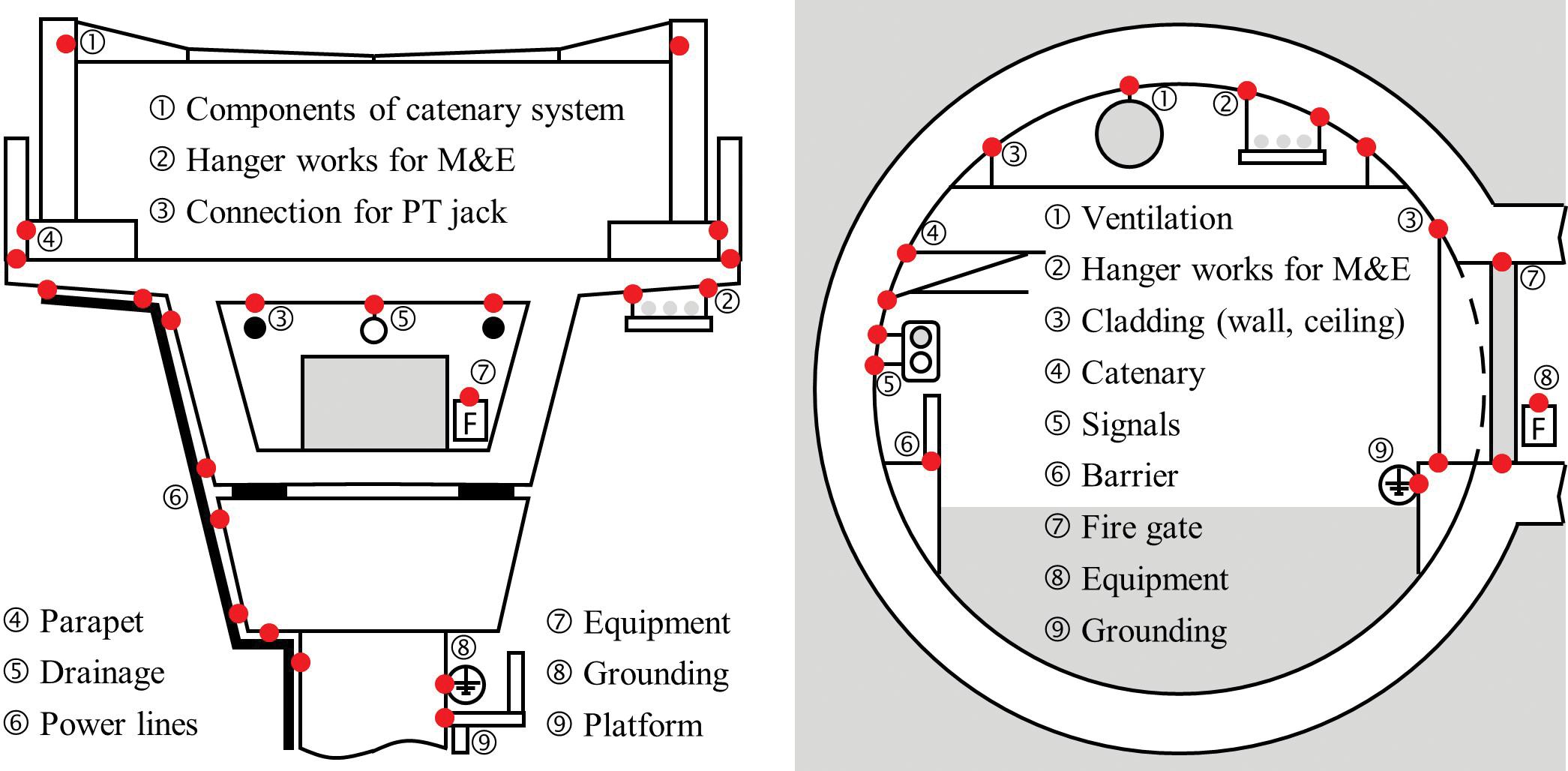
Proprietary design software (Figure 5 ), developed to facilitate extensive calculations, enables the engineer to carry out the structural analysis of qualified products thoroughly and efficiently. Typically, the design software, e.g. JORDAHL® EXPERT, also supports the analysis of anchor channel pairs, often used to fix baseplates with four channel bolts (Figure 5 ). In addition, interfaces allow the data exchange between the design software and Building Information Modeling (BIM) software. This is important since BIM has become more critical and sometimes mandatory, particularly for infrastructure projects.
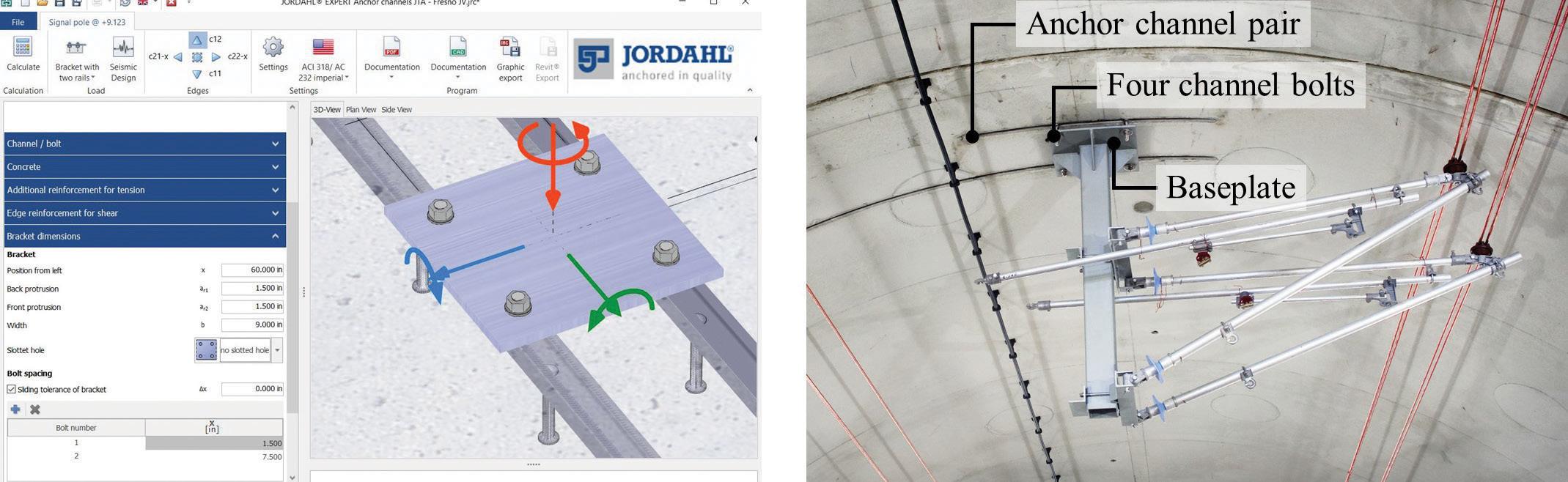
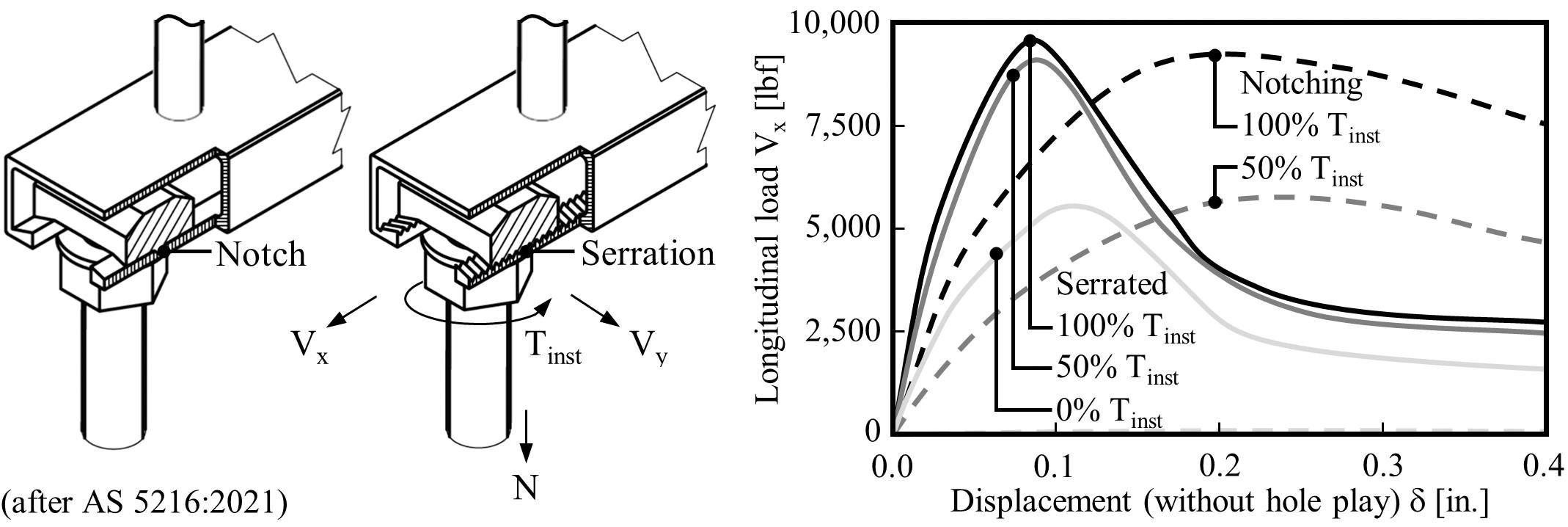
Qualification
AC232 is primarily a guideline providing acceptance criteria for qualification testing of anchor channels to receive an Evaluation Service Report (for example, ESR-2854), containing the product-specific data required for its design. While static qualification is standard, seismic qualification is optional but typically required for important infrastructure projects like bridges and tunnels – even in regions of low seismicity. Anchor channels can be seismically qualified only if they can transfer load in the longitudinal axis of the anchor channel by mechanical interlock. Note that compression loads normal to the concrete surface are taken by direct load transfer from the baseplate. Tension and shear load tests are conducted on anchor channels cast in concrete test members for qualification testing. Test members are unreinforced to evaluate the load performance for the worst-case scenario. Apart from additionally placed anchor reinforcement, any steel reinforcement is neglected when calculating the design load capacity of the connection. For this reason, replacing the steel reinforcing bars with carbon fiber (CFRP) or glass fiber (GFRP) bars, used to prevent magnetic interference and accelerated corrosion
of embedded steel reinforcement, does not influence the calculated design load. Moreover, research has shown that the load performance of anchor channels cast in fiber reinforced concrete (FRC), popular for tunnel segments, is better than for anchor channels cast in conventionally reinforced concrete. Therefore, anchor channels cast in FRC can be designed using the same design assumptions.
The fatigue and fire performance of concrete anchors, including anchor channels, is not mandatory though may be relevant, particularly for applications in bridges and tunnels. The fatigue and fire qualification of concrete anchors is not fully regulated yet but can be tested (Figure 6 ) and reported if required.
Fatigue assessment may be critical for some connections at bridges and tunnels because of the repeating structural vibrations and pressure waves. Fatigue assessment of anchor channels and channel bolts in shear requires that the gap between the anchor channel and channel bolt and the gap between the channel bolt and baseplate is filled with adhesive to reduce the stress cycle. To this end, special gap filler sets have been developed, including a special nut for the injection and an insert to be installed together with the channel bolt before mounting the baseplate. The fatigue performance depends on the geometry, for example, at the joining of anchor and channel, and on the material selection and production technology. The fatigue strength of a poorly engineered anchor channel may be only a fraction of another anchor channel of the same size and static strength.
Fire rating of concrete anchors has recently moved into focus because fastened components are often relevant to fire safety. For fire rating testing, anchor channels are cast into the underside of a slab which forms the cap of a fire furnace. After dead load weights are connected to the channel bolts, the fire furnace is typically heated according to a standard temperature curve (STC), as defined in the International Standard ISO 834-1, Fire-Resistance Tests – Elements of Building Construction – Part 1, General Requirements (almost 2000°F after 120 minutes), which serves as an alternative to the ASTM E119, Standard Test Methods for Fire Tests of Building Construction and Materials. The time-to-failure is measured for a simplified yet conservative evaluation method to define design strengths as a function of required fire resistance time.
Summary
Anchor channels with their channel bolts are an iconic fastening system for heavy and sustained static, and seismic and fatigue loads. Only products with stringent, independent third-party evaluations according to the AC232 acceptance criteria have a recorded Evaluation Service Report (ESR) issued by the ICC-ES. e ESRs certify their suitability for static and seismic applications. AC232 amends the provisions for concrete anchors stipulated in the ACI 318 reinforced concrete code to ensure that the system can be designed according to the state-of-the-art. Free design software can help the structural engineer design thoroughly and efficiently. Properly
designed, qualified anchor channels with channel bolts allow robust anchoring in concrete structures, including bridges and tunnels.■
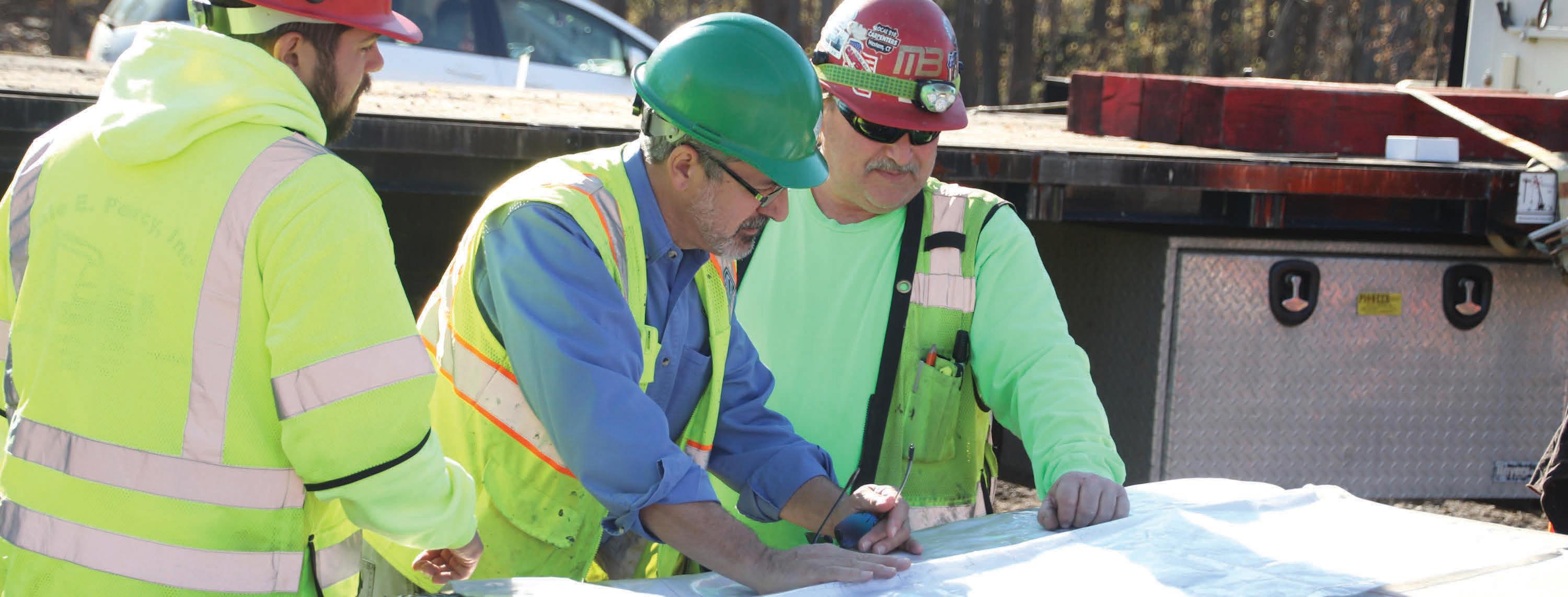
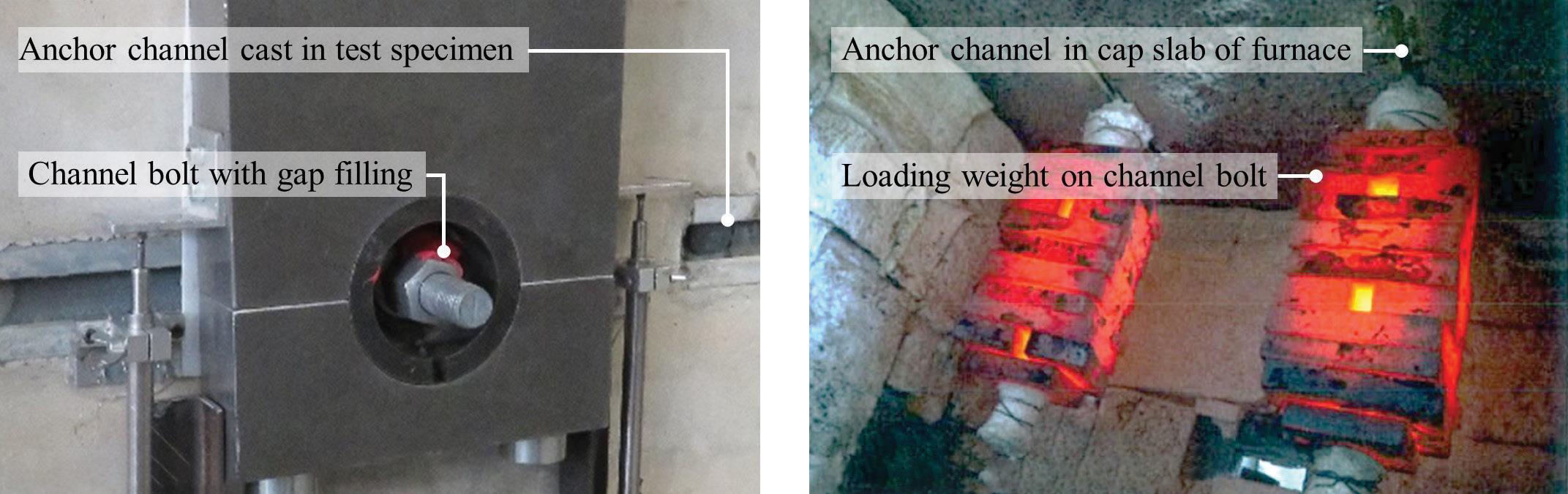
Christoph Mahrenholtz is the Head of Engineering at JORDAHL Germany (christoph.mahrenholtz@jordahl.de).
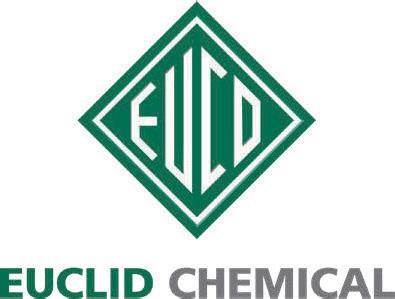
Donald F. Meinheit, retired from Wiss, Janney, Elstner Associates –Chicago, is the past chair of ACI 355 – Anchorage to Concrete and a member of ACI 318B – 318 Subcommittee B – Anchorage and Reinforcement. (dmeinheit@wje.com).
Nicholas Cekine III is a Senior Structural Engineer at JORDAHL USA (nick.cekine@jordahlusa.com).
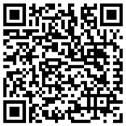
Ductile Coupled Reinforced Concrete Shear Wall System
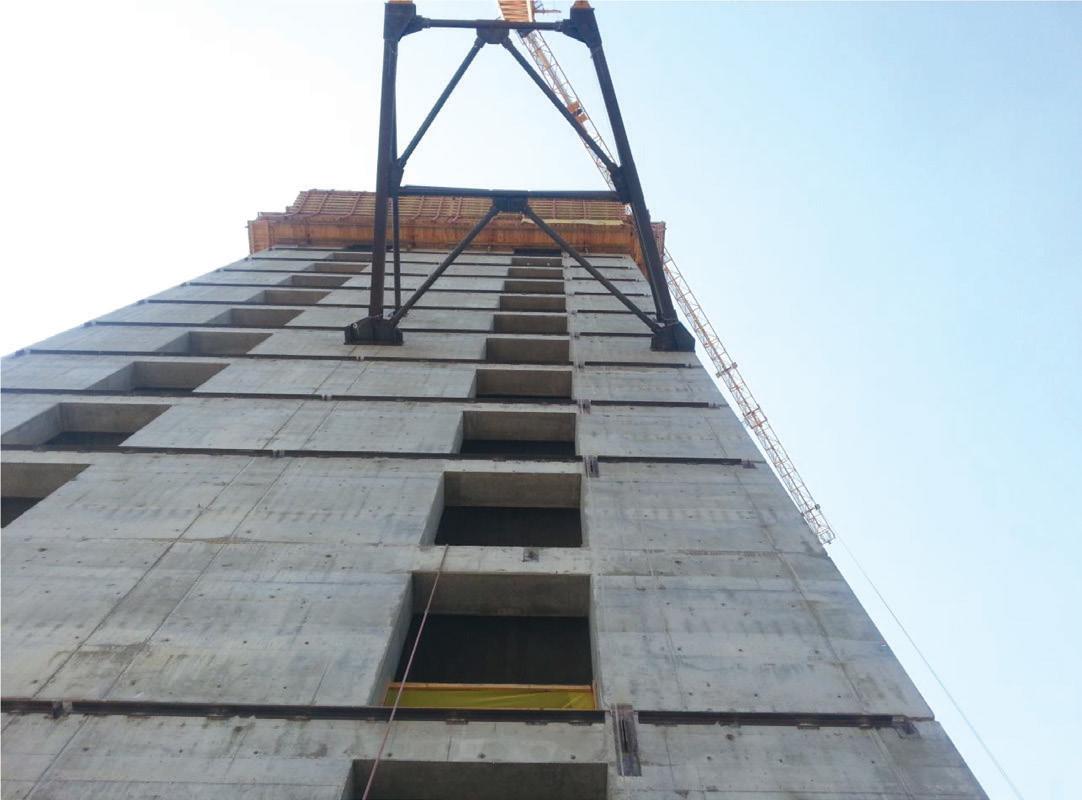
Per ACI 318-19 and ASCE 7-22
By S. K. Ghosh, Ph.D.This article provides background on the recognition of ductile coupled shear wall systems of reinforced concrete in ASCE 7-22 Minimum Design Loads and Associated Criteria for Buildings and Other Structures (ASCE 2022) Table 12.2-1, Design Coefficients and Factors for Seismic Force-Resisting Systems. The system itself is defined in ACI 318-19 Building Code Requirements for Structural Concrete (ACI 2019).
Reinforced concrete shear walls are commonly used in buildings for functional as well as structural reasons. Functionally, shear walls are useful in buildings because they serve as partitions between spaces. Structurally, they make buildings laterally stiff, particularly when used interactively with moment frames, thereby helping to keep lateral deflections within desirable or tolerable limits.
Often, such walls are pierced by numerous openings for windows, doors, and other purposes. Two or more walls separated by vertical rows of openings, with beams at every floor level between the vertically arranged openings, are referred to as coupled shear walls. When a coupled shear wall system is subject to lateral wind loads or earthquake forces, shear forces are generated at the ends of the coupling beams. These accumulate into a tensile force in one of the vertical wall segments and a compressive force in the other vertical wall segment. Due to these tensile and compressive forces, the couple resists a part of the overturning moment at the base of the wall system, leaving the remainder of the overturning moment to be resisted by the vertical wall segments themselves (Figure 1).
The ratio of the overturning moment resisted by the tension-compression couple to the total overturning moment at the base of the
Figure 1. A coupled shear wall system.
coupled wall system is often referred to as the degree of coupling. The shorter and deeper the coupling beams, the higher the degree of coupling. When the degree of coupling is very low, the two vertical wall segments tend to behave like individual isolated walls. When the degree of coupling is very high, the entire coupled wall system tends to behave like a shear wall with openings. However, it should be noted that as inelastic displacements develop in the coupling beams, the degree of coupling tends to lose its significance.
An example of a coupled shear wall system is shown in Figure 2. The vertical wall segments are often referred to as wall piers. This article avoids this terminology because “wall pier” is a defined term in ACI 318. Many vertical wall segments forming part of a coupled shear wall do not satisfy the ACI 318 definition of wall pier.
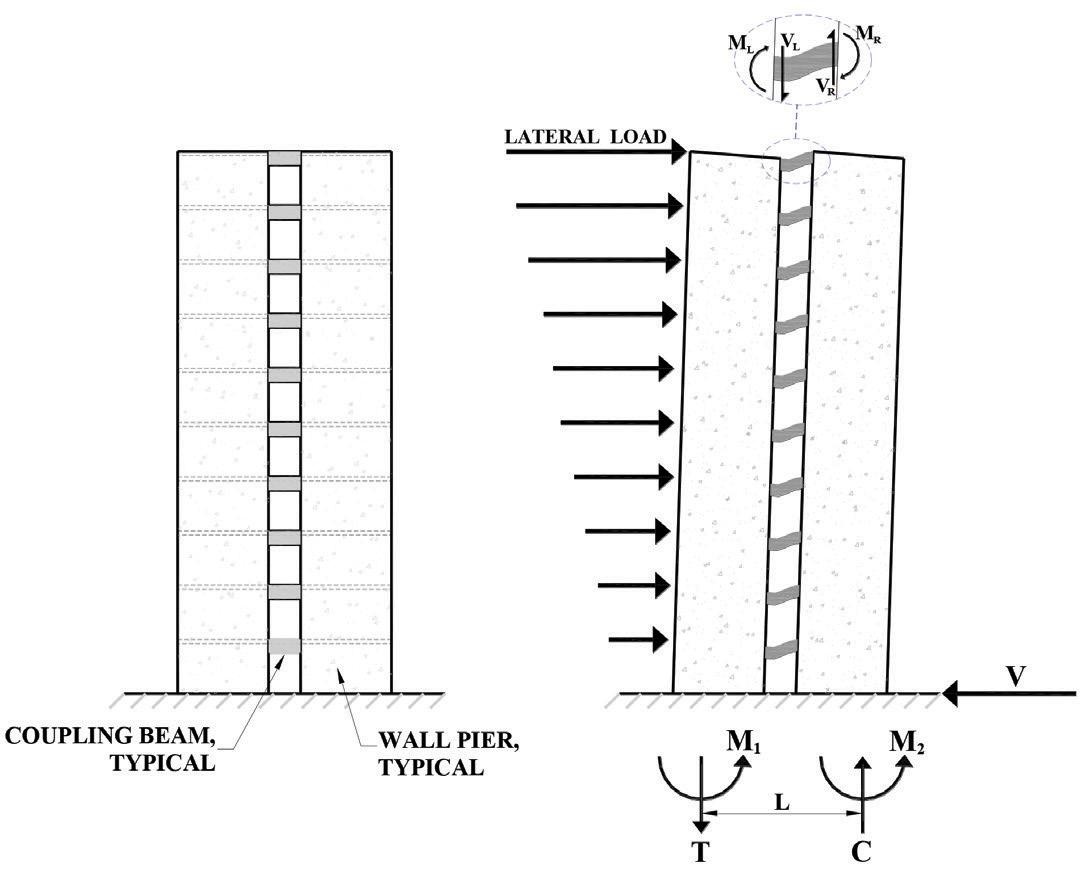
A coupled shear wall system can be designed to dissipate a considerable amount of earthquake energy in the coupling beams before flexural hinges form in the walls. This typically occurs at the bases of slender vertical wall segments with height-to-length ratios larger than or equal to two. Energy dissipation in coupling beams can be by shear yielding if their span-to-depth ratios are low. Flexural yielding at the ends of coupling beams occurs if their span-to-depth ratios are larger.
Although such coupled wall systems are highly suitable as the seismic force-resisting systems of multistory buildings, they were not recognized as distinct entities in Table 12.2-1 of ASCE 7 through its 2016 edition. Therefore, such systems needed to be designed using R-values that essentially ignored the considerable benefits of having the coupling beams, which can dissipate much of the energy generated by earthquake excitation. This article reports on a successful effort to remedy this situation.
Coupled shear wall systems are recognized as distinct from isolated shear wall systems in Canadian (CSA Group 2014) and New Zealand (Standards New Zealand 2006) standards. They are also accorded higher response modification factors, given their superior seismic performance.
ACI 318-19 Provisions
According to Bertero (1977): “Use of coupled walls in seismic-resistant design seems to have great potential. To realize this potential, it would be necessary to prove that it is possible to design and construct ‘ductile coupling girders’ and ‘ductile walls’ that can supply the required strength, stiffness, and stability and dissipate significant amounts of energy through stable hysteretic behavior of their critical regions.”
Thus, the discussion needs to focus on not just coupled walls but on ductile coupled walls consisting of ductile shear walls and ductile coupling beams
In the 2019 edition of ACI 318, a new system definition has been created to recognize the Ductile Coupled Structural (Shear) Wall (DCSW) system. The shear walls in such a system must be special structural walls in conformance with ACI 318-19 Section 18.10, and the coupling beams must comply with the detailing requirements in ACI 318-19 Section 18.10.7. There are additional important considerations.
The performance objective of the ductile coupled shear wall system is for the majority of energy dissipation to occur in the coupling beams. This is analogous to strong-column/weak-beam behavior in moment frames. Studies were conducted by Magnusson Klemencic Associates (MKA) to identify system characteristics that lead to coupling beam energy dissipation of no less than 80% of total system energy dissipation under MCE ground motions.
In these studies, nonlinear response history analyses were carried out using spectrally matched ground motion records on various coupled shear wall archetypes. Archetypes ranged from 5 to 50 stories in height and considered a range of longitudinal reinforcement ratios in the coupling beams and the shear walls. The results of these analyses are presented in Figure 3. The x-axis represents the aspect ratio (clear span-to-total depth) of the coupling beams. D designates a diagonally reinforced beam design, and M designates a moment frame beam design. The quantity on the y-axis is the percentage of total system energy dissipation in the coupling beams alone. The resulting trend
shows the coupling beams dissipating the majority of system energy between aspect ratios of 2 and 5.
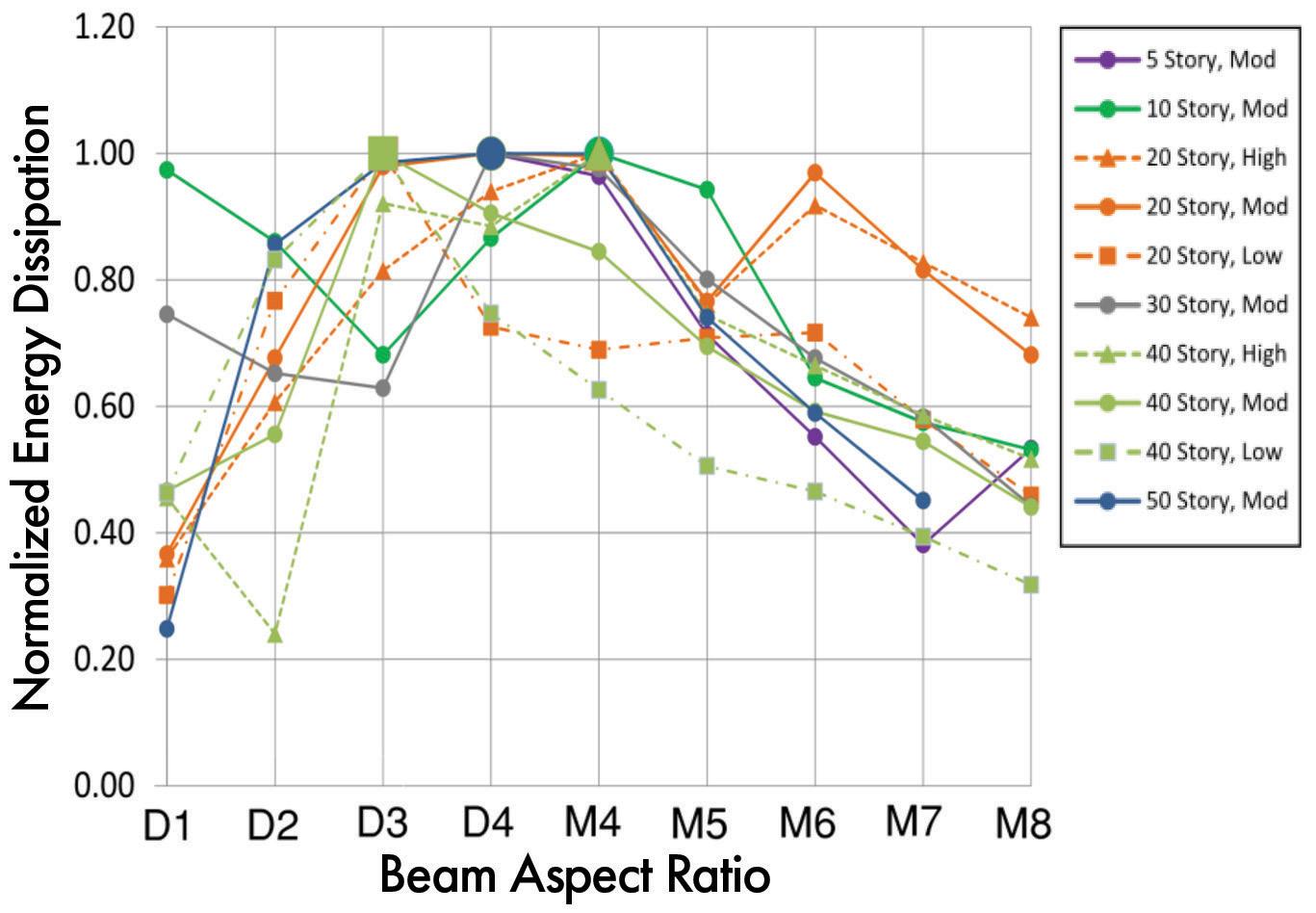
The primary characteristics of a ductile coupled shear wall system were found to be governed by geometry. Squat walls were too stiff to allow sufficient story drift for coupling beams to become inelastic. For this reason, shear walls in the DCSW system need a total heightto-length aspect ratio of no less than 2.0. Squat coupling beams were found to over-couple the seismic force-resisting system, leading to significant energy dissipation in the shear walls. As such, coupling beams in DCSW systems need to have length-to-total-depth aspect ratios of no less than 2.0 in all cases.
Very slender coupling beams, designated as having aspect ratios greater than 5.0, are too weak to contribute sufficient hysteretic energy dissipation and are allowed in no more than 10% of the levels of the building. Lastly, coupling beams conforming to these geometric constraints must be present at all levels and are required to develop 1.25fy at each end to dissipate the intended amount of energy. This last
is intended to preclude
fixed-pinned coupling beams that have been utilized where insufficient length exists to develop the coupling beam reinforcement into the adjacent shear wall.
ACI 318-19, Section 2.3 – Terminology, defines structural wall, ductile coupled as a seismic force-resisting-system complying with Section 18.10.9. The requirements discussed above are found in Section 18.10.9.
ASCE 7-22 Provisions
Issue Team (IT) 4 of the Provisions Update Committee (PUC) of the Building Seismic Safety Council (BSSC) developed a proposal to add three line items to ASCE 7-16 Table 12.2-1, featuring the ductile coupled wall system of reinforced concrete (see Table, page 13). The line items are under A. Bearing Wall Systems, B. Building Frame Systems, and D. Dual Systems with Special Moment Frames. Based on a FEMA P-695 study (FEMA, 2009), R = 8, Cd = 8, and Ωo = 2.5 were proposed for all three line items. The height limits are the same as for corresponding uncoupled isolated wall systems. A minimum height limit of 60 feet is imposed on seismic force-resisting systems featuring ductile coupled walls because this system is simply not efficient for low-rise multistory buildings.
FEMA P695 Studies
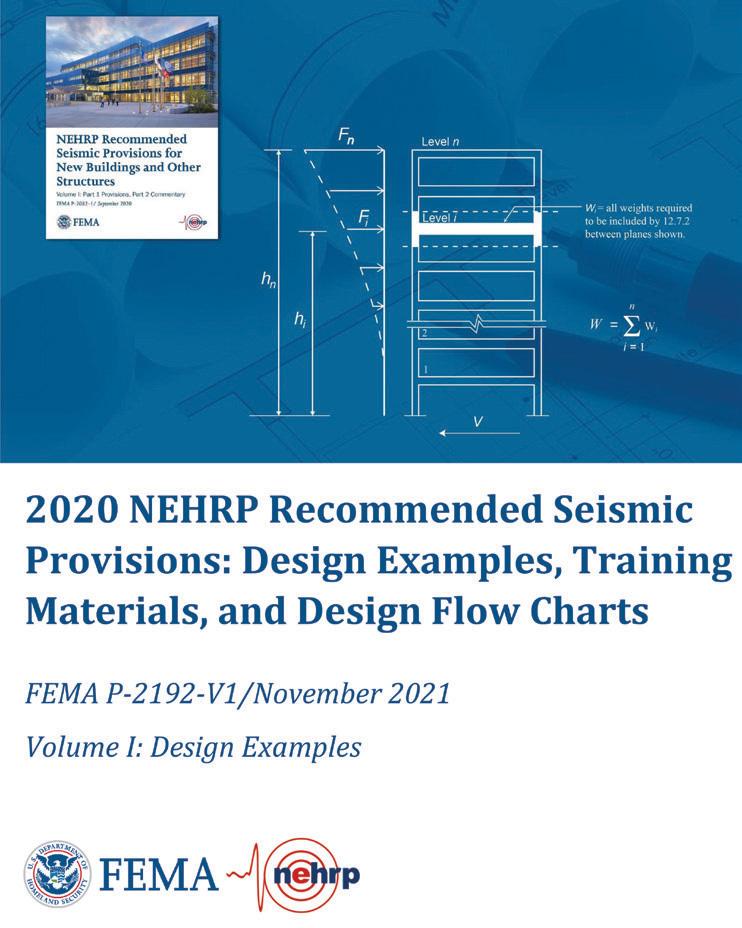
The proposed response modification factors for seismic force-resisting systems featuring reinforced concrete ductile coupled shear walls were validated (Tauberg et al., 2019) using the FEMA P695 methodology. A series of forty-one ductile coupled shear wall buildings were designed using a range of variables expected to influence the collapse margin ratio, with the primary variables being building height (i.e., 6, 8, 12, 18, 24, and 30 stories), wall cross-section (i.e., planar and flanged walls), coupling beam aspect ratio
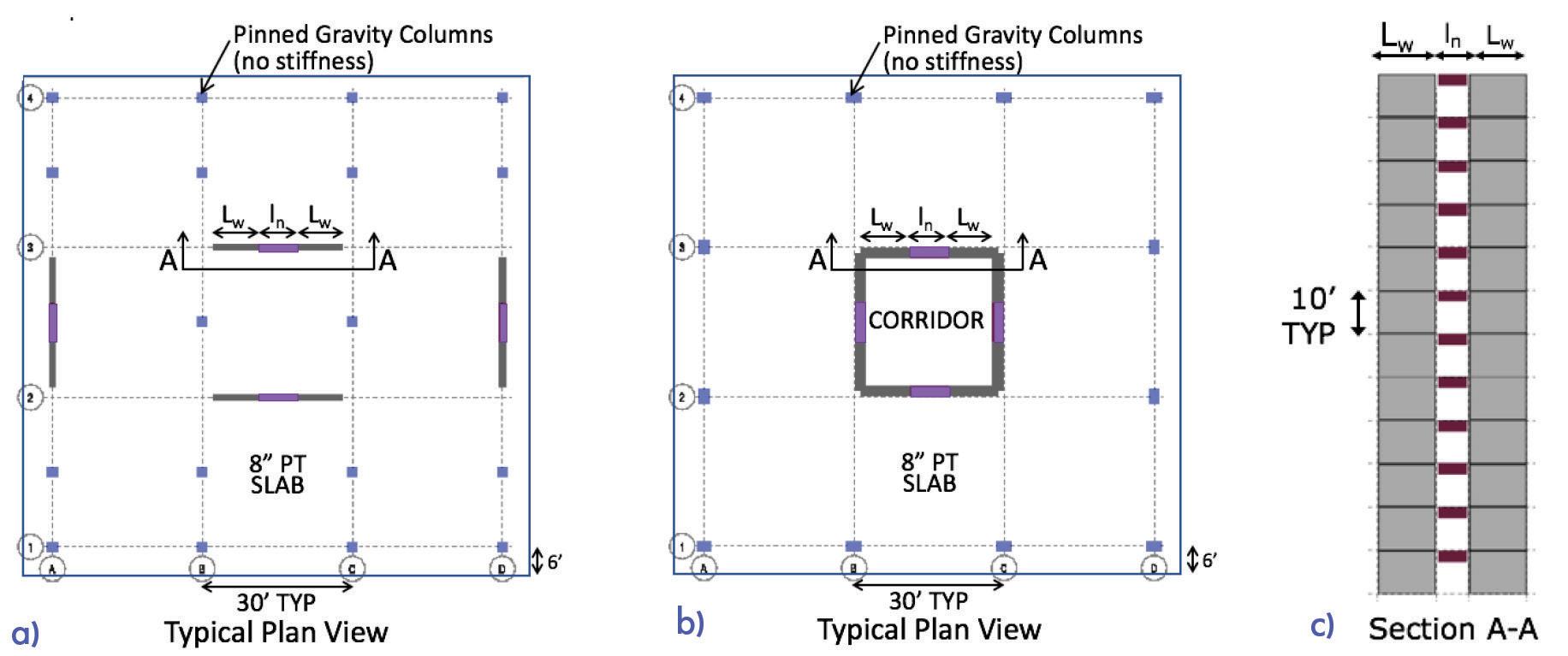
(ln/h) ranging from 2.0 to 5.0, and coupling beam reinforcement arrangement (i.e., diagonally and conventionally reinforced).
There have been four significant ACI 318-19 changes, all adopted in the FEMA P695 study:
1) 18.10.3.1 (shear amplification) – would typically require design shear (required shear strength) Vu to be amplified by a factor of up to 3 (similar to New Zealand, Canada).
2) 18.10.6.4 – requires improved wall boundary and wall web detailing, i.e., overlapping hoops if the boundary zone dimensions exceed 2:1, crossties with 135-135 degree hooks on both ends, and 135-135 degree crossties on web vertical bars.
3) 18.10.6.2(b) (Check on mean top-of-wall drift capacity at 20% loss of lateral strength) – requires a low probability of lateral strength loss at MCE-level hazard.
4) 18.10.2.4 – requires minimum wall boundary longitudinal reinforcement to limit the potential of brittle tension failures for lightly reinforced walls.
For details on these important changes, reference can be made to Ghosh, Taylor (2021a, 2021b).
The range of variables was chosen considering those used to define a DCSW system in ACI 318-19. The resulting designs have the minimum wall area (length and thickness) required, which is governed by shear amplification and the requirement that walls sharing a common shear force not exceed a shear stress of 8√f ´ c Acv (where Acv = gross area of concrete section bounded by web thickness and length of section in the direction of shear force considered and f ´ c = the specified compressive strength of concrete).
Typical floor plans and a wall elevation view are presented in Figure 4.
A conservative approach was used to define collapse for the FEMA P695 study, i.e., the collapse was taken as a 20 percent drop in lateral strength. This approach is conservative because the loss of axial load carrying capacity typically does not occur
until lateral strength drops more significantly, e.g., an 80 percent drop. In some studies, axial failure has been assumed to occur at a specified roof drift ratio, which has been typically 4 to 5 percent (Kircher et al. 2010), whereas, in this study, the conservative approach used resulted in roof drift ratios that were typically not more than 3 percent.
A system overstrength factor of Ωo = 2.5 is proposed based on nonlinear static pushover analysis results indicating that the mean overstrength values of the performance groups range from 1.31 to 2.13. e proposed response modification factor, R = 8, was validated based on incremental dynamic analysis results indicating that the mean Adjusted Collapse Margin Ratio (Tauberg et al. 2019) values of the performance groups ranged from 1.99 to 2.84, corresponding to collapse probabilities of less than ten percent, based on using a conservative definition of collapse as noted above. e deflection amplification factor of Cd = 8 is proposed based on damping considerations and the assessment of median roof drift responses from design level earthquakes compared to design roof drifts. Overall, the results of this study suggest that an overstrength factor Ωo = 2.5, a response modification factor R = 8, and a deflection amplification factor Cd = 8 are appropriate seismic design parameters for RC Ductile Coupled Wall systems that are designed per ASCE 7-22 and ACI 318-19 provisions.
Design Example
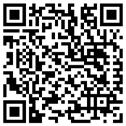


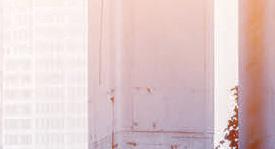
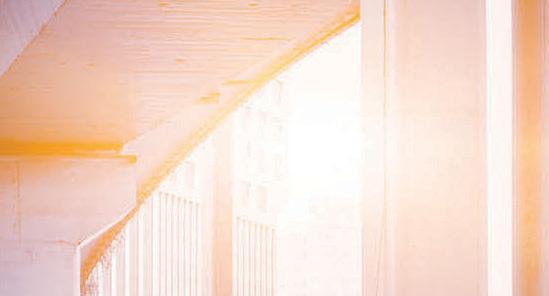
A 22-story reinforced concrete residential building is designed (Ghosh 2021) following the requirements of ASCE/SEI 7-22 and ACI 318-19 in the recent FEMA Publication P-2192, 2020 NEHRP Recommended Seismic Provisions: Design Examples, Training Materials, and Design Flow Charts (Figure 5 ). e building consists of a flat plate-column gravity system with a central core formed by four reinforced concrete coupled structural walls acting as the seismic force-resisting system. e structural walls are designed as Ductile Coupled Reinforced Concrete Shear (Structural) Walls. is complete design example is expected to be a valuable resource to the practitioner.
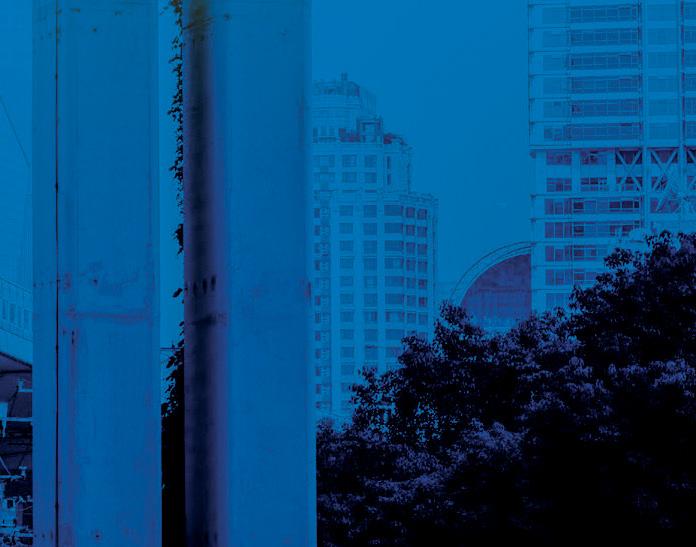
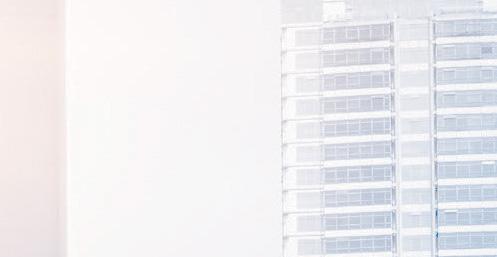
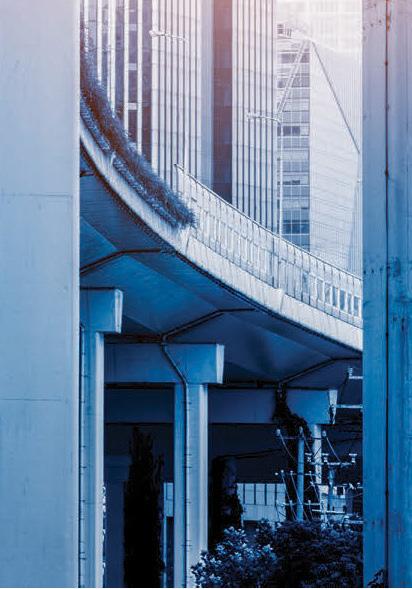
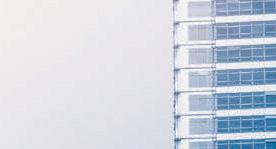
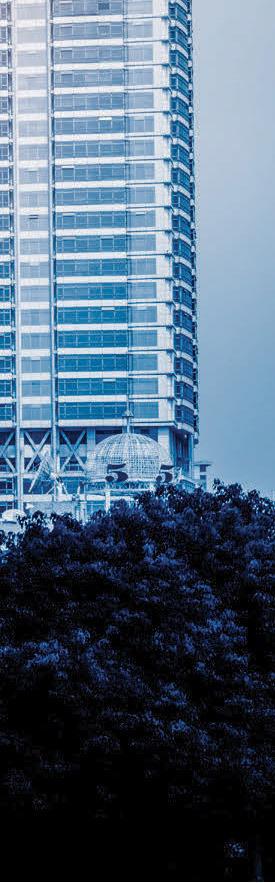
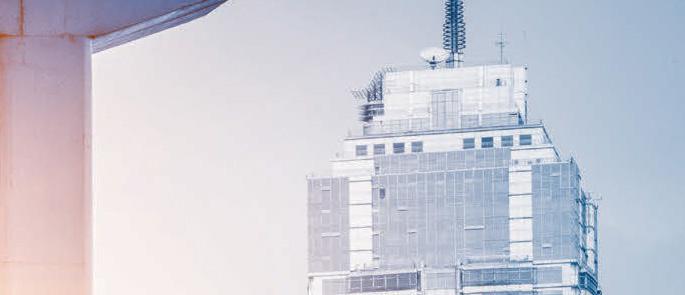
Conclusion
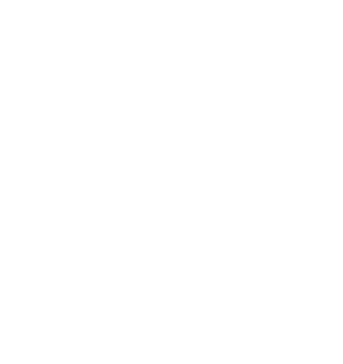
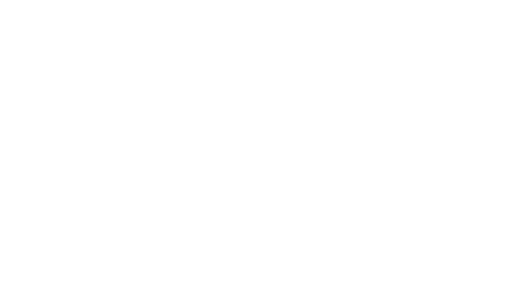
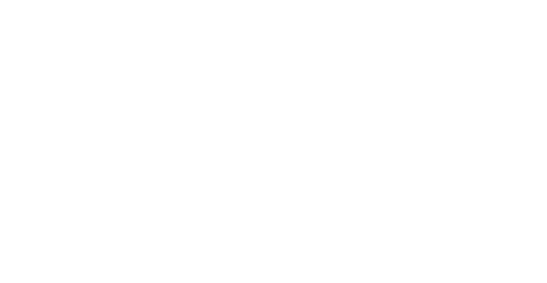
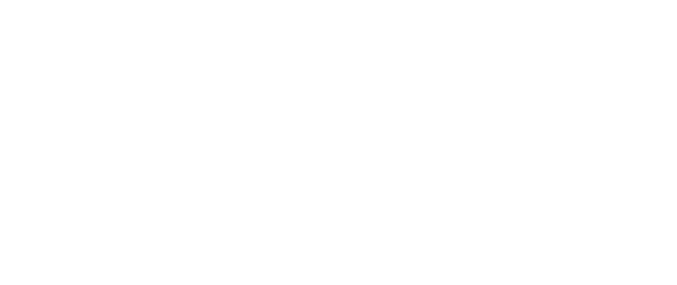
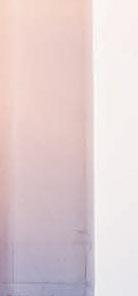
is article discusses the recognition of a ductile coupled concrete shear wall system as a distinct seismic force-resisting system in ASCE 7-22 Table 12.2-1. In addition, the ductile coupled concrete shear wall system is defined in ACI 318-19. is development should pave the way

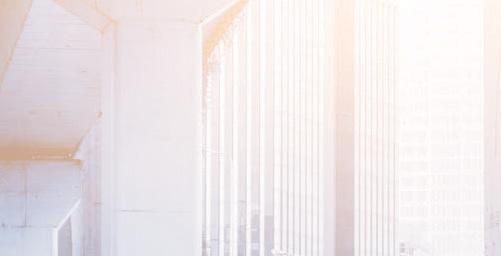
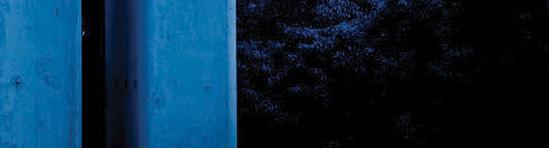
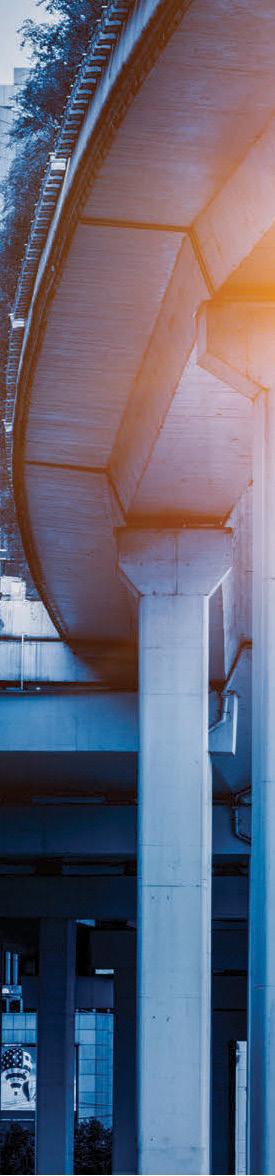

structural SUSTAINABILITY
Alternative Cements
Compliance Beyond the Code
By Mahmut Ekenel, Ph.D, P.E., FACIAs the most widely used manufactured material on the globe, concrete is one of the materials that may be considered part of society’s foundation. Concrete can be a highly durable, resilient, and affordable material in the built environment, supporting sustainable, economic, and social development when properly proportioned. In addition, concrete can be molded into nearly any shape at the job site, bringing versatility to construction and making concrete a highly popular construction material.
On average, over 4 billion metric tons of cement are produced yearly for concrete production. On the other hand, it is estimated that cement production is responsible for up to 8 percent of global greenhouse gas emissions among all such releases. A recent study showed that if the cement industry were a country, it would be the third-largest carbon dioxide emitter worldwide, topped only by the U.S. and China. Almost half of concrete’s CO2 emissions are created during the manufacture of cement clinker. Although energy demand in clinker production has been reduced over the last few decades, it is still the most-energy intensive part of the cement-making process. It requires heating a mixture of raw materials in a kiln to approximately 2642°F (1450°C) for modern portland cements. It is expected that future demand for concrete, fueled by population growth and the need for infrastructure rehabilitation and reconstruction, will continue to drive CO2 emissions from cement production higher.
CO2 emissions from cement, 2020. Source: Global Carbon Project, OurWorldinData.org
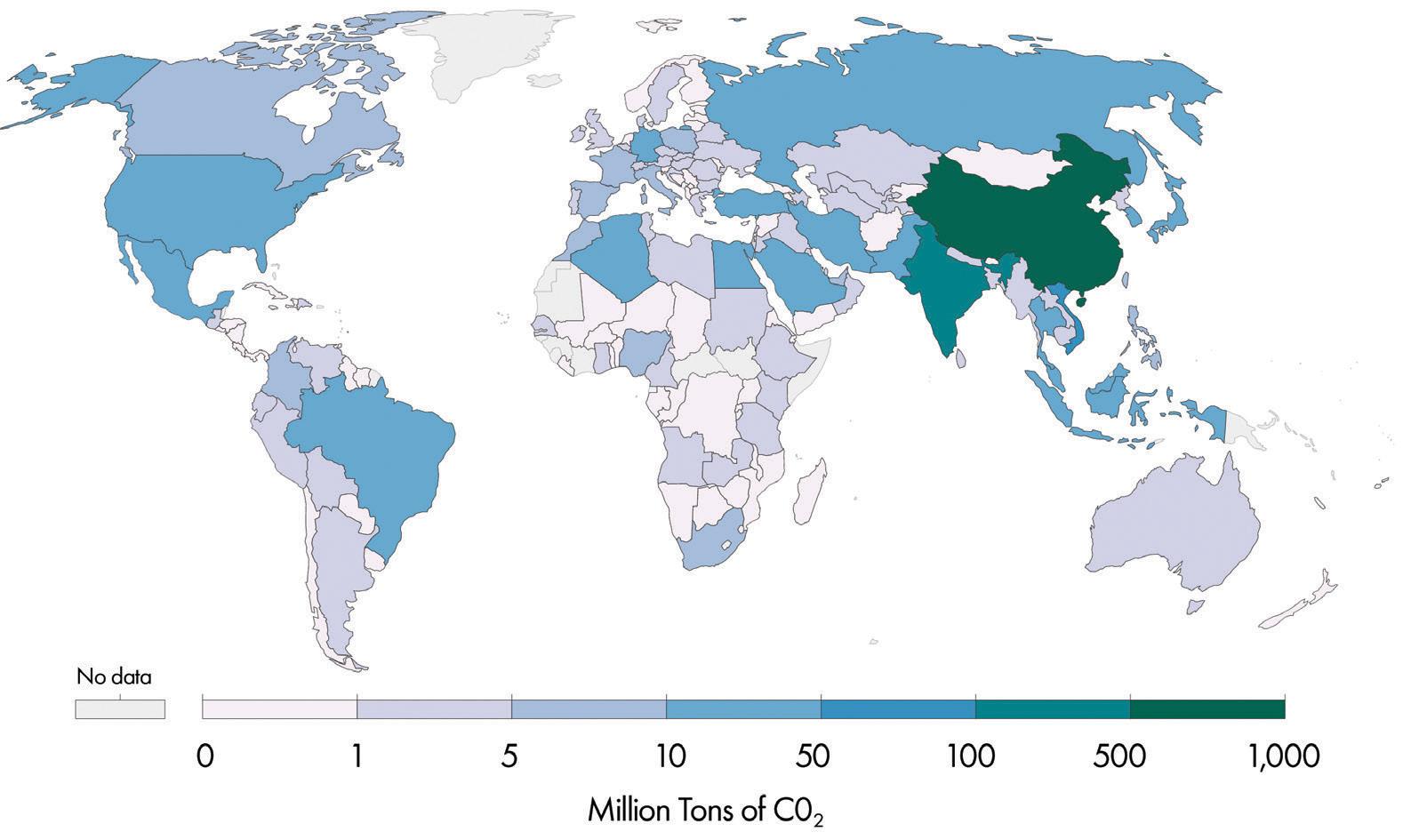
Motivated to break this linkage, alternative cements (also known as non-portland cements) with reduced CO₂ emissions compared to traditional building code-compliant cements are being developed and increasingly utilized in concrete in recent years. One of the major incentives came from the State of California, the world’s fifth-largest economy. The state of California signed groundbreaking legislation in September 2021 to zero-out carbon pollution from cement in their state construction. Therefore, California is currently trying to establish interim targets for reducing cement’s greenhouse gas intensity and developing a strategy to achieve net-zero greenhouse gas emissions associated with cement used within California by 2045. It is speculated that California’s initiative may even likely influence cement production and use in the rest of the nation, opening doors wider for low-carbon alternative cements.
One of the concrete industry’s significant questions for the use of alternative cements is building code compliance of such cements, partly because most have proprietary formulations that may not comply
with the building code requirements. Currently, the International Building Code (IBC), the predominant building code in the U.S. for new building construction, refers to the American Concrete Institute’s ACI 318-19, Building Code Requirements for Structural Concrete and Commentary, for code-specified cements, and does not contain provisions for alternative cements. ACI 318-19 has Section 26.4.1.1.1(a) for code-specified cements used in concrete (see Table). ACI 318-19 also has a new section that did not exist in prior editions (Section 26.4.1.1.1(b)) that allows the use of concrete made with alternative cements if they meet the performance requirements for the application, including “structural, fire, and durability.” Commentary to Section 26.4.1.1.1b refers to the ITG-10.1R guide, a report on alternative cements prepared by ACI Innovation Task Group 10. This report provides an extensive overview of alternative cements, case studies, and recommended testing methods. However, neither the code nor commentary of ACI 318-19 provides clear guidance about achieving building code compliance and acceptance conditions for evaluating structural, fire, and durability performance. Currently, ACI Committee 242 (Alternative Cements) is working on developing performance-based specifications for alternative cements, which may be considered for building code adoption in the future.
In addition, ASTM has published a standard for alternative cementitious materials, ASTM C1709 (Standard Guide for Evaluation of Alternative Supplementary Cementitious Materials (ASCM) for Use in Concrete). This standard has guidance with the intent of providing a technical approach to evaluating alternative supplementary cementitious materials such as pozzolans and hydraulic materials that fall outside the scope of code-specified standards such as ASTM C618, C989, and C1240. Although this standard provides good guidance, it
does not cover all alternative cements, is not referenced by the building codes, and does not provide full building code compliance. ASTM also has active working committees developing specifications for non-hydraulic and special cements. These ASTM specifications may also be considered for building code adoption in the future.
Building Code Compliance
In the United States, a system of model building codes is adopted by local jurisdictions, which have the authority to enforce construction regulations. The IBC is the predominant building code used throughout the United States to establish minimum requirements for new building construction. The IBC has been adopted in all 50 states, the District of Columbia, Puerto Rico, and the U.S. Virgin Islands. Furthermore, several other countries/jurisdictions, such as Saudi Arabia, Pakistan, and Abu Dhabi, use the IBC as a reference code to develop their national building codes.
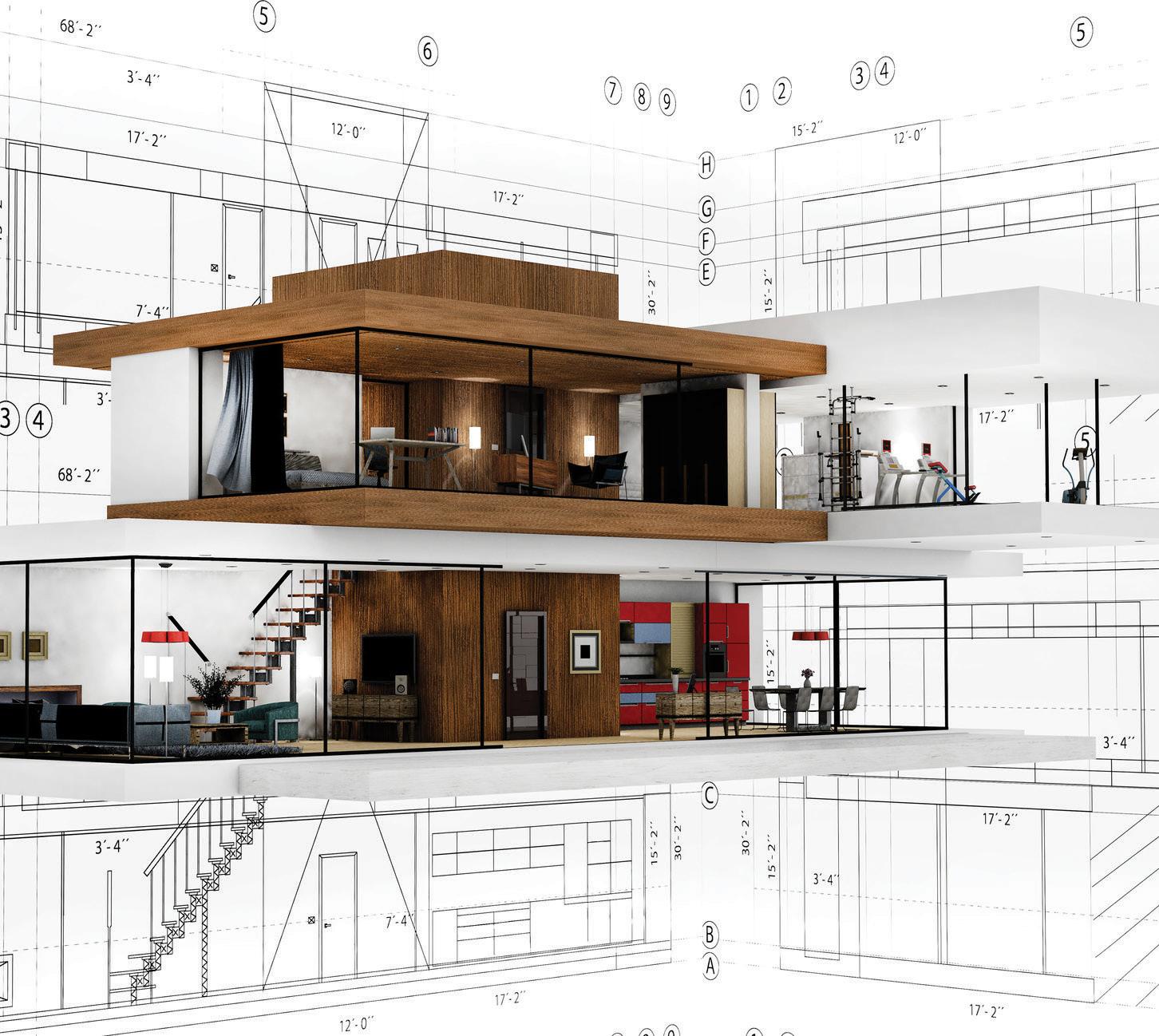
IBC Section 104.11 allows for the integration of new construction products, systems, and technologies not explicitly described in the code itself, permitting manufacturers to utilize their products if they demonstrate the products comply with the intent of the code. This demonstration is typically accomplished through product testing following established and peer-reviewed acceptance criteria documents.
Acceptance criteria documents outline specific product sampling, testing, and quality requirements that must be fulfilled to verify that a product is code-compliant.
Today, the only building code provision for alternative cements is Section 26.4.1.1.1(b) of ACI 318-19, directly referenced by Chapter 19 of the IBC. As stated previously, this ACI 318 section allows the use of concrete made with alternative cement if it meets the performance requirements for the application, including “structural, fire and durability.” Therefore, the ICC Evaluation Service developed an acceptance criteria document for alternative cements (AC529) under IBC Section 104.11.1. Section 104.11.1 of the IBC states that supporting data, where necessary to assist in the approval of materials or assemblies not specifically provided for in the code, can
consist of valid research reports from approved sources to demonstrate building code compliance verification usually issued following acceptance criteria. AC529 addresses the evaluation of alternative cements to comply with building code (IBC) objectives like structural strength, fire resistance, material properties, and durability. AC529 also outlines specific independent product sampling and quality requirements that must be fulfilled to obtain building code compliance verification. The evaluation results are summarized in a research (evaluation) report in accordance with Section 104.11.1 of the IBC and made available to code officials, registered design professionals, and the public.
Acceptance Criteria AC529
Acceptance criteria AC529, presented during an ICC Evaluation Service open public process and approved by an independent evaluation committee formed by selected code officials throughout the U.S., applies to low-carbon alternative cements activated by either water or a proprietary activator supplied by the manufacturer. Meeting these acceptance criteria is intended to demonstrate that a low-carbon alternative cement evaluated can be used in compliance with Section 26.4.1.1(b) of ACI 318-19 (as referenced by the 2021 IBC) or as an alternative to the cements outlined in Section 26.4.1.1 of ACI 318-14 (as referenced by the 2018 and 2015 IBC). The concrete produced using a low-carbon alternative cement can be used as structural and nonstructural, plain, or reinforced concrete provided the resulting concrete meets the design compressive strength and other specified engineering properties and durability requirements applicable for the end-use. This criteria for low-carbon alternative cements applies to concrete mixtures with normal-weight aggregates that comply with ASTM C33 or lightweight aggregates that meet the requirements of ASTM C330.
Acceptance criteria AC529 is titled as low-carbon alternative cement for use in concrete. Low-carbon alternative cement is defined as an
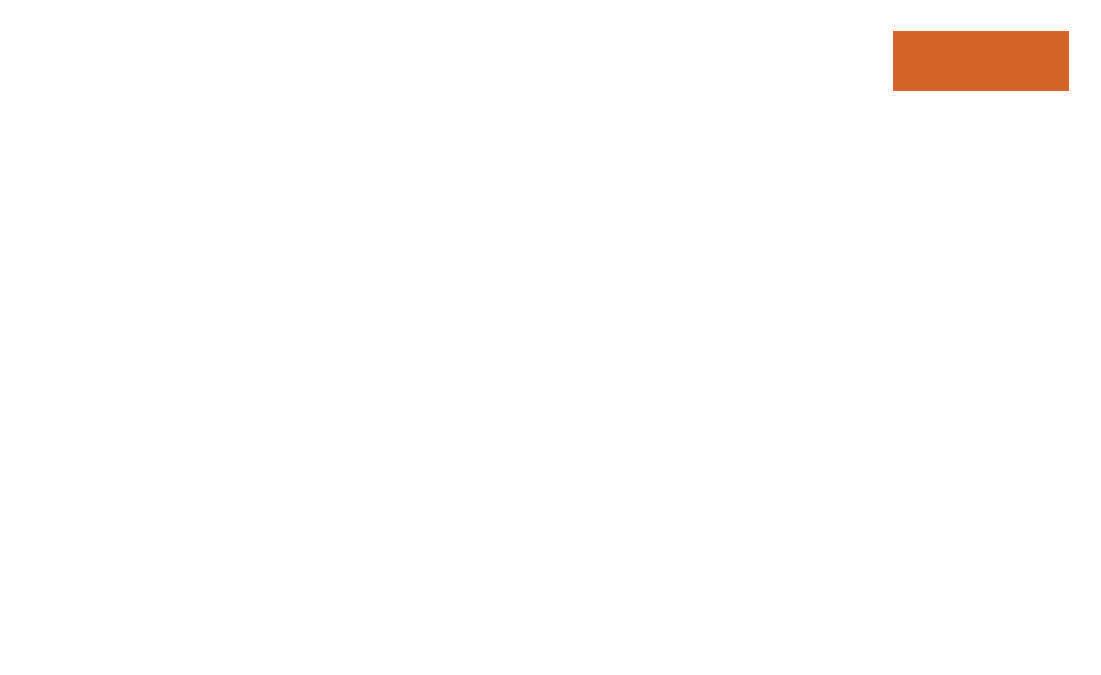
inorganic cement (mostly with proprietary formulation) that can be used as a complete replacement for portland or blended hydraulic cements and is not covered by applicable specifications for portland or blended hydraulic cements. This replacement cement has a reduced embodied carbon relative to the code-compliant portland cement being replaced. Embodied carbon is a property expressed in kilograms of carbon dioxide equivalent (CO2e) per kilogram of product or material. Carbon dioxide equivalent is a unit of measurement based on the relative impact of a given gas (CO2) on global warming (the so-called global warming potential). Embodied carbon is usually measured from cradle to end of construction.
Although some local authorities/jurisdictions have already included mandatory cradle-to-end-of-construction embodied carbon assessments as part of their planning process, there currently is no industry-accepted standardized calculation method. Therefore, AC529 does not provide guidance for calculating embodied carbon and requires this information to be obtained from the manufacturer directly or from an environmental product declaration report from approved sources. In addition to reduced global greenhouse gas emissions, which yields reduced environmental impact, alternative cements may also offer improved performance over portland cement in some applications in terms of durability and strength.
The following sections summarize the evaluation of low-carbon alternative cement properties and their effects on concrete mechanical and durability properties in accordance with AC529. More detailed test and acceptance conditions can be viewed in AC529. Almost all mechanical and durability tests in AC529 require testing two concrete mixture designs with two representative compressive strength levels.
Cementitious Material Properties: Each low-carbon alternative cement formulation must be tested for standard physical requirements in accordance with the Table in ASTM C1157 for Type GU to identify and fingerprint the cementitious material properties for building code compliance. In addition, if there is any deviation in terms of heat of hydration (ASTM C1702) and time of setting (ASTM C191) that differs from the ASTM C1157 requirements, deviations are to be reported in the code-compliance research (evaluation) report.
Concrete Compressive Strength: The compressive strength test under AC529 aims to determine a benchmark for mechanical and durability tests of the criteria. All concrete mixture designs and cementitious material contents must be established to meet or exceed the minimum compressive strength requirement of 2,500 psi (17 MPa) in accordance with Section 19.2 of ACI 318-19. In addition, testing two compressive strength levels that differ by 3000 psi (20.7 MPa) is required for various other tests required in AC529.
Equilibrium Concrete Density: AC529 requires testing to be conducted in accordance with ASTM C567. The equilibrium density is reported and used in the modulus of elasticity calculations.
Concrete Splitting Tensile Strength: The test aims to evaluate the splitting tensile strength of concrete produced with low-carbon alternative cements. Tests are to be conducted in accordance with
… the ICC Evaluation Service developed an acceptance criteria document for alternative cements (AC529) under IBC Section 104.11.1…
ASTM C496/496M. Average values must be higher than the value calculated by 6.7√f ´ c . e value of f´c used in this calculation must be the 28-day compressive strength in psi units obtained from the subsection above.
Concrete Modulus of Rupture: Flexural tests are to be conducted in accordance with ASTM C78/C78M. e average modulus of rupture of the three specimens must be higher than the calculated modulus of rupture value as required by ACI 318, Section 19.2.3.1. e value of f´c used for this equation is the 28-day compressive strength obtained from the subsection above.
Concrete Modulus of Elasticity: e test aims to evaluate whether lowcarbon alternative cements used in a concrete mixture adversely affect the modulus of elasticity of the concrete. Tests must be conducted in accordance with ASTM C469/C469M. e average modulus of elasticity must not be lower than the calculated modulus of elasticity in accordance with ACI 318, Section 19.2.2.1. e value of f´c used for this equation shall be the 28-day compressive strength obtained from the subsection above.
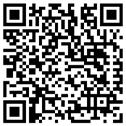
Shrinkage and Volume Change: e test aims to evaluate the shrinkage cracking performance of concrete with low-carbon alternative cements. Tests are to be conducted in accordance with ASTM C157/C157M with a maximum 0.065 percent average strain measurements of all tests.
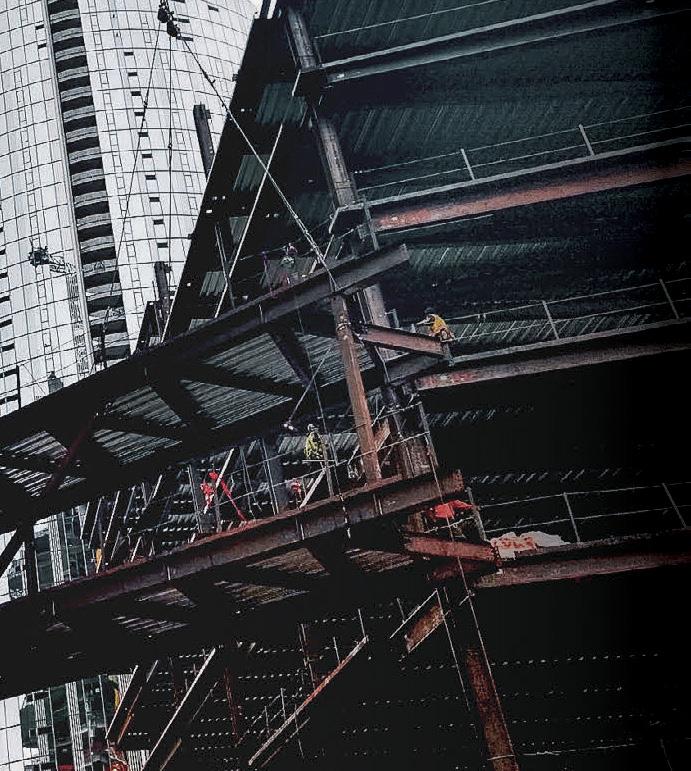
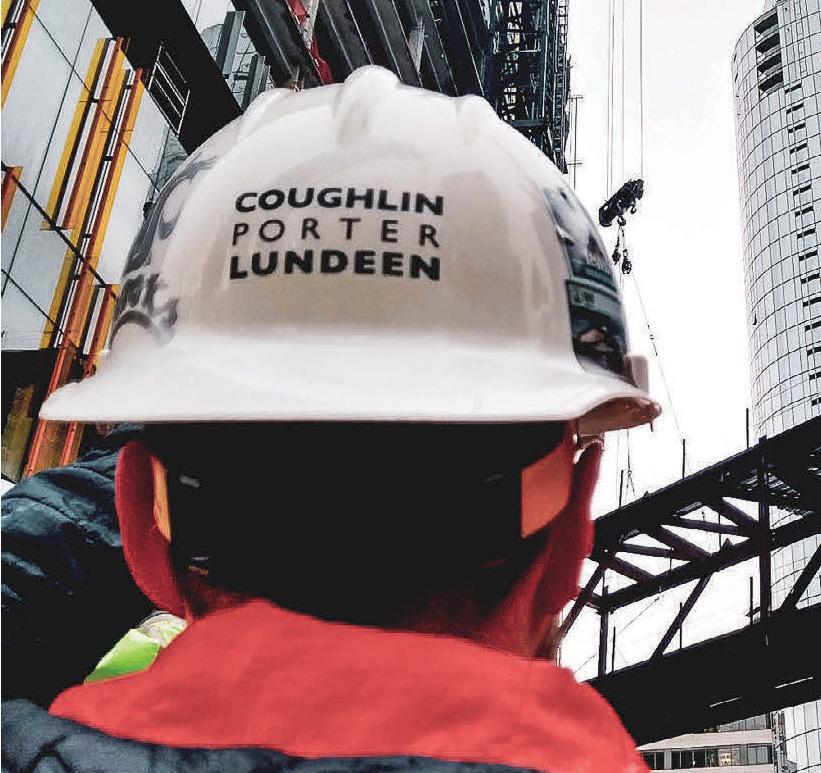
Freezing and awing Durability: is test aims to evaluate the durability of concrete subject to exposure to freezing and thawing conditions or deicing chemicals. Tests must be conducted in accordance with Procedure A of ASTM C666/C666M for a minimum of 300 cycles with a minimum durability factor of 80 percent.
Alkali-silica Reaction: Alkali-silica reactivity in concrete with lowcarbon alternative cement formulations must be tested in accordance with ASTM C311/C311M and ASTM C1293, including some modifications given in AC529. Results must indicate that lowcarbon alternative cement does not exacerbate alkali-silica reactivity if the 14-day expansion as a percent of control is a maximum of 100 percent. Alternatively, the low-carbon alternative cement does not exacerbate alkali-silica reactivity if the 12-month expansion in ASTM C1293 testing is a maximum of 0.04 percent.

Water Absorption: is test aims to evaluate the water absorption of the low-carbon alternative cement concrete mixture for durability and building envelope purposes. Tests are to be conducted in accordance with Procedure A of ASTM C1585 with a maximum water absorption of 8 percent.
Linear Coefficient of ermal Expansion: e coefficient of thermal expansion of low-carbon alternative cement concrete must be reported by the testing laboratory in accordance with ASTM C531 and included in the code-compliance research (evaluation) report.
Chloride Content: e test aims to evaluate the chloride content of concrete with low-carbon alternative cements. Reports of tests in accordance with ASTM C1218 must demonstrate that each cementitious material concrete complies with Table 19.3.2.1 of ACI 318-19 for maximum water-soluble chloride ion content in concrete.
Sulfate Resistance: Sulfate resistance of the low-carbon alternative cement concrete is to be tested in accordance with ASTM C1012. e low-carbon alternative cement concrete is considered sulfate resistant if the average expansion after 6 months does not exceed 0.10 percent. Fire-resistance: e purpose of the section is to evaluate the fire-resistance performance of concrete assemblies constructed with low-carbon alternative cements using either fire analyses in accordance with IBC Section 721 or testing concrete assemblies in accordance with ASTM C119 or UL 263. is evaluation may be omitted if low-carbon alternative cements in concrete are limited to non-fire-resistance rated construction assemblies in the published code-compliance research (evaluation) report.
Noncombustible Building Material: e purpose of this test is to demonstrate that concrete produced with the low-carbon alternative cements may be considered a noncombustible building material if the intent is to use in Type I, II, III, or IV construction per IBC Chapter 6. Quality Control: To satisfy the quality objective of IBC Section 104.11, AC529 requires annual inspections of each manufacturing facility under an approved quality documentation for continuous production consistent with the material tested for qualification.
Summary
e IBC is the predominant U.S. building code. IBC Section 104.11 allows for alternative materials, designs, and methods provided that such alternatives have been evaluated to meet code requirements. AC529 provides the evaluation criteria and acceptance conditions for quantifying the use of alternative cements in compliance with ACI 318-19 or as an alternative to ACI 318-14. e final research (evaluation) report issued in accordance with AC529 is intended to demonstrate building code compliance. It is primarily used by code officials and registered structural engineers in charge of the construction.
■
building BLOCKS
A Closer Look at Structural Lightweight Concrete
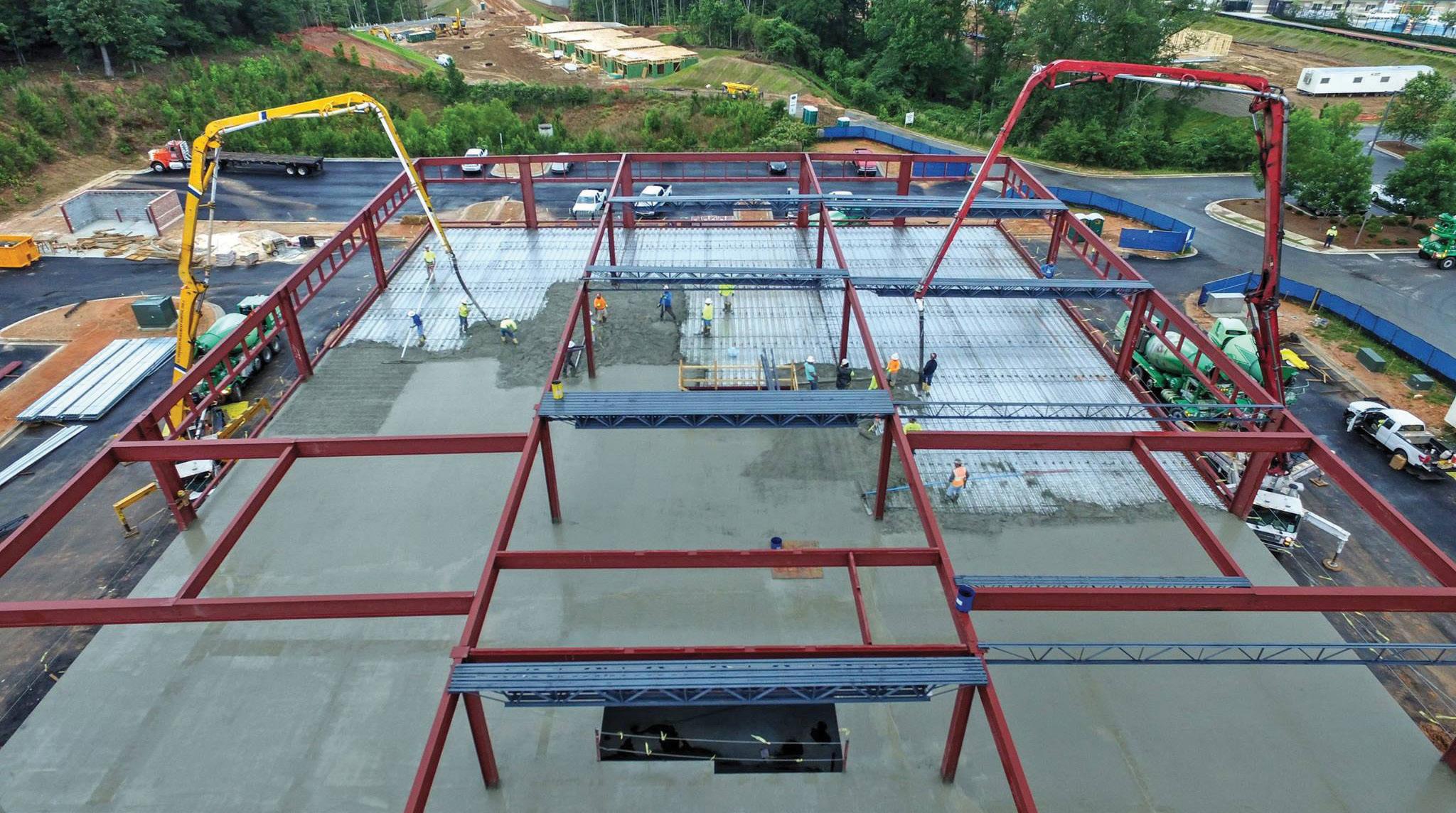
Myth Versus Reality
By Ken Harmon, P.E.Lightweight concrete has been a staple of the built environment for centuries, predating the Roman Empire. While some early lightweight concrete structures still stand, their materials bear little resemblance to today’s offerings. Industry professionals now recognize structural lightweight concrete as a strong, low-density mixture of Portland cement, water, and various combinations of normal weight aggregates and lightweight aggregates. In such concrete, lightweight aggregate produced from shale, clay, or slate is heated to approximately 2000 degrees F in a rotary kiln process. During this process, it softens and bubbles form that remain as unconnected pores when it cools.
Since the early 1920s, industry professionals have effectively employed structural lightweight concrete to solve weight and durability problems. Yet misconceptions about the material’s light weight and porous form and how they impact enduse performance in composite metal decks for floors and roof slabs still exist. This article reviews three relevant misconceptions to help set the record straight.
Myth #1
The water absorption of lightweight aggregate reduces the strength and durability of structural concrete.
A pervasive industry belief is that greater water absorption by aggregates reduces the performance properties of structural lightweight concrete. However, data from a study by Byard and Schindler at Auburn University (2010) indicate that the aggregate’s raw material has little bearing on performance.
The study compared the performance of structural lightweight concrete using three common mixture ratios:
• An internally cured mixture for which a fraction of the conventional fine aggregate (sand) was replaced with prewetted lightweight fine aggregate;
• A commonly used sand lightweight concrete mixture, for which lightweight coarse aggregate and conventional fine aggregate (sand) were used; and
• An all-lightweight concrete mixture that uses lightweight coarse aggregate and lightweight fine aggregate (no normal weight aggregate).
All three types of lightweight aggregate met the standard materials specifications, AASHTO M 195, Standard Specification for Lightweight Aggregates for Structural Concrete, or ASTM C330, Standard Specification for Lightweight Aggregates for Structural Concrete. As a control, these mixtures were compared to the performance of a normal weight concrete made with river gravel. (Caution: Although with the same title, AASHTO M 195 and ASTM C330 are two different specifications. Therefore, it is incumbent on designers to determine the differences.)
The results show that shale and clay lightweight aggregates have markedly higher absorptions than the slate lightweight aggregate (see Table). If Myth #1 were true, the mixtures using these materials should have had reduced strength and durability, but they did not.
There was no significant difference between the 28-day compressive and splitting tensile strength data for the shale and clay aggregates and the slate aggregate. Further, the splitting tensile strength for the slate aggregate was the lowest of the three mixtures. These outcomes dispel the myth that the absorption of the aggregate drives structural lightweight concrete’s strength and durability.
Myth #2
Lightweight concrete floors take much longer to dry.
Due to its internal pore network, lightweight aggregate absorbs and stores water before releasing it gradually over time, resulting in higher absorption than conventional aggregates. The aggregate is prewetted before mixing to maintain slump and allow pumping. This water needs to evaporate until the floor slab achieves equilibrium with the ambient conditions. While there is always the concern that moisture that leaves a concrete slab can bring alkalinity to the surface and negatively interact with some flooring adhesives, this concern is amplified with lightweight aggregates. The belief is that the excess water retained in the pores greatly extends the drying time, precluding it from use in buildings with fast-tracked construction timelines.
Recently, the Expanded Shale, Clay, and Slate Institute (ESCSI) sponsored a study (Craig, 2011) comparing lightweight and normal weight concrete slabs that refuted this myth. It revealed that while there was more initial water in the lightweight slabs, the drying times were similar to normal weight slabs. In fast-paced construction, it is important to note that the findings indicate that both types of concrete will likely not reach the industry-accepted limits when it is time for the installation of adhesive-attached floor coverings and will require moisture mitigation techniques.
Rewetting poses a greater obstacle to slab drying than initial water content. Until a structure is enclosed, the slab cannot begin to dry, regardless of whether it is lightweight or normal weight concrete. This makes it even more critical not to dismiss the benefits of lightweight concrete simply due to concerns over its initial water content.
Myth #3
Lightweight concrete is more expensive.
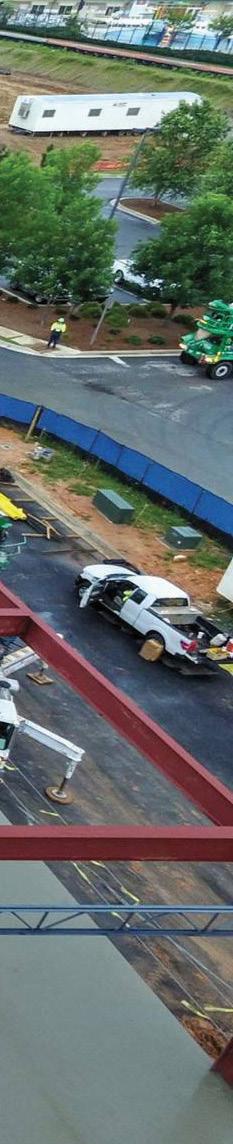
Another common industry misconception is that lightweight concrete costs more than normal weight concrete. While it is true that aggregate processing and shipping lead to higher per cubic foot costs, the overall reduction in concrete weight offsets the increased unit cost and can lead to savings. These economies are realized in a few ways, regardless of geographical location.
The unit weight of the concrete is reduced from about 145 pcf for normal weight concrete to about 115 pcf for lightweight concrete (using equilibrium density with adequate drying with time). This is an overall reduction of 21 percent. These savings compound in buildings requiring a fire-rated floor system because a lightweight concrete floor can be thinner than a normal weight concrete floor and provide the same fire rating. In either scenario, lightweight concrete reduces the floor slab density and overall load. With less material needed for the framing system and foundation, economies across the whole building are typically more than enough to offset the additional cost of lightweight concrete. More information on these savings can be found in the Five Story Commercial Building Study (Utelite Corporation, 2017).
Looking Ahead
With these three common structural lightweight concrete myths busted, readers can rest assured that the hardworking material remains a viable solution for the built environment. In fact, with demand for longer floor spans, minimal floor thicknesses, and lighter dead loads, structural lightweight concrete will stay at the forefront of commercial building.■
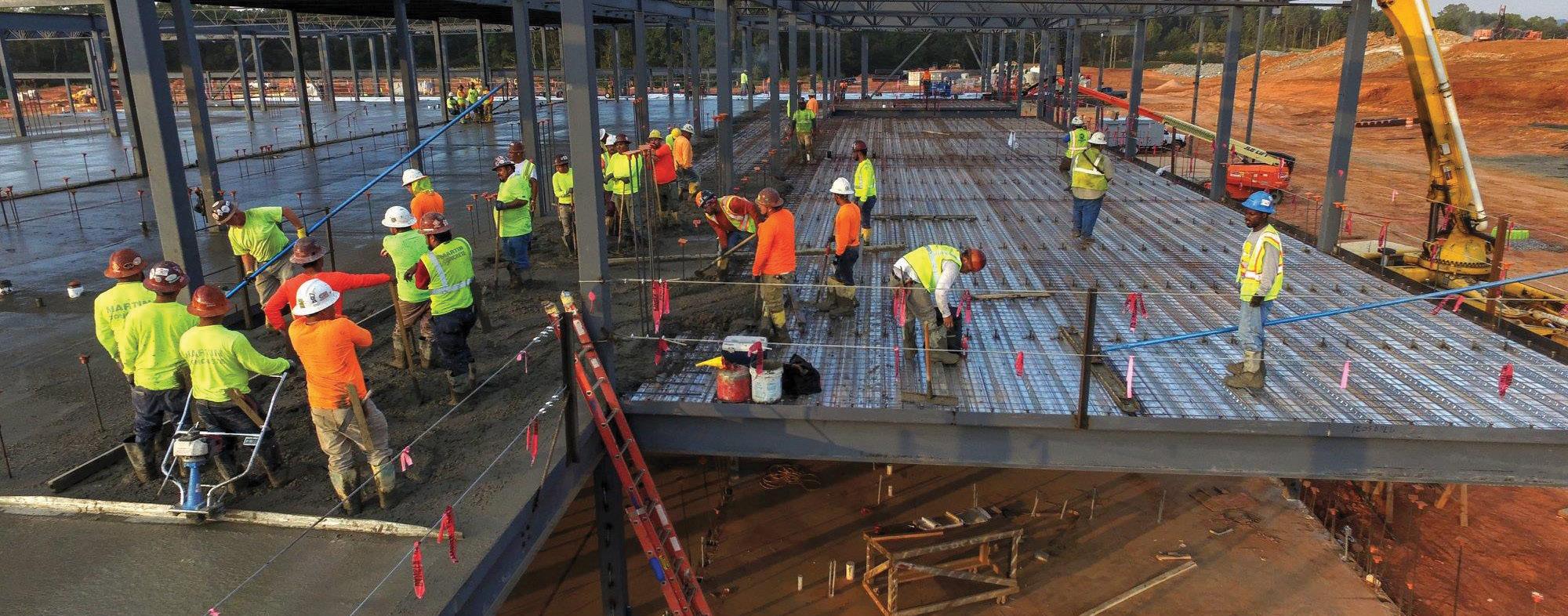
This article, all or in part, was published on the ESCSI website, June 2020. It is reprinted with permission. References are included in the PDF version of the online article at STRUCTUREmag.org
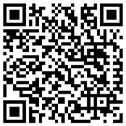
structural FAILURES
Avoiding Failures in Concrete Structures
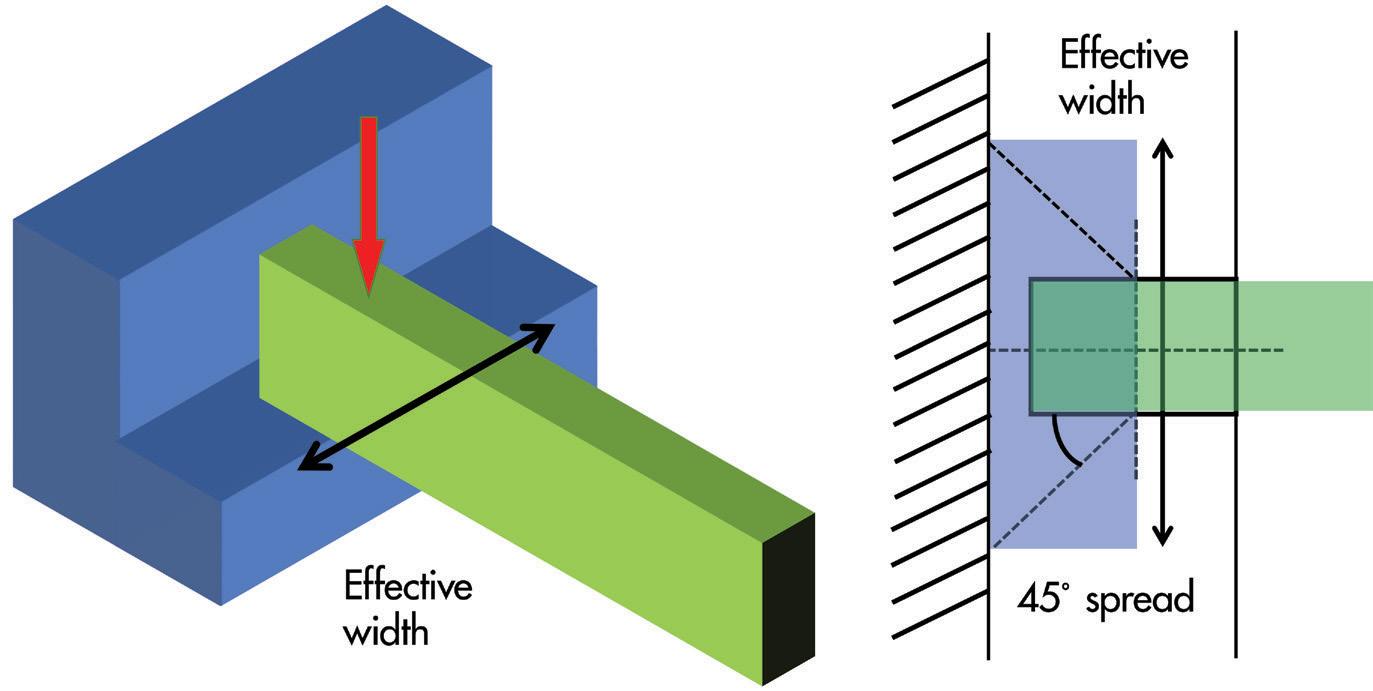
Reinforced concrete is a construction material widely used in many applications, including buildings, bridges, and other infrastructures. Due to its massive size and various redundancies, concrete structures are often considered solid, robust, and safe. However, there have been instances of concrete structure failures and collapses, many due to erroneous assumptions or oversight by the designers in evaluating the design situation or poor construction practices. This article highlights several design and construction situations that designers and builders should pay special attention to in order to prevent failures.
Design of Silos
Silo structures are notoriously known to many as a structure requiring special attention, not because of the external loads, such as wind or seismic, but due to the storage load of the bulk solid. The unwary designer might unrealistically simplify and assume the load to be hydrostatic pressure caused by a fluid, even though the actual storage material might be solid granular material or particulate in nature. The force exerted by particulate materials onto a silo container can be different. First, the designer must consider conditions during the filling up of the silo and discharging of the materials. There might be instances of eccentric loads (Figure 1) and higher loads (e.g., impact) caused by these processes. Next, there must be consideration of whether the presence of moisture, gas pressure, or other ancillary equipment imposes additional loads.
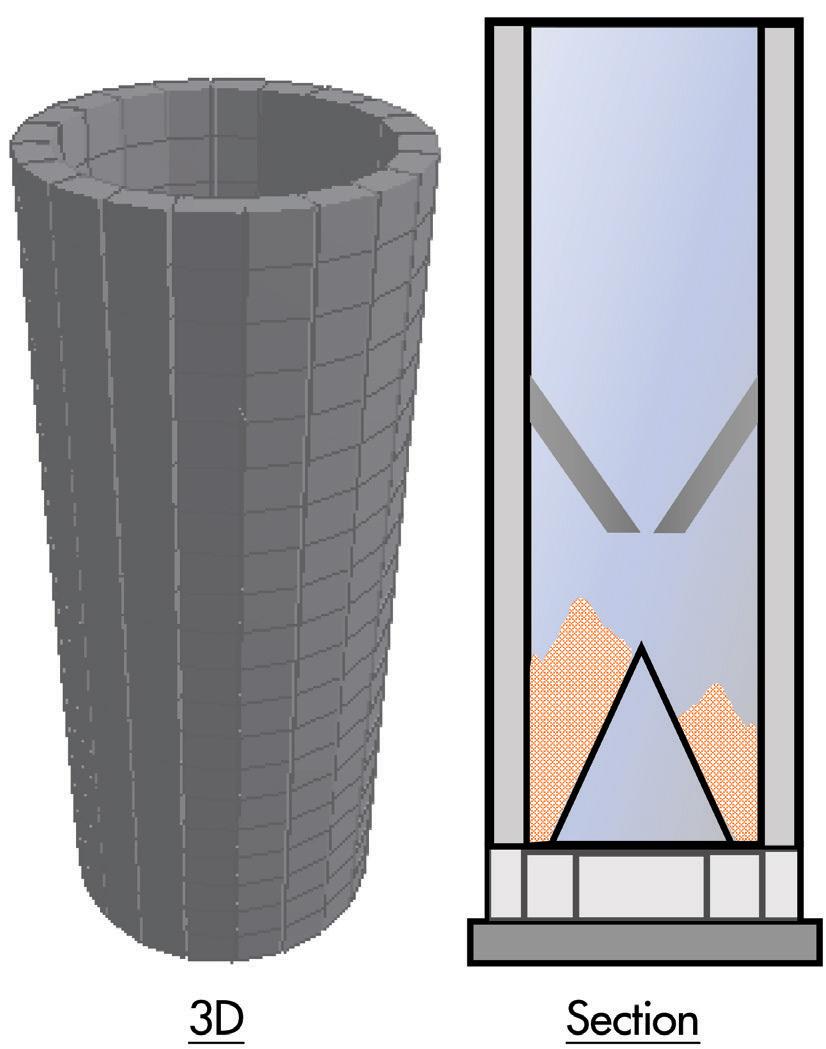
For example, some grains are known to absorb moisture and cause additional loads due to swelling of the grains. This example illustrates the importance of determining as accurately as possible the type of loadings exerted by different materials onto a structure, including loading magnitude, direction, and possible permutations in area. Detailing a concrete silo with a single layer of reinforcement to deal with hoop tension only by assuming symmetric loadings would clearly be inadequate.
Effective Width of Support Member
Consider a case of a concrete support structure with a wide corbel (or nib) to catch an incoming beam (Figure 2). Due to its short length compared to its depth, the design of the corbel should not be based on a bending formula. Instead, a strutand-tie model would be more appropriate to work out the necessary reinforcements to resist the load. However, there is still a need to work out the effective width of the corbel used in resisting the load and not assume the entire width of the corbel can be used. To work out the effective width, designers can refer to guidance on effective slab width at exterior slab-to-column connections, where a 45-degree spread from the centerline of the column is recommended. There may be a larger angle of spread if the transverse stiffness of the corbel is high, making it very rigid (e.g., thicker section or higher reinforcement ratio). But an overestimate of the effective width might give rise to over-prediction of capacity and result in design deficiency of this critical structural element. Underdesign of such low redundancy structural elements (e.g., cantilevers, simply supported beams) can be very dangerous because there is no alternative load path for redistribution, and failure is often likely to occur. Therefore, correctly selecting the effective width can be a crucial design aspect.
Nib Failure
One common way to support a brick wall façade is by designing a nib. Because of the small bearing length of the nib, it is necessary to place the reinforcements precisely. This ensures that sufficient bearing length of the nib is reinforced with reinforcement bars. Suppose the reinforcement bars were placed short, resulting in extra concrete cover,
or worse, using additional thicker plaster to achieve the required length. In that case (Figure 3), the unreinforced portion of the nib can easily be dislodged under the weight of the bricks. is example highlights the dangers of having extra concrete cover and failure in the unreinforced zones in a structural member, especially when such unreinforced zones are required to support heavy loadings.
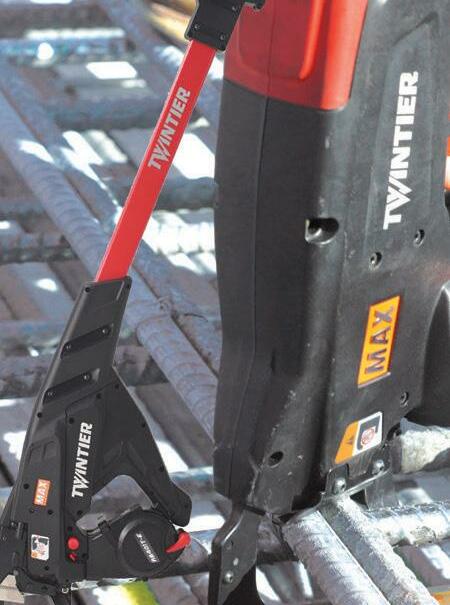
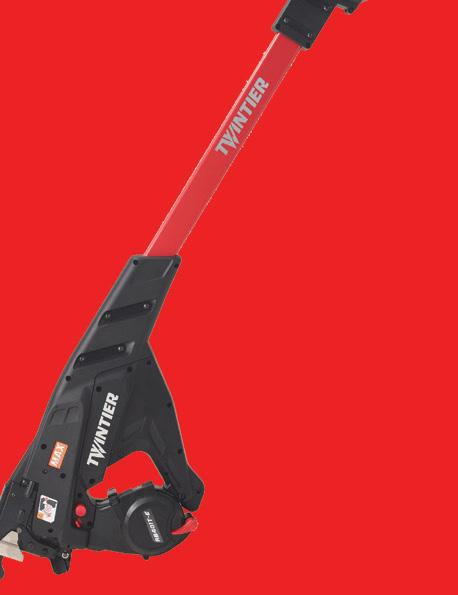
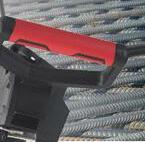
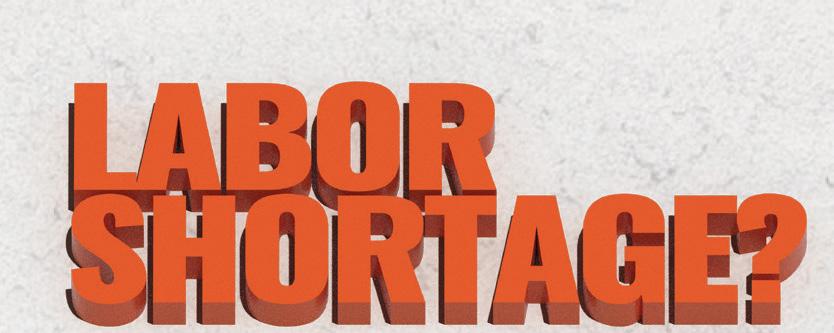
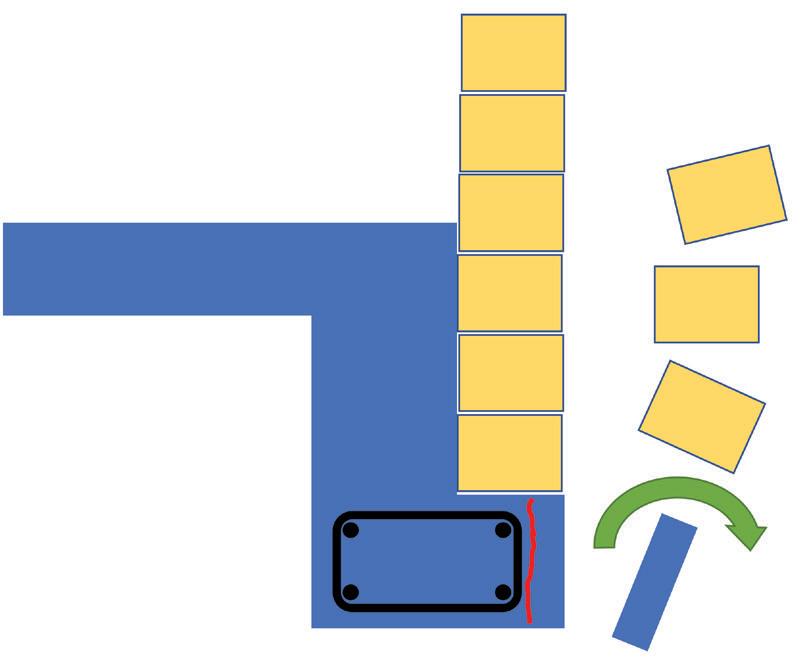
Cracks at Joints and Discontinuities


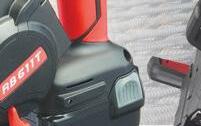
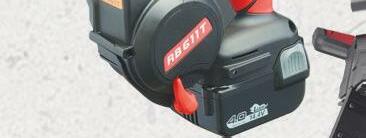


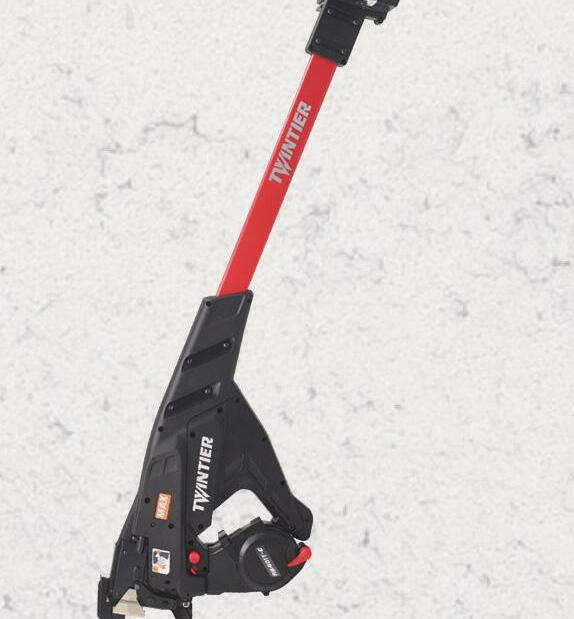
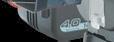
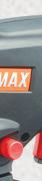
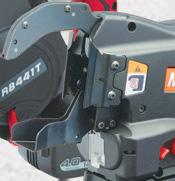

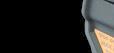
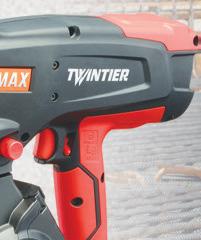
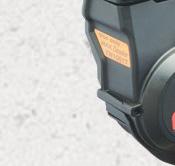
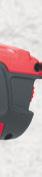
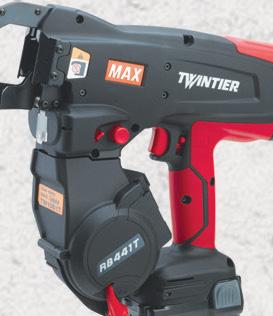

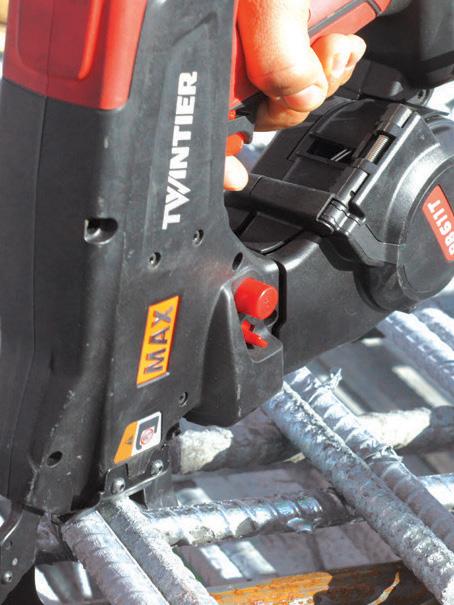
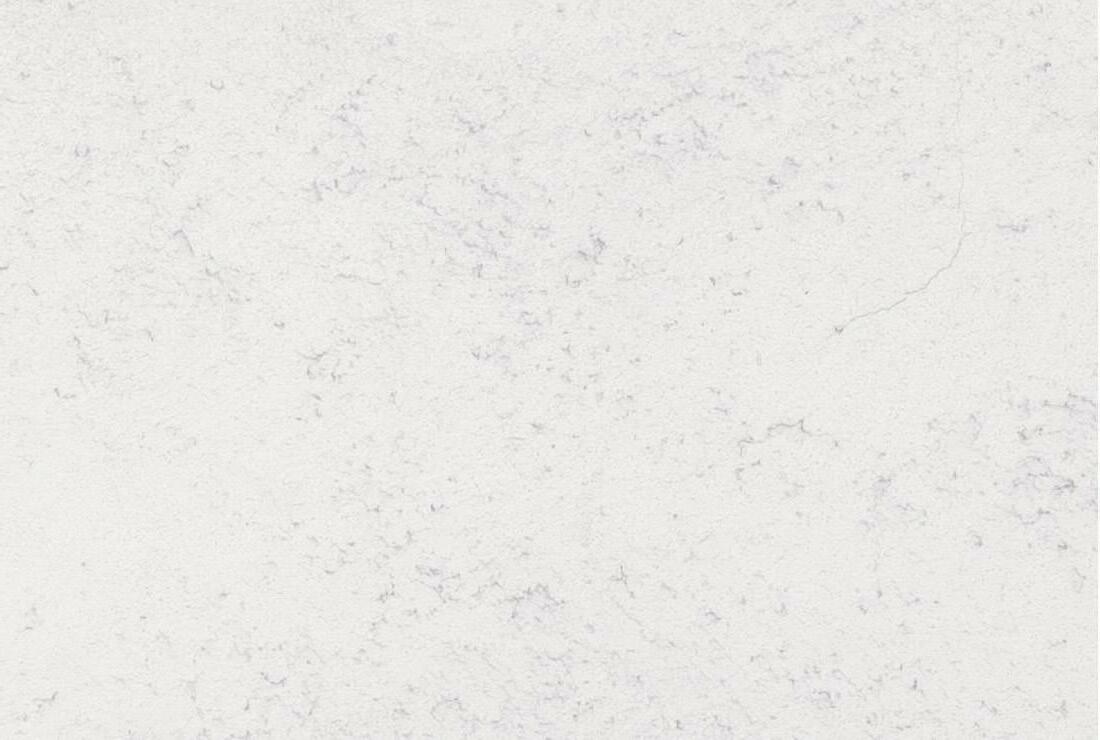
In concrete structures, high stress concentrations can occur in regions such as turnings and kinks (e.g., connecting truss members and inclined members, Figure 4, page 24). In addition to correctly identifying the type of actions to be resisted (i.e., axial, shear, bending, and torsion) by a particular structural member, it is necessary to detail the reinforcement bars correctly. Members that are not strictly beams (dominantly bending and shear) or columns (dominantly axial load) could potentially be subjected to a combination of more than one dominant action. e detailing process can become difficult due to awkward geometry and difficulty fitting bent bars in tight spaces,


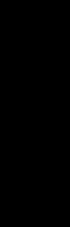
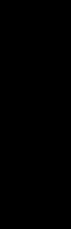
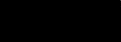
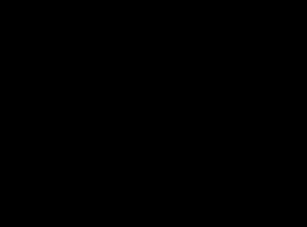
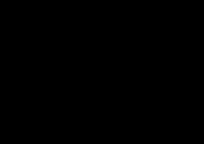
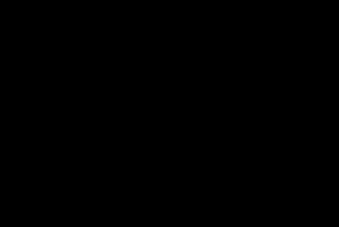
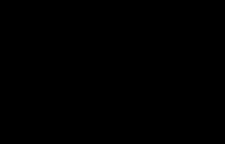
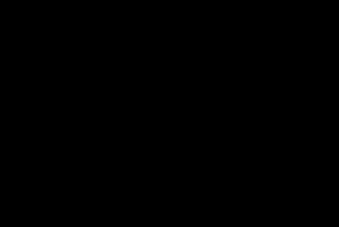
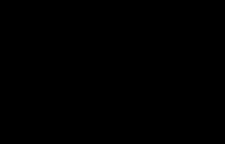

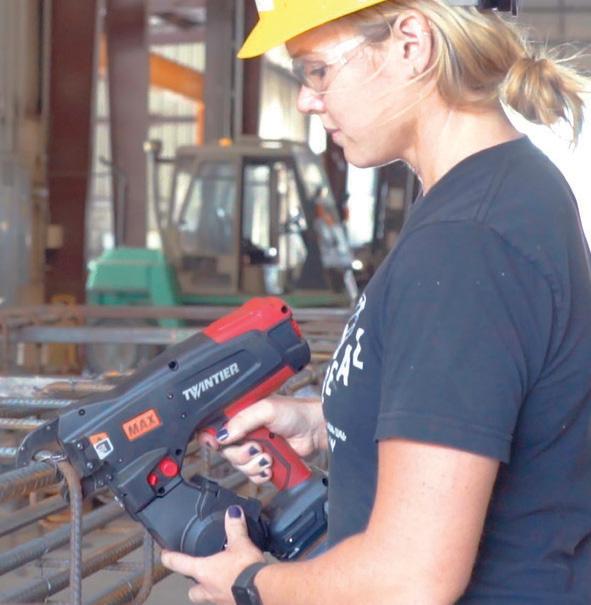
which may sometimes result in extra concrete cover that may not necessarily be desirable and could sometimes result in cracking. Splicing of reinforcing bars (especially if spliced all in one location) may introduce a weakness at the location of bar discontinuity. When bars need to be discontinuous, splicing should be staggered and preferably avoid highly stressed regions. Another design situation requiring attention is beams framing into columns at different levels on opposite sides. Designers should know that the opposite direction moments do not equalize and design for the actions imposed by such a configuration. is can be a more common issue in concrete construction, where there is often some fixity at the joints.
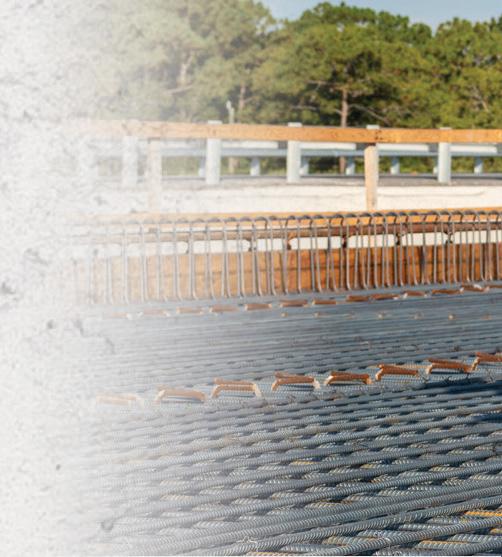
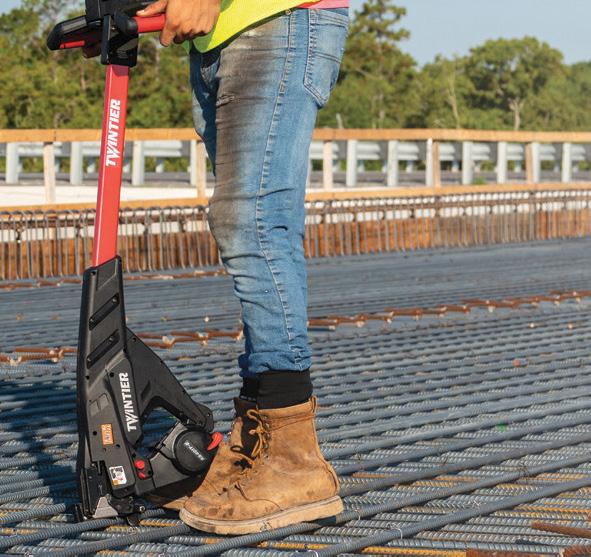
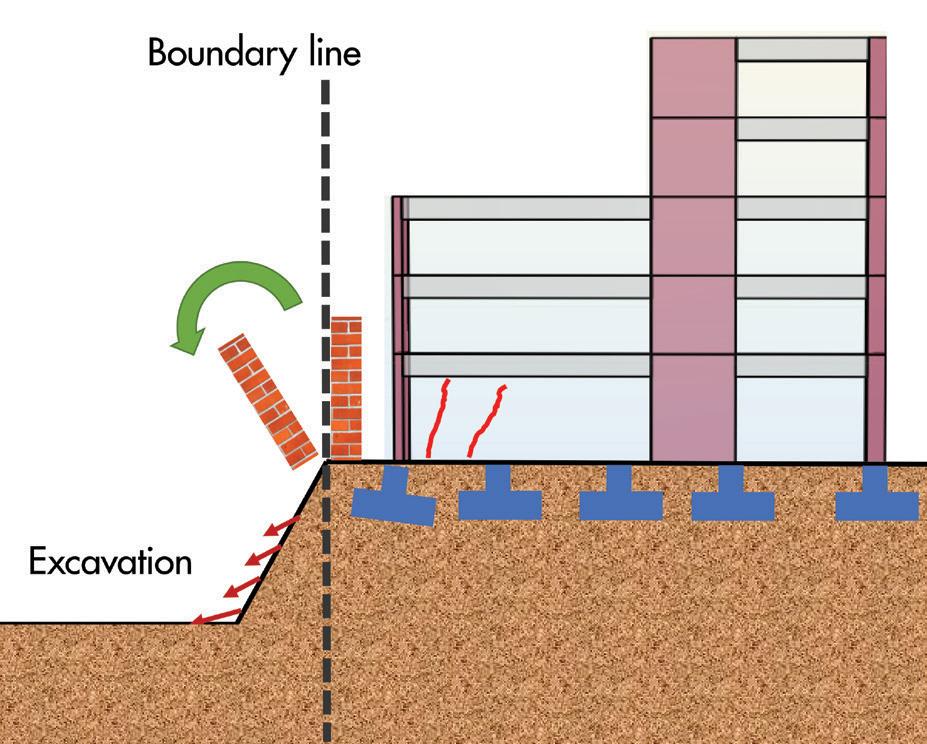
Flat Slabs to Span Full Load in Two Directions
When designing a flat slab system (Figure 5 ), designers need to note that the full load must be taken in the two orthogonal directions independently. Some designers mistakenly assume the flat slab system behaves similarly to a two-way spanning slab and underestimate the loading and bending moments, resulting in inadequate reinforcements. They might have distributed the full load in two directions (each direction taking one half) or designed for the full load in one direction but totally missed out the orthogonal direction. This misconception could have come from designing a one-way spanning slab where only one direction of spanning (the shorter span) of the slab is required. The other direction is also taking the full load but in the form of the beam and not the slab.
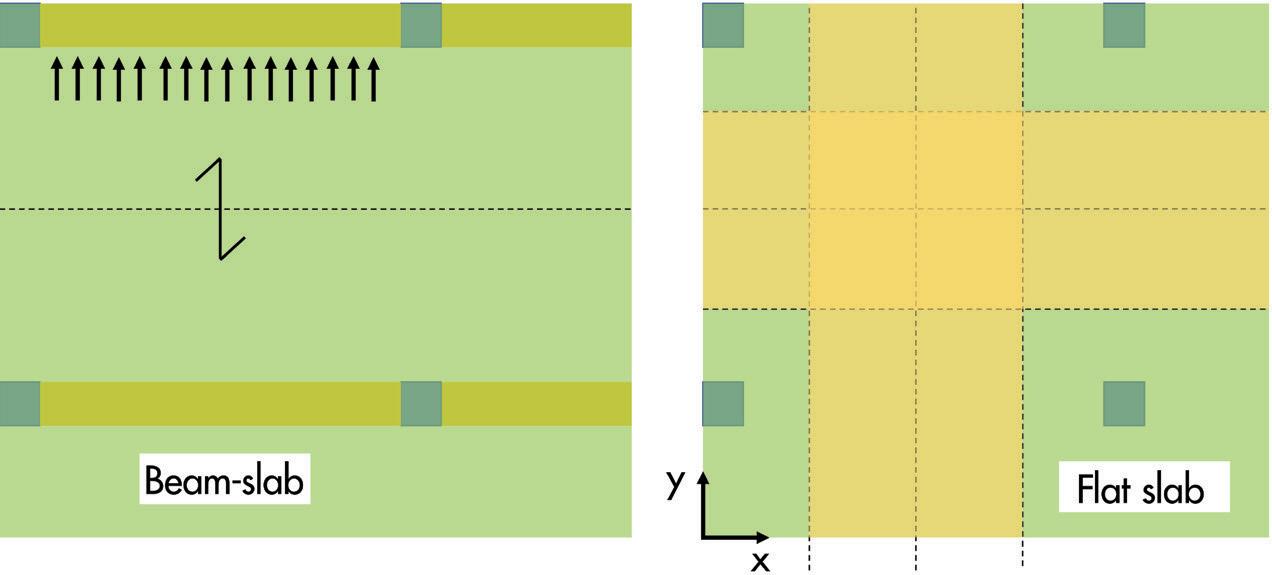
In a different scenario, some builders who are not familiar with beam-slab design may assume that a longer span is always more critical and be tempted to place larger reinforcement in a slab (with full beam supports) in the direction of the longer span, not knowing that the larger moment actually occurs in the shorter span. While builders are not expected to carry out structural design, they should still appreciate basic structural behavior and reinforcement placement concepts.
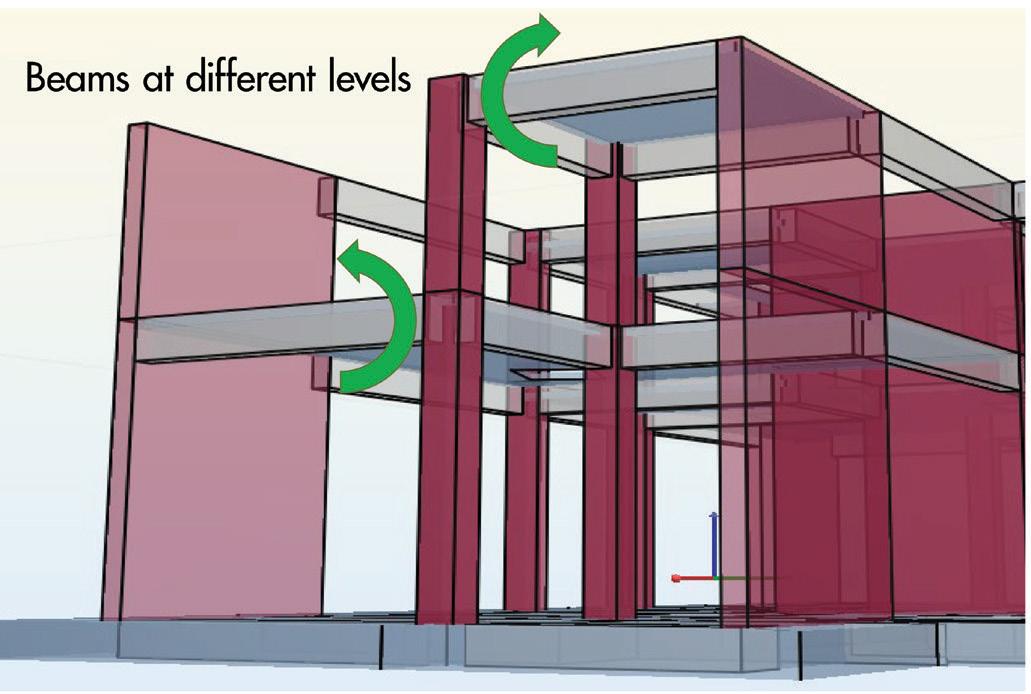
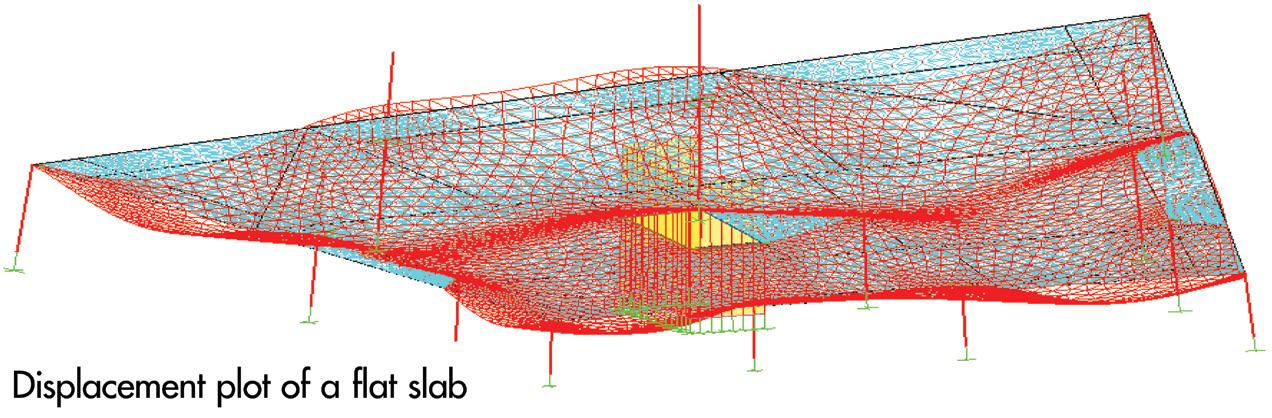
Works Near Existing Buildings
In many city centers and highly urbanized built-up areas, new development works might inevitably be located close to an existing building. The situation worsens if any excavation for basement or foundation works is required. Some builders make the mistake of excavating right next to an existing boundary wall, not knowing that such excavation work can undermine the foundation of the boundary wall and can cause it to topple (Figure 3). When ground movement is not appropriately controlled (e.g., open-cut excavation), the adjacent building’s foundation may experience movement (lateral and vertical) and cause damage to the super-structure. It is thus necessary to adopt an appropriate earth-retaining system that can limit the ground movements to acceptable levels. It may even be necessary to strengthen the adjacent structures’ ground or foundation in certain circumstances. Similarly, digging in front of a retaining wall may cause the wall to be weakened, as passive resistance in front of the wall is reduced when soil is excavated. This scenario can occur in hilly areas where retaining walls retain slopes and create usable areas. An overly-zealous contractor could commence excavation in front of a retaining wall without knowing such works could trigger a collapse. It is safer first to understand how construction works would impact the adjacent buildings and structures and then design a safe work procedure with strengthening measures to ensure that the existing retaining wall stability is safeguarded during any digging.
Temporary Support during Construction
There are two main areas to note during reinforced concrete construction: the support of the reinforcement bars and the wet concrete during pouring concrete. Reinforcement bars require some support to hold them in position before concreting. In very thick concrete elements, such as raft foundations or transfer plates, the area of reinforcement bars is likely to be correspondingly large. Some builders might overlook the lateral support required for such heavy top bars and rely on stirrup-type support to hold up these bars. For such cases, it is necessary to design a bespoke support system with adequate lateral bracing to prevent side-sway failure ( Figure 6 ). Formwork to support wet concrete needs to be snugly
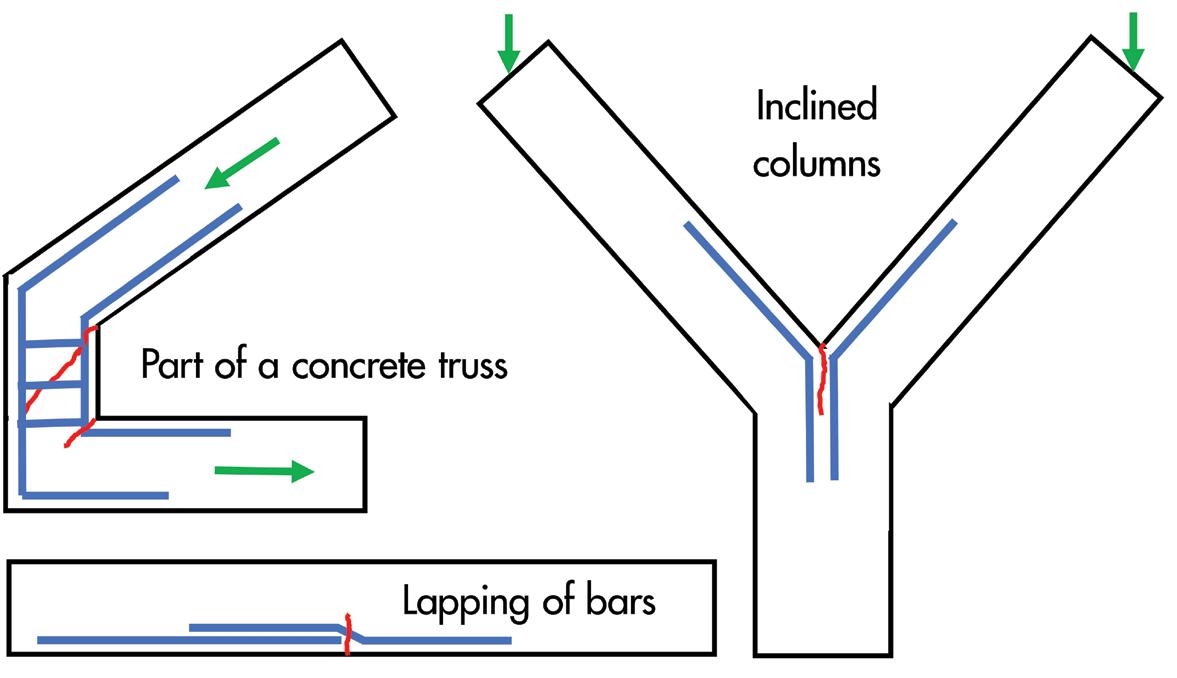
fi
tted and free of gaps that may allow concrete to fl ow through. is may sometimes be diffi cult when there are gaps in sloping or uneven ground. When concrete must be cast adjacent to an existing structure, builders must be mindful not to impose additional loads onto the existing structure. For example, such existing structures could be made of a brick wall with little capacity to resist the heavy lateral loads imposed by wet concrete ( Figure 6 ). In addition to designing formwork to take lateral loads, the designer also needs to ensure the overall concrete section or element to be cast does not lean onto the existing structure.
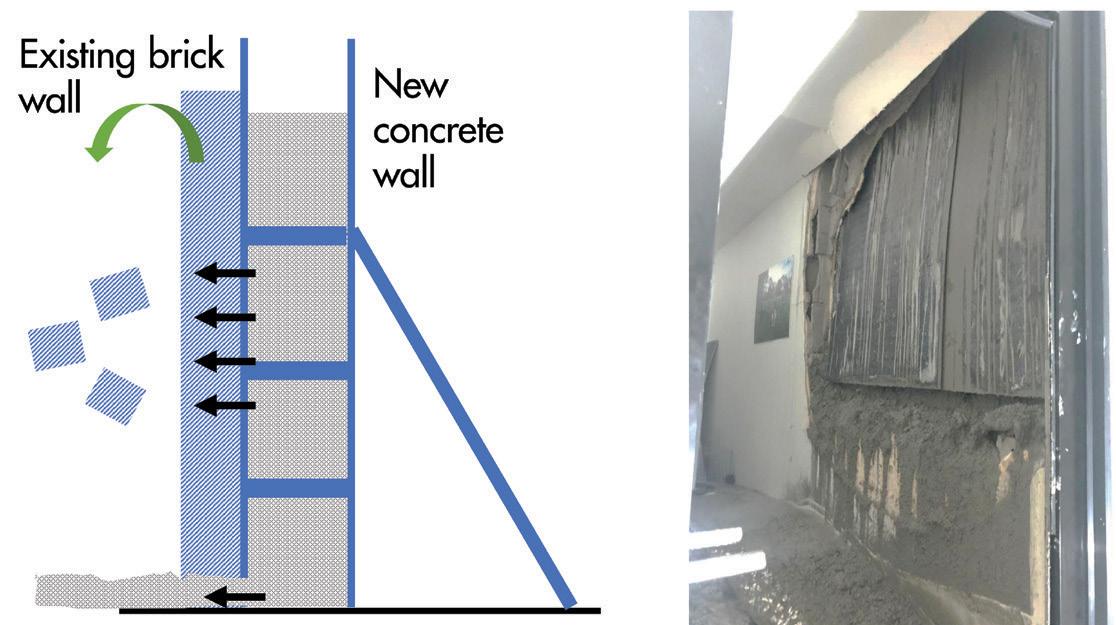
Specifications and Quality Control
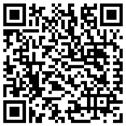

Concrete structures can sometimes fail during service life for various reasons, such as deterioration (spalling due to rusted reinforcements), attack by aggressive environments, lack of maintenance, overloading, or unauthorized alterations. erefore, correct specifications and quality control during construction are critical in ensuring concrete is durable to withstand weathering in its environment. Designers must pay attention to the exposure class and comply with requirements on minimum compressive strength of concrete, cover, cement content, etc. In highly aggressive situations, it may be necessary also to have special additives and protective coatings on reinforcement bars. Supervision and quality control during construction ensures that specifications on paper are met on-site, from concrete production and delivery to handling, placement, and curing.
Building owners should be informed by the designers on the usage of the building and be briefed on allowable loadings on floors, maintenance requirements, and not to make unauthorized alterations. Any unusual sightings of distress (e.g., cracks, deformations, settlement) should be referred to a qualified professional for assessment. Carefully designed reinforced concrete structural elements should have sufficient ductility and should not fail by sudden rupture or instability. Many mishaps can thus be avoided if building owners and occupants pay attention to signs of distress and take early and timely interventions to evaluate and remedy the situation.
Summary
Safe design and construction of reinforced concrete structures require attention to detail. First, the designer can select an appropriate structural system to resist the loads by understanding loadings and load path. Special attention should be paid to silos and flat slabs. Next, it is necessary to appreciate the concept of effective width when evaluating a section capacity under concentric load.
Reinforcement detailing and placement on-site are also important aspects to pay attention to in order to avoid cracking and failure of unreinforced zones of a member. e builder should always remember that wet concrete during placement and heavy reinforcements require robust temporary supports. e various failure modes should be fully understood to avoid underestimating the potential for failure.
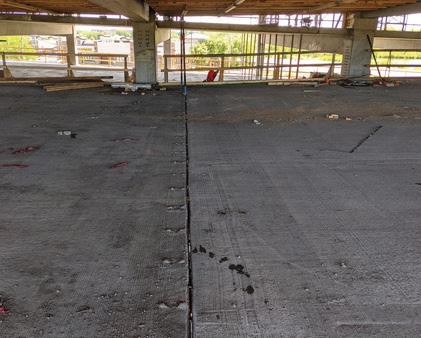
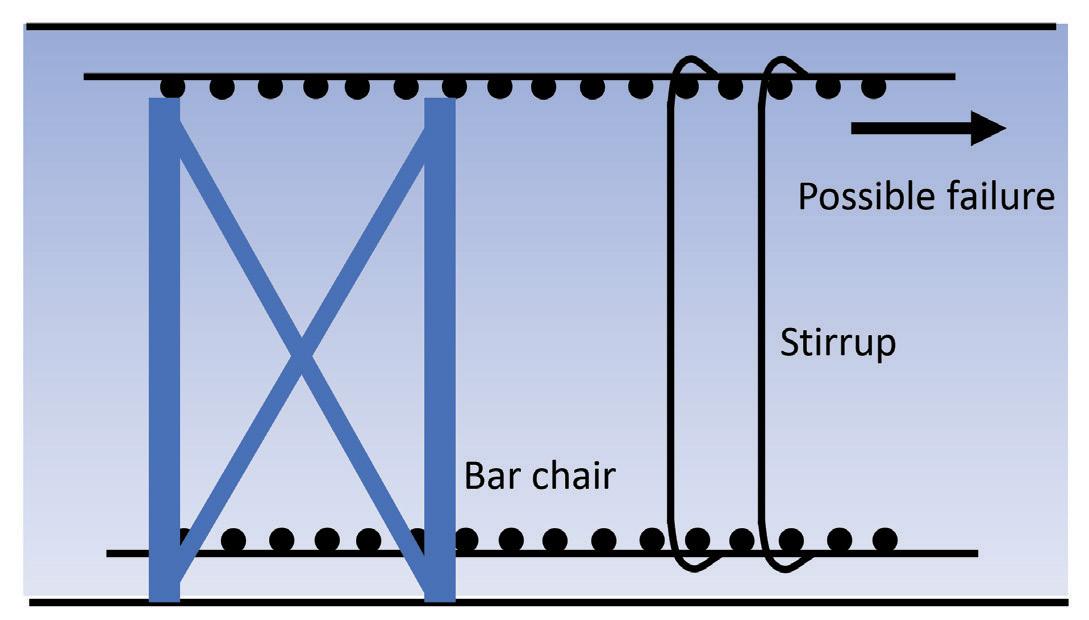
Correct specifications and quality control during construction ensure durable structures to prevent premature deterioration. In addition, building owners and occupants have a role in ensuring the continued safety of a building in its service life by performing periodic monitoring and maintenance.■
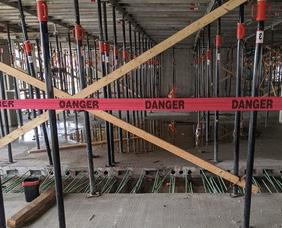
Hee Yang Ng is a Principal Engineer with a building control agency in the Asia-Pacific region.

Lindquist Hall Remodel and Seismic Renovation
By Jeremy L. Achter P.E., S.E., LEED AP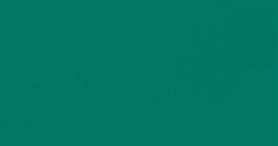
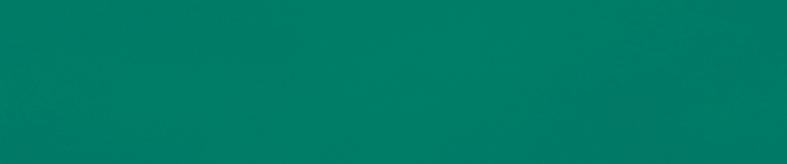
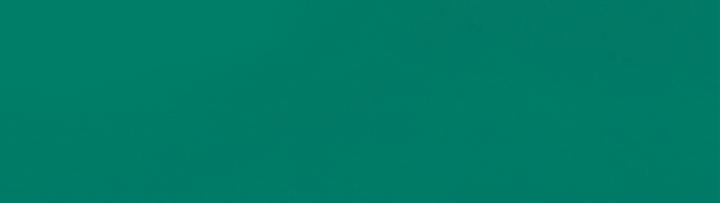
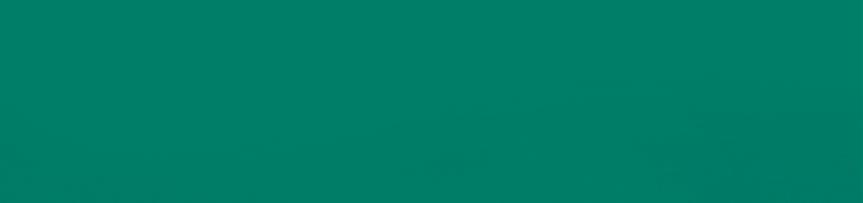
The old Social Science Building at Weber State University, located in Ogden, Utah, was constructed in 1970. Programmatically, the building has been one of the most heavily used on campus, serving the social sciences, humanities, and many other departments throughout its life. One would be hard-pressed to find a graduate of the University who had not taken a class there. The concrete building,
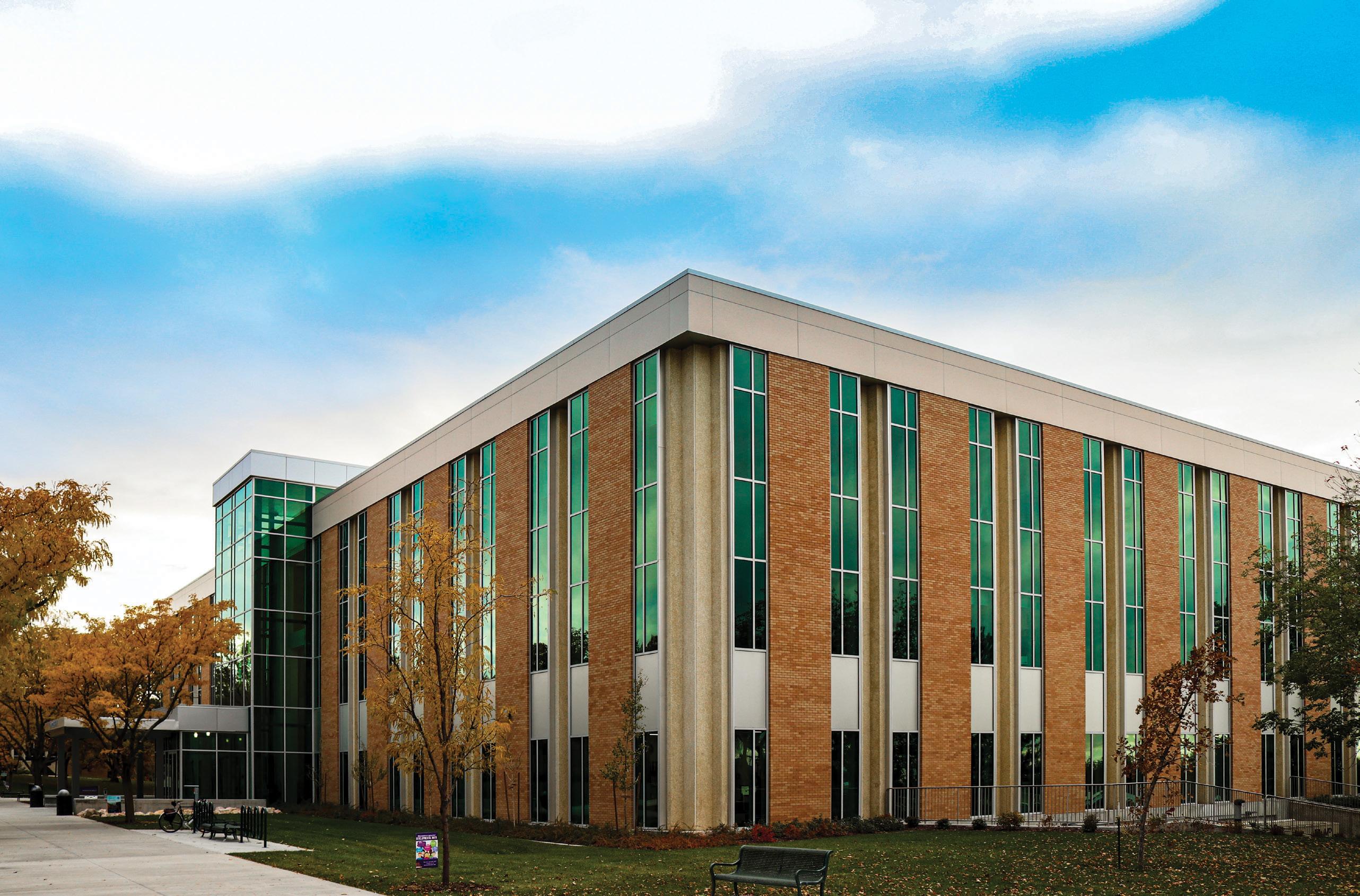
consisting of one floor below grade and three floors above, remained largely unchanged in its 45-year useful life.
The University’s wish was to completely remove and replace the antiquated mechanical and electrical systems within the building and redesign and reconstruct the architectural space to better serve the ever-evolving student population. Lindquist Hall, as the building would be renamed, is located in Northern Utah, within one-half mile of the Wasatch Fault and in Seismic Design Category D. An initial feasibility study was undertaken to determine an order of magnitude cost-estimate comparison of a complete remodel and seismic upgrade versus demolishing and replacing the entire building. The feasibility study resulted in a decision to keep the building, selectively demolish it down to the structural bones, and rebuild it. The electrical, mechanical, and civil consultants were tasked with designing completely new systems that complied with the 2015 International Building Code while fitting within the confines of the existing structure and the new architectural program, and minimizing impacts to as much of the existing building as possible.
The Structural Engineer of Record (SEOR) was responsible for developing and designing a complete seismic upgrade system for the building. Every floor level consisted of topped pre-cast double tees, and the roof consisted of un-topped single and double tee pre-cast joists. The joists were supported on a combination of cast-in-place and pre-cast girders that were in turn supported on cast-in-place and pre-cast concrete columns or the walls of two separate cast-in-place concrete interior stair towers and a mechanical shaft. These three concrete cores were the lateral force-resisting system for the building.
In addition to the full seismic upgrade, structural repairs, structural modifications, and new structural elements required for the

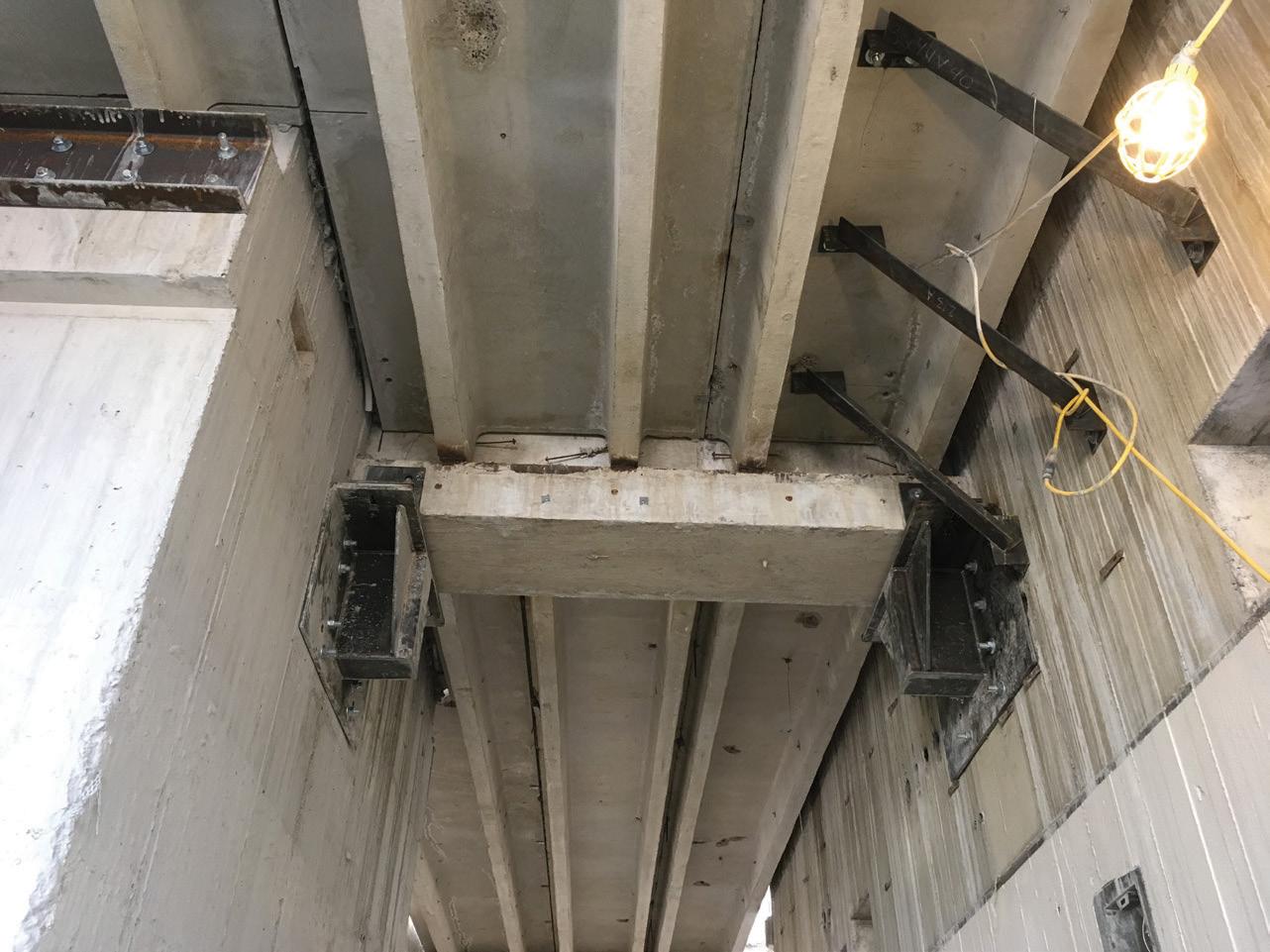
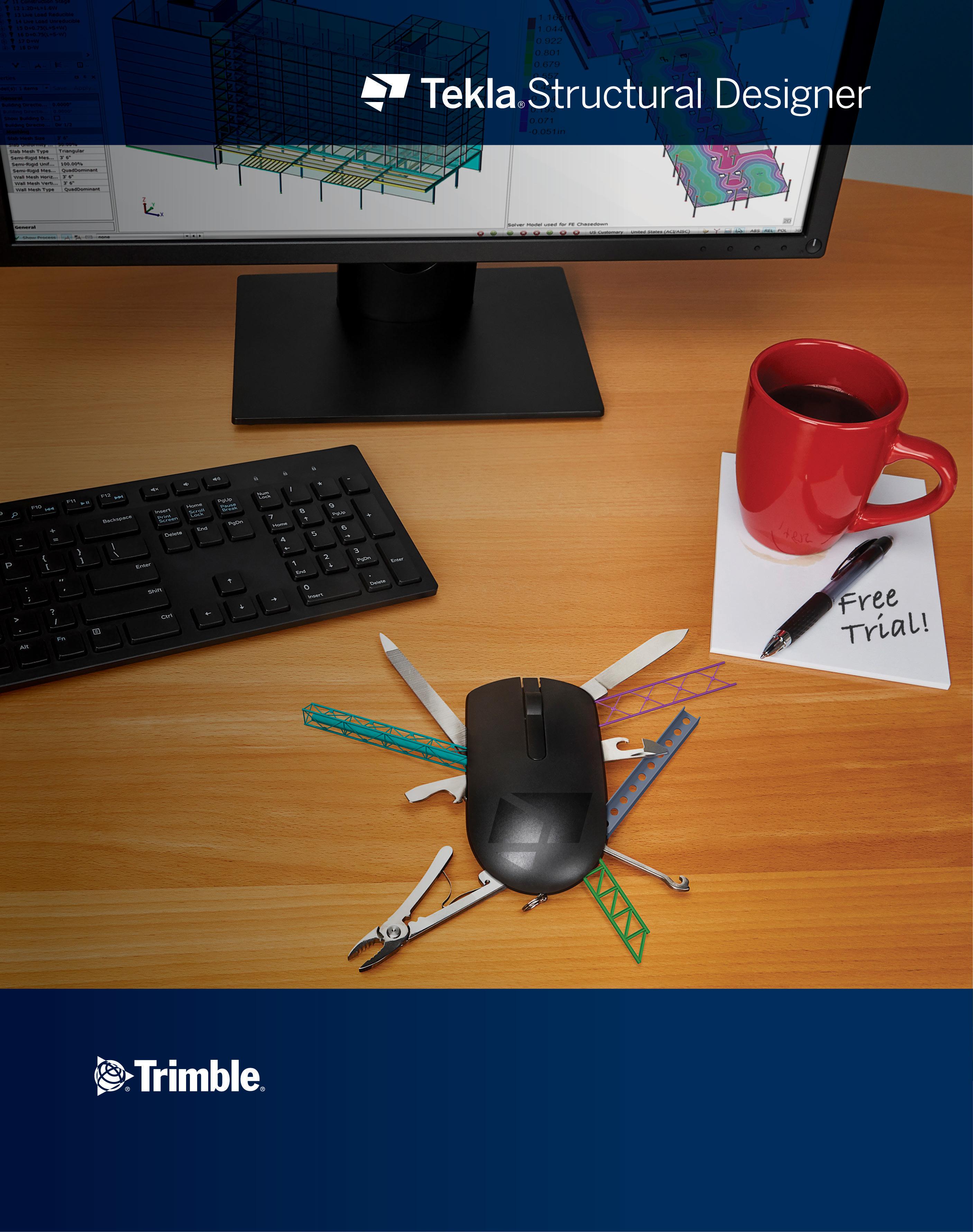
architectural program were designed and detailed. Some of these architectural elements included installing a new elevator shaft within the building footprint, removing two separate areas of floor structure near the building perimeter to create open continuity between multiple floors, and inviting natural light into the core of the building through strategically placed curtain wall systems. The building envelope was also completely replaced with metal stud, and a brick veneer system was placed outboard of the existing slab edge by approximately 16 inches, necessitating the extension of the floor slabs.
Several challenges were identified through an in-depth structural evaluation, and solutions were developed.
• The inherent lateral force resisting consisting of the core walls was insufficient in strength and ductility to brace the building.
Solution: De-couple the existing building from the existing shear wall system and replace it with a modern, ductile, well-performing buckling-restrained braced frame (BRBF) system. This task proved difficult because the concrete cores had to remain intact and support the gravity loads imparted by the concrete girders and joists connected to or bearing on the shaft walls.
• The building was extremely heavy, resulting in very large seismic forces.
Solution: Remove the existing, non-performing, un-topped, very heavy pre-cast double-tee concrete roof system and replace it with a light, steel roof framing system with a design-as-required new metal deck diaphragm with necessary chords and collectors. This solution served a double purpose by significantly reducing mass and facilitating the new design of an appropriate roof diaphragm.
• Existing pre-cast concrete cladding panels, and their connections to the structure, were determined to have insufficient strength and no ductility to resist out-of-plane seismic and wind forces.
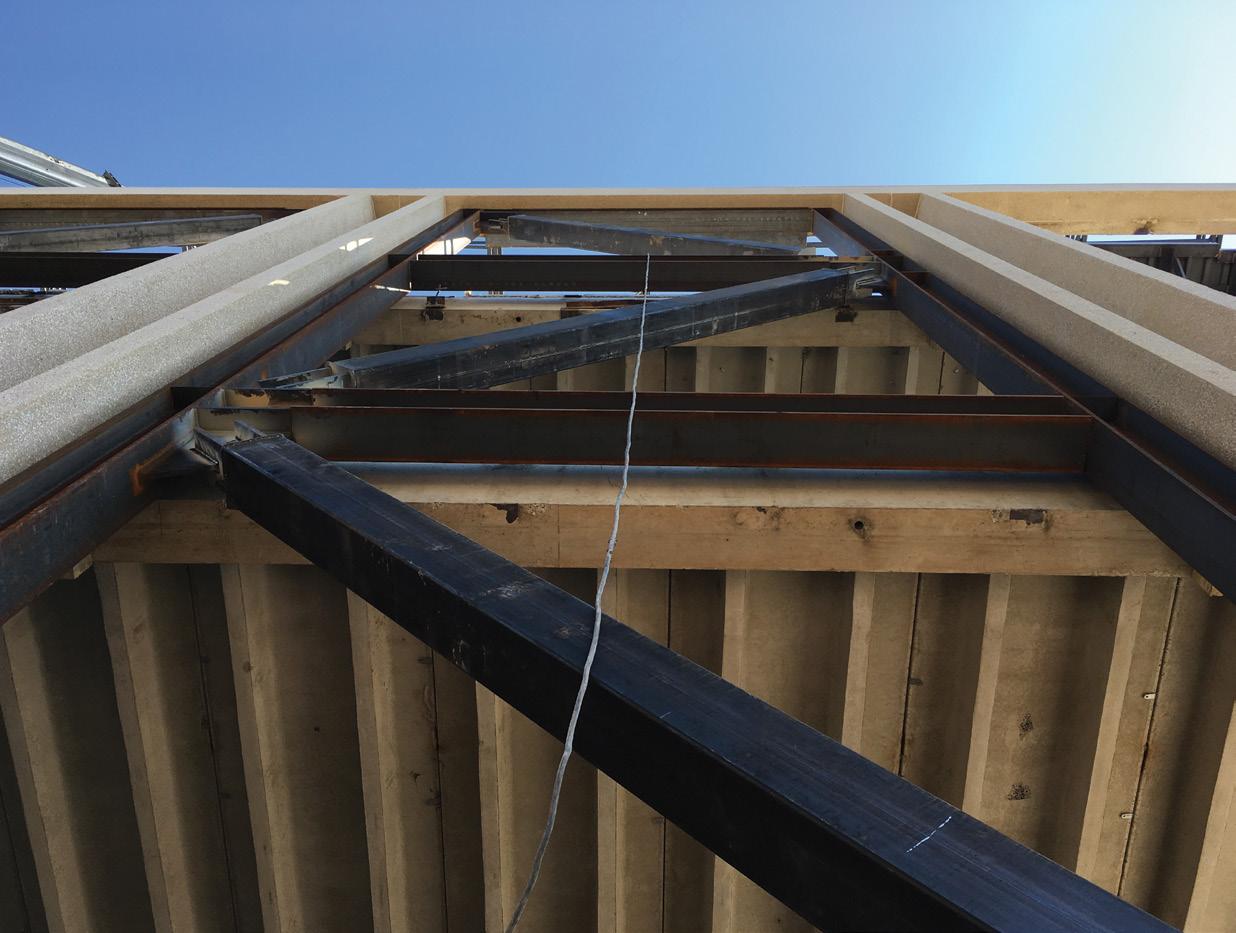
Solution: Remove all the cladding elements from the building and replace them with a combination of new brick veneer backed by cold-formed metal framing and curtain wall systems.
At the time of the initial design and construction, earthquake design was in its infancy, so seismic detailing, including adequate diaphragms with appropriate chords, collectors, and shear transfer elements, shear
Figure 3. A sample detail of one of the many girder support brackets.
wall designs with adequate reinforcing and boundary elements, etc., was essentially non-existent. Throughout the building’s life span, a plethora of MEP openings were added to the walls that compromised the integrity of the lateral force resisting system. An extensive predesign testing protocol identified concrete strengths and reinforcing patterns and was used to calculate existing floor diaphragm capacities. The diaphragm was determined to have adequate shear strength but lacked the required chords and collectors. Furthermore, the diaphragm lacked continuity across girder lines where the topping slab was poured flush with the tops of the girders. Therefore, carbon-fiber overlays were designed and installed to create chords, collectors, and diaphragm continuity across discontinuous planes where required on three floor levels.
The decision to remove the existing roof and replace it with a steel structure was also reviewed programmatically to determine the most economical solution. An independent cost estimate determined that the cost to remove the concrete and replace it with steel would result in a subsequent weight reduction and reduced seismic upgrade requirements. The decision to remove and replace the concrete roof with steel resulted in a cost savings of approximately $1,400,000.
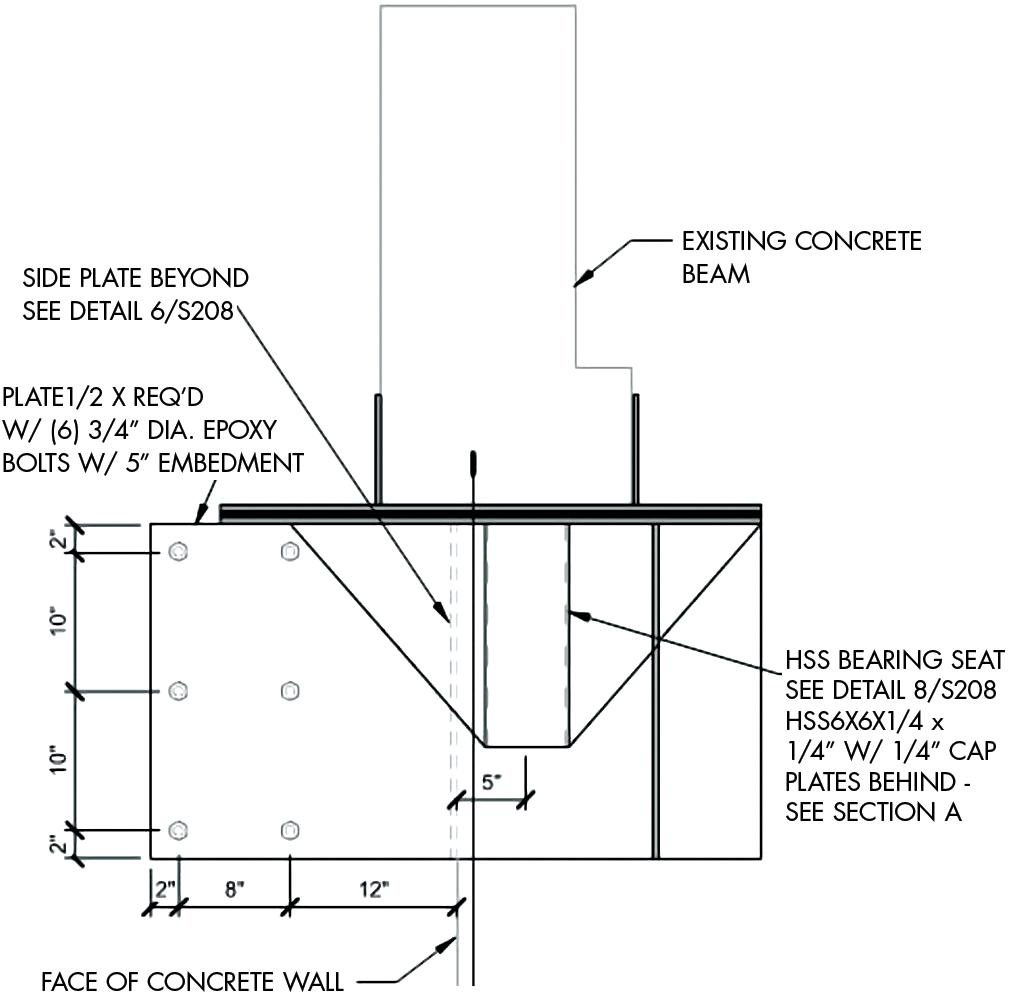
The existing shaft walls are effectively removed from the lateral force-resisting system to allow the new BRBF system to perform as intended. The amplified lateral drift was determined at each level; the intent was to free the upgraded structure from the shaft walls. To accommodate this, while the floor’s shafts actively supported joists and floor girder loads, each girder was cut back to allow up to 3 inches of lateral movement in any horizontal direction at the floors while maintaining gravity support of the in-place floor dead and subsequent required live loads. In addition, the structure was designed to pass over and rest upon the concrete cores at the roof level while allowing up to 5 inches of lateral movement in any direction at the roof level.
The complex design to support the concrete girders involved a double slide bearing system. Large epoxy-bolted brackets were designed to attach to the core walls with robust bearing seats to support concrete beams that now terminate up to 3 inches from the wall. The double
slide bearings consist of Teflon slide bearing brackets positively connected to the beams and slide bearing plates inversely connected to the bearing seats that allow for necessary slide in two directions while maintaining gravity load-carrying ability.
To justify this approach, the existing concrete stair towers and mechanical shaft were analyzed to verify their ability to free-stand within the building, not to impart lateral loads to the structure, or, inversely, to take lateral loads from the structure.
De-coupling the existing building from the existing concrete cores proved to be a complex task. The girders connecting to the shafts and the shafts themselves are all cast-in-place and essentially poured monolithically. The girders are inverted ‘T’ shapes where the pre-cast tees rested upon the the T's horizontal leg. Because there are many different bearing and connection conditions where the girders support different tributary lengths/areas, the centroid configuration of each girder connection to the shaft was inconsistent in its placement, and the amount of drift accommodation was different at each level of each girder, the reaction does not necessarily coincide with the centroid of the shape. Because of these nonuniformities, each girder bearing end condition is different, and each new steel plate bracket is custom designed specifically for each condition (Figures 1 (page 26) and 2).
Figure 4. Roof collector at BRBF beam.
Not only are the girders cut loose and re-supported, but diaphragms are released and detailed to accommodate a similar range of lateral movement. Details were designed and systems installed to ensure the concrete towers were independent. P-delta analyses were also conducted to verify that existing columns would remain stable under the lateral drift of the new system.
Due to the configuration of the existing concrete columns and girders at the building perimeter, all of which were pre-cast and were to remain unchanged, the new braced frame system is designed to fit inside the column/beam envelope (Figure 3). The precast concrete column and girder system is outboard of the original floor plate and serves as exposed elements in the finished building. Because the new frames are intended to sit in-plane with the girder and beam system, the floor plates have been extended up to 16 inches outward to connect, collect, and transfer required forces and tie the BRBF frames to the building for out-ofplane loading. In addition, complex steel systems have been designed to transfer diaphragm collector forces from the new flexible roof, below and out-of-plane, to new braced frame beams that transfer lateral forces to buckling restrained braced frames (Figure 4 ).
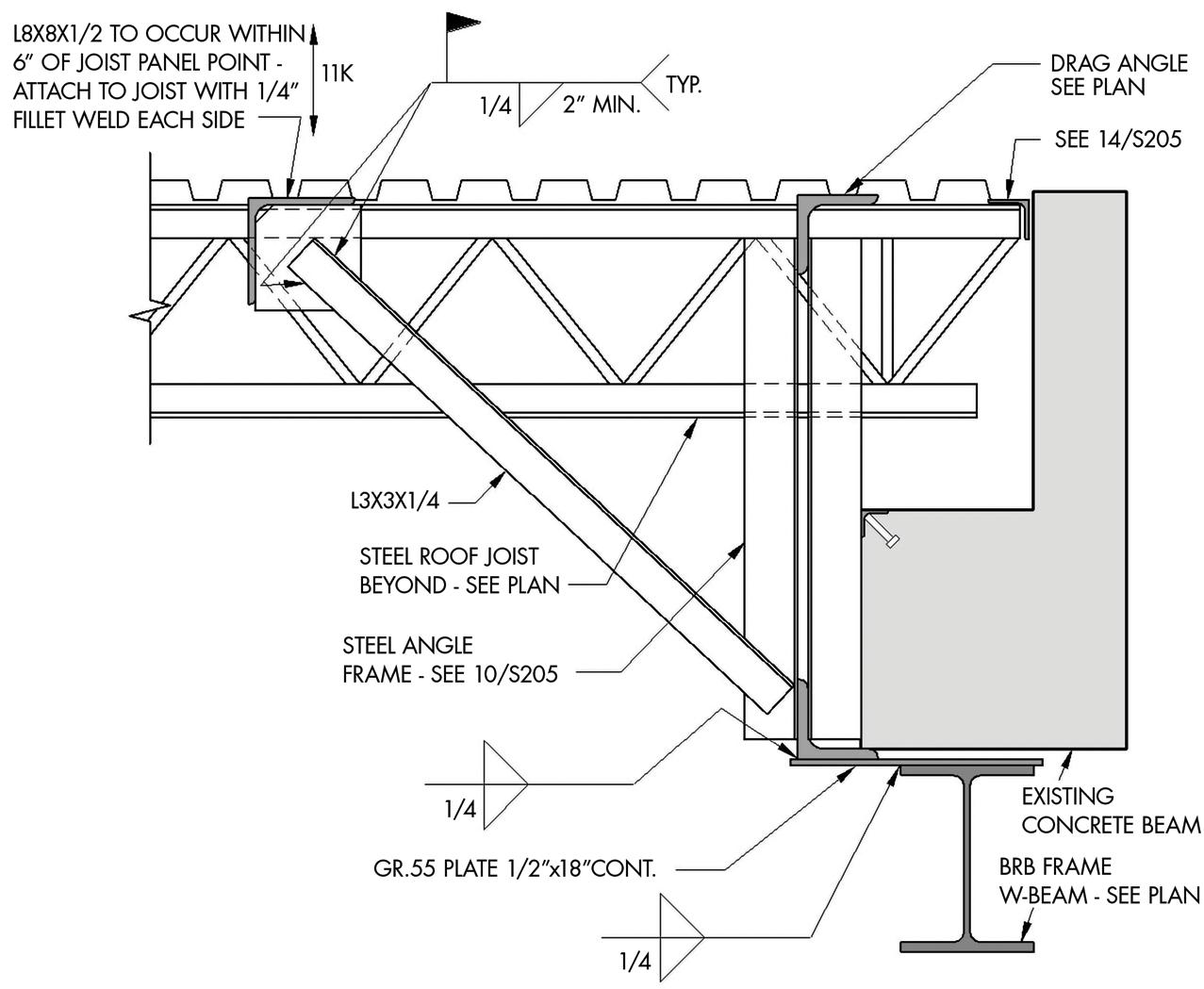
The new braced frame columns are connected at their base to concrete grade beams strategically keyed into the existing concrete foundation walls. The column connections are designed so that once the shear forces within the brace are transferred into the grade beams, they are distributed throughout the entire foundation wall (Figure 5).
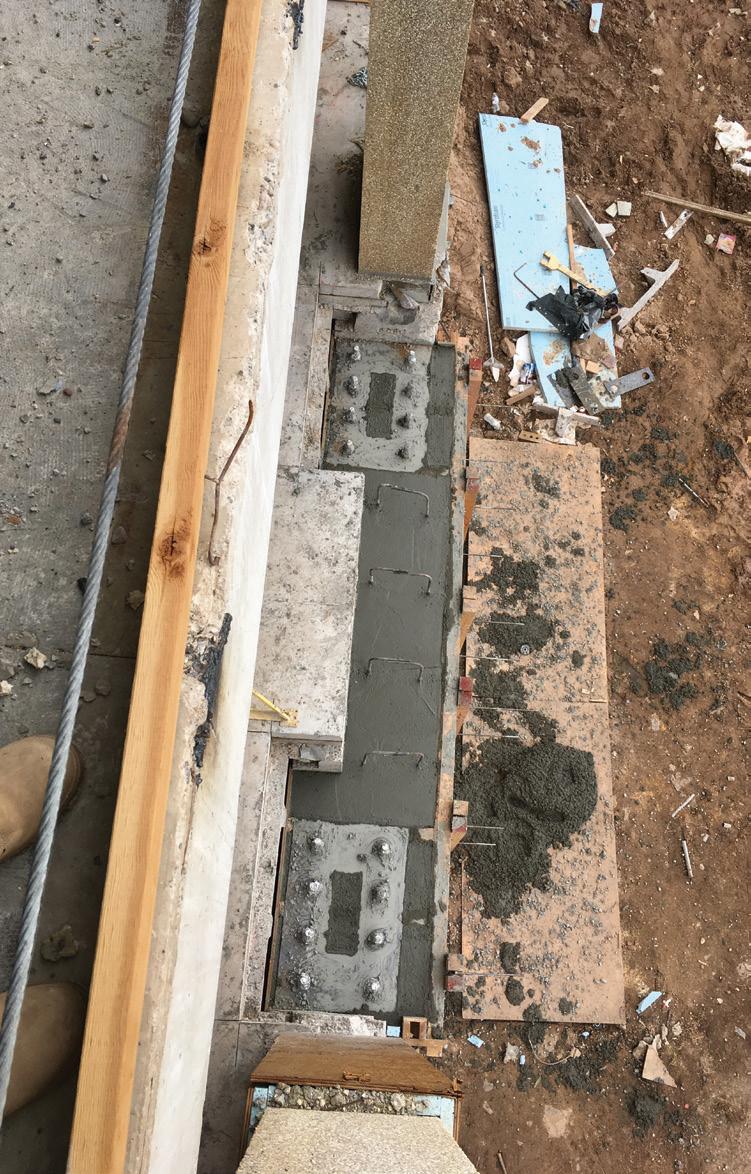
Because the existing building is supported entirely on cast-in-place concrete piles, a finite element model was created for the entire foundation wall system. The overturning and shear forces from the braced frames and required gravity loads were applied to verify individual pile strength. Each pile’s vertical and lateral capacity was ascertained by working closely with the project geotechnical engineer and utilizing existing building drawings and original design data. The reduced dead loads from the roof replacement offset the increased seismic loads from the braced frames, and it was determined that new pile loads would be within the capacity of the individual piles.
For its first 45 years, Lindquist Hall at Weber State University, as it is now referred to, stood as one of the most prominent, heavily used, and recognizable buildings on the campus. With the completion of this successful project in 2019, Weber State continues its outstanding legacy of creating modern examples of stateof-the-art, resilient, and sustainable structures on campus for many years to come.■
(jeremya@arwengineers.com).
Project Team
Owner: Weber State University
Structural Engineer: ARW Engineers
Architect: GSBS Architects
Contractor: Big-D Construction
Steel Fabricator: AMFAB Steel Specialties, Inc.
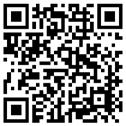
HAWAII STATE HOSPITAL’S NEW PATIENT FACILITY
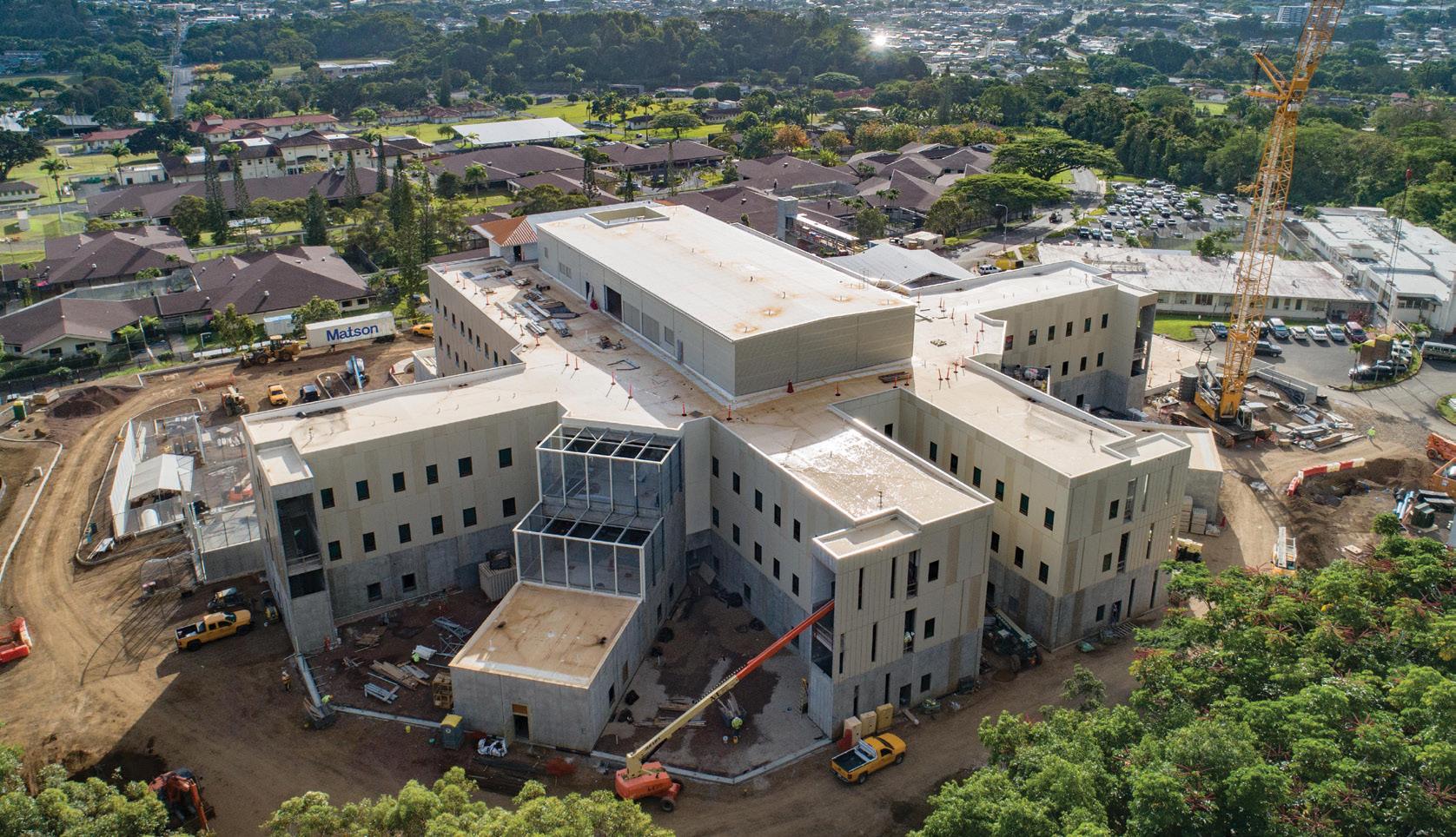
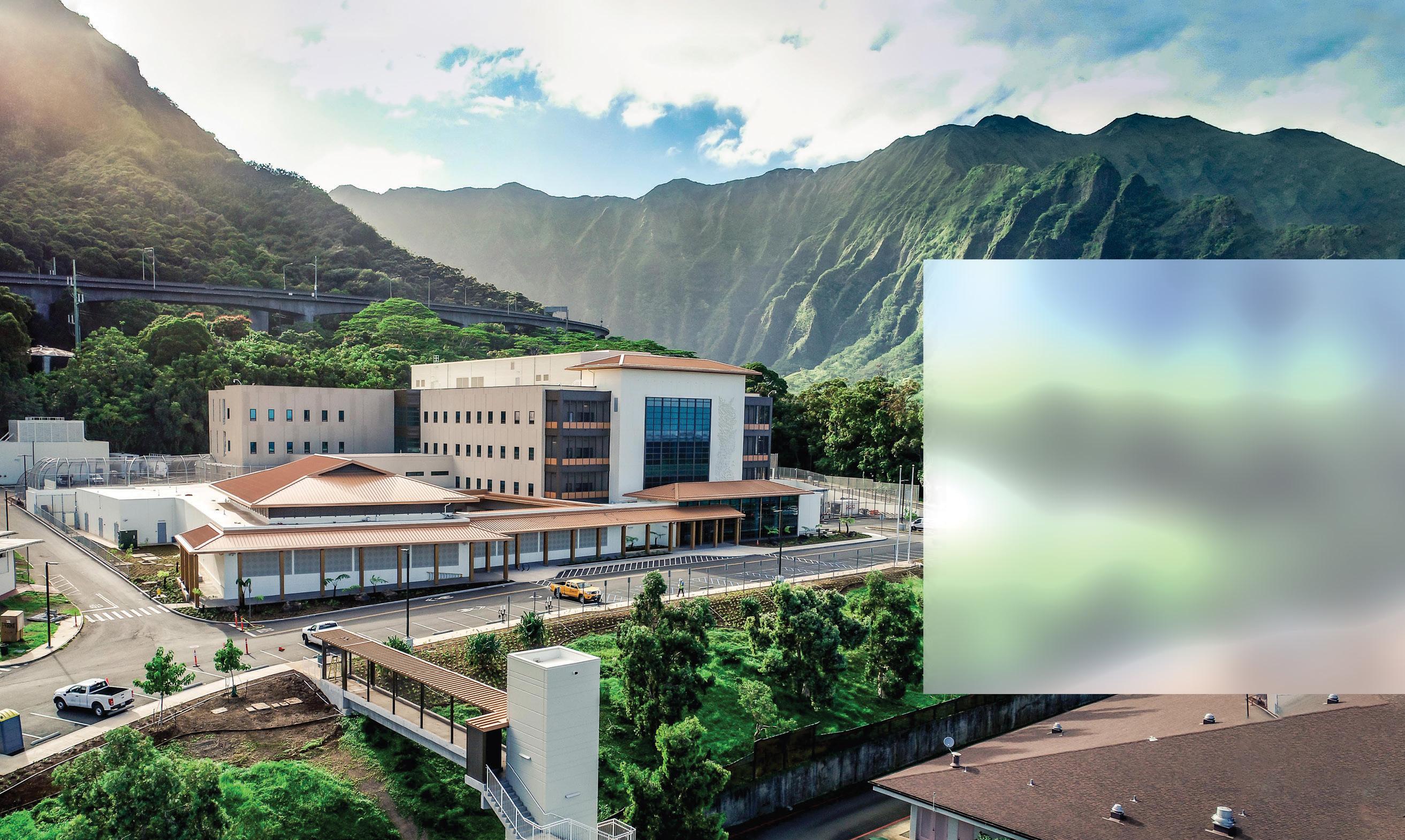
The $160 million New Patient Facility at the Hawaii State Hospital is a four-story, 144-bed, 196,944-square-foot secured psychiatric facility on an existing psychiatric hospital campus. At the foot of the Ko’olau Mountain Range, this facility includes patient care units, a rehabilitation mall, multiple office spaces, a standalone central utility plant, a separate gymnasium for recreational therapy, and a site elevator with a walkway bridge structure. Due to the ever-increasing patient population and a high percentage of high-risk forensic patients, the existing hospital could not keep up with the demand, and a larger facility was needed. e project was executed using the design-build delivery method led by Hensel Phelps.
Building Description
e 63-foot-tall main building measures 310 feet in the north-south direction and 384 feet in the east-west direction. e building’s footprint is not the typical rectilinear shape. Instead, six patient wings extend out from the center core of the building (Figure 1). e separated patient wings were formed in such a way to provide protection and privacy for select groups such as women, the elderly, and violent patients.
e ground floor spaces serve various functions, including a chapel, courtroom, library, multiple offices, art rooms, kitchen and dining spaces, and a secured outdoor rehabilitation mall. Levels 1 through 3 consist of wings for patient living and multiple office spaces.
e design follows the 2006 International Building Code (IBC) with amendments by the City and County of Honolulu. With a building classification of Occupancy Category III, the building is designed for a basic wind speed of 105 mph and a Seismic Design Category C. Due to the building’s irregular shape, a modal response spectrum analysis was performed to determine the seismic loads on the structure.
Structural System
e project site is where an existing hospital building was demolished. e building foundation consists of shallow spread footings on in-situ residual and saprolite soils. Upon excavation, it was discovered that the excavated soil could not achieve the same
strength characteristics of the in-situ material and could not be used as structural fill. Instead, the excavated soil was mixed with crushed concrete to attain the soil bearing capacities required for the loads imparted by the building.
The facility must be highly resilient to protect the patients, staff, visitors, and greater community from environmental and man-made threats. Therefore, the primary structure must be capable of resisting forces from earthquakes, hurricanes, and the continuous onslaught of a corrosive tropical environment. In addition, the structure must be of durable construction that minimizes future maintenance but still provides an aesthetically pleasing form. The original concept for the facility utilized load-bearing CMU walls in place of precast concrete walls at the facility’s exterior and all interior stair/elevator cores. Although slightly more costly than CMU, precast concrete walls saved considerable construction time and were the best choice for Hawaii’s remote location.
The primary structure consists of load-bearing exterior precast concrete walls and interior precast concrete columns supporting a floor system with precast concrete hollow core planks at typical locations and double tees at heavier loaded areas with longer spans (Figure 2). Precast concrete facilitated construction by allowing portions of the building to be fabricated offsite, then delivered and assembled on-site with minimal interruption due to weather. It also provides an efficient structural system with the strength to support all floor/ roof loading, including the weight of substantial non-load bearing concrete masonry partition walls, and the rigidity to limit deflections and control perceptible vibrations. Furthermore, precast concrete is an inherently durable material detailed to withstand the corrosive tropical environment of Hawaii and ease future maintenance. The building foundation consists of shallow spread footings such as isolated column footings, continuous wall strip footings, and mat footings at elevator cores. The roofs of the mechanical and elevator penthouses consist of a bare metal deck supported by structural steel beams. In contrast, the gymnasium roof consists of a bare metal deck supported by structural steel trusses.
The lateral force-resisting system mainly consists of intermediate precast concrete shear walls. The structural diaphragm, which distributes and transfers laterals forces to the shear walls, consists of a 4-inch cast-in-place concrete topping at typical floors and a 3-inch cast-in-place concrete topping over precast framing at roof diaphragms.
Constructability and Design Challenges
Located in the city of Kaneohe on the east side of Oahu, the site receives a substantial amount of rain and moisture. On-site CMU construction would have unacceptably delayed the project. The use of precast concrete allowed the project schedule to be maintained and ultimately saved about six months on the schedule.
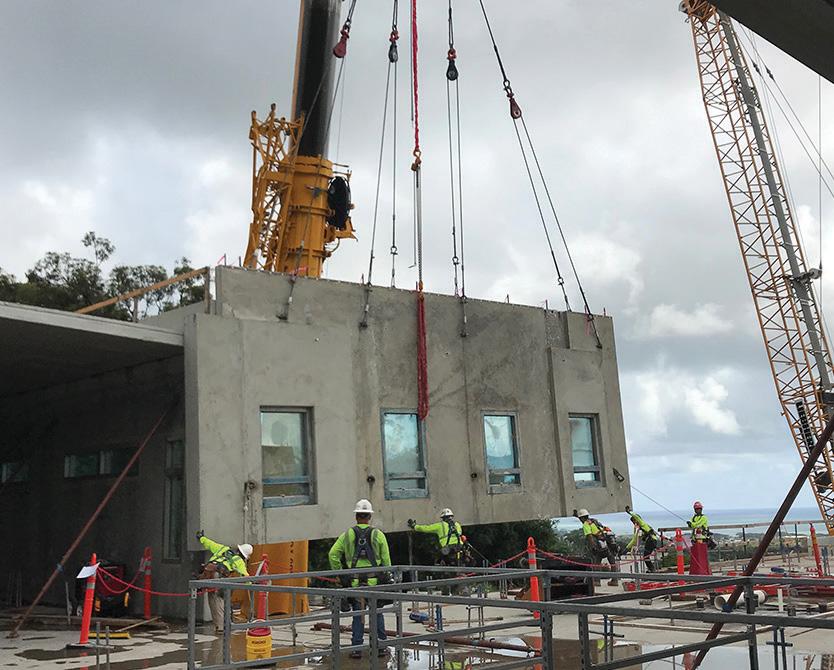
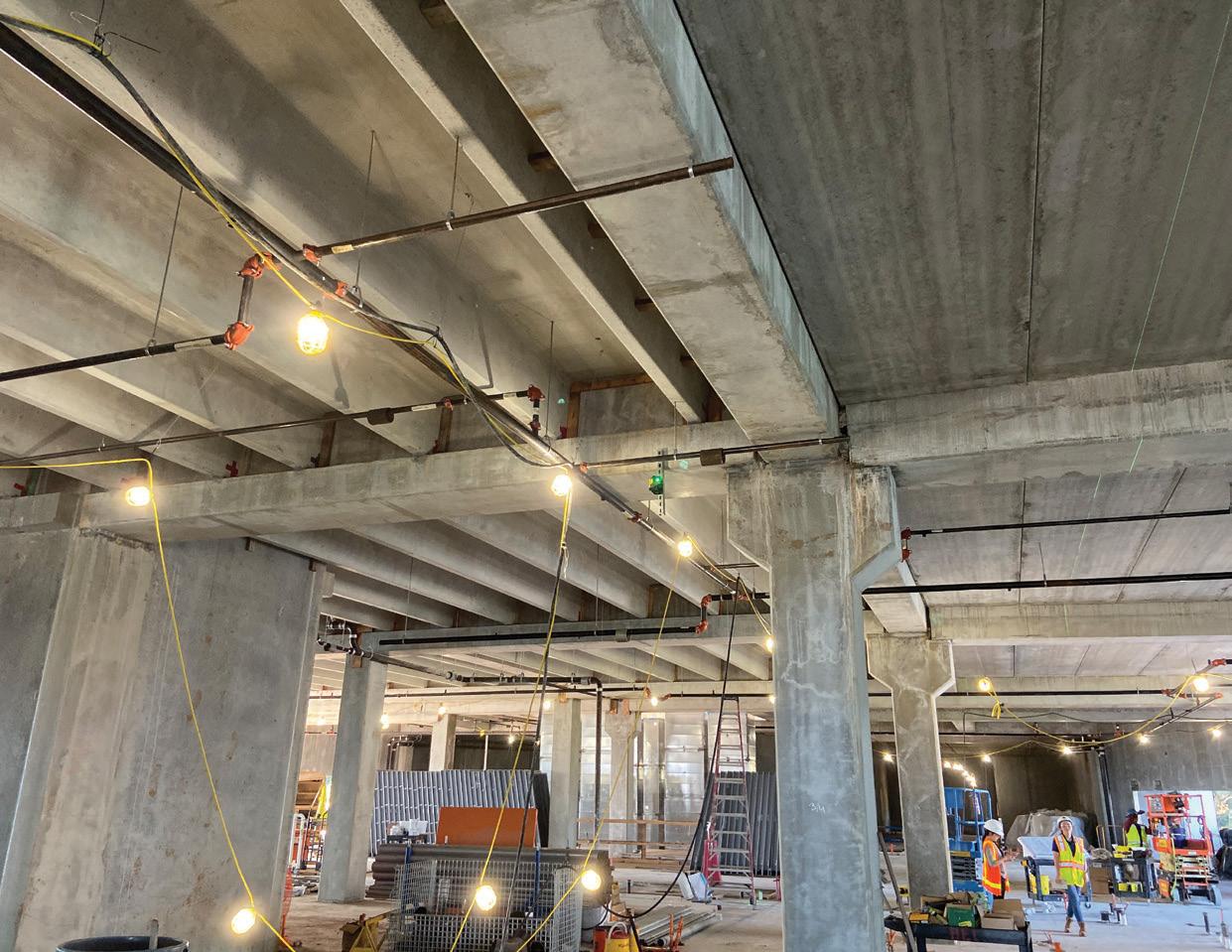
Precast concrete was also the best choice for Hawaii’s remote location. Other gravity load-resisting systems (structural steel and cast-in-place (CIP) concrete) were evaluated for this project, but precast concrete was the optimal choice to meet budgetary and schedule restraints for several reasons. Precast concrete is locally sourced and manufactured, whereas structural steel must be fabricated on the mainland and shipped to Hawaii. Any major field issues during erection are much more difficult and time-consuming to address with structural steel since required materials may not be locally available. The need for fire-proofing was also a drawback for structural steel. The factors mentioned above and potential delays due to shipping made the locally produced precast a more attractive option. Like precast concrete, CIP concrete is locally sourced. However, with the wet and rainy conditions at the site, cast-in-place concrete construction faced schedule issues similar to those with CMU, such as fewer available days for construction.
Several structural challenges were efficiently addressed with the use of precast concrete. The Occupancy Category III structure is subjected to moderate to high seismicity, hurricane-force winds, and a corrosive tropical environment. The use of load-bearing precast concrete walls at the exterior of the facility simultaneously addresses these forces and the environmental factors. The intermediate reinforced precast concrete walls are designed as shear walls for resistance to in-plane earthquake (and hurricane) forces. The walls also resist out-of-plane hurricane-force winds and are detailed to withstand Hawaii’s climate with minimal future maintenance. The climate and tropical environment in Hawaii facilitate accelerated corrosion, partly due to the higher amount of chlorides in the atmosphere and the humidity. Several measures were taken to slow down the corrosion rate, such as using hot-dip galvanized steel when exposed to weather or in unconditioned spaces, using zinc-rich paint coating where field welds could not be avoided, and applying multiple coats of elastomeric coatings at exterior faces of the precast concrete walls. In addition, the precast concrete mix used very low w/c ratios (w/c = 0.35) and included fiber reinforcement to help increase the concrete durability. Precast
concrete wall panels were also provided with prestressing strands to help control cracking.
Security for patients, staff, and the community is a critical design consideration for a psychiatric hospital. The exterior shell of the facility (i.e., all exterior walls and roofs) had to meet stringent security requirements such as limitations to reinforcement bar spacing as required in the Facility Guidelines Institute’s (FGI) Guidelines for Psychiatric Hospitals and the FGI Design Guide for the Built Environment of Behavioral Health Facilities. Achieving the requirements for vertical enclosures using load-bearing precast concrete wall panels was very efficient. The size and spacing of the reinforcement steel were slightly adjusted to meet the security requirements, but this did not add any appreciable cost to the panels. Precast concrete eliminated the need for thick steel security panels at the exterior of the building, which would be required if non-load bearing lightweight framing was used. Furthermore, the floor system of 8-inch-thick hollow-core precast sections with cast-in-place concrete topping satisfied the security requirements without any additional enhancements.
Another design challenge was creating an exterior aesthetic that presents a non-institutional, non-threatening character sensitive to public perceptions and responds to Hawaii’s climate, environment, culture, and customs. The precast concrete wall panels provide visual interest, scale, and delight through various configurations and finishes. Form liners were used on the panels along the main walkway at the front entrance to introduce a Hawaiian Piko pattern. Piko in Hawaiian is another word for the navel where life begins, and the symbol brings new life and purity to the world. It also represents peace, tranquility, spirituality, and a strong sense of regrowth or new beginnings. The intricate repetitive design cast into the precast panels creates a unique Hawaiian sense of place for all those entering the facility.
It was crucial that this high-security facility integrate into the architecture of the existing surrounding hospital buildings and that the building’s overall form was sensitive to the neighboring community and displayed a Hawaiian sense of place. The use of precast concrete wall panels with various finishes and reveal patterns accomplished this goal without compromising strength, durability, or security.
Innovations and Accomplishments
This was the first project that the precast manufacturer (GPRM) cast the window frames, including the glazing, into the panels with the concrete pour. In researching other precast producers, this may have been the first time this was done with the glazing already in the frame. This technique required a new and enhanced level of
attention in planning, detailing, and executing the precast fabrication work to protect the glazed window units and circumvent breakage during production, handling, transporting, and erecting the panels (Figure 3, page 31). This novel approach to installing the window units compressed the schedule and allowed the building to be enclosed from the elements much quicker. With the windows cast together with the wall panels, a higher level of quality control than field installation was achievable, enhancing the interface’s quality and durability between windows and walls.
In addition, the prefabricated security window assemblies are operable, allowing for natural ventilation while incorporating enhanced security features such as special handles that cannot be used for self-harm and that eliminate the possibility of passing contraband or throwing things from windows.
The standalone site elevator/stair tower and bridge structure are simple but unique designs allowing staff and guests to access the new facility from the lower campus levels. The elevator tower consists of stacked precast concrete wall panels forming a core. A 32-inch-deep precast double tee bridge spans from the elevator tower to the upperlevel abutment. The bridge is covered by a lightweight steel canopy supported by the bridge structure. The precast concrete stairs wrap around the elevator tower and are supported using flag walls, concrete wall segments cast with the wall panel that cantilever from the panels. See Figure 4 for the bridge structure and site elevator/stair tower with flag walls. The unique flag wall design eliminated exterior support columns, which would have been difficult to install within the tight footprint, and reduced the cost of the tower.
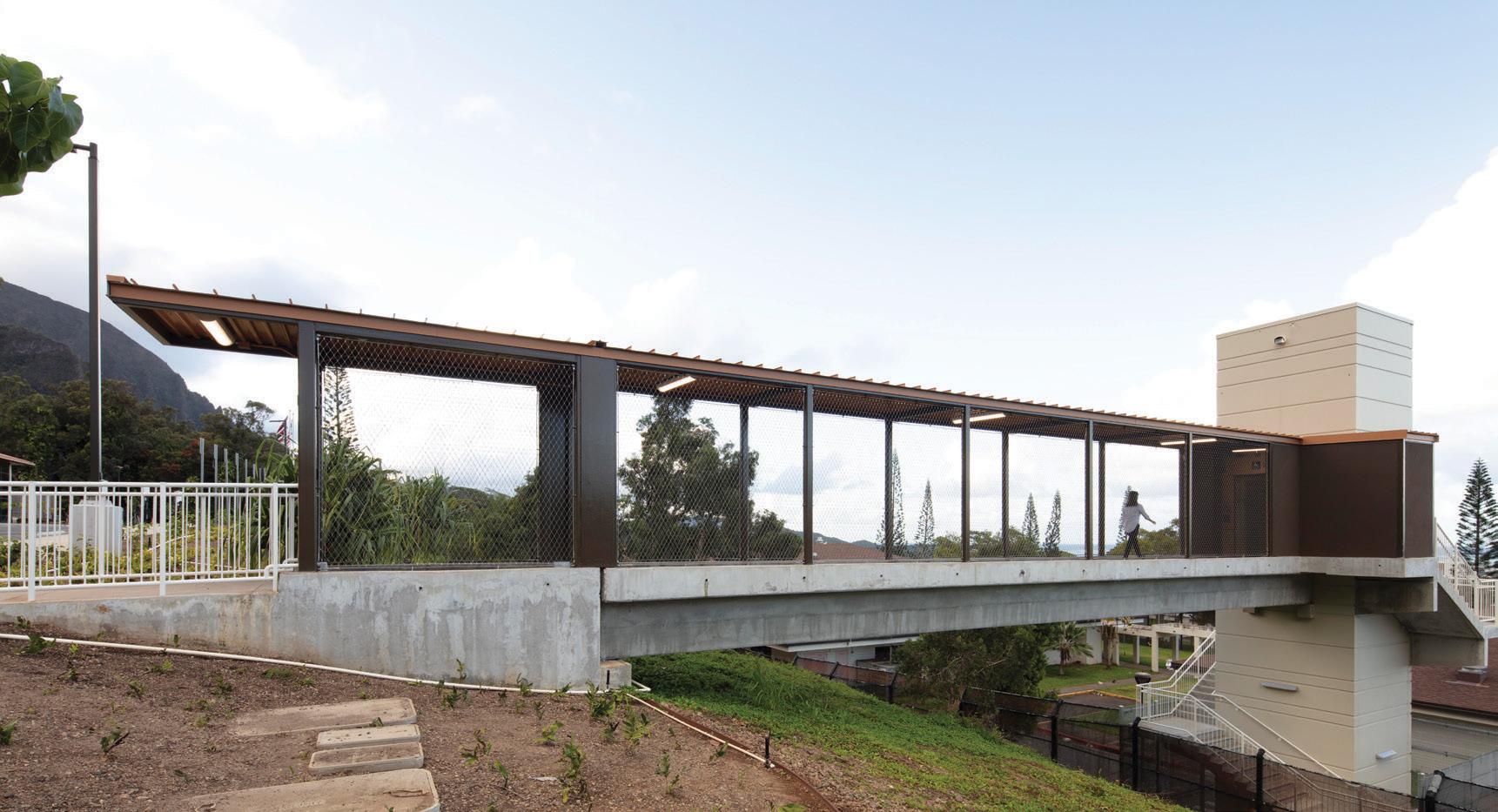
Conclusion
Through the constant collaboration of the design-build team and the adaptability afforded by the design-build delivery method, the construction of the Hawaii State Hospital New Patient Facility was completed on time and within budget despite the challenges faced. Although not evident initially, the precast option with a higher material cost resulted in an efficient design, saving the project time and other associated costs inherent in concrete properties. It is not always the lowest cost structural system that produces the most efficient and economical design. This also highlights the benefits of the design-build process. Without the Contractor’s input, there would have been significant impacts on the schedule, and structural work may not have gone as smoothly.
■
Kevin Galvez is a Senior Structural Engineer at BASE (kgalvez@baseengr.com).
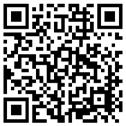
Frank K. Humay is Vice President at BASE (fkh@baseengr.com).
Owner: State of Hawaii, Department of Health
Structural Engineer of Record: BASE
Architect of Record: G70
General Contractor: Hensel Phelps
Precast Concrete Supplier: GPRM Prestress
ACI 318 PLUS
SUBSCRIBE TODAY: An annual subscription that provides users with digital interactive access to ACI CODE-318-19, “Building Code Requirements for Structural Concrete and Commentary,” along with in document access to related resources and enhanced digital search features through all code provisions and commentary.
Includes full digital interactive access to the ACI Detailing Manual and the ACI Reinforced Concrete Design Handbook, subscribers can make digital notes alongside ACI CODE-318-19 provisions and commentary, and navigate content by section, by chapter, and/or by provision.
For access and to subscribe, visit www.concrete.org/ACI318
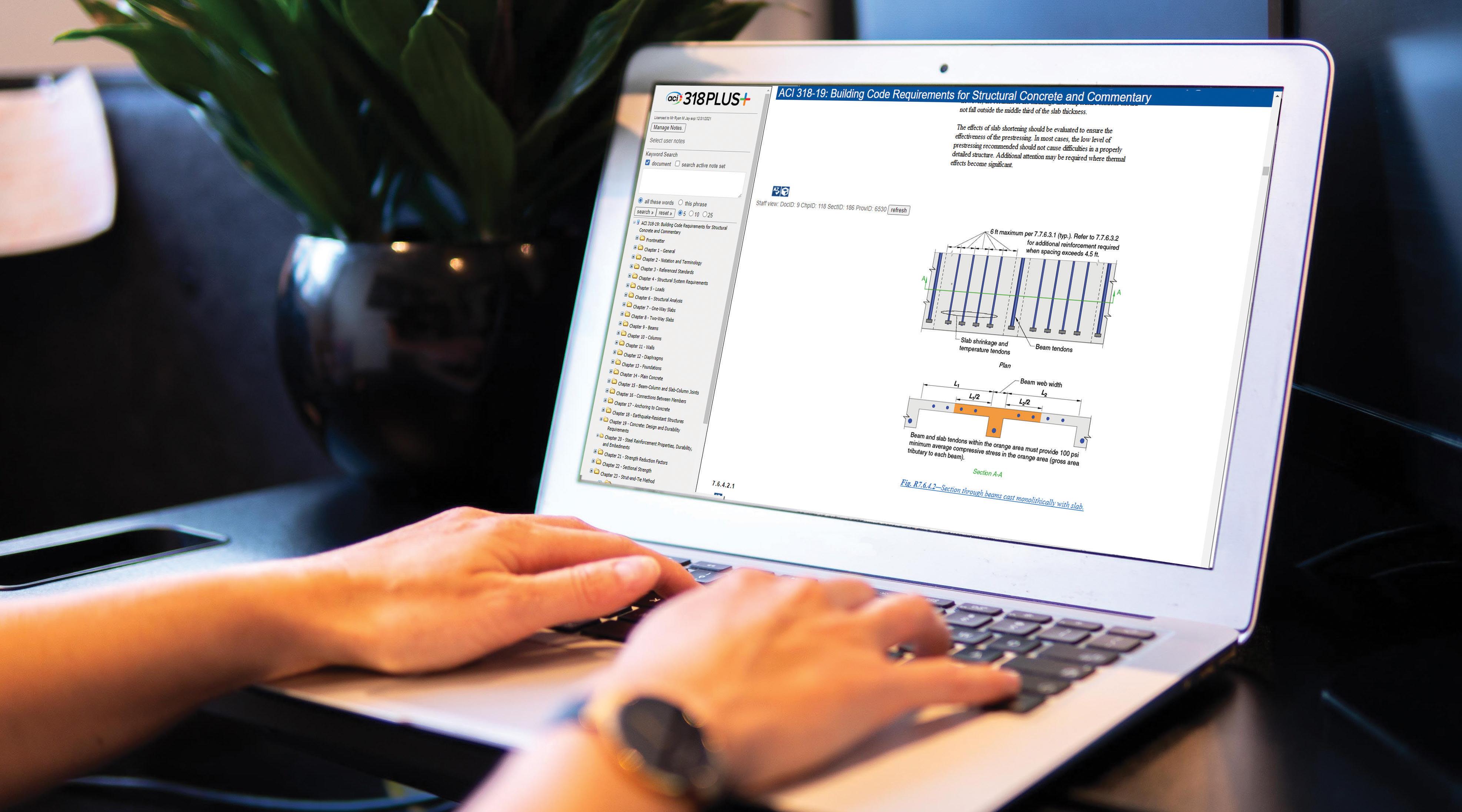
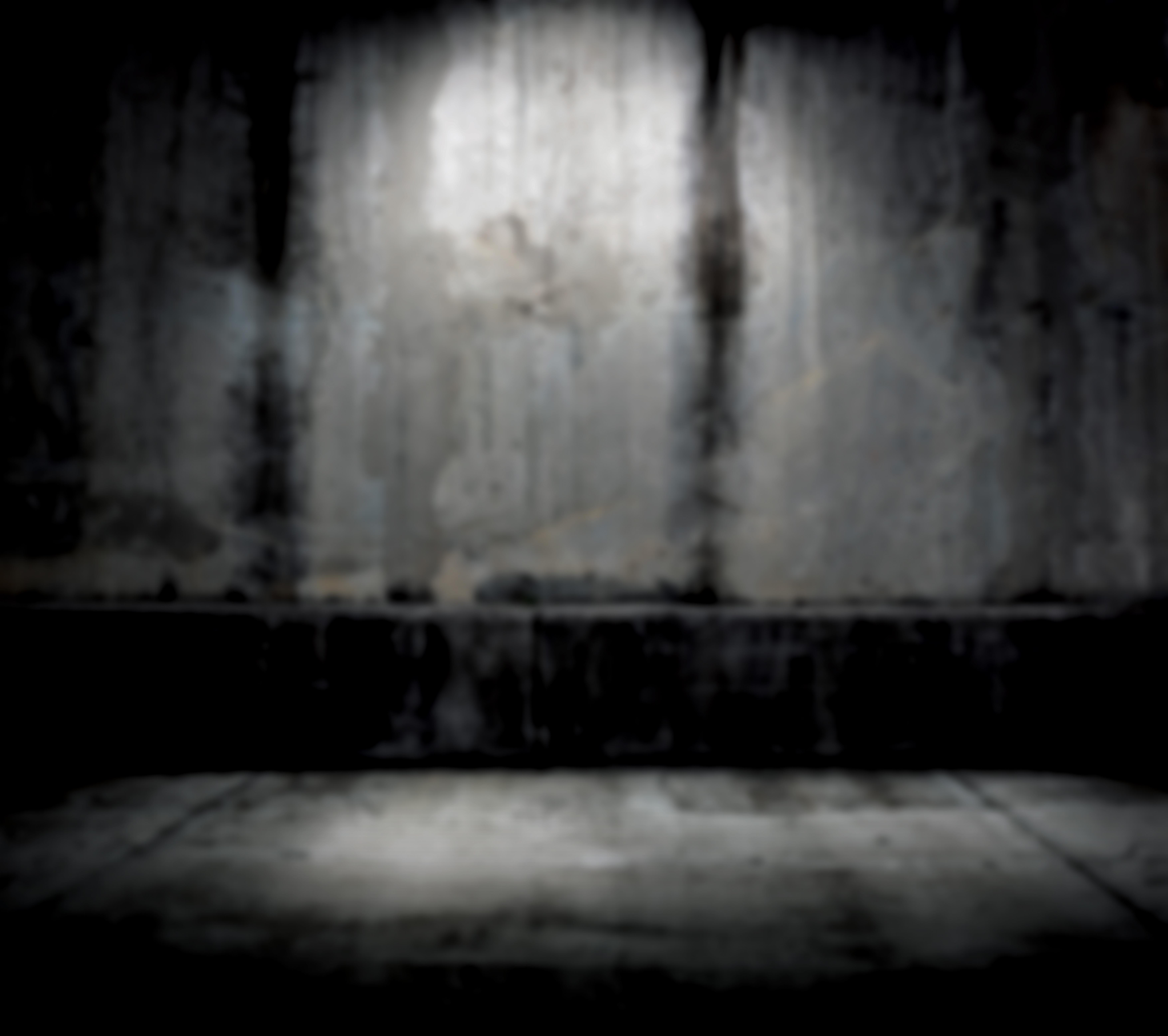
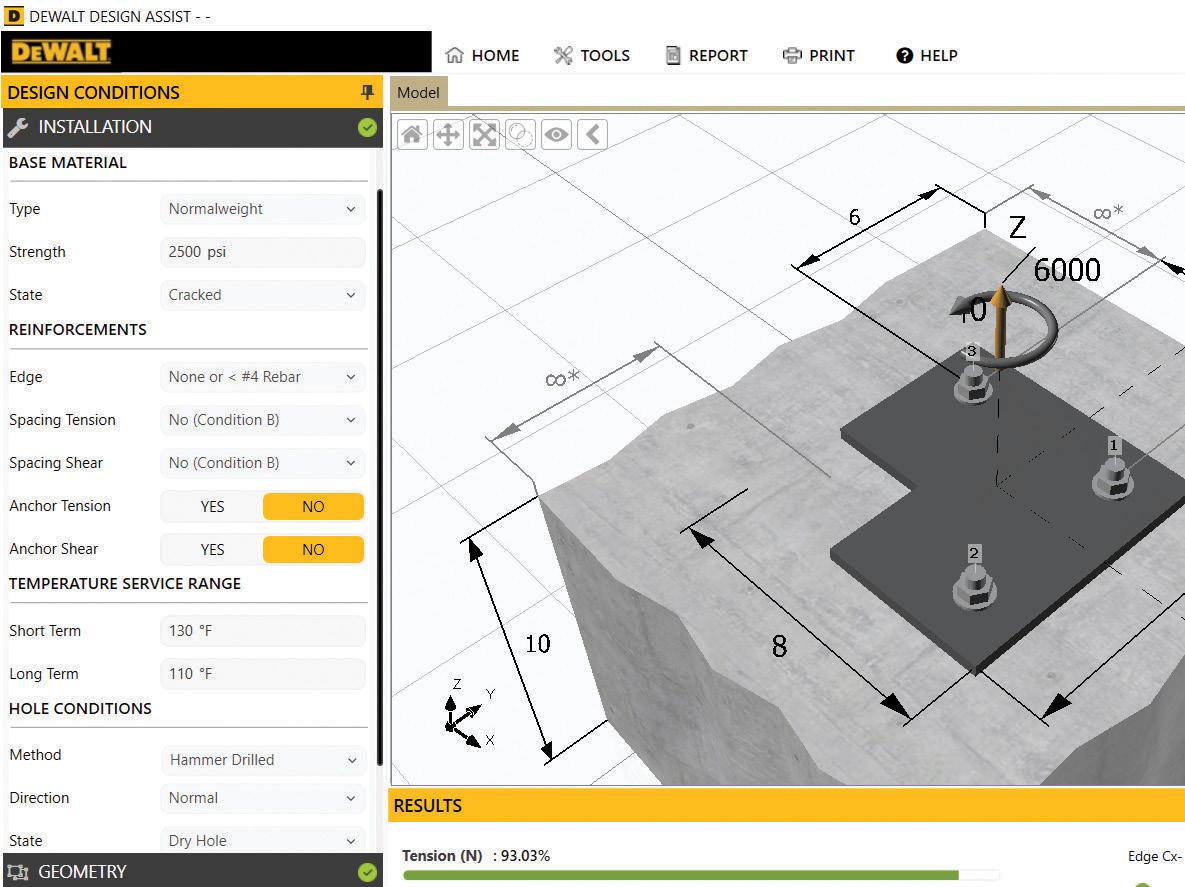
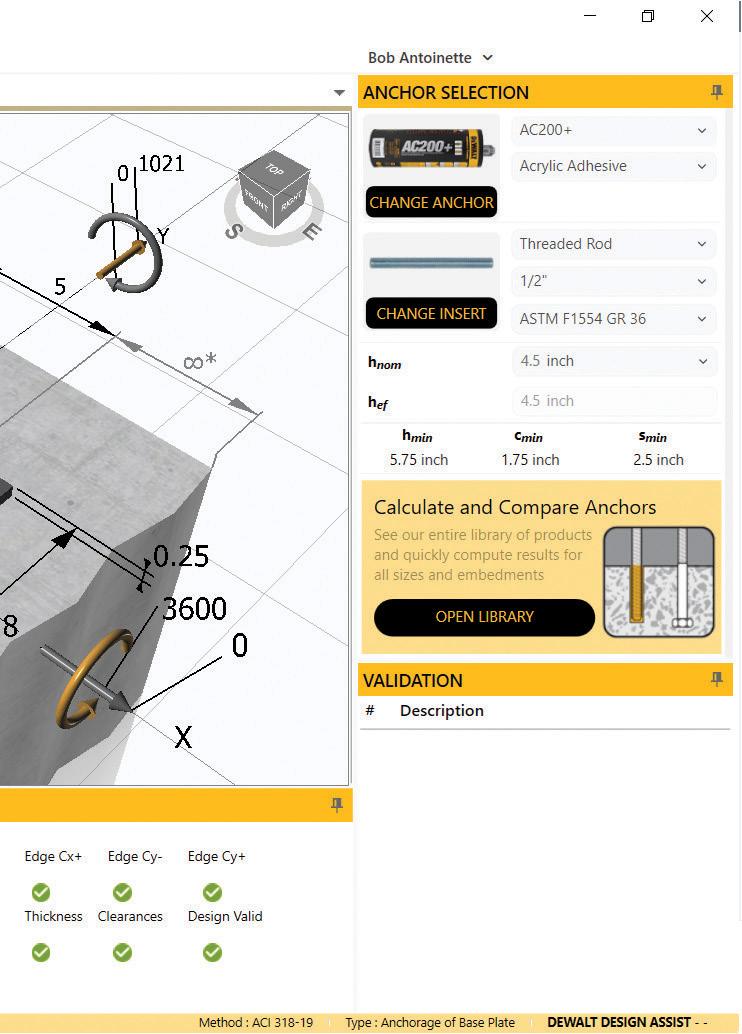

SOFTWARE CONCRETE ANCHORS & FASTENERS
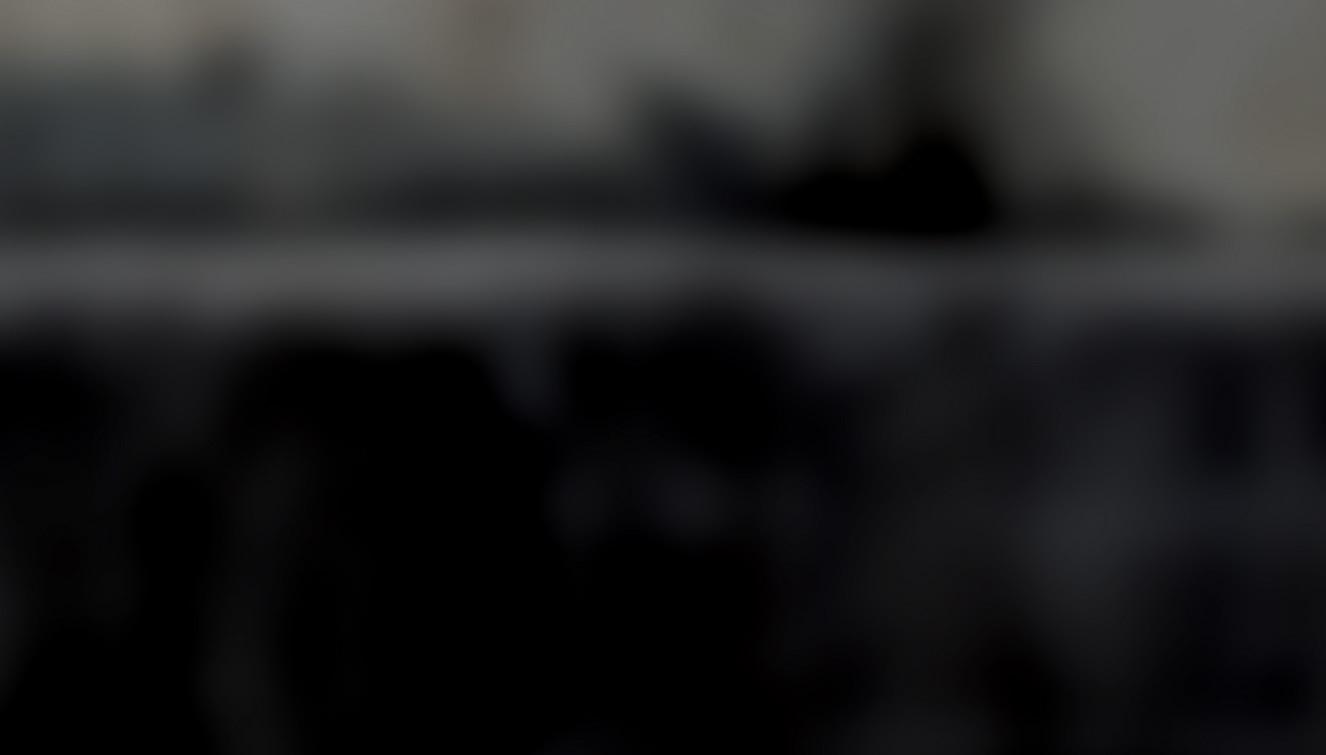
KEY FEATURES AND BENEFITS
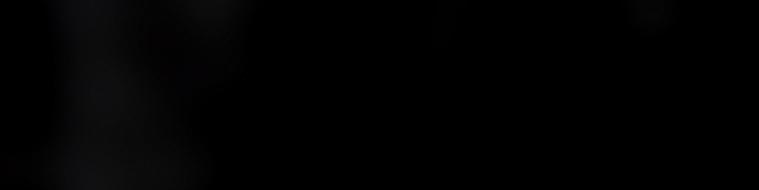
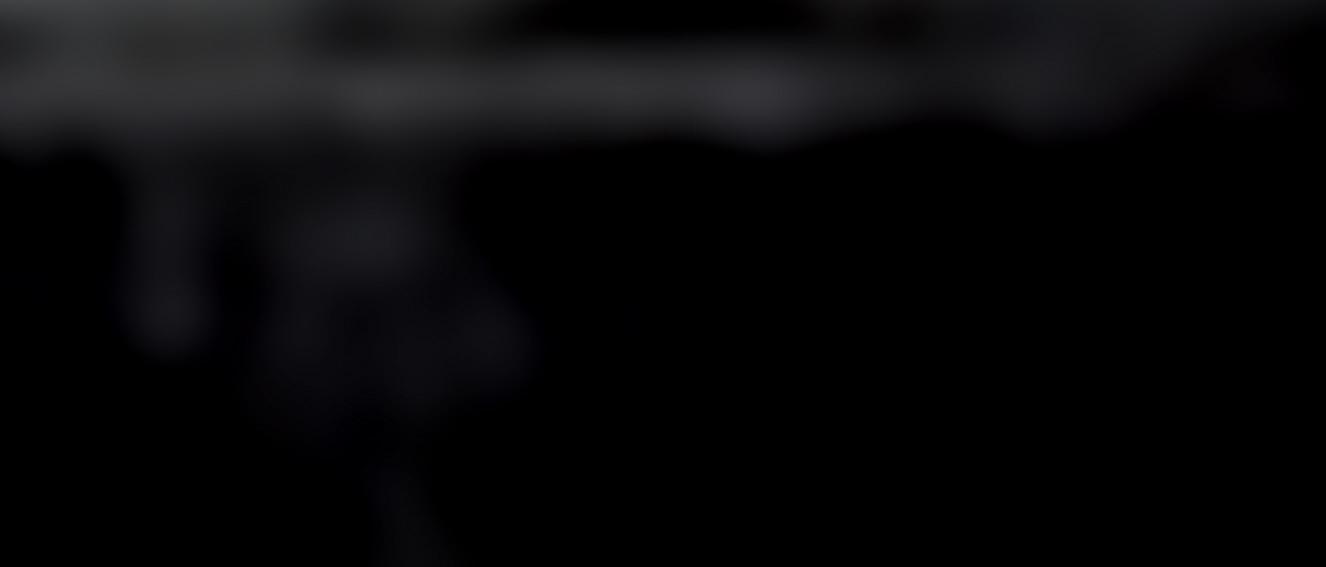
Base Plate Anchorage
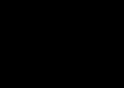
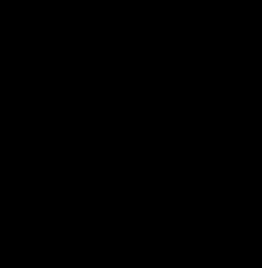
The standard tool to use when considering anchorage to concrete, allows complete geometric flexibility with the anchor patterns or base plate shape. Designs are calculated to be compliant with the latest major published design criteria and use products that have been tested by independent approval bodies for performance.
Equipment Anchorage
Further extending the functionality of base plate designs, you can now leverage this tool to model wind and seismic forces acting on equipment and resolve optimal anchorage solutions at a larger scale.
Anchorage to Deck Members
Into the floor or overhead, this functionality considers the unique designs where anchoring to concrete deck members is required. Various considerations are taken into account for the multiple cast-in or post-installed products DEWALT has to offer.
Base Plate Thickness Solver
Using finite element analysis, this tool discretizes the baseplate into elements to calculate the optimal thickness to determine when the plate is infinitely rigid. A heat map is generated to highlight the distribution of the stresses on the plate.
Post-Installed Rebar Design
Enables the design of post-installed rebar doweling using cast-in place design methodology rather than the limit states used in anchor design.
Anchor Comparisons
Comparisons of anchors can occur in several ways within DEWALT Design Assist. For a broad analysis, quickly calculate and filter the entire anchor library with a click of a button. To take a more detailed look, use the Anchor Comparison Tool to easily see differences across anchor types, sizes and brands to intelligently and effectively select the optimal product for your design.

Independently Verified
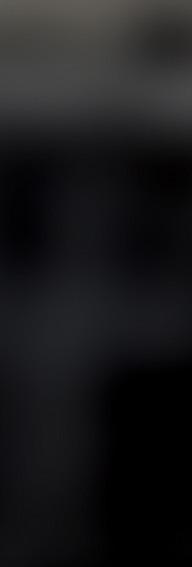
To provide the best possible design aid DEWALT has partnered with leading engineering firms for the development of the core calculation logic. In addition to extensive internal software testing, various design scenarios were independently validated to ensure accuracy and precision for the most critical designs.
Versatility
Adaptable approach in DDA allows designer to choose between pre-defined or customized load combinations. Advanced interaction of tension and shear forces feature allows designer to choose between trilinear (1.2) option and traditional (5/3) approach.
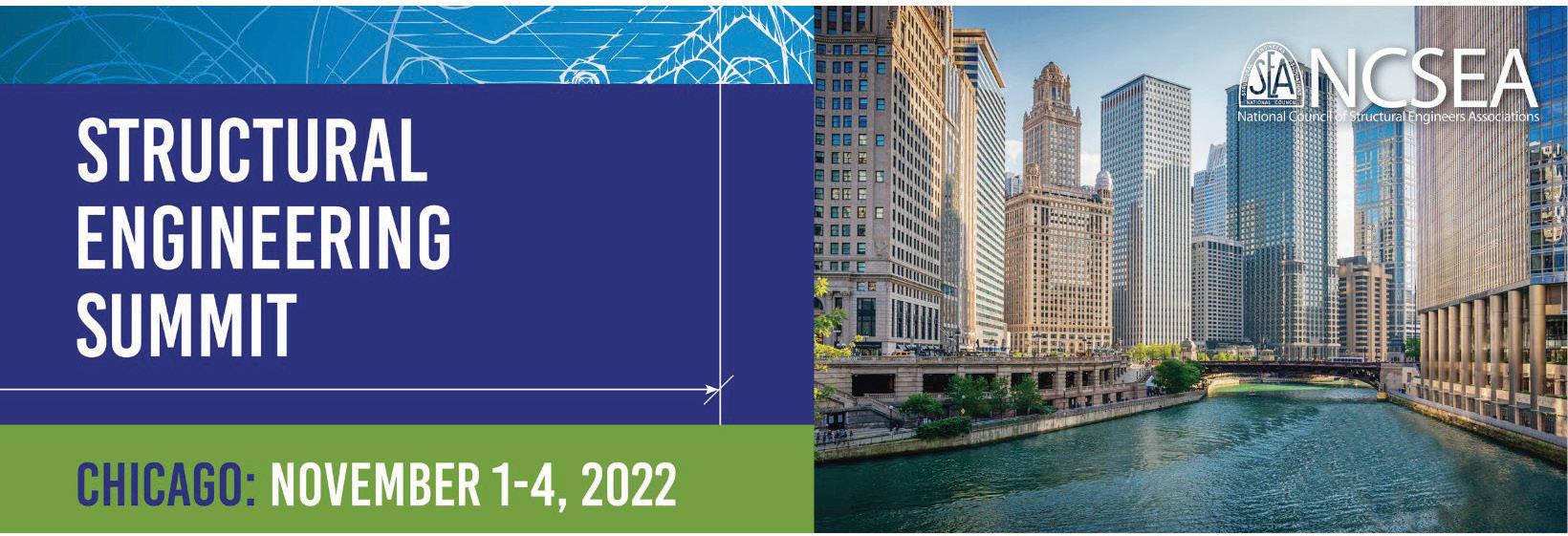
structural RESILIENCE
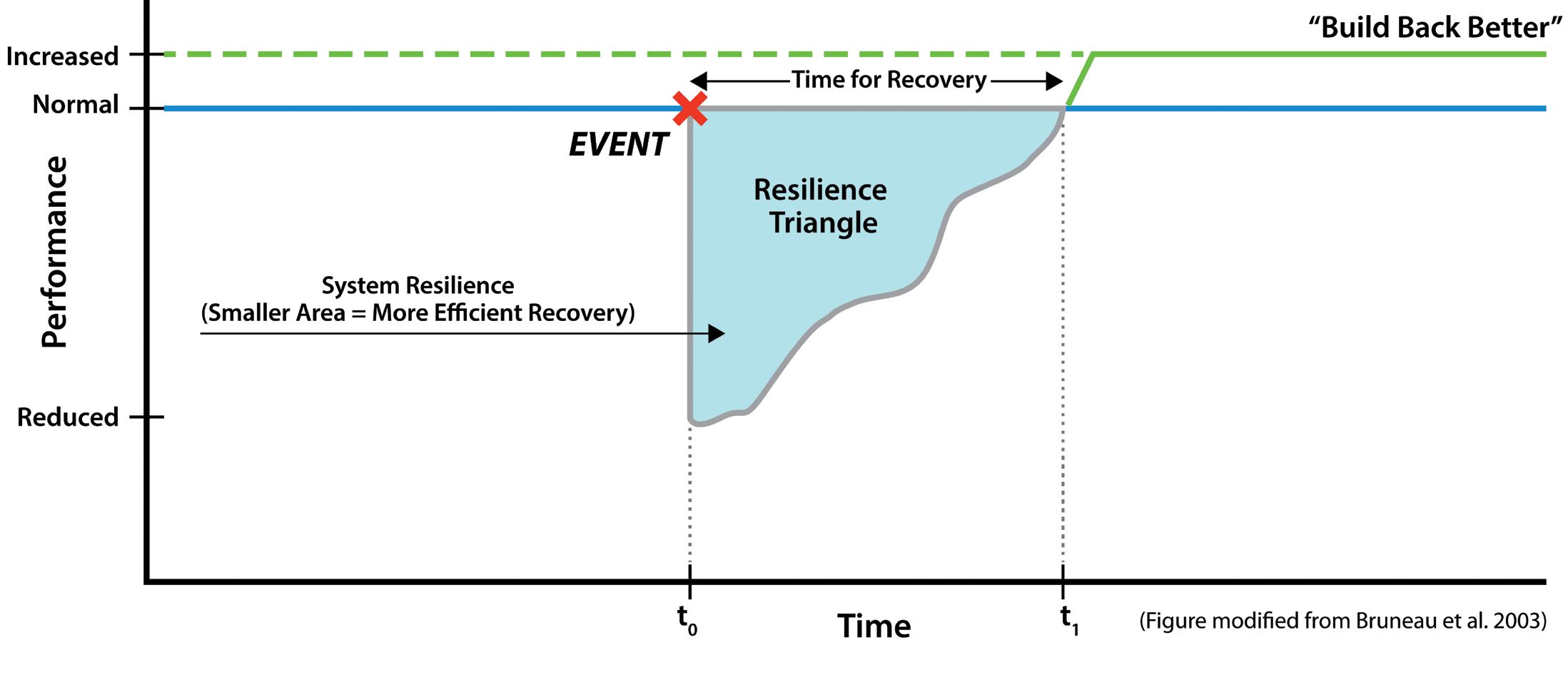
Adapt and Transform
Part 2: Performance Metrics to Measure Recovery
By NCSEA Resilience CommitteeThe NCSEA Resilience Committee is committed to educating the structural engineering community about the ever-evolving concepts of resilience and functional recovery. Adapt and Transform: COVID-19 Lessons for a More Resilient Future ran in STRUCTURE in October 2021. That article explored the concept of adaptability to the FEMA four-phase disaster management cycle characterized by “prepare, respond, recover, mitigate” (Figure 1) and considering the challenges and disruptions of the pre-vaccine COVID-19 pandemic.
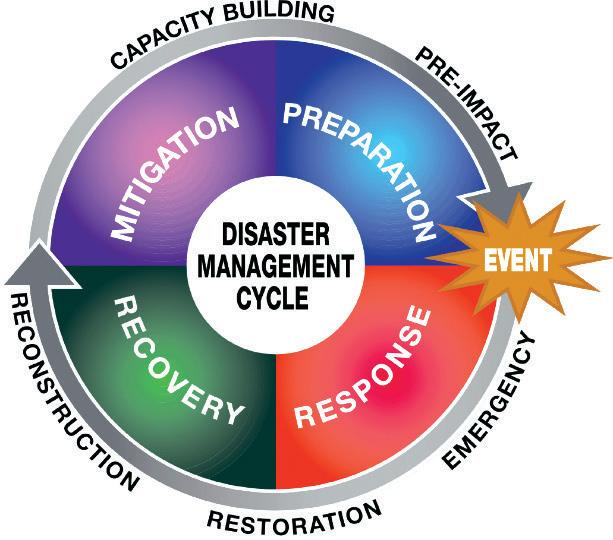
The Part 1 article presented ideas about lessons learned from the COVID 19 hazard and some applications in a resilience context, using these lessons in building design in a traditional environmental hazard environment. While the characteristics of COVID-19 are somewhat distinct from other hazards because of the lengthy impact duration and worldwide effects, one can infer that climate change might represent a similar hazard for consideration. With this realization, the Committee observed that the prolonged response and recovery period had triggered a paradigm shift to a new normal. When applying this paradigm shift to the resilience triangle (Figure 2), one can recognize that the previously steady state of normalcy was not static. Before the pandemic, resilience was targeted toward recovering toward a “Build Back Better” target, which is now a political term because of legislation. Still, it has always been referenced when speaking about resilience, meaning to rebuild infrastructure that is better than the original.
Now we question the definition of “better” and the need or desire to replace damaged infrastructure at a level that may result in better performance during the next event. The COVID19 pandemic shows us that the goal of resilience may not be shortening the time it takes to return to prior functionality post-hazard, typically called recovery. Instead, we may be better served to exploit the ability of a system that quickly evaluates real needs in a post-hazard environment to potentially identify a new functionality if warranted. This paradigm shift signals the need to consider adaptive resilience for infrastructure. Adaptive resilience is a concept that supports measuring recovery in a more holistic way, assuming that the recovery target may always be shifting. Adaptive resilience takes advantage of varying degrees of infrastructure functionality, encouraging flexibility in recovery.
This article strives to identify performance metrics that could be used to measure recovery assuming the need to recognize and incorporate adaptive resilience. For example, in the early phases of the pandemic, the New York City (NYC) Metropolitan Transportation Authority (MTA) published ridership data for public transport. Not unexpectedly, in April 2020, ridership dropped 93.3% from the 2019 weekday average. Before the pandemic, the MTA system carried 8.3 million riders on an average weekday. Over two years later, ridership has yet to return to normal, hovering around 60% of the pre-pandemic capacity (Figure 3).
If one considers commuting via public transit as a recovery metric, NYC is far from recovered. However, consider that NYC has been evolving toward a new normal that requires less commuting for the past two years. e City may be recovered beyond what might be considered 60% of a pre-pandemic normal if we consider the characteristics of a post-pandemic state. Considering long commuting times commonly associated with working in NYC, it is likely that a hybrid work-fromhome arrangement may contribute to a new normal for those who used to commute 5 days each week into the City but now commute 3 days each week, which contributes to the reported 60% of pre-pandemic levels. e FEMA disaster management cycle and the resilience triangle indicate that NYC falls short of a resilient response to the pandemic if using functionality applied to MTA ridership as a metric for the physical infrastructure dimension of recovery. However, suppose you look at how employees and companies have adapted to remote working in terms of computing power, versatility, flexibility, multi-tasking, and work-life balance. In that case, one can easily argue that NYC today is resilient and possibly more resistant to future hazards. Of course, adverse impacts of hybrid work-fromhome arrangements on local businesses add an additional layer of complexity to this quandary. However, considering ridership, one can conclude that recovery may be complete.
Similarly, the NCSEA Resilience Committee also looked at digital monetary transaction data as a potential metric for recovery. Interestingly, the

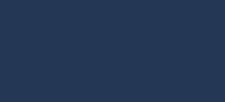
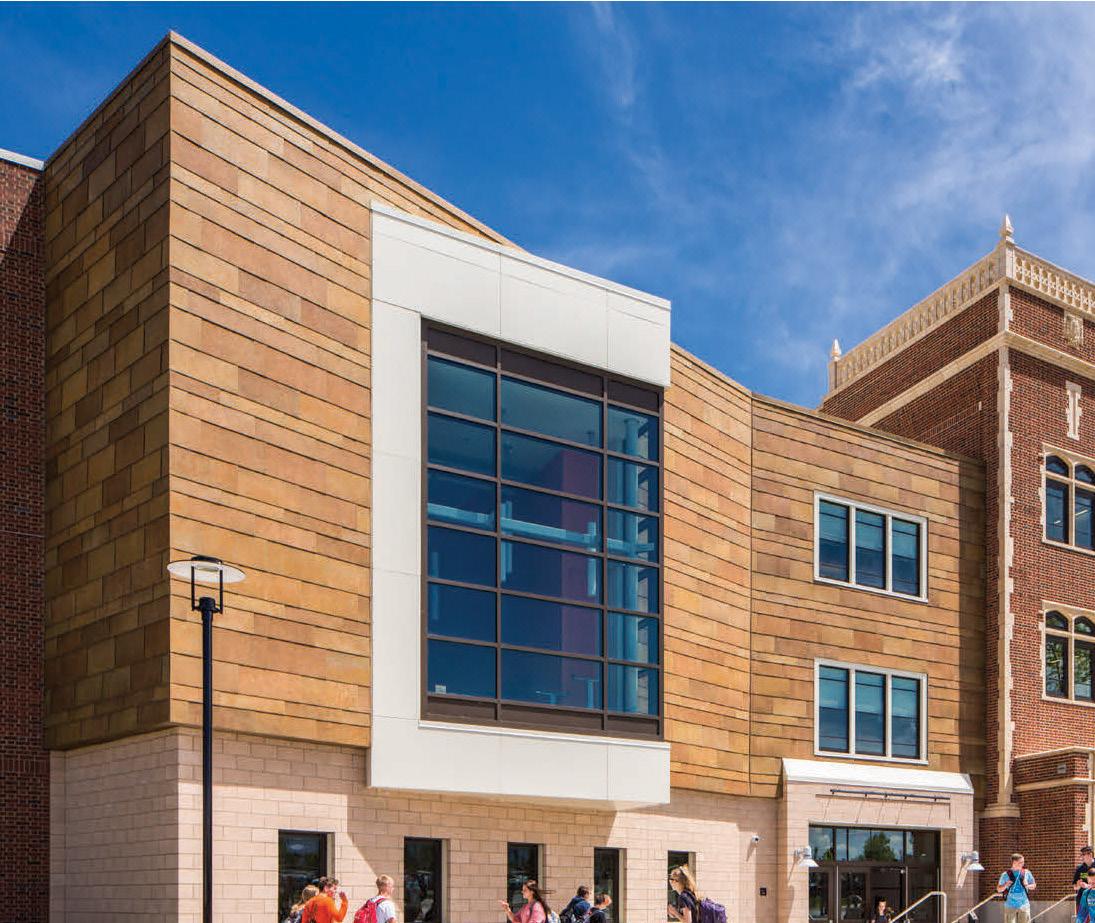
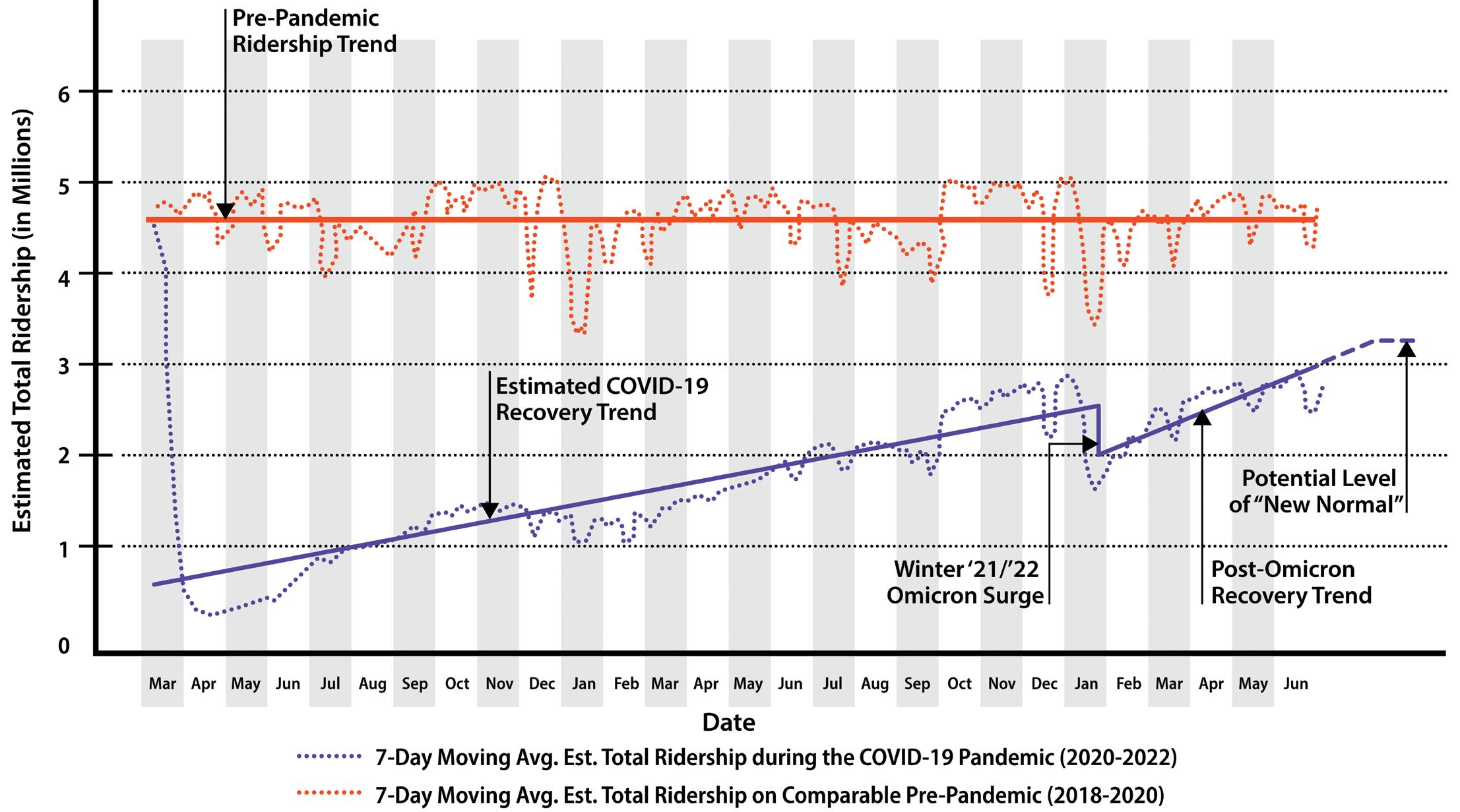
data revealed a significant increase in digital transactions as the world shifted from sales at brick-and-mortar stores to virtual ones. Overall, the search for recovery performance metrics proved very complex because individuals and communities did not seek to improve the affected infrastructure. Instead, the communities adapted and realigned with new technologies and processes that allowed for an acceptable level of functionality in a different context.
Because of this significant shift from single dimension recovery, the Committee moved away from attempting to isolate key recovery metrics and focused on how challenges are addressed (process-oriented
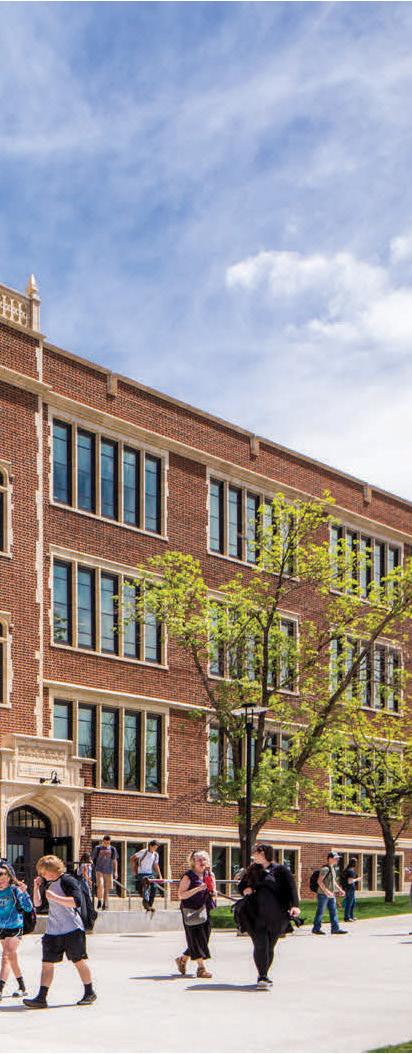
resilience). The Committee considered that true resilience might be measured by returning to some desired performance or functionality that is not directly related to pre-event steady-state conditions. This realization validated the premise discussed in Part 1 by suggesting that communities are not recovering or mitigating following the FEMA disaster management cycle phases. Instead, they adapt and transform toward a more sustainable condition by being flexible and responsive (Figure 4 ).
In the past, evolving conditions were managed through defined time horizons (design life or mean recurrence intervals) and performance goals that assume a future condition that might or might not be science-based. But this assumption ignores that humans are adaptive, and our designs should consider that fact. For example, many employees never want to return to an office full-time now that it has been shown that high productivity is still possible with remote work. Likewise, because of the human decision factor, MTA ridership may never return to pre-pandemic levels (ignoring population growth).
requirements. Architects and engineers are masters at squeezing all these requirements into the sausage maker to produce a building that will minimize first costs and maximize profits today. But what happens when the next Superstorm Sandy affects 13% of the U.S. population or the next global pandemic produces a decade-long (or longer) shift in the residential market?
Figure 4. Alternative Disaster Management Cycle post-COVID-19.
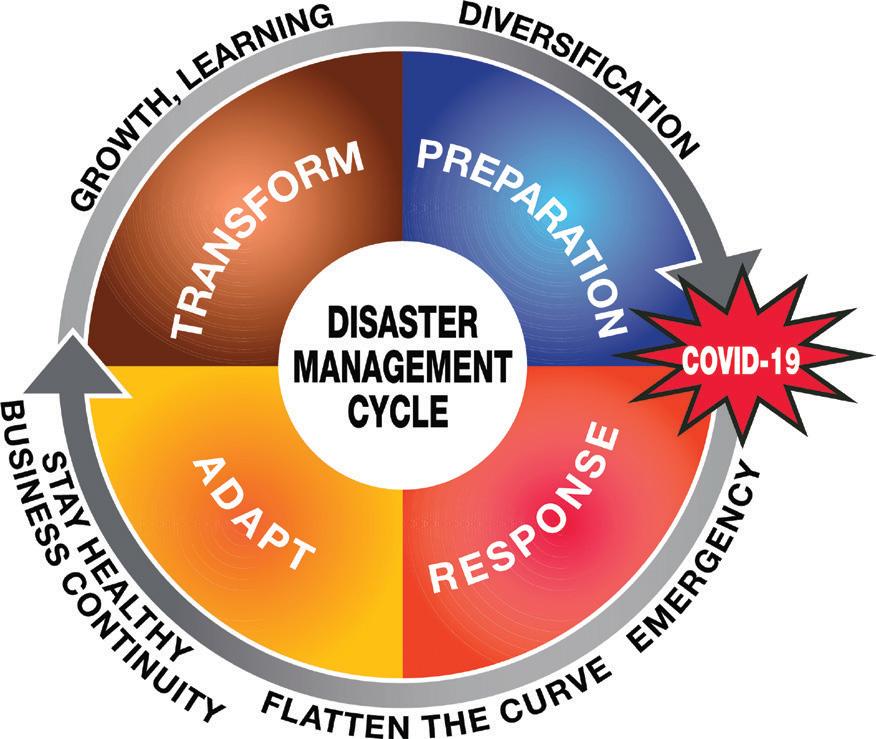
The pandemic has, and will continue to, affect the built environment, but not as much as a single catastrophic event (e.g., an earthquake). So how can these pandemic-based lessons we are learning be implemented with new projects? We know that outcome-oriented resilience calls for projects that can withstand and be quickly repaired when subjected to extreme loading to provide for the continued use of functions within the building or infrastructure. In contrast, process-oriented resilience produces projects that are planned, implemented, and managed in a way that prepares for and adapts to changing conditions, both known and unknown (e.g., due to the pandemic or climate change). COVID-19 has taught us that when we do not know the outcome (a definite target to build towards, now thought of as “build back better”), we instead need to focus on the process we use to produce the most flexible future programs and systems.
A process that enhances resilience is referred to as resilience thinking, founded in systems theory. Resilience thinking focuses on maximizing a community’s capacity to meet future challenges, realizing the cobenefits of a project in real-time, minimizing negative consequences across social, environmental, and economic dimensions, and ultimately working towards improving people’s quality of life. The process, which seeds learning and adaptation, produces results with characteristics of a resilient system–reflective, resourceful, inclusive, integrated, robust, redundant, and flexible, instead of specific performance metrics. It assumes high uncertainty and low control over future conditions. It is constantly adapting to meet and exceed the identified needs of the population based on the desire for a specific performance or functionality, emphasizing functionality and livability in preparation for an uncertain future.
A key lesson from the pandemic might be to consider revising the hyper-specialized nature of today’s buildings. Amazon and Starbucks have taught people that they can have exactly what they want when they want it, which is fine for a pair of socks or a latte but not appropriate for a building that will be useful for more than 50 years. For example, imagine a new multi-story residential building built within a flood zone (a condition that is allowed in most jurisdictions). Today the building needs to have a particular unit mix, maximize the number of units, fall within the zoning envelope, meet the target of the current flood standards (along with other hazards), and a long list of other
Traditional thinking creates redundancy outside of the hazard area and reinforces assets within the hazard area. Now imagine the same building planned and designed using principles of adaptive resilience. To consider future relative sea-level change (or elevated river levels), the second floor could be designed to become the entry-level in the future, with the ground floor converted into wet floodproofed space. This design accepts potential future water levels rather than resisting them. Additionally, each clear floor height could be stretched to 10 or 11 feet, floors could be designed with a higher live load capacity, and building systems could transport vertically using designated shafts that can be shifted as necessary via floor knockout panels. Considering these changes, a residential floor today could be converted to an office or clinical floor 15 years later.
Certainly, today there are numerous challenges to this strategy with respect to zoning laws, elevator capacity, first costs, and rental/ sale prices. But is it reasonable to focus only on the requirements of today when planning a $100M multi-decade investment? In addition, resilience thinking will stretch discussions of sustainability and carbon footprint as the first cost will undoubtedly increase, but the long-term impact can be better controlled. An adaptable building can also be designed to last 100 years instead of 50. Two $100M buildings constructed over 100 years represent a less effective investment than a $115M initial investment with $50M of reconfiguration during the same timeframe. Of course, there is a chance that the original $100M building can last for 100 years with general system maintenance – but the ever-increasing set of external threats does not support that theory. The building industry and structural engineering profession are moving towards a focus on adaption and transformation, usually because the entitlement process prevents the development of large buildings in all but a few of the most vibrant metropolitan regions. Since resilience is adaptive and hard to quantify, people should shift their focus to attributes and characteristics. Planners, owners, and design professionals should keep a keen eye on the desired performance or functionality for a built system responding to the living world within a community context, using resilience thinking to holistically widen the aperture beyond a specific project. Understanding how a given asset connects to the living and built ecosystem becomes the focus. As we face future environmental hazards, pandemics, and climate change, we envision a new normal that holds a generational quality of life above all else.■
Reference is included in the PDF version of the online article at STRUCTUREmag.org.
National Council of Structural Engineers Associations (NCSEA) Resilience Committee was founded to develop positions and recommendations on issues in the emerging field of resilience-based planning and design. The members represent SEAs throughout the U.S., working together to infuse resilience thinking into the practice of Structural Engineering (ksmoore@sgh.com).
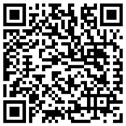
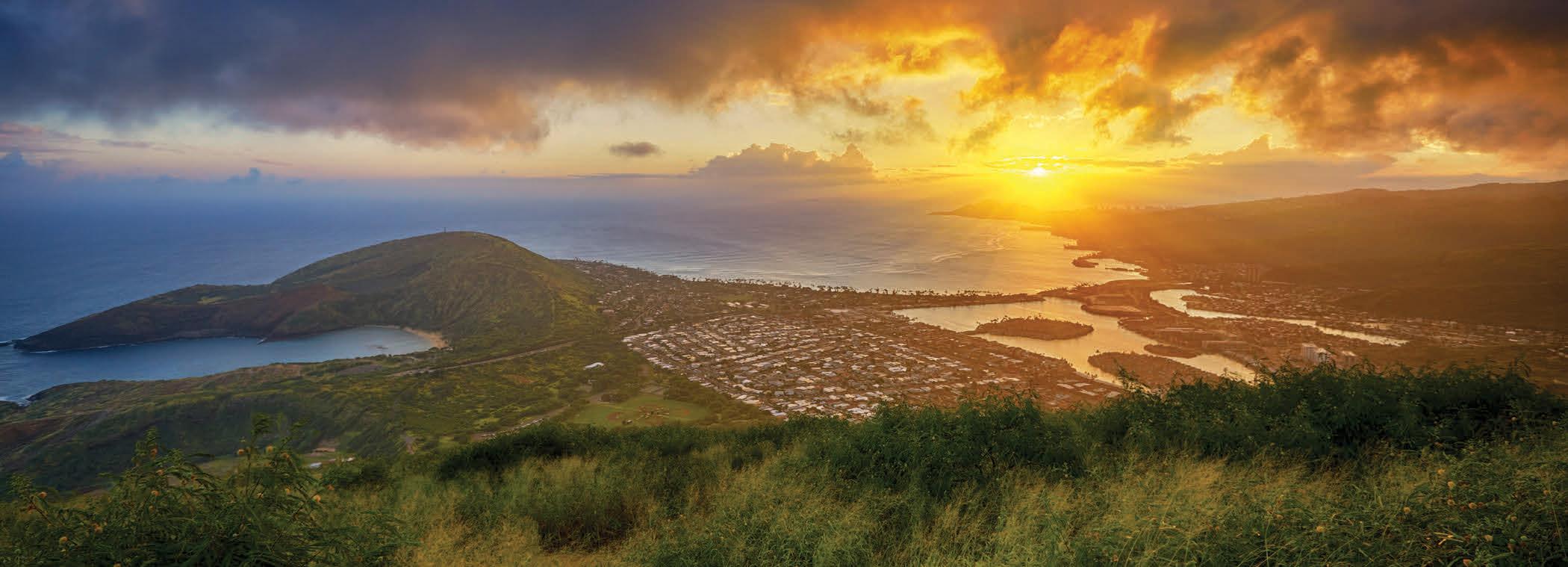
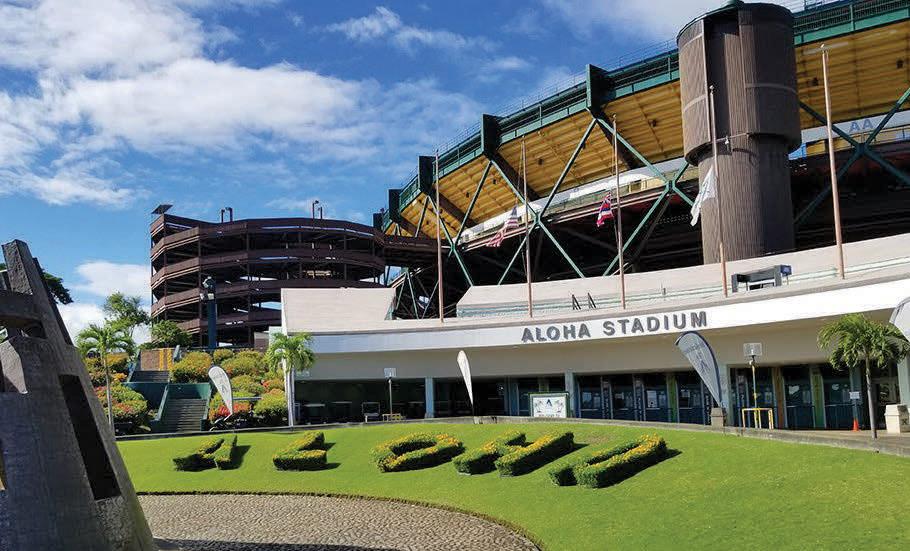
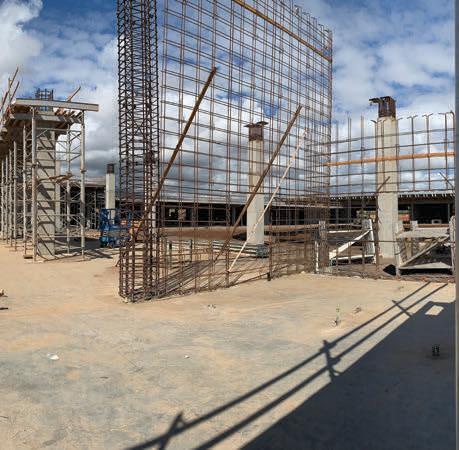
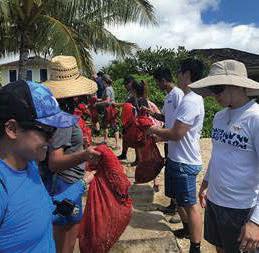
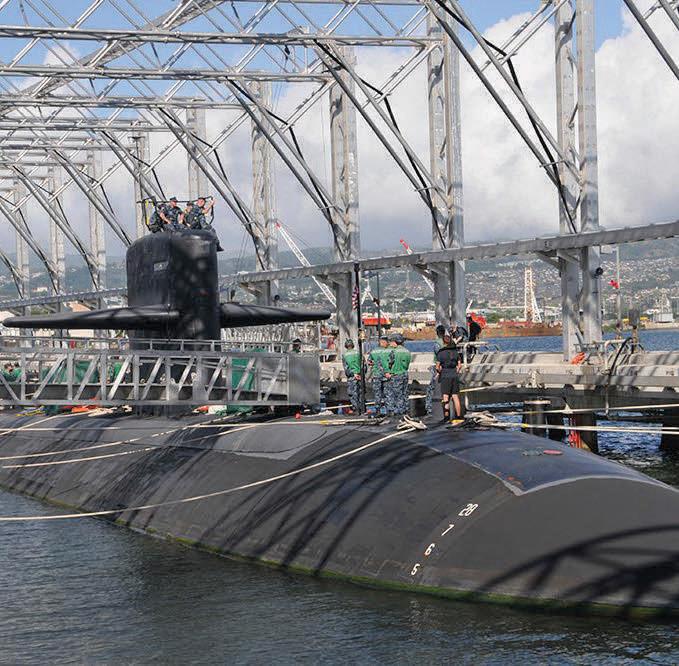
Automation and the Future of Structural Engineering – Installment 4
By Eytan Solomon, P.E., LEED AP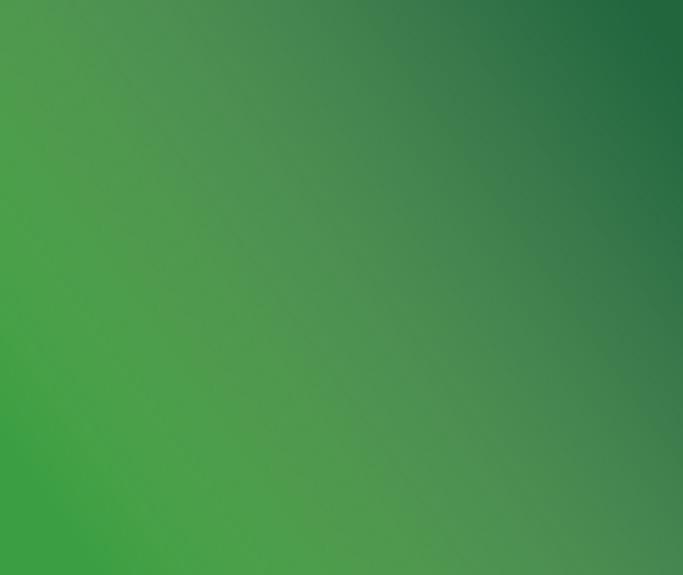
Continuing our series on automation – December 2021 (Installment 1), March 2022 (2), June 2022 (3) – I sat down (virtually) in April 2022 with two more industry experts in digital design: Steve Reichwein, a Senior Associate at Severud Associates Consulting Engineers, and Carlos de Oliveira, a founder of Cast Connex. Below are highlights from our discussion.
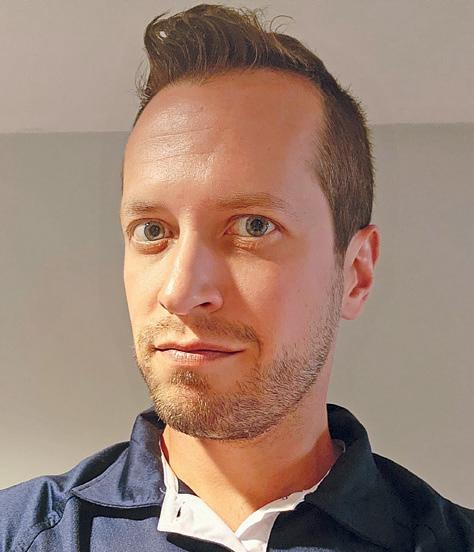
You both gave an excellent presentation to SEAoNY back in January about the Madison Square Garden Sphere at the Venetian in Las Vegas. As an introduction for the STRUCTURE magazine audience, could you provide an overview of that project and how parametric design and other digital tools were used in the design and fabrication?
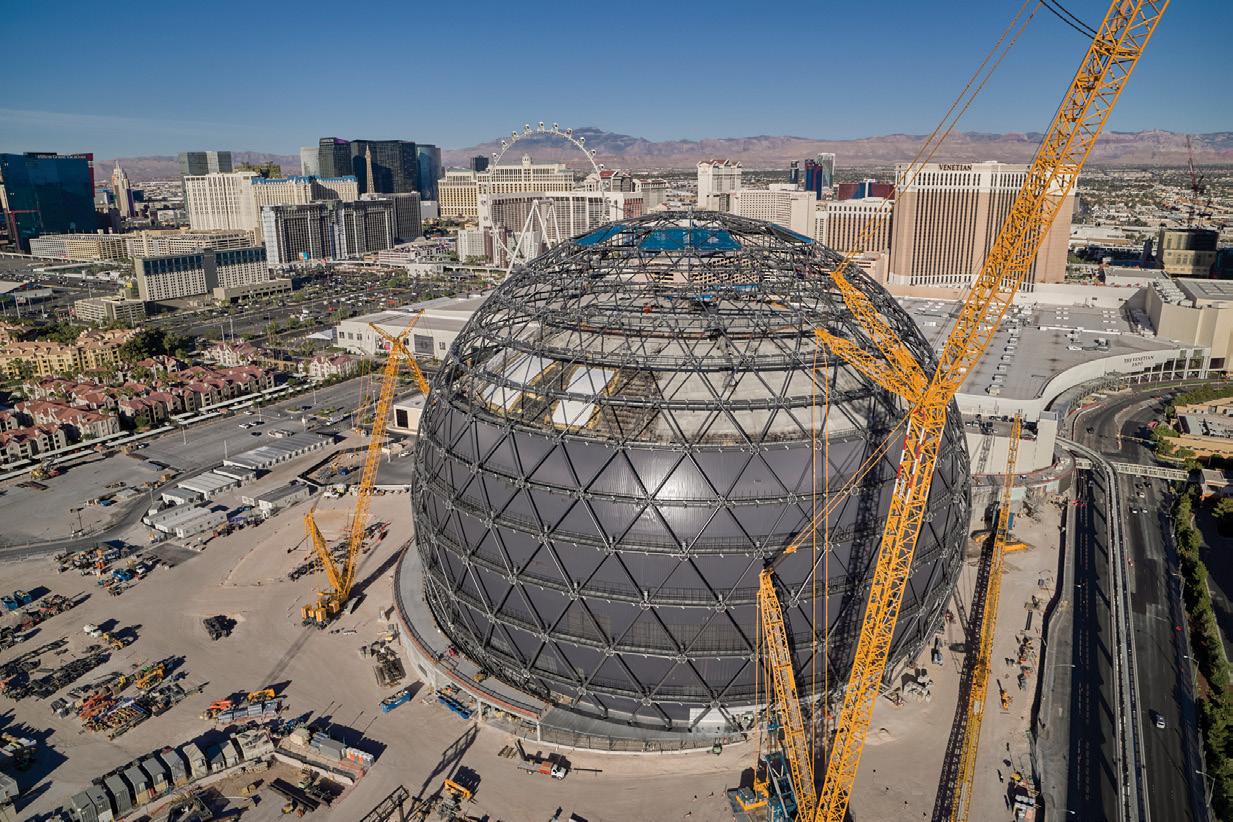
Steve Reichwein: The project is an 18,000- to 20,000-person capacity entertainment venue that will mainly be used for concerts, E-sports, and immersive events. The media plane is the project's focus: that’s the inside LED screen comprised of over 45,000 LED tiles or over 200,000 square feet of surface area. The media plane is a rather interesting structure that is not ground-supported, except at the back, where there’s a large portal opening for the proscenium. The rest of it is hung from a 440-foot-diameter dome roof that is essentially the roof of the venue. About 12 feet from the venue structure is the sphere that everybody will see from the outside: the 520-foot-diameter geodesic sphere. It’s not a complete sphere; it is cut off roughly 100 feet below the equator at ground, but it goes up roughly 375 feet above ground through the apex. That portion will also be wrapped in an LED video system. The trellis system has about 25 miles of curved steel pipe. While the main structure is not curved, it is segmented at each node. And then there are almost 760 miles of LED strands. So
it’s a crazy amount of electronics and structure tied to that external geosphere primary structure.
Carlos de Oliveira: Cast Connex engineered and supplied cast steel nodes for both the exoskeletal geodesic structure on the outside of the venue and the structural backup for the “immersive surface media plane” on the inside. On the outside, you have a spherical structure; on the inside, it is actually a slightly elongated spheroid. But both structures use cast steel nodes at the intersections of the primary structural members. The geosphere is rationalized with latitudes with diagonals that intersect, creating a diagrid with six members framing into each node. Near the top, where Severud undertook additional rationalization to reduce piece count, we have some nodes that accommodate up to 8 members framing into them.
Our first step in designing the casting is to understand the geometric repetition that the structure offers. On the outside, given the nature of how the structure was rationalized, all of the nodes around each of the latitudes are geometrically identical. Once we establish the kind of structural rhythm of that geometry, we can use parametric design in our process to help us build the three-dimensional models of each one of the nodes. And then, with force information provided by the Structural Engineer of Record, we can optimize the castings. So we start with a solid shape of material. Then we look at where the stresses are in that material and start pulling away material where it’s not necessary, and then we optimize the nodes for just the structural loading that is applied to them. We, of course, have to consider how we manufacture those components – how the liquid metal will freeze inside the molds that will produce this component – and so, frequently, we have to add a little more material back to make the component more castable. We try to shape the part to promote what we
call directional solidification. The liquid metal will start to freeze at the extremities. That solidification front will propagate towards the heavier sections of the part, and that’s where we will connect liquid metal reservoirs to the casting. Thus our design process is driven first by structural geometry and loading and then by manufacturing constraints.
How much of that decision-making to add and remove material can you teach the computer to do, versus how much must be the engineer steering things hands-on?
Carlos de Oliveira: Well, we’re not there yet in our process. We are not nearly as automated as Steve can get with his parametric design. But the first step might be topology optimization, where we start with a design space and apply the loading to it. We can see areas that are not highly stressed and remove unnecessary material. That process can be relatively automated. However, the output of that process may not result in a particularly useful shape from a practical perspective. For example, material may be missing to keep water or debris from collecting inside the connections. So you end up building back material from that perspective. And then, of course, we must consider manufacturability.
I see many parallels with the design side. Like how you can teach the computer to automate to a certain degree, but then there are the common-sense human things you still have to do yourself. Do you envision the process getting more automated sometime in the future?
Carlos de Oliveira: I think that there is an opportunity for it. All the steps in our design process are types of analyses, and we currently use different software packages to carry out each step. For example, the solidification analysis is happening in software called Magmasoft. And our stress analysis is occurring in a piece of software called Hyperworks. And our 3-D modeling and shaping of the part are happening in SolidWorks. Unfortunately, we’re not yet tying them together nearly as well as possible, and iteration is somewhat manual. If we’re going to get closer to automation in our processes, we have to be able to tie those pieces of software together in a much better fashion, more like how Steve can do with all of the pieces of software that he uses.
Steve Reichwein: Let’s start with the geosphere because that’s more or less the easy one. Basically, we have a sphere with certain loads applied to it – gravity, wind, seismic, and so forth. So when all the superimposed loads are your constants as well as the size, shape, and support conditions, your variables are endless. We started by using algorithmic tools and Grasshopper components, such as Ladybug, Lunchbox, or Kangaroo, to mesh the sphere into equilateral triangles closer to Bucky’s (Sir Buckminster Fuller’s) traditional sphere. Because it is clearly the most optimized in terms of weight, we started there as a base point. But then, after getting Carlos and W&W [the steel subcontractor] involved early in the process, we started to clearly see that there were going to be issues with that type of sphere. First, given the geometric complexities and lack of continuous rings needed to stabilize the structure in phases, it would need a significant amount of shoring. Second, there was repetition in node connectivity and member length. However, you would have a node down near the base that matched a node all the way at the top, but the loading was totally different, so there was no repetition in structural demand. We started to see those pitfalls very early in the process, and we rethought our design of the geosphere completely. We changed the sphere from a traditional icosahedron-based “Bucky” sphere to a “polar geosphere”; it’s essentially a series of continuous, flat latitudes forming complete, elevated rings bisected by 64 diagonals forming a triangularized grid shell from top to bottom. We also focused on congestion elimination for the apex, which was a huge savings. The final scheme weighed more
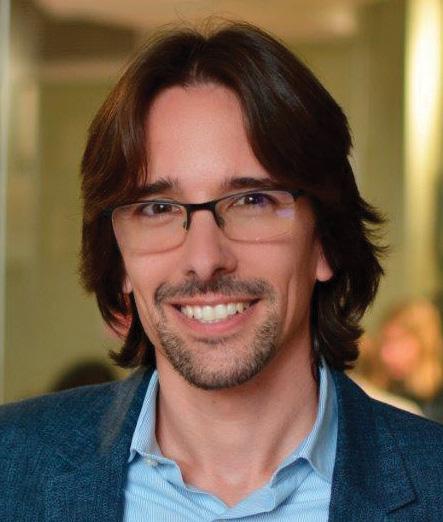
than the base scheme, but we used input from W&W to compare the structural schemes' actual costs; the polar geosphere turned out to be approximately 25% less cost when all factors were considered. And then, a similar exercise was done for the Dome roof. If we had used the rules of thumb from old steel journals, we would have had 1,000 extra tons of steel in the Dome roof.
Overall, the computational optimizations, simulations, and goalfinding saved the project tremendous material weight and money. We plugged our algorithm into AWS supercomputing and other cloud computing to speed up simulations and decrease the computing time. They were like 2-hour runs that would eventually converge, but it would run a million different schemes. Given that amount of data, we would hone in on the global minima in terms of weight and cost. However, the human element of experience and engineering judgment is critical in this stage. You must have the experience to weed out some of the outrageous simulations. Seeing the results in stages may also warrant some tweaking to the AI, which can help automatically weed out “bad” results for future runs.
Would you say steel is the structural material that lends itself best to design automation?
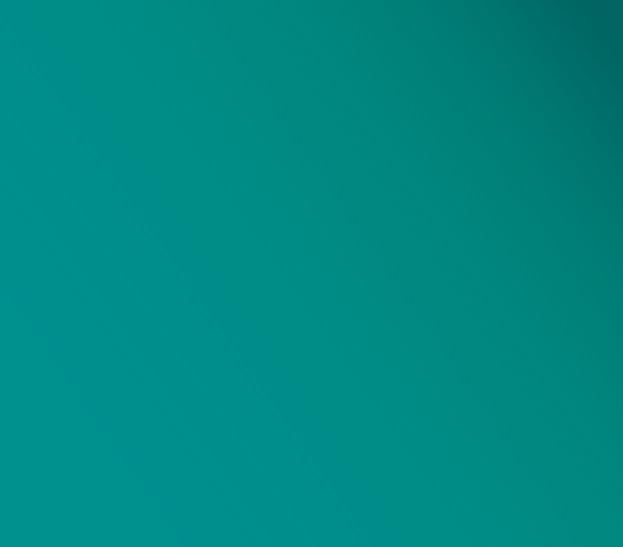

Steve Reichwein: No, I don’t necessarily agree with that. Both steel and concrete can be optimized individually for material weight and compared against each other using cost. I actually have an algorithm I use for tower studies early on that optimizes and compares steel structures versus concrete structures; cost is the ultimate equalizer for both. We use a structural analysis plug-in called Karamba, made by an Austrian engineer (Clemens Preisinger in conjunction with Bollinger–Grohmann). We’ve actually piloted and gone back and forth with the developer directly to give input and develop the program further. It has an excellent optimization tool for concrete where you can essentially do a shear wall core, run it in the optimization, which uses virtual work, and gives you optimized thicknesses of each piece of mesh for the entire tower in less than a minute. It’s super quick. As far as laying out the core, it won’t help you with that, but you can build AI programming into the layout or start with the traditional topographical analysis and figure out roughly how big your cores should be. Additionally, the architect may have some constraints on the core layout that gets built into the algorithm.
It takes longer to do the concrete optimization because you’re using finite element shells, where there are so many more elements as opposed to steel structure, which is comprised of stick elements. But there are cloud computing opportunities, as well as cloud-based
Really modern steel fabrication shops just don’t look anything like fabrication shops of old.
Carlos de Oliveira
plug-ins. So you can plug them directly in Grasshopper, and it will run certain nodes with cloud-based supercomputing.
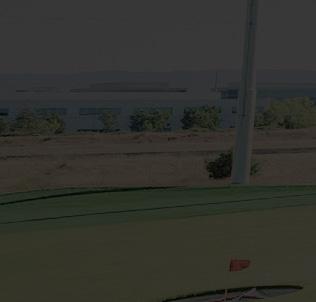
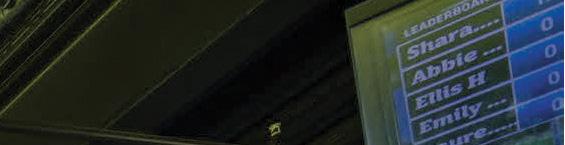
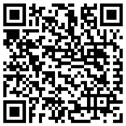
Have you let architects play around with those tools, in between calls to the structural engineer?

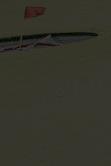
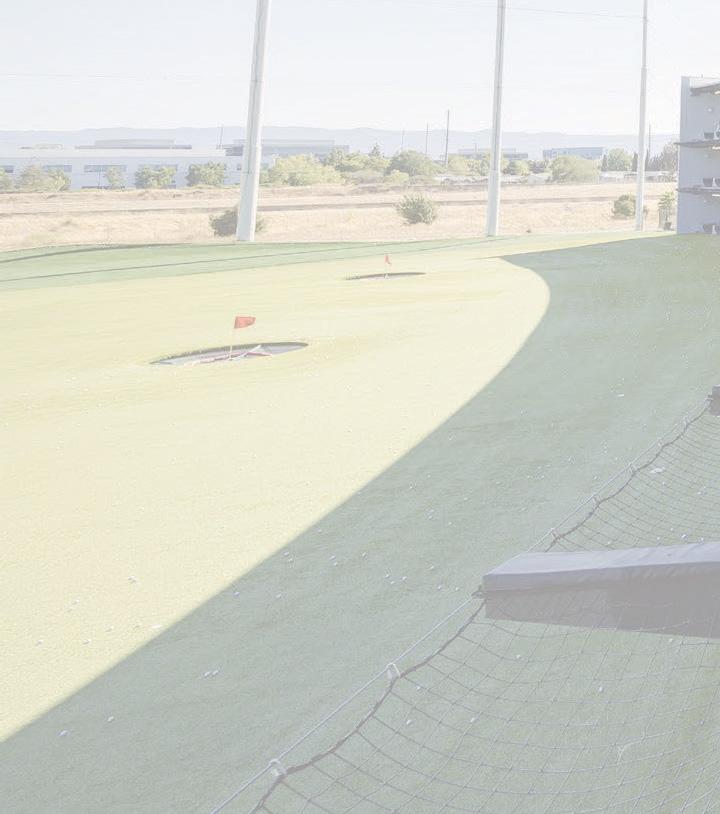
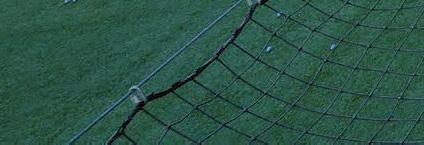
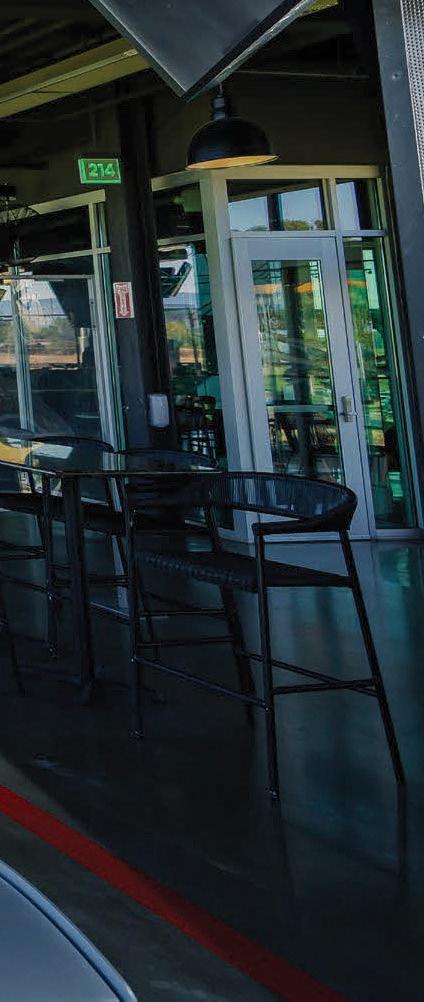
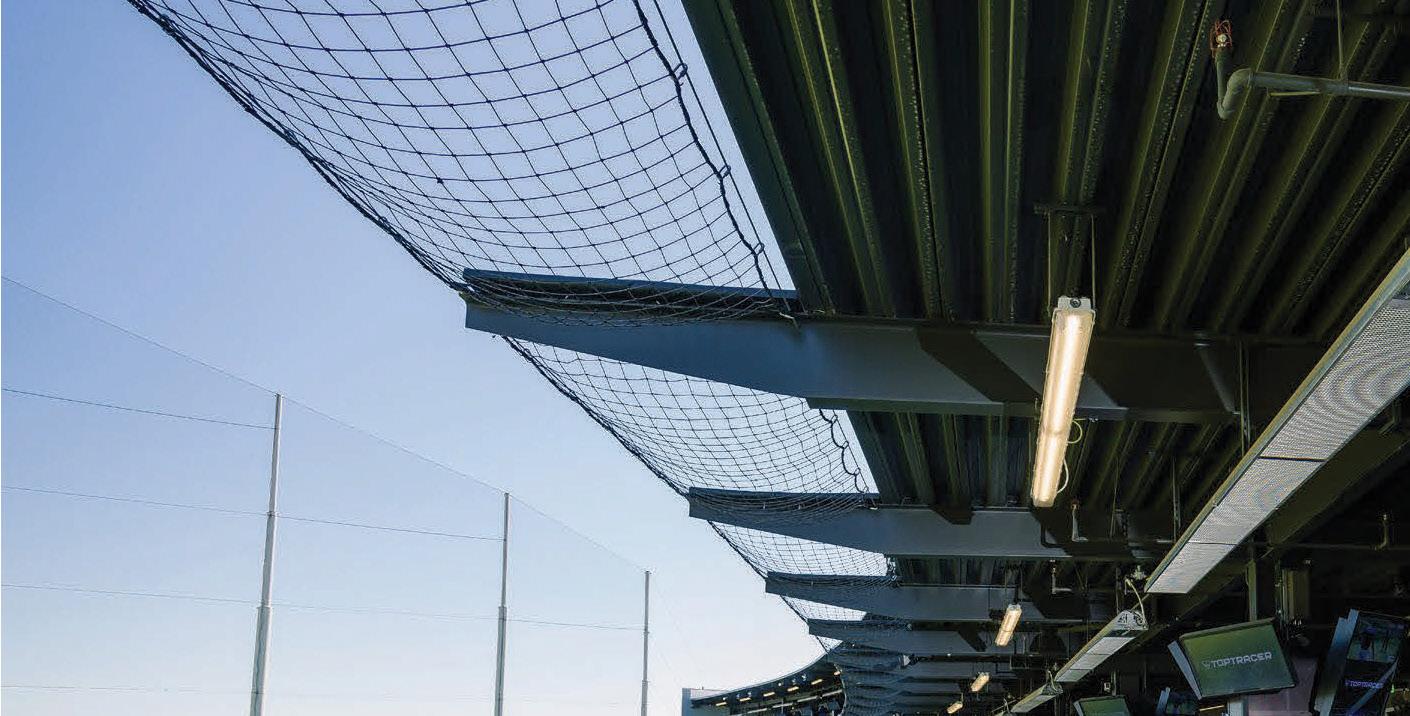
Steve Reichwein: Yes. We worked with Shapedriver a little to make some tools that architects could just plug in height. It was a simple box that didn’t get very complicated, but it was basically our tower optimization algorithm dumped into a Cloud-based computing service; then, you can share that with anybody. It’s all cloud-based, so the client will just need a link to access it, and then they plug in the variables and run the program in literally seconds. It’s very quick.
Carlos de Oliveira: It’s interesting when you think about how we do topology optimization as we start with a design space to which we apply loading. en we look at the lowest stressed material, which gets eaten away from our models. In contrast, you can compare that with the way that you might approach a steel-framed structure like the MSG Sphere’s geosphere, for example, where you start with a preconceived structural approach and then use the algorithms to optimize several specific parameters simultaneously – like the number of latitudes and the number of nodes at each latitude. In this approach, you go directly to more buildable solutions, whereas topology optimization requires more post-processing to develop a useful solution.
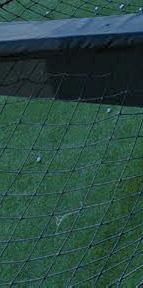
It seems like some parts of the construction side are already quietly implementing automation tools. Can you give any examples?

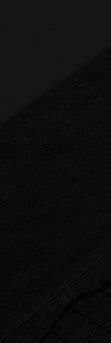
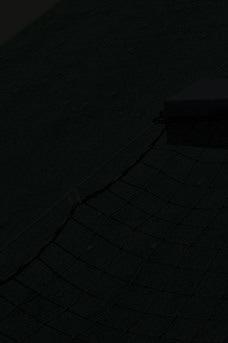
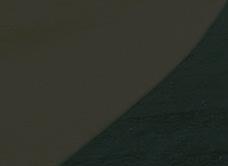
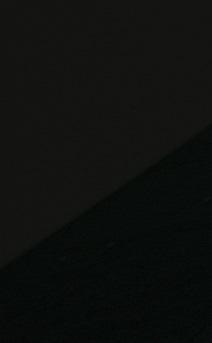
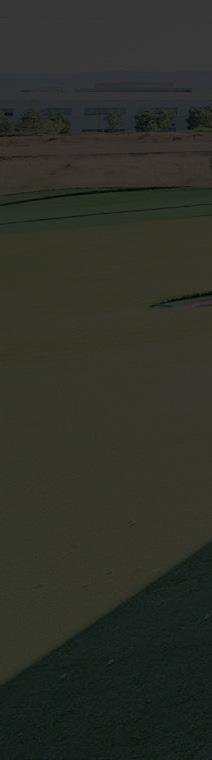
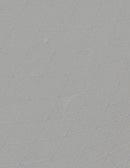
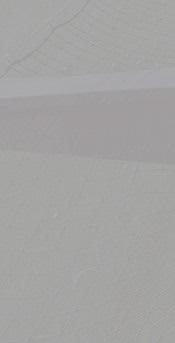

Steve Reichwein: Connections, in general, are getting fairly automated. Q-nect will run an algorithm on your entire structural steel
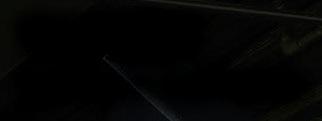
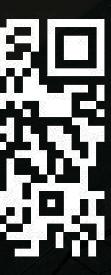
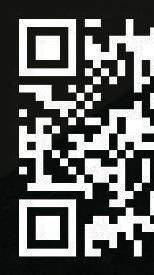
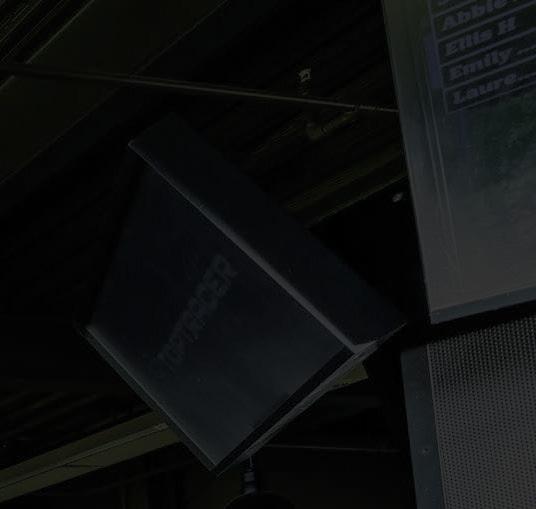

building if you give them loads and geometry. ey basically just need a Revit model with parameterized beam end reactions, and it will design all the connections and detail them, all in about an hour. Ridiculously fast. at’s built on tools commonly available now through Grasshopper and Dynamo, where you could do something similar yourself or bring in Q-nect as part of the team.
Carlos de Oliveira: Not recently, but it reminds me of when shops moved towards plasma-cut plate CN files to try and make optimal use of plate material to cut as many pieces out of one big piece as you can, so you nest them. Now there are even automated weld fitting machines. Really modern steel fabrication shops just don’t look anything like fabrication shops of old.
Do you think robots will take all our jobs someday?
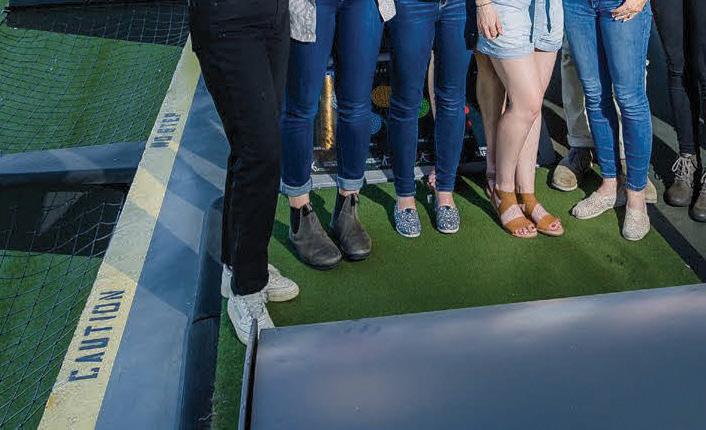

Carlos de Oliveira: e best structural engineers and the best architects are the creative ones. e most striking architecture, the most interesting buildings, speak to us on a human level. I don’t think computer code can create the places, buildings, communities, and cities we want to live in. So, although parts of our profession have been automated, and more and more will be automated, I think an essential aspect of our profession can never be automated: the creativity and the human aspect of what we do.■
Many thanks to Steve and Carlos for sharing information about the project and their insights on these trends in our industry.

Eytan Solomon is a Senior Associate at Silman and a member of STRUCTURE’s Editorial Board (solomon@silman.com).

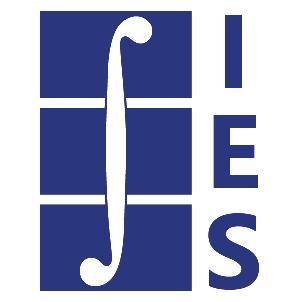
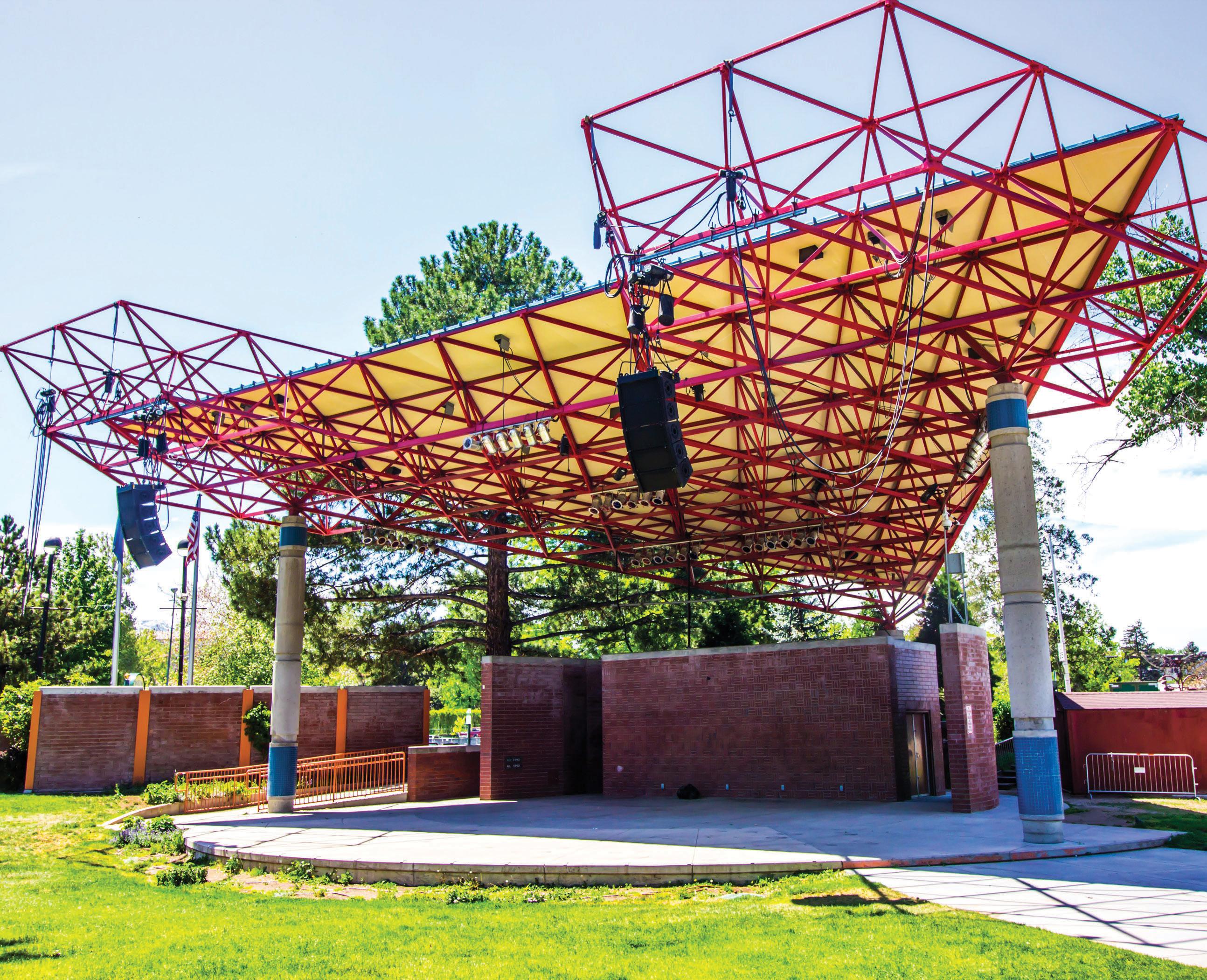
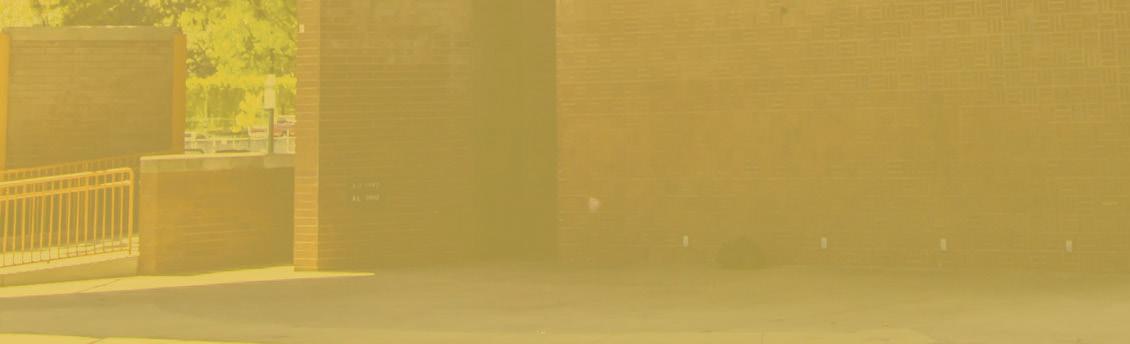
basic EDUCATION
NCSEA 2021 Practitioner Survey
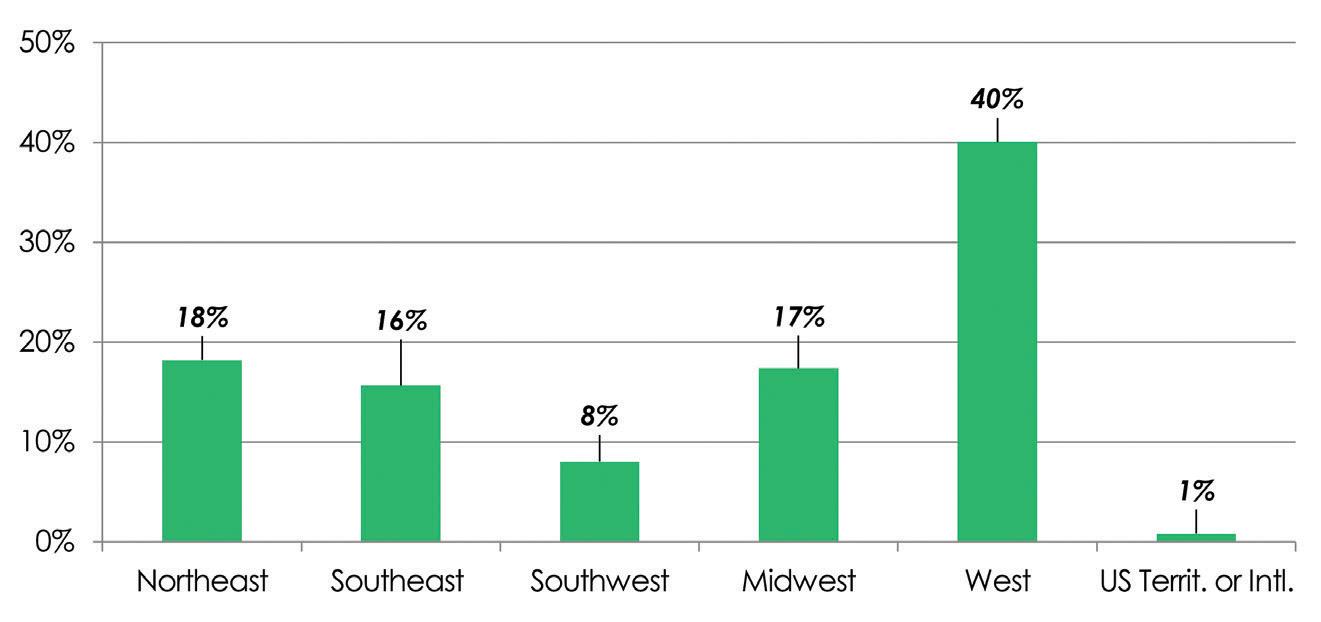
What are Expectations for New Hires Entering the Workforce?
By Michelle Kam-Biron, P.E., S.E., F.SEAOC, Brent Perkins, P.E., S.E., and Scott Francis, P.E., LEED APStructural engineering firms have a vested interest in selecting new hires with the skills to flourish in an office environment and an education that prepares them to solve the myriad of technical challenges that structural engineers encounter daily. Additionally, students who obtain an accredited degree from a civil/structural/architectural engineering program are interested in obtaining a job that applies their education and helps them advance their careers.
A Curriculum Survey was developed as a resource for structural engineering firms and students to better understand the education provided by universities. This survey provided the supply side of the equation but lacked the demand side. For example, what type of education do structural engineering firms desire and require of their new hires? A Practitioner Survey provides a resource to describe the skills and educational requirements that structural engineering firms value in new hires. Additionally, the focus of the Practitioner Survey was on the education of the structural engineering student and how it relates to real-world applications, industry demand, and technical preparedness.
Figure 2. Practitioner response experience distribution.
The Basic Education Committee (BEC) published two surveys in 2016 related to the NCSEA recommended curriculum; the Curriculum
Survey, which canvassed colleges and universities to gauge course offerings, and the Practitioner Survey, targeting design professionals to better understand the skills necessary to enter the structural engineering profession. In 2019 and 2021, the BEC launched new Curriculum and Practitioner surveys to better understand the issues and look for trends. Like previous surveys, they were administered via email and web links publicized by NCSEA. The 2019 curriculum survey article can be found on the BEC webpage https://bit.ly/3BCg1Ws, and the 2021 Practitioner Survey is summarized in this article.
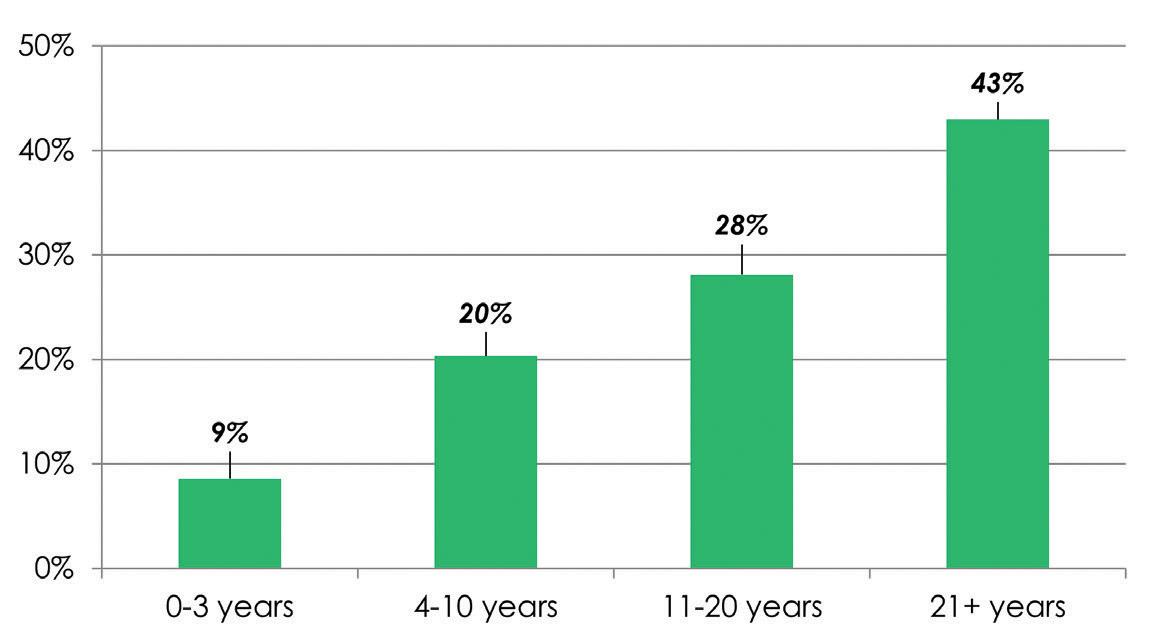
Survey Overview
The 2016 and 2019 Curriculum Surveys focused on finding more information about the frequency and availability of the twelve (12) structural engineering courses recommended by NCSEA as core courses for structural engineering students.
Figure 3. Practitioner response to geographic location.
Figure 1(online) shows the extent of the coursework and skills surveyed and the perceived importance indicated by the respondents.
Survey Results
The 2021 Practitioner Survey had a 24% increase in responses over the previous survey. Over 500 practitioner responses were received. The professional respondents’ experience distribution ranged from 0-3 years (8.6%) to over 21 years (43%). A full breakdown of this distribution can be seen in Figure 2
The geographic distribution of responses throughout the United States was balanced amongst the practitioners between the Northeast, Southeast, Southwest, Midwest, and Western U.S. (Figure 3). Some respondents indicated their firm has multiple locations or their work is in multiple regions, which was considered when evaluating the data. The BEC presents this data to demonstrate that survey results reflect opinions from across the country. Although there were twice as many responses from the West, regional differences were insignificant except for a few topics discussed later in this article.
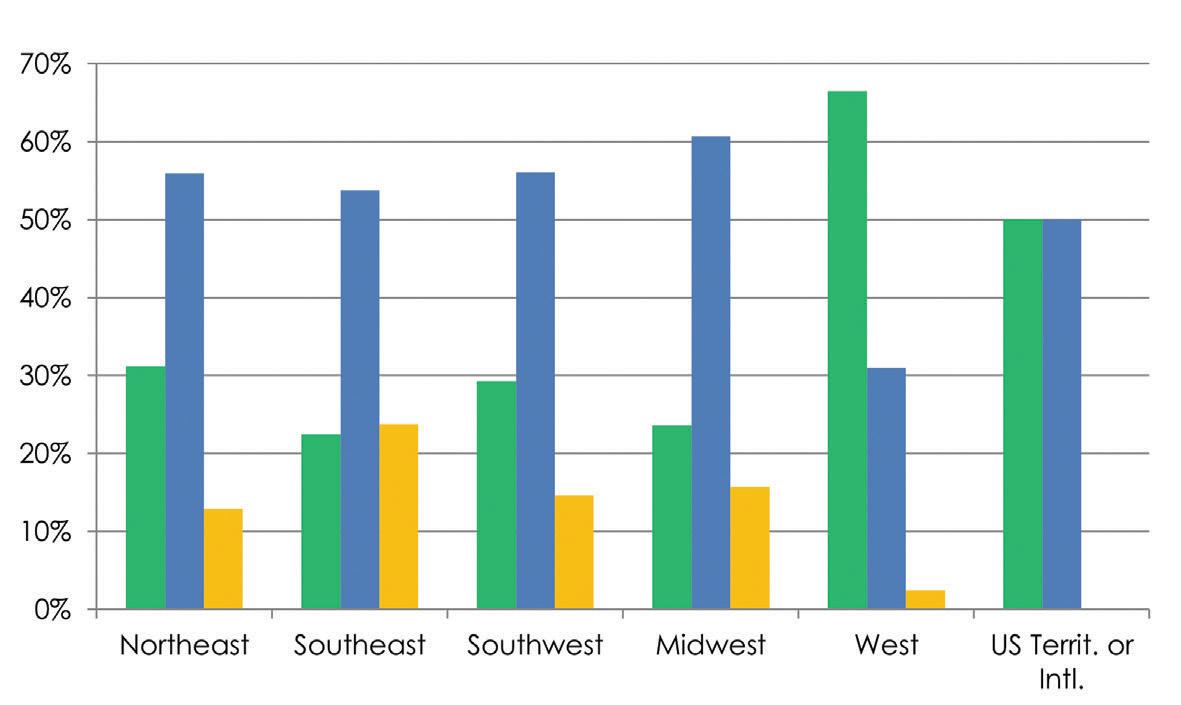
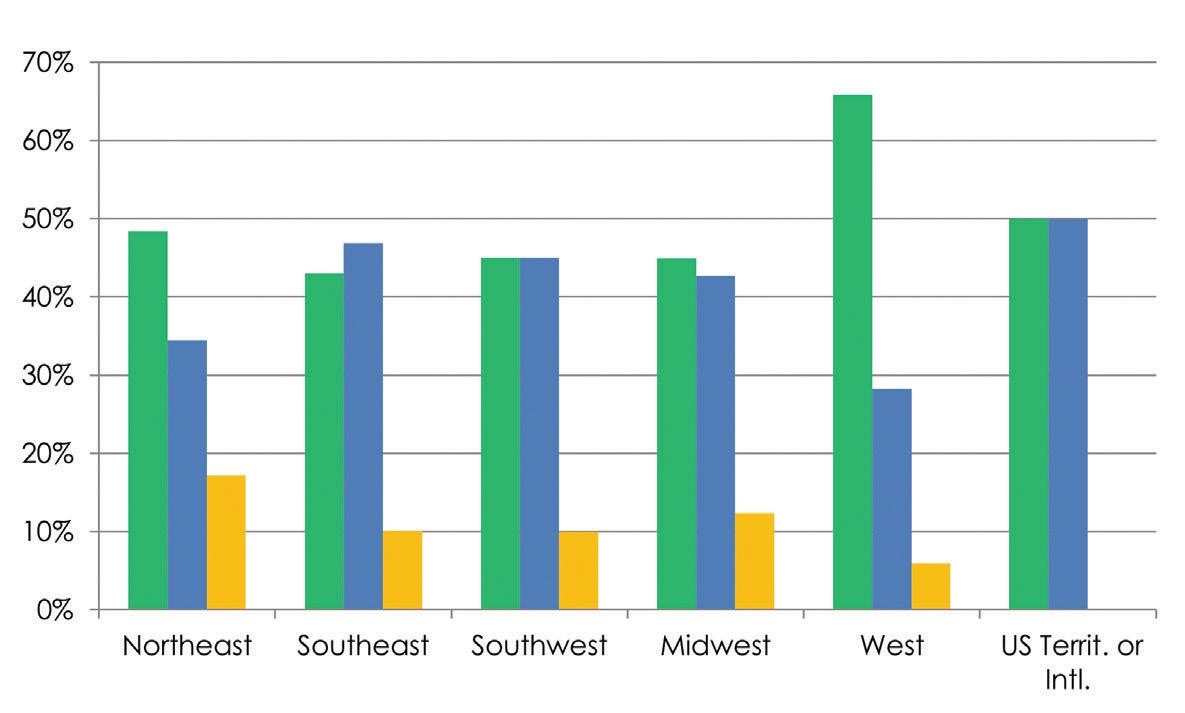
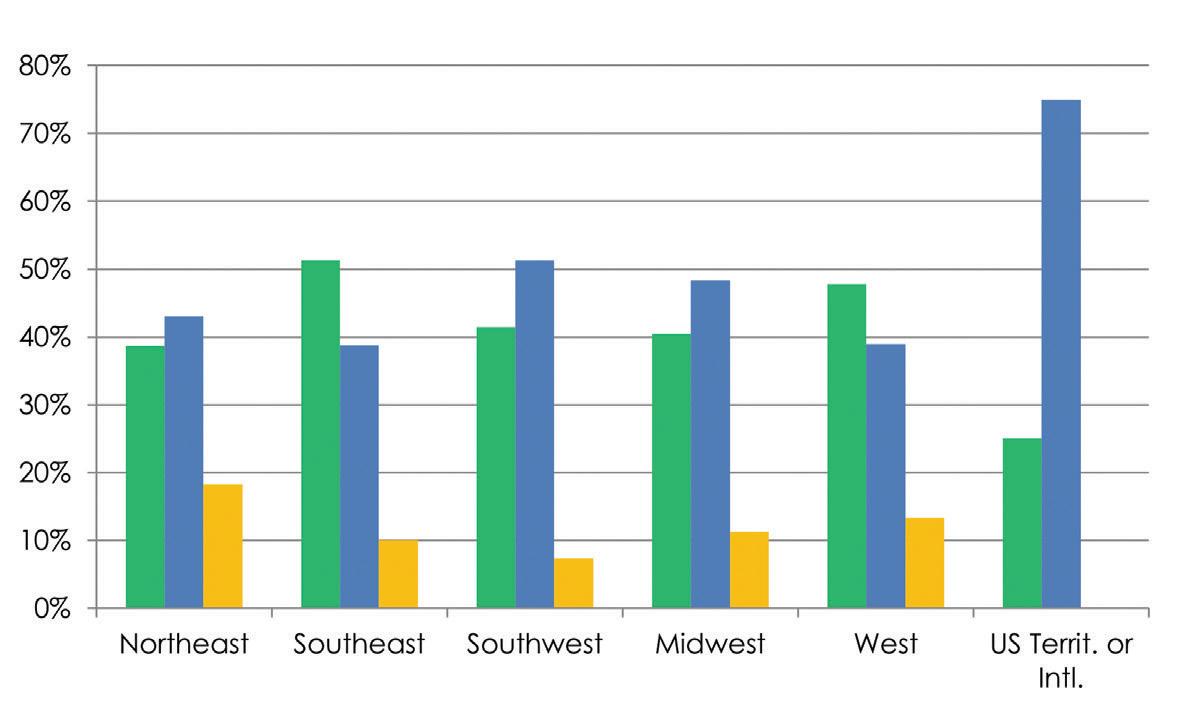
Except for Structural Analysis III (85%), Prestressed Concrete Design (70%), and Masonry Design (87%), more than 90% of the practitioners responding indicated the recommended curriculum topics should be included in or are very important to structural education.
As with the 2016 Practitioner survey, the 2021 survey’s highest-ranked non-core class or technical skill was Load Paths/Load Flow. Second to that was Structural Stability Results of the Curriculum survey indicated that Load Paths/ Load Flow are generally integrated into a group of design courses instead of being standalone courses. Notably, a few institutions have specific courses dedicated to loading, load paths for members/elements, building systems, and connection details. The importance of this topic and how the BEC will address this in the recommended course curriculum are noted below. The least ranked were Introduction to Architecture or Architecture History, Blast/Progressive Collapse, and Sustainable Design
As mentioned above, the survey attempted to attract a distribution of respondents from across the structural engineering profession: practice work type, firm size, geographical region, and years of experience. The goal was to identify if these variables played a role in ranking the importance of topics for education in structures. The results showed that these variables were not a differentiator for “most important topics” such as Structural Analysis, Steel Design, Concrete Design, and Masonry Design. However, Wood Design and Earthquake Engineering were ranked about 20% higher in the Western region than in other regions (Figure 4a, 4b, and 4c). It should be noted that although the “Other U.S. Territories or International” responses show a significant difference, this region consisted of less than 1% of the responses, and the low number of respondents may have skewed the difference in response. Additionally, the survey successfully attracted a diverse sample of respondents, as shown in Figures 2 and 3. Most respondents primarily work on buildings, consistent with the NCSEA membership.
Figure 4a.
Figure 4b. Practitioner response on the importance of Wood Design, by region.
Figure 4c. Practitioner response on the importance of Earthquake Engineering, by region. Very important; Graduates should complete a full course in this subject Important; Subject should be covered in the curriculum Not important; Subject is not essential and may be included as an elective
The Practitioner Survey provided the committee with insight through actual survey responses. However, personal comments by practitioners provided a greater understanding of how they value the education of structural engineering students and what they view as important to sustaining their profession and business. For example, technical communications and writing skills were strongly acknowledged in the 2016 survey responses, and this trend was noted to be even stronger in the 2020 survey. Additionally, when
asked about the importance of including soft skills such as communication, creativity, flexibility, leadership, public speaking, and teamwork in the engineering curriculum, 44% thought these topics are very important, and a majority of course work should be spent on soft skills, 53% agreed that it was important but not a primary focal point. As one respondent explained: “We have had students start at our firm with graduate degrees who were far less prepared for starting work than others who only had a 4-year
Very Important: Majority of course work should be spent on subject
Important: Should be covered, but not a primary focal point
Not Important: Brief overview, but course focused on other topics such as the use of structural analysis software
Figure 5a. Importance of emphasizing classical “hand” methods of structural analysis such as the portal method, moment distribution, and slope deflection in the undergraduate curriculum.
undergraduate degree. A university education can only teach so much. It cannot make up for students lacking soft skills which should have been learned in High School. It cannot serve as a substitute for the very needed training and mentoring by their employer after graduation.” – practitioner
Most of the personal responses expressed views and opinions regarding the need for understanding load path, classical structural analysis methods, and interpreting results. Many practitioners also expressed the need for students to be involved with real-world applications, design projects, introduction to full building design and load path, and the building code and design process (Schematic Design, Design Development, Construction Documents, and Permitting). Detailing and construction techniques were common critiques mentioned by respondents.
Likewise, many respondents (~43%) feel that basic knowledge and hand calculation methods are required. However, computer programming, modeling, and software are needed at the university level to complement students’ education (~57%). Figure 5a shows the response distribution from a question on the survey regarding classical calculation methods and computer modeling. From the responses, it can be derived that structural analysis and classical methods should not leave the curriculum. However, understanding structural behavior and interpreting computer analysis results are essential.
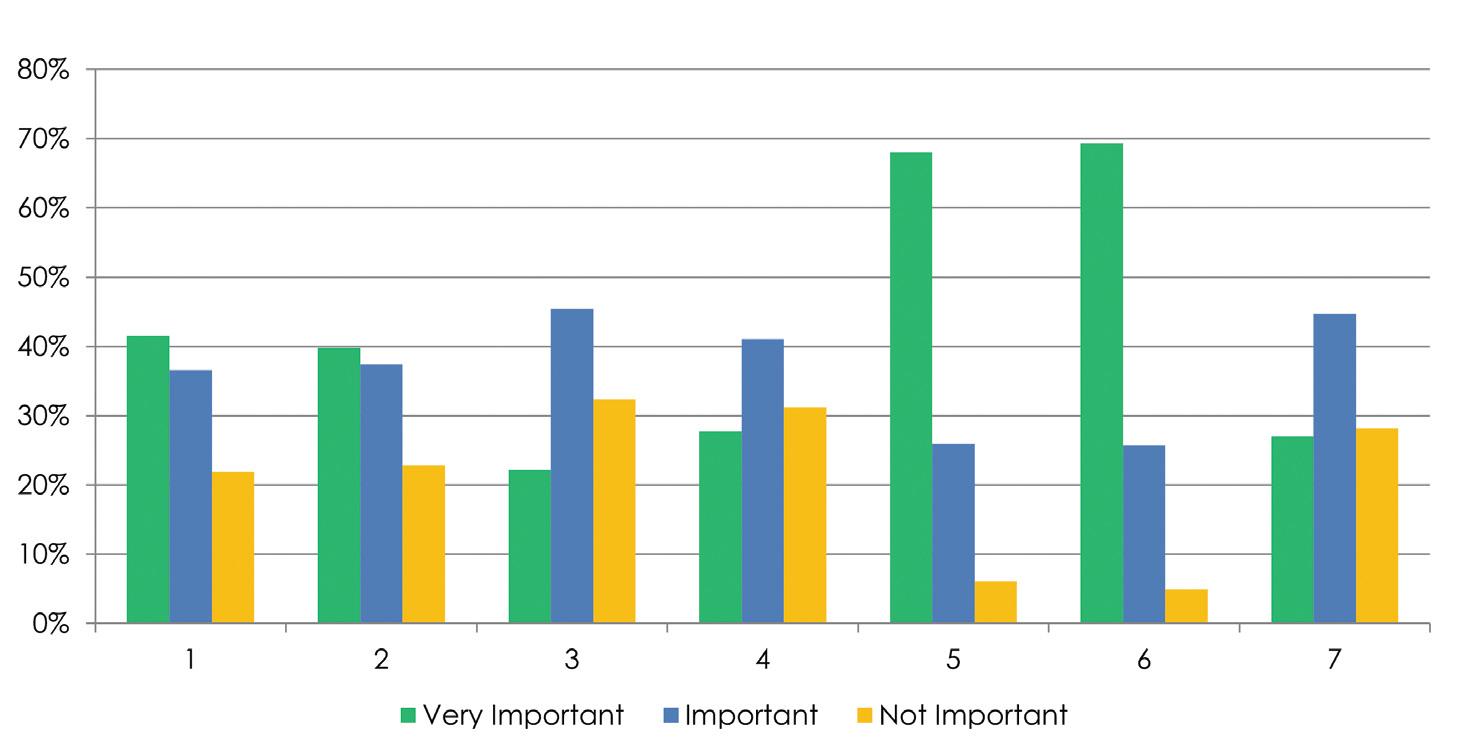
Like the previous Practitioner Survey, current survey results highlighted the need for students to bridge the gap between using computer models and successfully checking and understanding results (Figure 5b).
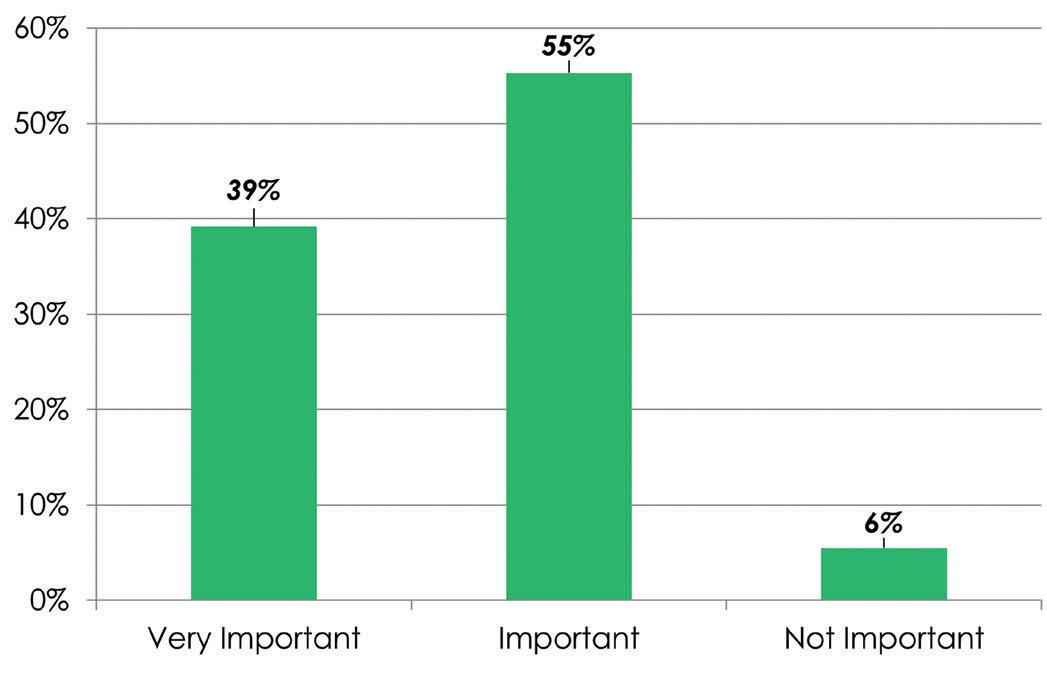
The Practitioner Survey also highlights the need for practical application of material topics, understanding the building code, and detailing and understanding building systems rather than just building elements.
Furthermore, the education of structural engineering students is vital to sustaining and safeguarding the profession. Without proper training and knowledge, billable time is potentially affected, along with concerns for public safety.
Practitioner vs. Curriculum Surveys
The Practitioner and Curriculum survey participants were both asked to identify if the NCSEA Recommended Structural Engineering Curriculum courses and the additional subjects listed above were either 1) very important – graduates should complete a full course in this subject, 2) important – subject should be covered in the curriculum, or 3) not important for new graduates entering the workforce. The survey results indicate practitioners and educators agree on the recommended structural engineering coursework. However, a closer comparison of the Practitioner Survey and Curriculum Survey results yielded some identifiable differences.
For example, Figure 6a (online) shows that while practitioners and educators both agree that Steel Design II, Concrete IIA: Advanced Reinforced Concrete Design, Wood Design, Masonry Design, Structural Stability, and Wind Design should be considered either a very important or important component of a structural engineer’s education, the practitioners generally felt that these courses needed a complete course in the subject while the educators felt that these courses should be covered in the curriculum but did not necessarily warrant a dedicated course. Technical Communications was also identified as either very important or important by both the practitioners and educators. Still, educators generally preferred that Technical Communications be taught in a dedicated course. In contrast, practitioners were agreeable to covering this topic as part of the curriculum but not necessarily as its own course.
As shown in Figure 6b (online), a significantly larger percentage of engineers felt a separate course was necessary for teaching both load paths/load flow and structural stability courses. At the same time, educators generally agreed that these courses were important but did
1 Preliminary design of systems (lateral or gravity load resisting systems)
Preliminary structural member sizing
Lateral analysis of buildings/bridges/systems
Structural analysis of building elements (beams, columns, walls)
Verify and interpret computer analysis results
Understand structural behavior 7 Structural design (code based for common materials, load resisting systems, and elements)
Figure 5b. Importance of “hand” calculation methods such as the portal method, moment distribution, and slope deflection, when completing certain tasks in the workplace.
not respond that they needed to be standalone courses. Sustainable Design and Construction Management were both viewed by educators as more essential topics than by practicing engineers, as indicated in Figure 6c (online)
Practitioner and educator survey participants were also surveyed about the preparedness of new graduates entering the workforce with the question, “Are new graduates and rising professionals with an undergraduate degree adequately prepared when entering the workforce?” Practitioners and educators overwhelmingly agreed that graduates with only an undergraduate degree are generally not prepared, with over 72 percent of practicing engineers and 83 percent of educators responding to the question with “Not so much – they have a general understanding but need more technical knowledge and skills.” Survey participants were also asked, “Are new graduates and rising professionals with a graduate degree adequately prepared when entering the workforce?” Eighty percent of educators and 62 percent of practitioners responded, “Most definitely – they have the tools and skills and are ready to perform entry-level structural engineering tasks.”
Summary
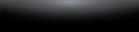
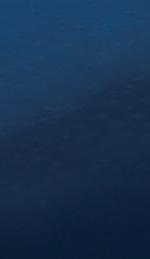
The BEC’s goal is to assess the surveys’ results to look for consensus and divergence of opinions between the academic and practicing professionals about the courses and topics students complete and how they prepare them to enter the workforce. One possible result of these surveys would be considering changing the recommended curriculum. Based on the survey results, this does not seem warranted at this time. However, a curriculum change alone will not satisfy nor meet all needs of the profession nor address the evolving nature of structural engineering. In addition, these courses are only one piece of the preparation, training, and skill requirements sought in graduating students by structural engineering firms. The survey responses help define where the structural engineering community seeks additional education. NCSEA will continue to look for gaps in student preparation and attempt to provide appropriate resources to fill these gaps and better serve the profession. These surveys aim to influence the education topics for structural engineering students, promote continuing education of design professionals, and identify the qualifications of entry-level engineers who will shape the profession’s future. Ultimately, the BEC would like these surveys to be utilized by students, universities, and the structural engineering community. Additionally, the surveys
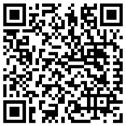
provide an avenue to better understand educational requirements, raise awareness of the obstacles to providing the recommended curriculum, and hopefully, inspire the industry to support and augment student education.■

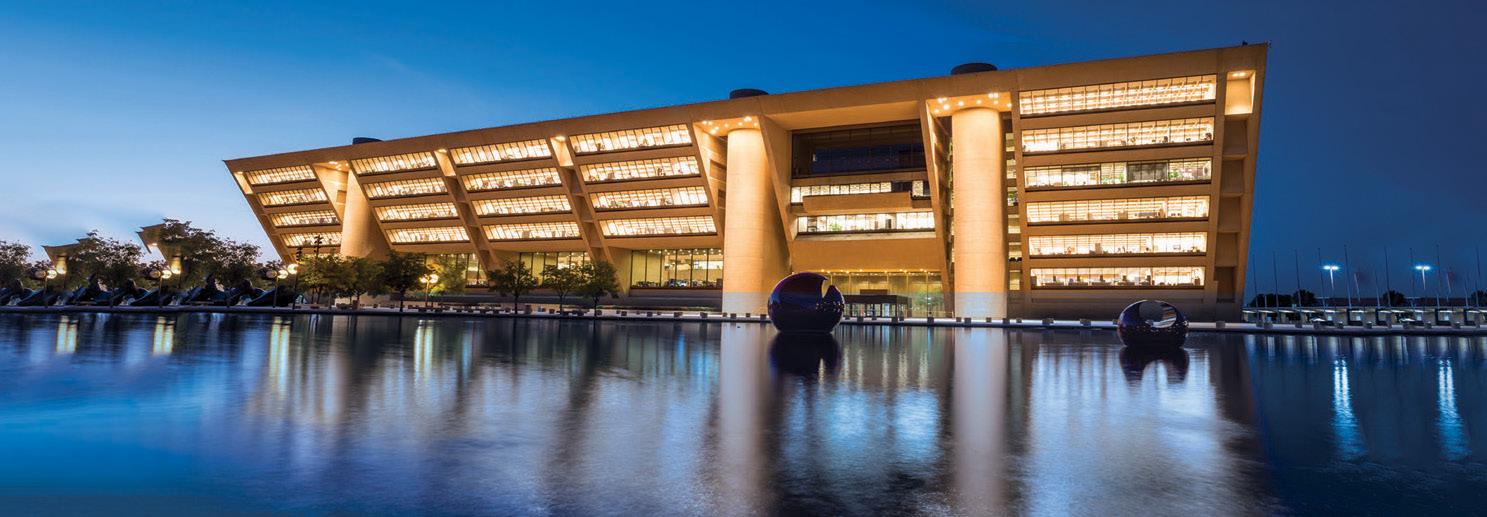
Michelle Kam-Biron is a Mass Timber Specialist with Structurlam Mass Timber Corporation (mkambiron@structurlam.com).
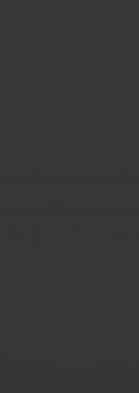
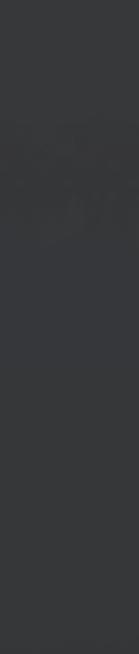
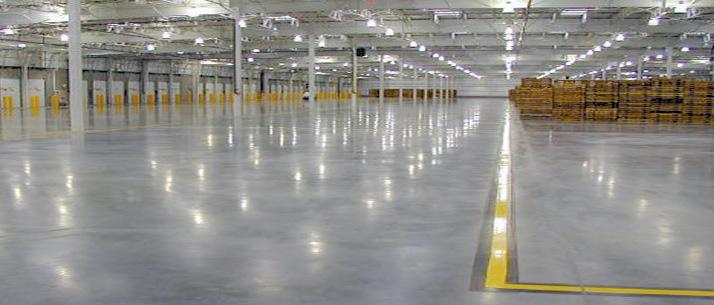
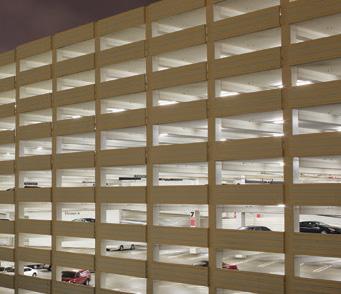
Brent Perkins is a Senior Project Engineer with Dudley Williams and Associates, P.A. (bperkins@dwase.com).
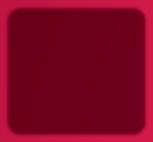
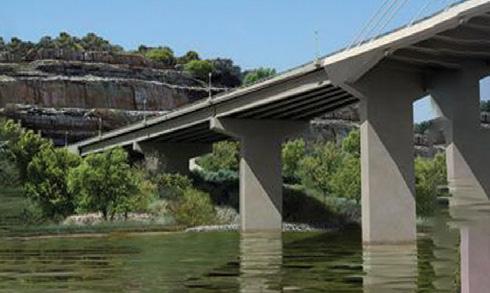
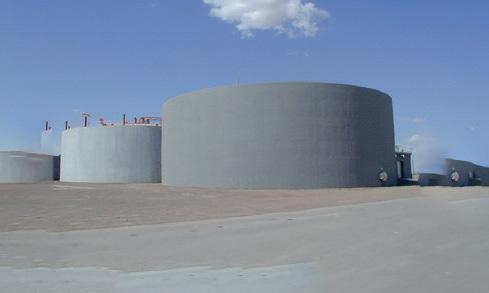
Scott M. Francis is a Project Manager and Buildings Group Leader with Wiley|Wilson (sfrancis@wileywilson.com).
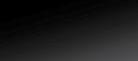
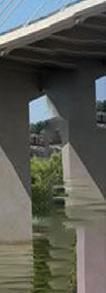
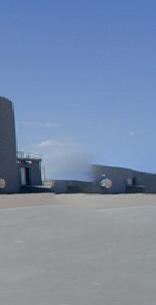
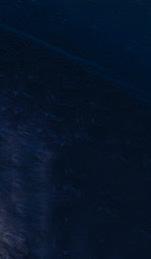
historic STRUCTURES
Fr. Hennepin Bridge, Minneapolis, Minnesota, 1855
19 th Century Mississippi River Bridges Series
By Frank Griggs, Jr., Dist.M.ASCE, D.Eng, P.E., P.L.S.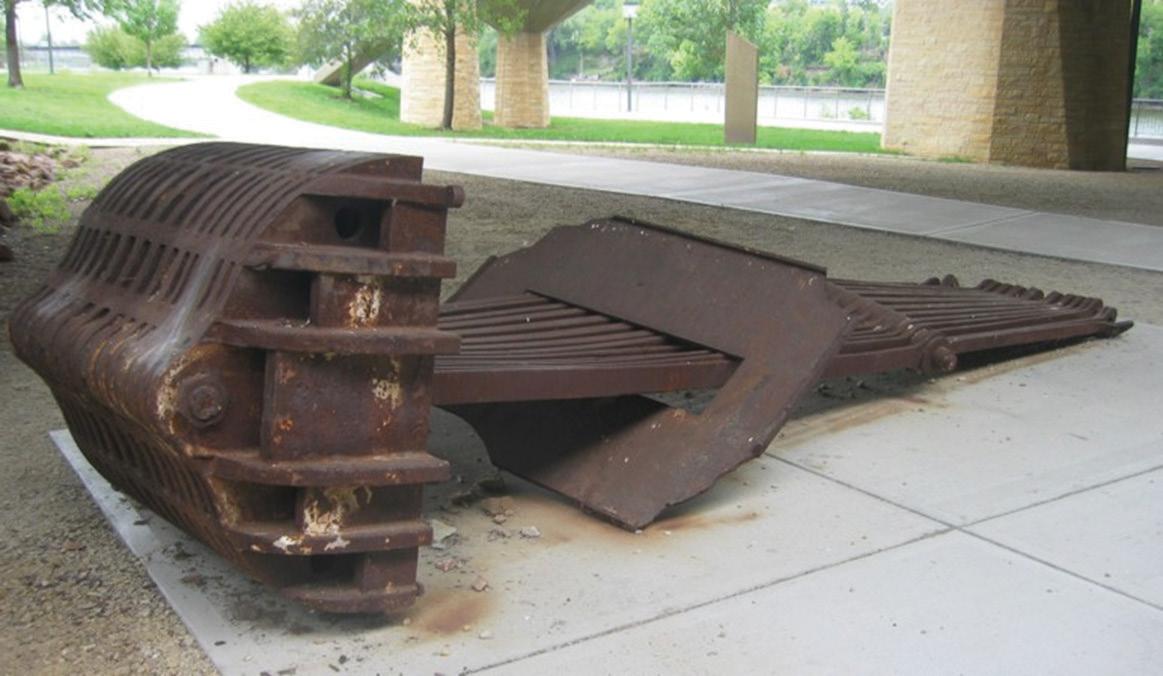
The Mississippi was the last major river to be crossed as the nation moved to the west. From its headwaters in Lake Itasca in Northern Minnesota to its discharge into the Gulf of Mexico, the river, with its tributaries, drained the entire United States between the Appalachians and the Rocky Mountains. It had a watershed of 1,245,000 square miles or approximately 40% of the country’s total land mass. It formed the boundary of ten states; Minnesota, Wisconsin, Iowa, Illinois, Missouri, Kentucky, Tennessee, Arkansas, Louisiana, and Mississippi. With the advent of the steamboat, it became a major shipping lane, and shippers wanted free navigation of the river without bridge piers.
In the early 19th Century, wood bridge builders like Timothy Palmer, Theodore Burr, and Lewis Wernwag built the first long-span bridges across the rivers in the eastern United States, precursors to the long spans that would be needed to cross the mighty Mississippi. They were followed by the iron truss bridge builders, Squire Whipple, Wendel Bollman, and Albert Fink. Then, starting with Charles Ellet, Jr., John A. Roebling, and Edward W. Serrell, iron wire cable suspension bridges were built across the Schuylkill, Niagara, Ohio, and Monongahela Rivers. These were built in relatively developed regions of the east and close to iron works and existing rail connections. The Mississippi, while not on the frontier, was close to it, and everything needed to build bridges had to be brought in from the east.
1855-1877.
approaches by engineers and bridge companies. In addition, many of the needed bridges required the approval of two states and the Federal government before being built. This series of articles chronologically describes how engineers of the 19th century met these challenges and designed and built bridges to cross the mighty river starting in 1855.
The Mississippi, with the challenges of required long spans, was a river that was tidal for much of its length and whose water level could vary as much as 20 feet in times of flood. Crossing the river required innovative
Fr. Louis Hennepin, a Franciscan Priest, first explored the upper Mississippi River. In 1680, he discovered St. Anthony Falls while searching for the river’s source. He named it in honor of St. Anthony of Padua. Two branches of the river flowed around Nicolett Island, and over time the cities of Minneapolis on the west bank and St. Anthony on the East Bank were settled. The only place the river could be crossed without a ferry was above the Falls on a rock ledge in the summer and over the ice in the winter.
As the population grew, Franklin Steele and John Stevens, two local businessmen, built a pile bridge from St. Anthony to the island and set up a rope ferry across the western channel. In March 1852, they received a charter from the Territorial Legislature (Minnesota did not become a state until 1858) to form the Mississippi Bridge Company. The questions were what kind of bridge should be built and who would design and build it? At the time, the population of the two cities totaled less than 1,500, and, even with the charging of tolls to cross the bridge, costs had to be kept to a minimum. In addition, a large number of piers in the river, even though on rock, would be expensive and hard to maintain given the problems with ice.
These constraints pointed to a suspension bridge with wrought iron wire cables and wooden or stone towers. Ellet’s 1842 Schuylkill River
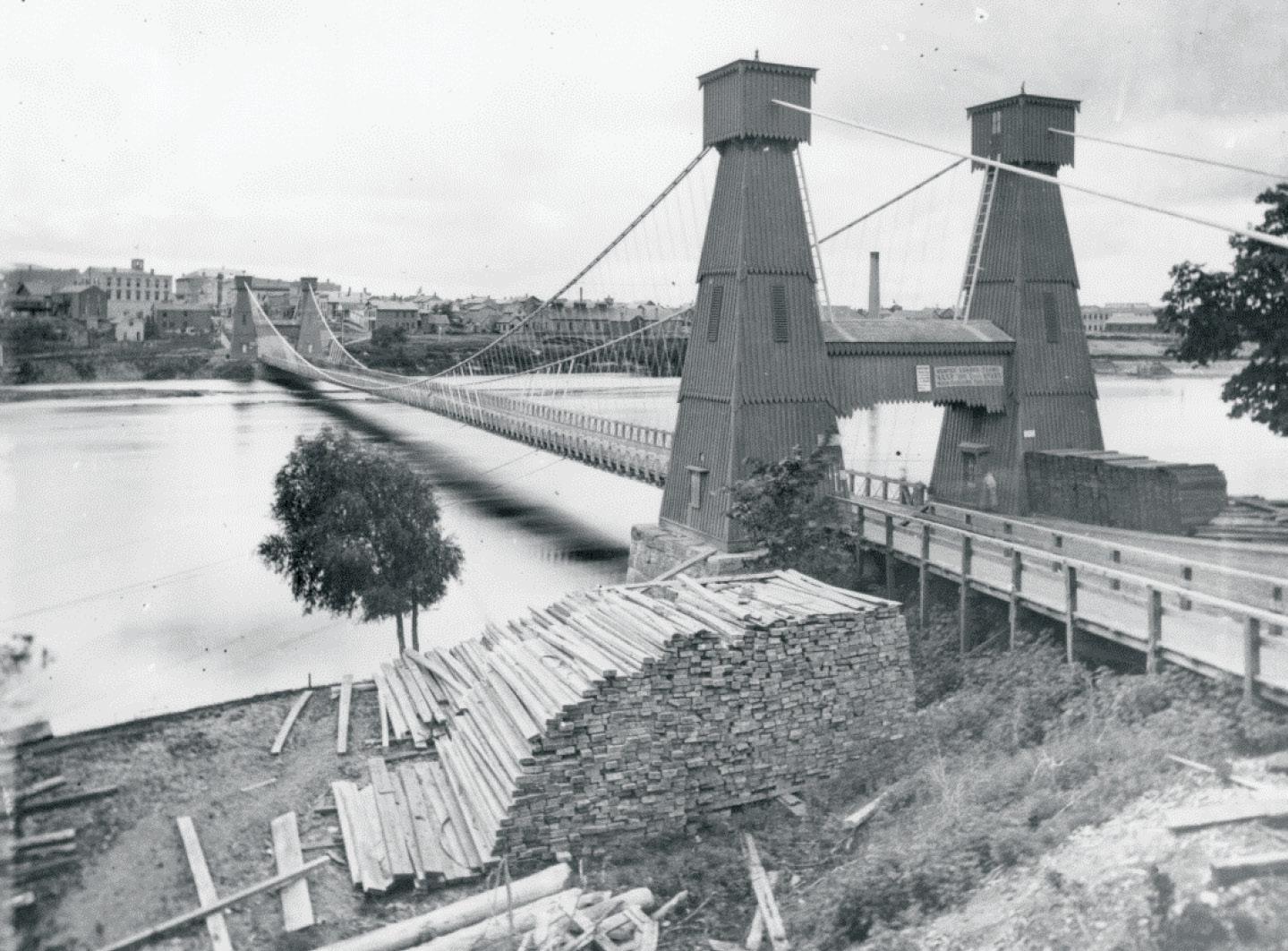
had stone towers, and his temporary 1849 bridge over the Niagara River had wooden towers. His 1,010-foot-span Wheeling Bridge across the Ohio River in 1849 had stone towers. Edward W. Serrell built his Lewiston/Queenston bridge across the Niagara River in 1851 and his St. John, New Brunswick Bridge in 1853, with Thomas M. Griffiths as his assistant, using wrought iron wire cables and stone towers. All of John A. Roebling’s short-span bridges, primarily for aqueducts, also had stone towers, and he was in the process of replacing Ellet’s Niagara Bridge with stone towers.
The Bridge Company selected Griffiths to design and build their bridge, probably with the help of Serrell, in 1854. His wooden towers “were strong frames of white pine timber, consisting each of 16 posts, 12 inches by 12 inches, so arranged that at each corner of a frustum of a right pyramid, with a square base of 14 feet by 14 feet, there were four respectively, but separated each from each by a space sufficient for the ventilation of the timber, and the passage of the tie bolts necessary to hold them together, and up to the system of interbracing. These posts were capped with three courses of white oak timber, the direction of the grain being reversed in each tier, and the whole trenailed through and through.” Cross bracing connected the towers at about the third point, and the entire tower was covered in wood, including widened caps over the tops of the towers.
His anchorages were custom-made to fit the site’s geology, which consisted of a 10-foot layer of limestone underlain by a layer of sand. Griffiths wrote, “The anchorage was obtained by working eight holes, eight inches wide and three feet long, through the limestone rock which is ten feet in thickness and of nearly horizontal stratification; underneath which lies the white sand. Through these holes, the link bars of the anchorage chains were thrust and secured to cast iron plates of about 1500 pounds each, which were taken into their positions underneath the ledge through tunnels driven into the easily excavated sand. Thus a very secure anchorage was obtained, at a comparatively small cost.”
For his cables, he wrote, “The four cables contained in all 2,000 strands of No. 10 hard drawn charcoal iron wire, made by Messrs. Cooper & Hewitt [Trenton, New Jersey]. These cables were formed of skeins of 124 and 126 strands, which were ‘laid up’ on the Island and moved and raised to their positions on the towers.” Note there were two separate “skeins” for each cable, and they were not wrapped into a single cable. From these cables, his suspenders “made of eight rounds of No. 10 wire formed into a skein; the upper end or ‘bite’ of which passed over a cast-iron yoke, which embraced the two cables
on either side. The lower end or ‘bite,’ with a small casting interposed, supported either end of the floor beams.” The cables had a sag of 47 feet and were cradled 10 feet, meaning they were 10 feet closer together at midspan than at the tower for added lateral stiffness. The suspenders were spaced 3 feet 9 inches on-center and sloped from the cables back toward the towers.
His deck was 17 feet wide with 3½ by 14 inches cross floor beams placed 3 feet 9 inches on-center (the same as the spacing of the suspenders). Light stringers ran the length of the bridge with a deck surface of oak planks. Railings were in the form of Howe trusses for safety and added stiffness under each cable and down the centerline of the bridge. When done, Griffiths had built an inexpensive (~$36,000) suspension bridge with a main span of 620 feet across the Mississippi. It was opened on January 23, 1855, in a grand ceremony during the dead of a Minnesota winter. In his opening speech, the Territorial Governor, Willis A. Gorman, stated, “Who knows, but this mighty structure may yet bear the commerce of the Pacific, as it mingles with that of the Atlantic!” Griffiths, in writing about the opening, waxed poetic, “… a hastily extemporized pageant glided at full gallop over the snow-covered rolling prairie (so soon to teem with the industry of a city), amid the jingle of sleigh bells, which in their numbers, vied supremacy over the discordant clang of a recently organized frontier band; and with the National colors borne aloft, from hill to hill, unfurled to full length in the dazzling sunlight by the Northern breeze, the generous enthusiasm of the occasion, gave vent to praises loud and long in honor of the bold projectors of the enterprise, and of those who had been mainly instrumental in its success.”
The bridge was damaged in a wind storm shortly after its opening but rebuilt in a short time. The toll for pedestrians was 3¢, or 5¢ for a round trip, horses and mules cost 15¢, cows and oxen cost 10¢, and pigs and sheep cost 2¢.
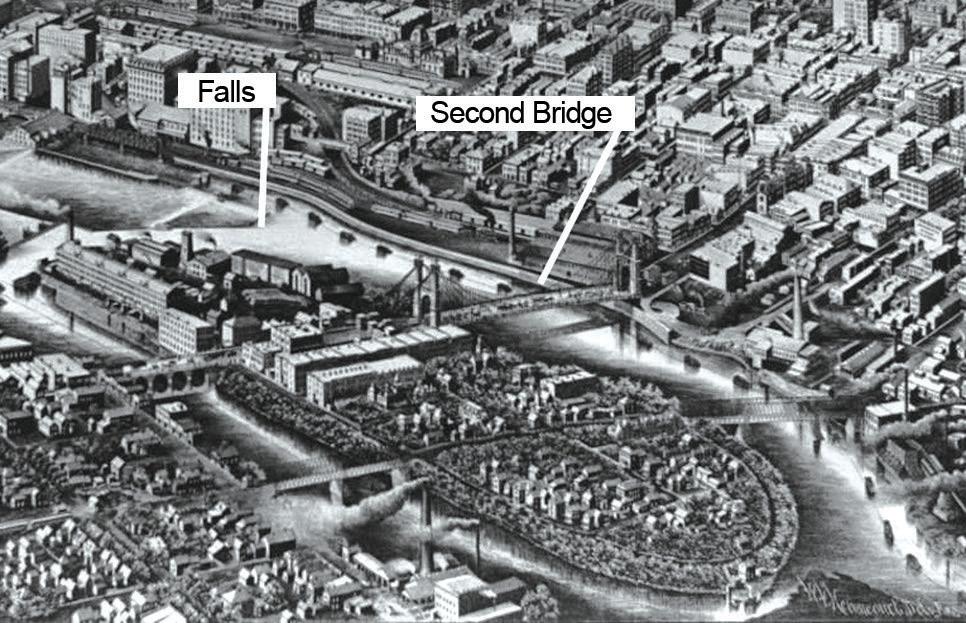
It was replaced in 1877 by Griffiths with another suspension bridge with ornate stone towers. Later in 1891, it, in turn, was replaced by a three-span steel arch bridge. However, the Fr. Hennepin Bridge will always hold the title of the first bridge across the Mississippi. Currently, another suspension bridge, the fourth at the site, opened in 1990 and continues to serve traffic.
■
Dr. Frank Griggs, Jr. specializes in the restoration of historic bridges, having restored many 19 th Century cast and wrought iron bridges. He is now an Independent Consulting Engineer (fgriggsjr@twc.com).
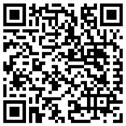
The Mississippi, with the challenges of required long spans, was a river that was tidal for much of its length and whose water level could vary as much as 20 feet in times of flood.
Altair
Phone: 248-614-2400
Email: sframe-sales@altair.com Web: www.altair.com/s-concrete
Product: S-CONCRETE
Description: A trusted industry standard, quickly and accurately designs reinforced concrete beams, columns, and walls. Reduce design time by automatically designing thousands of concrete elements simultaneously to produce a comprehensive engineering design report. Now with ACI 318-19 design code support for continuous concrete beams. Free trials available.
ASDIP Structural Software
Phone: 407-284-9202 Email: support@asdipsoft.com Web: www.asdipsoft.com
Product: ASDIP STEEL
Description: An advanced software for the design of steel members and connections, such as composite/ non-composite beams, steel columns, base plates, anchoring to concrete, shear connections, and moment connections, per the latest design codes. ASDIP STEEL comes with 5 intuitive modules that will substantially simplify time-consuming calculations for your structural designs.
DEWALT Anchors and Fasteners
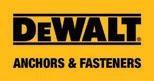
Phone: 800-524-3244 Email: anchors@dewalt.com Web: http://anchors.dewalt.com/anchors Product: CCU+ Critical Connection Undercut™ Description: DEWALT Anchors and Fasteners launches a new heavy-duty concrete anchor for use in critical applications where a robust anchor with low displacement is necessary. e CCU+ Critical Connection Undercut is ICC-ES qualified under ESR-4810 for use in cracked and uncracked concrete. e anchors are Made in the USA.
ENERCALC, Inc.
ENERCAL C
Phone: 800-424-2252 Email: info@enercalc.com Web: https://enercalc.com Product: ENERCALC Structural Engineering Library
Description: Library's newest modules are Flitch Plated Wood Beam and Steel Base Plate by FEM. Both modules can help designers refine design loads on anchor rods, common bolts, and framing anchors. Our subscriptions now provide both installed and cloud use, plus ENERCALC 3D FEM and earth retention modules.
ANCHOR guide
Hohmann & Barnard
Phone: 800-645-0616
Email: jenniferm@h-b.com Web: h-b.com
Product: ermal 2-Seal™ Anchors
Description: Available for all types of construction backups and feature stainless steel barrels to seal both the insulation and the air barrier. ermal 2-Seal Anchors are designed to decrease thermal transfer and allow for continuous insulation in wall assemblies.
IES - Easy Structural Software
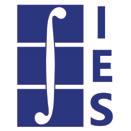
Phone: 800-707-0816 Email: info@iesweb.com Web: www.iesweb.com Product: IES VAConnect
Description: Design base plates by AISC Design Guide #1 or FEA and perform anchorage calculations for ACI 318. Both, independently, are difficult by hand! With VAConnect you will get the job done quickly and accurately. Works alone or with IES VisualAnalysis. Free trial download.
Listings are provided as a courtesy, STRUCTURE is not responsible for errors.
RISA Technologies
Phone: 949-951-5815 candicec@risa.com risa.com
RISAConnection
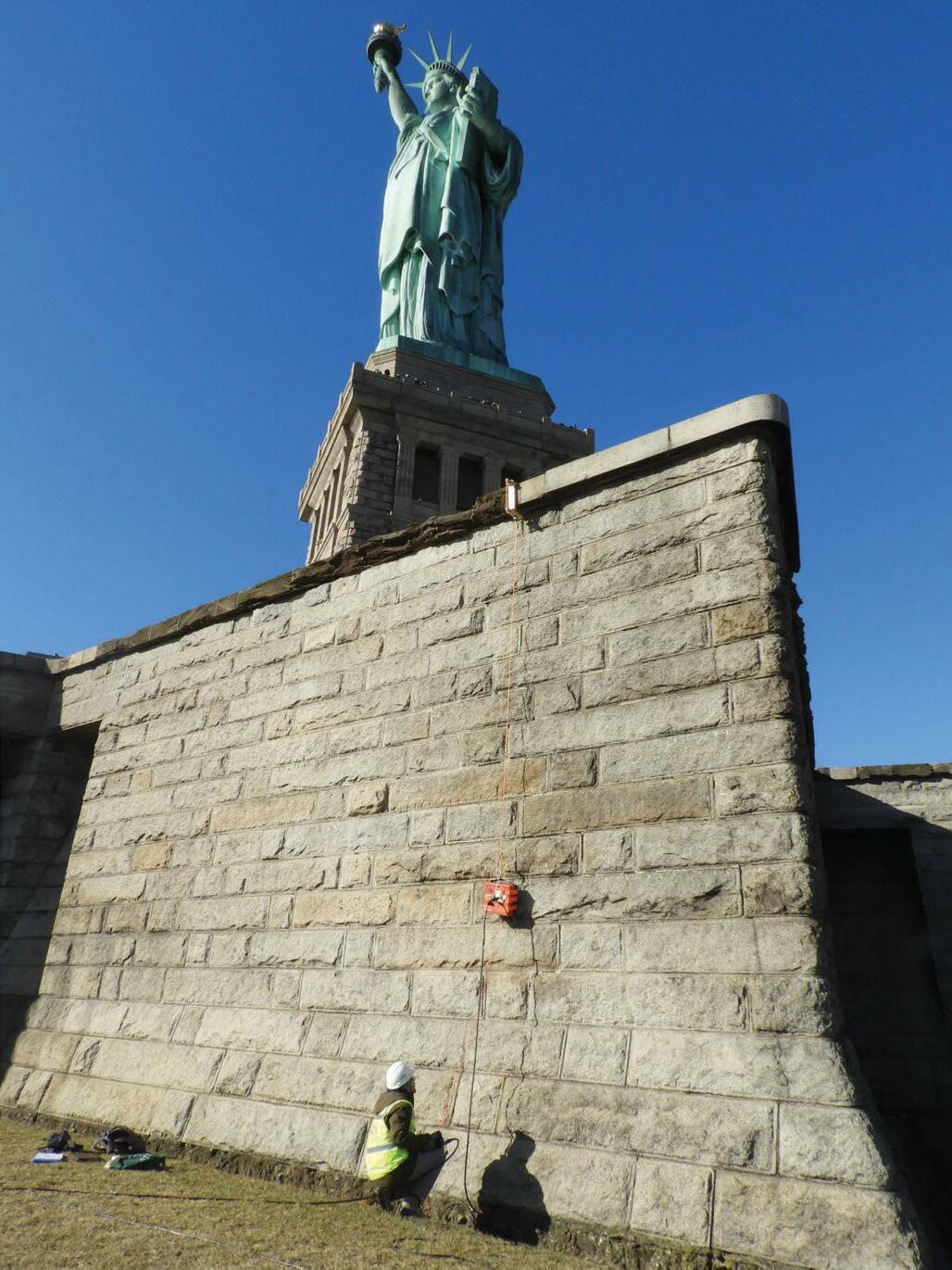
Description: e cutting edge of next-generation connection design software and features full 3-D visualization as well as expandable reports for every limit state. RISAConnection includes integration with Hilti Profis for anchorage design, support for column cap plate moment connections, and updated HSS tube connection design according to the Canadian Steel Code.
Phone: 678-737-7379 jodi.hendrixson@trimble.com www.tekla.com/us
Tekla Structures
Description: An Open BIM modeling software that can model all types of anchors required to create a 100% constructible 3-D model. Anchors can be created inside the software or imported directly from vendors that provide 3-D CAD files of their products.
Tekla Structural Designer
Description: With Tekla Structural Designer, engineers have the power to analyze and design multi-material buildings efficiently and cost effectively. Physical, information-rich models contain all the intelligence needed to fully automate design and document projects, including end force reactions communicated with two-way BIM integration, comprehensive reports, and drawings.
Williams Form Engineering Corp.
Phone: 616-866-0815
Email: williams@williamsform.com Web: www.williamsform.com
Product: Anchor Systems
Description: Williams Form Engineering Corporation has been providing threaded
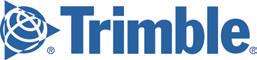
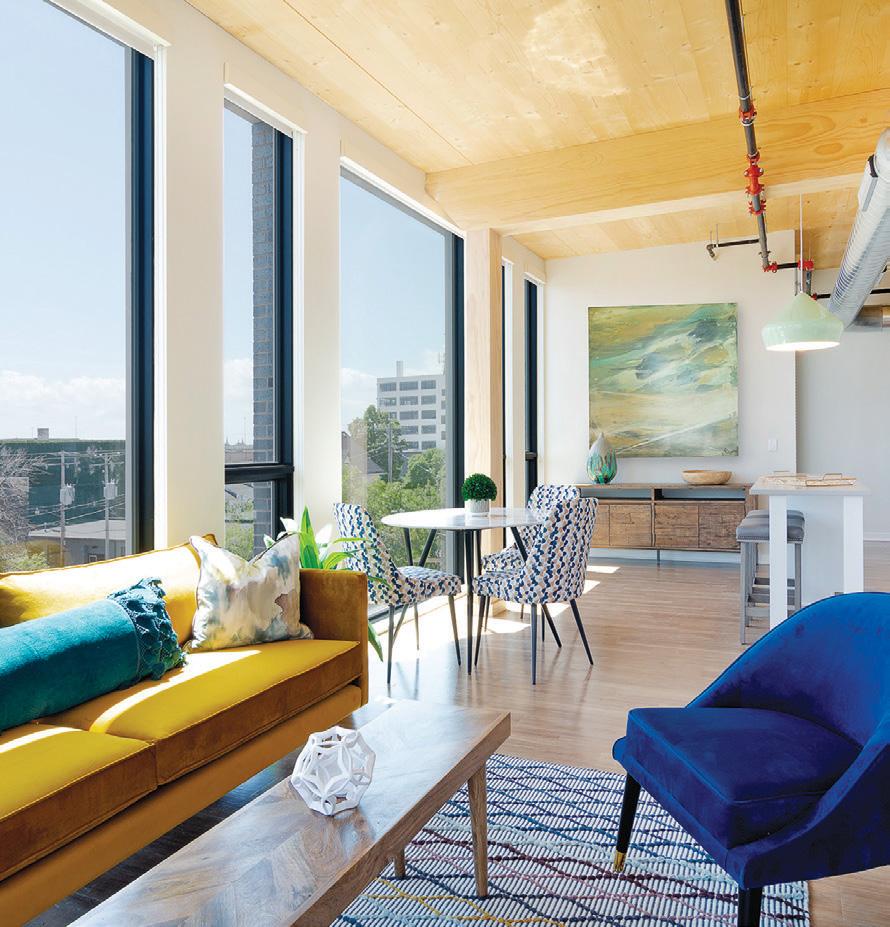
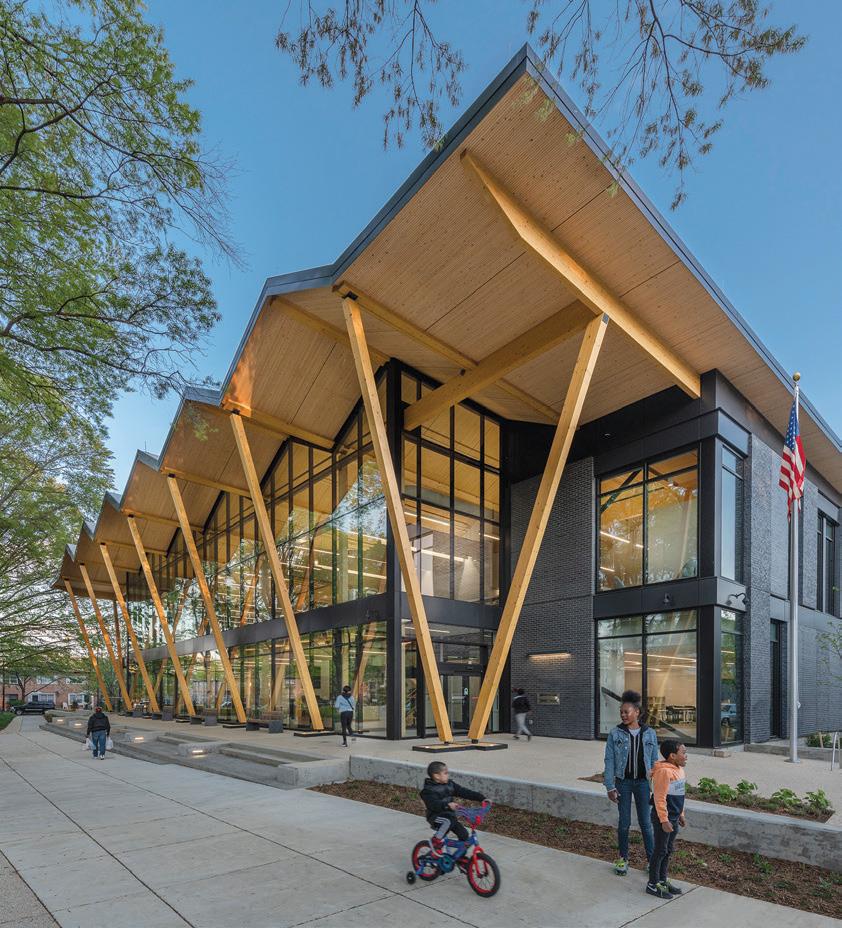
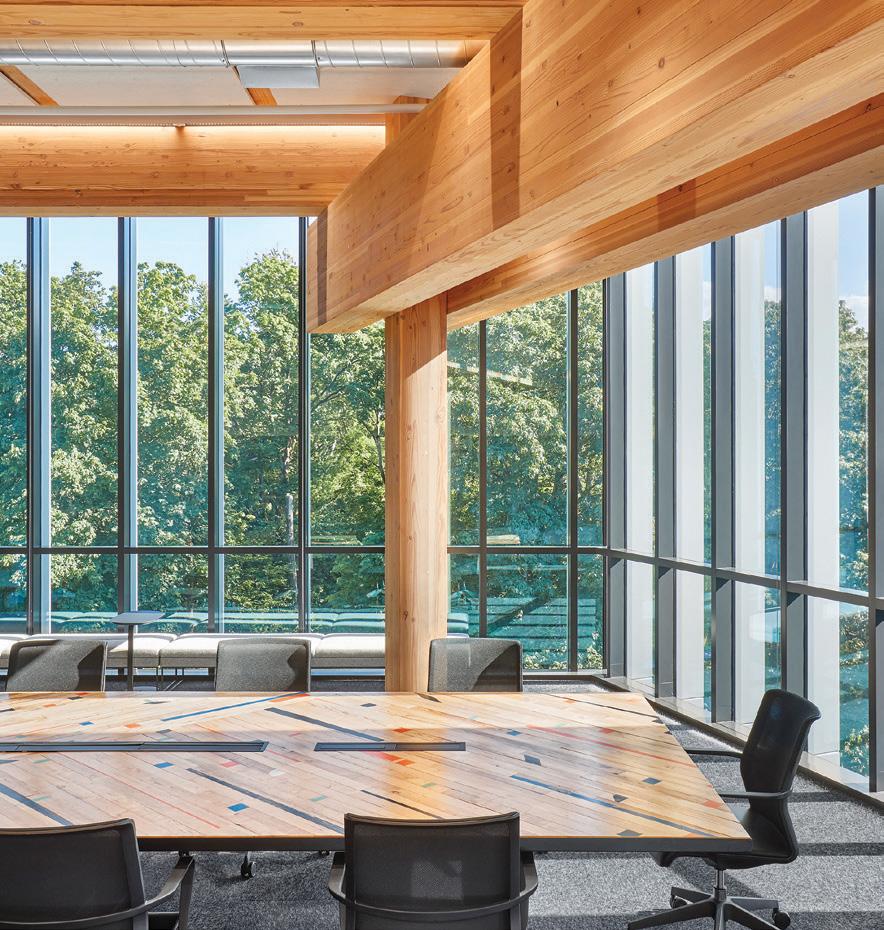
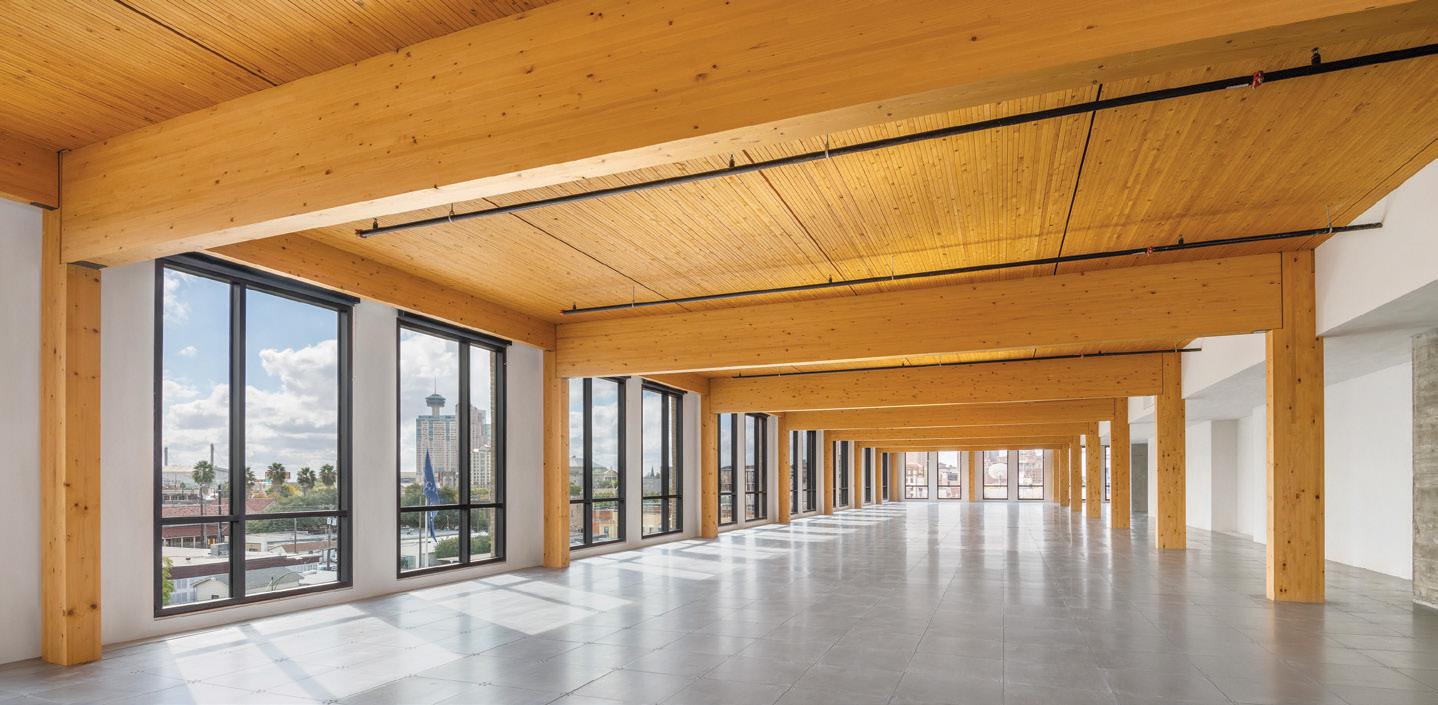
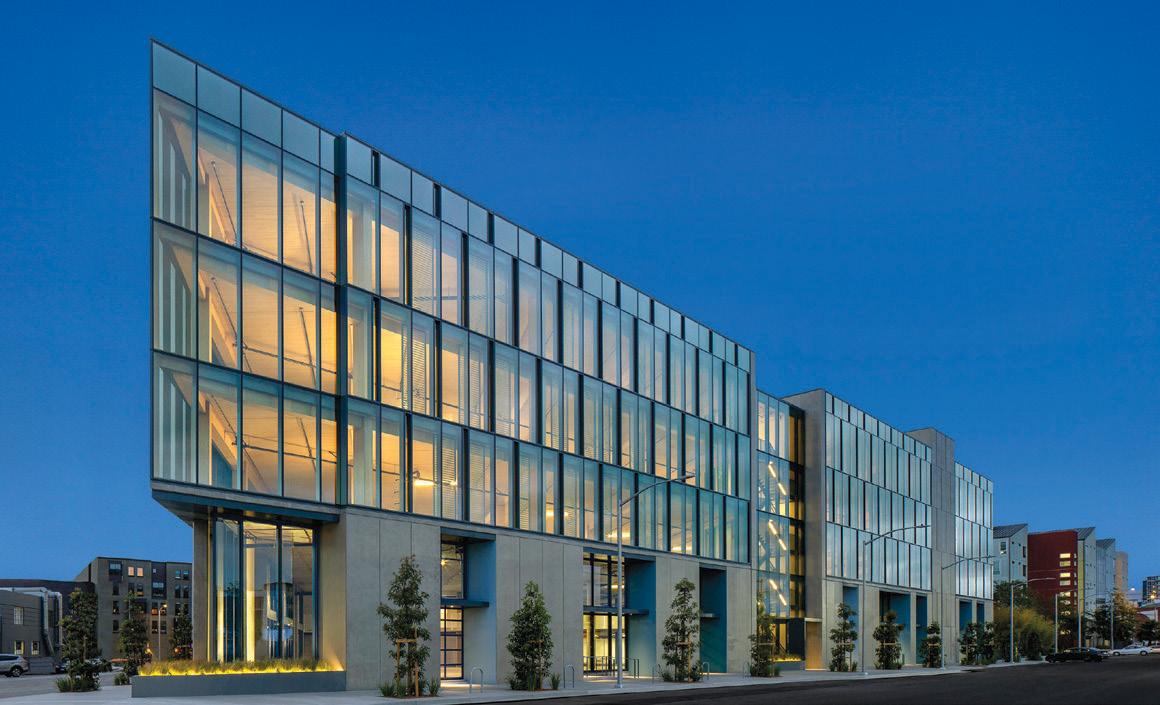
STRUCTURAL ENGINEERING Resource Guide
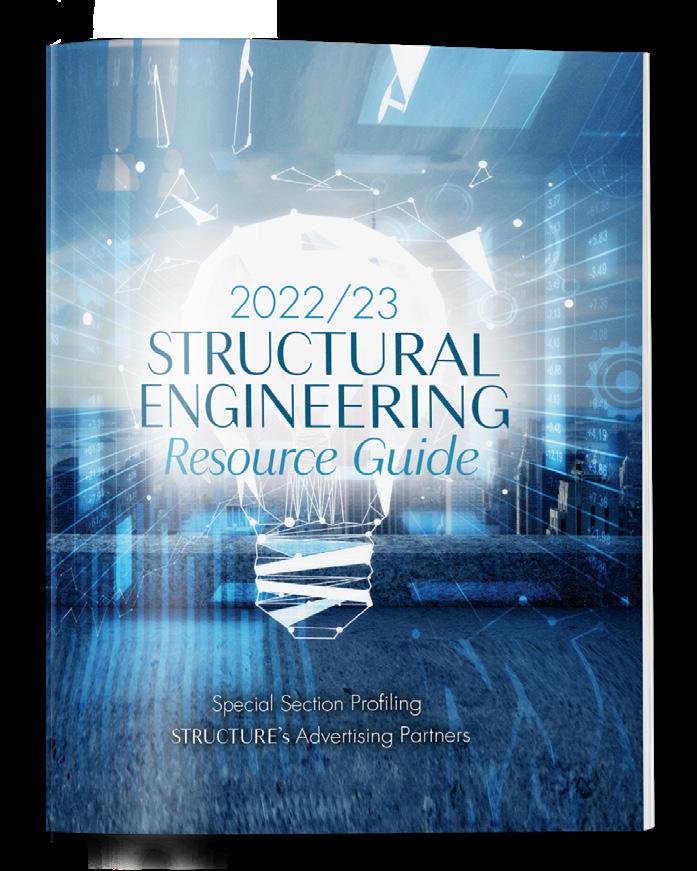
Included in the October edition of STRUCTURE magazine, this highly anticipated resource includes category listings of products relevant to SEs.
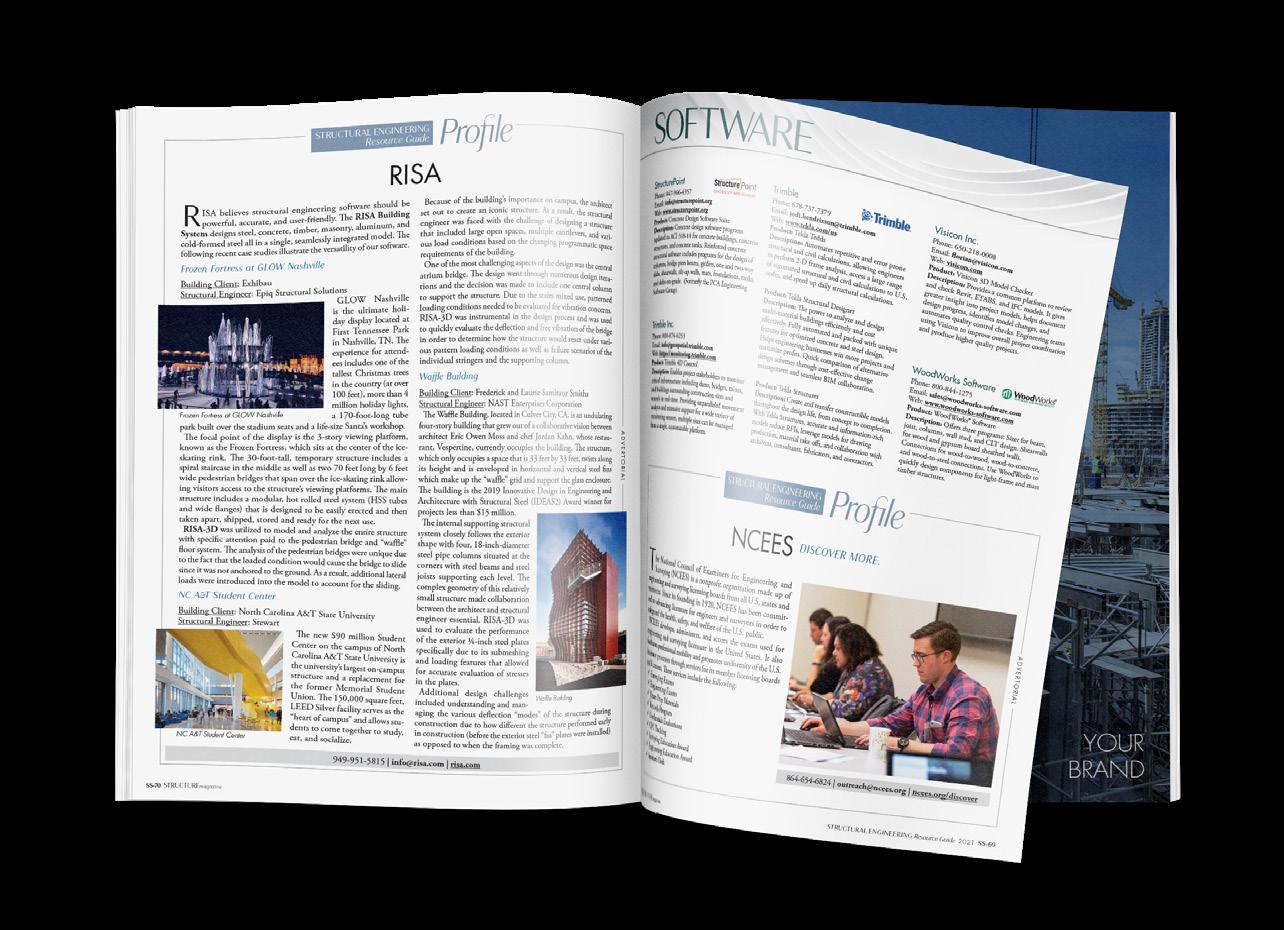
Also included are STRUCTUREsolutions Profiles – full-page or half-page Company Profiles created from supplied editorial copy and graphics. Profiles are available for vendors and engineering firms!

CASE business practices
Working with a Geotechnical Engineer
By ACEC Staff and CASE Executive CommitteeStructural engineers charged with designing a structure depend upon the input of geotechnical engineers to determine the appropriate foundation type for a particular site and building design. Although both are critical team members on a project, the geotechnical engineer and structural engineer may be engaged through different processes, and each may have different clients though parallel responsibilities.
Structural engineers are often the most qualified members of the design team to advise the owner on what services, recommendations, and documentation are required from a Geotechnical Engineer on their project. Aside from the geotechnical engineers themselves, SEs have the best understanding of the likely structural and foundation systems and loadings, the implications of site soil characteristics on the structural design, and the most common local foundation design and construction strategies.
A request for a proposal for geotechnical engineering services should be prepared by the owner or owner’s representative (architect) with input from the structural engineer. The RFP should include building type, anticipated loads, and specific design criteria desired by the design team and/or required by building codes. The selection of a geotechnical engineer should be based primarily on qualifications rather than fees. The right geotechnical engineer reduces risks and could result in savings for the Owner in construction costs for the project. (A comprehensive list of the Geotechnical Engineer’s scope of work is contained in CASE Tool 6-2.)
One of the geotechnical engineer’s primary scopes of service is to prepare a geotechnical report that includes data from field and laboratory work which documents the data collected, the geotechnical engineer’s interpretation of the information, and his/ her recommendations for foundations and earthwork for the proposed project. There is no fixed template for a good geotechnical report. The geotechnical issues for a particular project depend on the local geology, site topography, project scope, and applicable code. A geotechnical report should be prepared early in the project before finalizing significant design decisions. The geotechnical report provides vital information for the design team to complete their work.
Guidance available from CASE
• CASE Guideline 962-I: Structural Engineer’s Guide to Working with a Geotechnical Engineer
• CASE Tool 6-2: Scope of Work for Engaging Subconsultants
• CASE Contract #10: An Agreement Between Structural Engineer of Record and Geotechnical Engineer of Record
Although the Geotechnical Report may be provided to the contractor as a reference document, it should be clearly stated that the report is not part of the contract documents prepared by the structural engineer. The geotechnical report is provided for informational purposes only since subsurface conditions are never guaranteed. Such reports often contain non-mandatory language, multiple foundation options, or references to proposed designs that are no longer part of the project. The contract documents themselves need to define the entire scope of work for a project without relying on reference documents such as a Geotechnical report. Examples would be defining the depth of over-excavation or minimum pile embedment. However, the structural engineer can cite the geotechnical report (title/author/date) in the structural drawing set as a basis of design.
The geotechnical engineer should be retained to review the Contract Documents before they are issued for bids. The purpose of such a review is multi-fold. It gives the geotechnical engineer a chance to ensure the design team interprets and implements their foundation design recommendations correctly. Also, if the project design has evolved since the geotechnical report was written, as it frequently does, the geotechnical engineer can be brought back into the communication loop. The last review confirms that the design still reflects the best geotechnical advice. It is also a good time to confirm the Special Inspection program for the foundation work that can be spelled out in the relevant specification sections. The geotechnical engineer can provide, or at least edit, the earthwork, deep foundation spec sections, and Foundation portion of the Statement of Special Inspections.
Geotechnical recommendations in a project geotechnical report are typically based upon the results of limited subsurface exploration. As a result, geotechnical recommendations are considered preliminary until the actual site conditions are verified through
field observation and testing. Geotechnical observations and testing include verification of fill material and compaction requirements, confirmation of bearing conditions for shallow building foundations and proper installation procedures for deep foundations, and the supervision of pile load tests. These tests and observations are critical to ensuring that the recommendations included within the geotechnical report are consistent with actual field conditions and result in adequate foundation design and construction. As such, they should only be performed by a qualified, licensed geotechnical engineer.
Structural engineers should either directly specify or strongly recommend to owners that the geotechnical engineer for the design phase be retained to perform geotechnical observations and testing for the foundations, even if that engineer is not retained to perform observations and testing for the entire project. The project geotechnical engineer has the benefit of involvement in the project’s design phase. The continuity in responsibility for the original geotechnical recommendations and on-site verification of those recommendations provides clarity in any legal disputes that may arise. When the owner engages a second geotechnical engineer, state engineering practice laws and regulations should be referenced for guidance regarding liability.
A properly prepared geotechnical report for a project is essential for the structural engineer to adequately design and analyze the structures for which they are responsible. All parties involved should understand the critical role that the geotechnical engineer and the structural engineer play. Properly engaging both disciplines with explicit scopes of service and expectations enhances any project.■
The Coalition of American Structural Engineers (CASE) resources, a Coalition of the American Council of Engineering Companies, can be found at www.acec.org/CASE
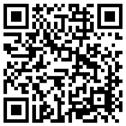
NCSEA News NCSEA


National Council of Structural Engineers Associations

2022 Diversity in Structural Engineering Scholarship Award Winners

Meet the Future of Structural Engineering!
e NCSEA Foundation Diversity in Structural Engineering Scholarship Program was established in 2020 to award funding to students who have been traditionally underrepresented in the profession. is year, the program received more than 50 scholarship applications from across the country.
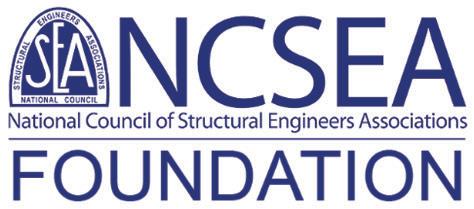
After thoroughly reviewing all the applicants, the NCSEA Foundation awarded six scholarships for 2022. Four were NCSEA scholarships, and two were the first-ever partner scholarships in this program. e SEA of Northern California (SEAONC) established an endowed scholarship as part of the NCSEA Foundation program, and the SEA-Metropolitan Washington (SEA-MW) established a named scholarship. Each student received a scholarship award of $3,000. In addition to the scholarship, each recipient is invited to attend the 2022 Structural Engineering Summit as a guest of NCSEA with a travel stipend to the event generously provided by Computers & Structures Inc.
SEAONC Diversity in Structural Engineering Scholarship
Ivett Luna Moreno –
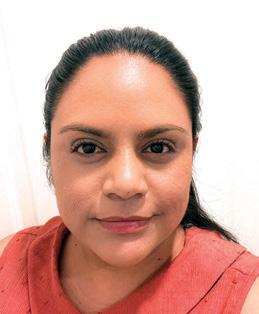
University of California San Diego
Ivett Luna Moreno is currently pursuing her master’s degree in Structural Engineering at the University of California San Diego. Her goal is to make significant contributions to the Structural Engineering profession and contribute to the community she came from. Having come from a disadvantaged situation, she recognizes the need to expose minority youth to future STEM careers as well as support the cultivation of their goals.
NCSEA Diversity in Structural Engineering Scholarship
Axel Soto – Virginia Tech
Axel Soto will be an upcoming graduate student at Virginia Tech. He loves to contribute ideas and solutions to a team to solve complex problems. After graduating, he plans to be a licensed structural engineer while also giving his time to his local community.
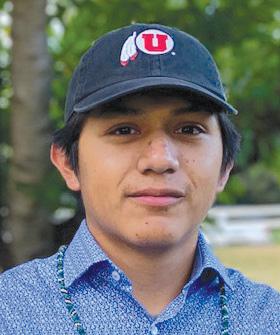
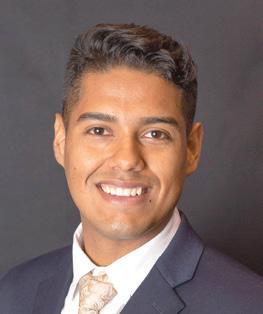
NCSEA Diversity in Structural Engineering Scholarship
Ravyn Rapley – Worcester Polytechnic Institute
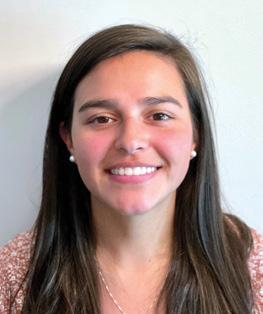
Ravyn Rapley is a rising junior studying civil engineering with a minor in gender, sexuality and women's studies at Worcester Polytechnic Institute. Following the completion of her bachelor’s, Ravyn plans to pursue a master's in structural engineering. Ravyn has an interest in the designing and construction of bridges. Ravyn is also an active member of the Black Student Union as well as the ASCE chapter on campus. is past year she was also involved in the Gender and Leadership Opportunity for Women workshop and the Leadership Delta Pearl Mentor program for BI-POC women on campus.
SEA-MW Diversity in Structural Engineering Scholarship
Malik Corum – University of Virginia
Malik Corum is currently pursuing a Ph.D. in Structural Engineering at the University of Virginia. Along with a Ph.D., he is also pursuing a graduate certificate focus in Cyber-Physical Systems. Being a recipient of this award is special because it reinforces his belief that diversity within structural engineering will lead to continued advances and progress within this field. His goal is to continue building his knowledge toolbox so that he may become an asset to the field of structural engineering.
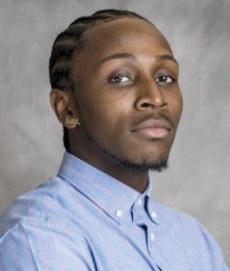
NCSEA Diversity in Structural Engineering Scholarship
Kameron Gonzales – University of Utah
Kameron Gonzales is a senior studying Civil Engineering at the University of Utah. Having grown up on both a reservation and a large city, Kameron has seen the drastic effects of poor infrastructure on Indigenous communities. As a member of the Shoshone-Paiute Tribes of the Duck Valley Indian Reservation, Kameron hopes to one day use his education to improve the infrastructure of his home reservation and others around the country.
NCSEA Diversity in Structural Engineering Scholarship
Larissa Lopez – Tennessee Tech University
Larissa is a senior civil engineering student at Tennessee Tech University concentrating in structural engineering. Prior to attending Tennessee Tech, Larissa graduated from East Tennessee State University with a degree in construction engineering technology and served as a project manager at a local construction company. Larissa lives in Middle Tennessee with her husband and their Australian Shepard, Benji.
News from the National Council of Structural Engineers Associations
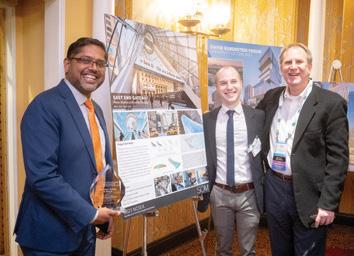
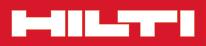
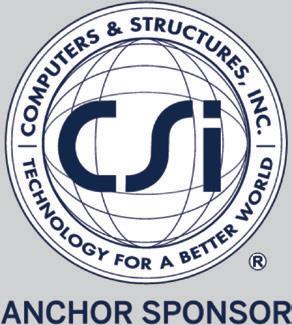
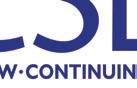
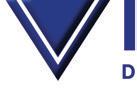
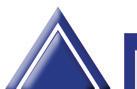
Will We See You in the Windy City?
• Over 14 Hours of high-quality, expert-led education.
Thank You to Our 2022 Summit Sponsors
and com-
• An amazing lineup of SE thought leaders from across the country.
• Keynote Speaker Adrian Smith FAIA shares his vision of the future of the built environment and the evolution of design professionals.
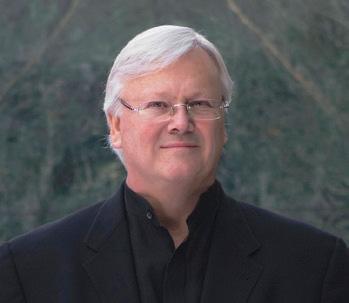
• Keynote Speaker Dr. Ivan Joseph teaches us his secrets to help individuals and teams reach goals they never thought possible.
• Access to the largest structural engineering-focused Exhibit Hall and leading industry suppliers with solutions for you.
• Opportunities to engage and interact with hundreds of practicing structural engineers—leaders in the field, influential minds, and curious problem solvers.
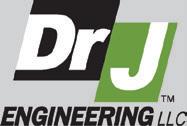

To learn more and register, visit ncseasummit.com.
Visit www.ncsea.com/education for the latest news on upcoming webinars and other virtual events.
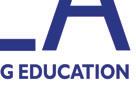
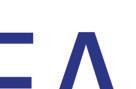
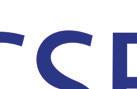
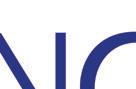
Purchase an NCSEA webinar subscription and get access to all the educational content you’ll ever need! Subscribers receive access to a full year’s worth of live NCSEA education webinars (25+) and a recorded library of past webinars (170+) – all developed by leading experts; available whenever, wherever you need them!
Courses award 1.0-2.0 hours of Diamond Review-approved continuing education after the completion a quiz.
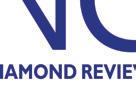
SEI Update
The 2022 SEI Standards Series
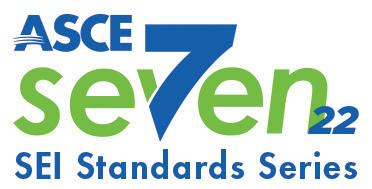
Review ASCE 7-22, changes from ASCE 7-16, the Digital Products/Hazard Tool, and join the discussion with the expert standard developers. 1.5 PDHs per session.
• September 8, 2022: How &Why to Use ASCE 7-22 in Your Practice Join SEI host Jennifer Goupil, P.E., F.SEI, M.ASCE for a discussion with guests Alexander Griffin, P.E., S.E. and Michelle L. Wilkinson, P.E., S.E., M.ASCE Learn more and register at https://collaborate.asce.org/integratedstructures/sei-standards.
Upcoming ASCE Continuing Education Live Programs
Snow Loads in ASCE 7-22, What’s New and Different
Instructor: Michael O’Rourke, P.E., Ph.D., F.SEI | .1 CEUs | Live Webinar Wednesday, September 7, 2022 – 12:00 pm - 1:00 pm ET
Significant Changes to the Wind Load Provisions of ASCE 7-22 Part 3 – NEW
Instructor: T. Eric Stafford, P.E. | .1 CEUs | Live Webinar Tuesday, September 13, 2022 – 12:00 – 1:00 pm ET
Designing for Tornadoes: New Requirements in ASCE 7-22 (GOCDTL23)
Instructor: Dr. Marc L. Levitan, Ph.D., A.M.ASCE | 1 CEU | Guided Online Course Monday, September 19, 2022 – Friday, October 21, 2022
Seismic Loads (GOCSL23)
Instructor: Finley A. Charney, Ph.D., P.E., F.ASCE, F.SEI | 2.4 CEUs | Guided Online Course Monday, September 19, 2022 – Friday, December 9, 2022
Practical Design and Renovation of Slabs on Grade – NEW (7054IW2022)
Instructor: Alexander Newman, P.E., F.ASCE | .4 CEUs | Virtual Workshop Wednesday, September 21, 2022 – Friday, September 23, 2022
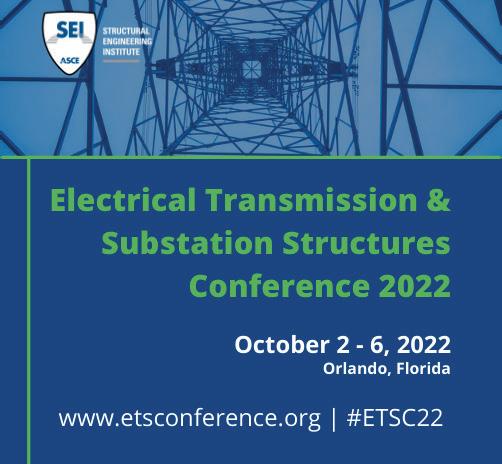
Arbitration and Mediation (CEW74092022)
– ASCE Week Las Vegas Webcast
Instructor: Nils J. Gransberg, Ph.D., AC, A.M.ASCE | .8 CEUs | Live Webinar Sunday, September 25, 2022 | 11:00 am – 8:00 pm ET
Structural Condition Assessment of Existing Structures
– ASCE Week Las Vegas Webcast
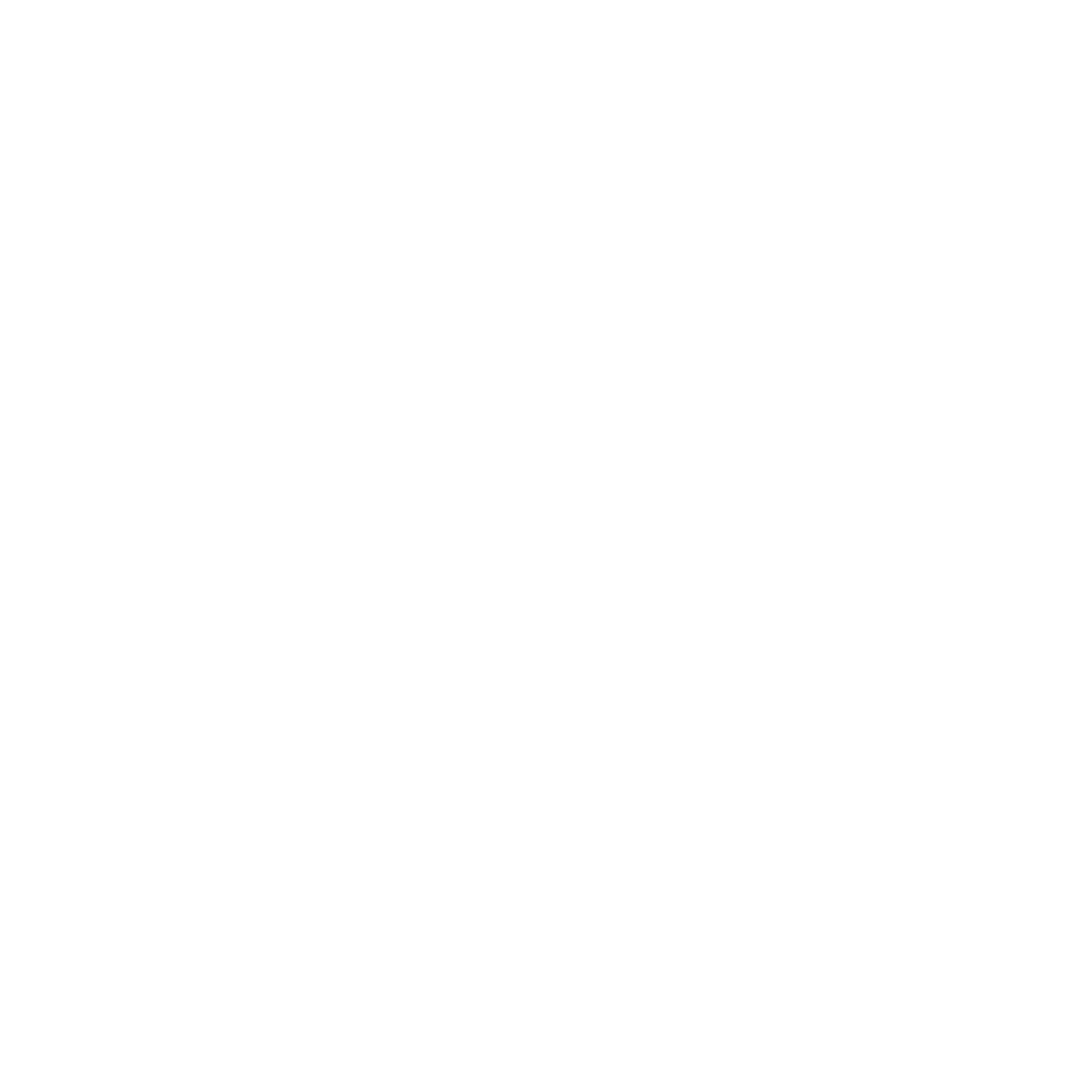
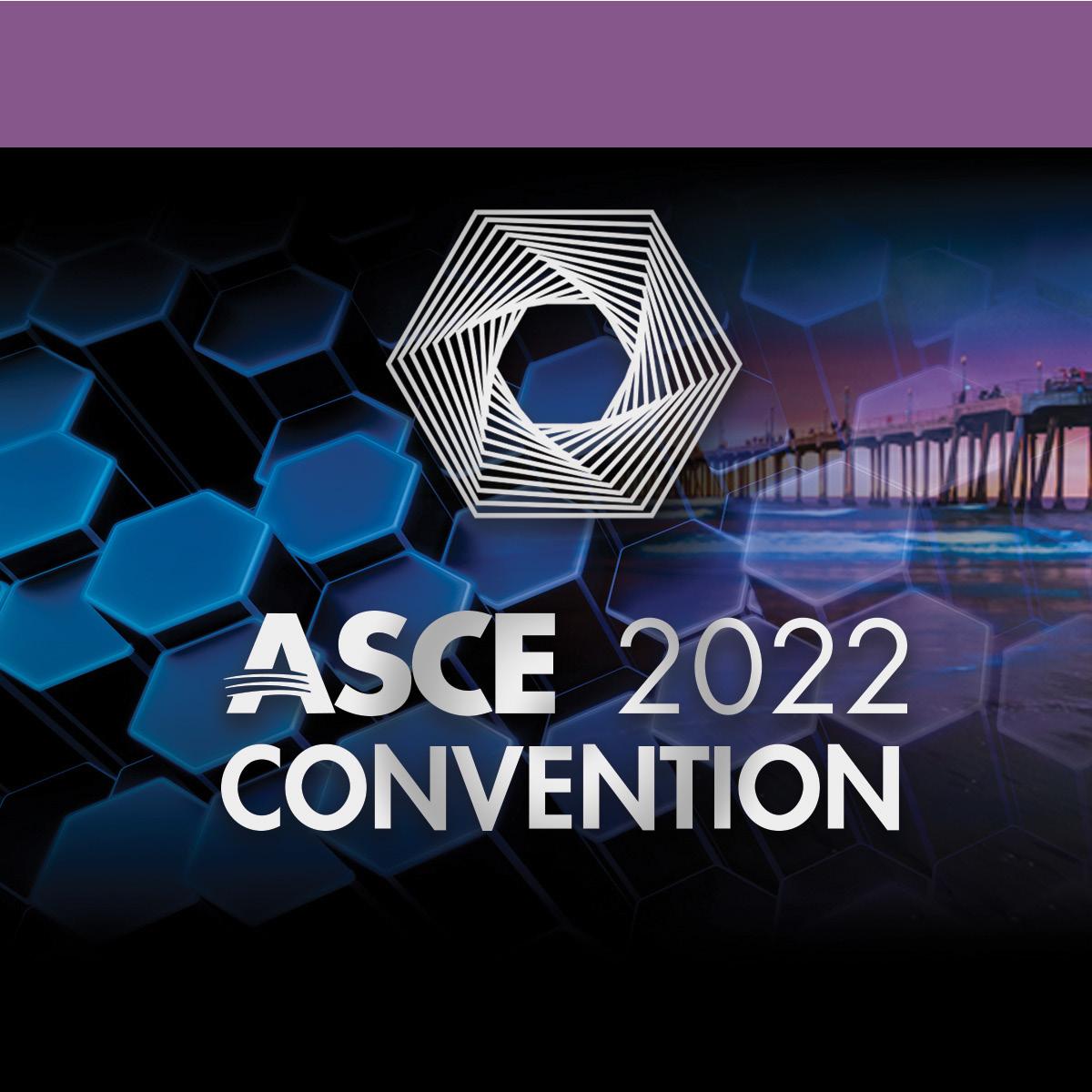
Instructors: Brian K. Brashaw, Ph.D., omas M. Gorman, Ph.D., P.E., M.ASCE, Larry D. Olson, P.E., M.ASCE, Dennis A. Sack, P.E., Steven Smith, CWI | Live Webinar ursday, September 29, 2022, and Friday, September 30, 2022
Learn more at https://mylearning.asce.org/diweb/catalog/item/id/9386038.
News of the Structural Engineering Institute of ASCE
Membership
Don’t Miss these Member Benefits
Join today at www.asce.org/SEIMembership.
• Infrastructure Investment and Jobs Act (IIJA) Resource Center Visit the IIJA Resource Center to understand and track implementation of the bipartisan infrastructure law. Includes legislative analysis, a list of open grant applications, opportunities to weigh in on implementation, and a schedule of upcoming funding distribution cycles.
•
AccessEngineering
Gain high-quality, multidisciplinary engineering information essential for professionals, faculty, and students. Get the right results fast through dynamic interactive features. Includes the most widely used McGraw-Hill engineering publications and preparatory materials for the P.E. and F.E. exams.
• Over 700 titles, including references, textbooks, and code commentary
• More than 150 problem-solving videos
• Extensive Excel spreadsheet calculators
• Topic- and role-specific webinars
• Interactive graphs and downloadable tables
Welcome to the New SEI Puerto Rico Chapter
Led by Ricardo Herrera, Jorge Hidalgo, and Dr. Gustavo Pacheco, the PR Chapter’s plans include promoting best practices by PR Structural Engineers; providing technical and professional education, resources, and events; promoting ASCE/SEI membership; collaborating with the recently established GI PR Chapter; and encouraging young engineers. https://sections.asce.org/puerto-rico
2022-2023 SEI Board of Governors
The SEI Board is composed of two representatives from each of the five SEI Divisions (Business and Professional, Codes and Standards, Global, Local, and Technical Activities), one young professional appointee, one appointee from ASCE, the SEI President, SEI Past President, and the SEI Managing Director as a non-voting member. The following includes recently elected SEI Board officers. Welcome to Governors starting terms October 1 – shown in italic:
Michael Joseph Bolduc, P.E., M.ASCE
Laura E. Champion, P.E., F.ASCE, SEI Secretary
John Cleary, Ph.D., P.E., F.SEI, M.ASCE
Jerome F. Hajjar, Ph.D., P.E., F.SEI, F.ASCE, SEI President-elect
Edwin Huston, P.E., F.SEI, M.ASCE, SEI Treasurer
Robin A Kemper, P.E., LEED AP, ENV SP, F.SEI, Pres.19.ASCE
Takahiko Kimura, P.E., F.SEI, M.ASCE
Chad Schrand, P.E., F.SEI, M.ASCE
Donald Scott, P.E., F.SEI, F.ASCE, SEI President
Kenneth Lee Sharpless, P.E., F.SEI, F.ASCE
Stephanie Slocum, P.E., M.ASCE
J. Greg Soules, Ph.D., P.E., P.Eng, S.E., F.SEI, F.ASCE Elaina Sutley, Ph.D., A.M.ASCE Victor E. Van Santen, P.E., S.E., F.SEI, F.ASCE, SEI Past President James Wacker, P.E., M.ASCE
Thank you for your service and leadership!
Finishing terms September 30: Randall P. Bernhardt, P.E., F.SEI, F.ASCE | Aimee Corn, P.E., M.ASCE | Joseph G. DiPompeo, P.E., F.SEI, F.ASCE
SEI Futures Fund Sponsors Externship for SEI Graduate Student Chapter, University of Texas, Arlington
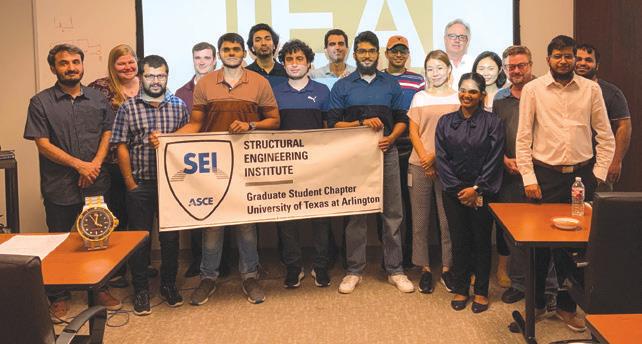
Fifteen students from the SEI Grad Student Chapter, University of Texas, Arlington, participated in an externship event on May 19 at the IEA Dallas Headquarters for an office tour, networking, and job-seeking tips with practice professionals and HR, meetings to review ongoing projects and workplace dynamics, and learning field-required software. The project was led by Chapter Chair Lihong April Mao, EIT, S.M.ASCE, and made possible by the SEI Futures Fund and the Local Activities Bridge the Gap program promoting student and professional member interaction. Learn more and give www.asce.org/SEIFuturesFund
Errata
SEI Standards Supplements and Errata including ASCE 7. See www.asce.org/SEI. To submit errata, contact sei@asce.org.
CASE in Point
Events for Structural Engineers
ACEC Fall Conference
October 16-19, 2022, Colorado Springs, Colorado

ACEC sponsors two major national meetings each year: the Annual Convention and the Fall Conference. National meetings provide attendees an opportunity to obtain information about issues that affect the industry through informative education, networking, and exhibits. The Coalition of Structural Engineers (CASE) hosts a roundtable at each meeting to discuss issues specific to the field of structural engineering. Hope to see you there! www.acec.org/conferences/2022-fall-conference

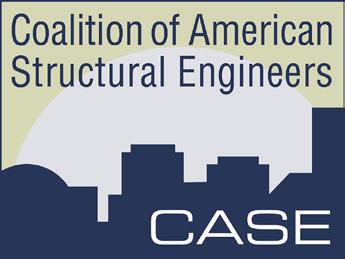
Business of Structural Engineering Bootcamp: Claims and Contracts
November 1, 2022, Chicago, IL
Are you positioned to succeed in a highly competitive profession? You know how to design and understand the code, but do you understand the business implications of your daily activities? NCSEA and CASE have teamed up to better equip the structural engineering leaders of today and tomorrow with tools they can use to excel at the business side of structural engineering. Join us Tuesday, November 1, 2022, from 8:30 am to 4:30 pm. www.ncseasummit.com
Student News
Your monetary support is vital in helping CASE and ACEC increase scholarships to those students who are the future of our industry. All donations toward the program may be eligible for tax deduction and you don't have to be an ACEC member to donate!
Donate today: www.acecresearchinstitute.org/scholarships
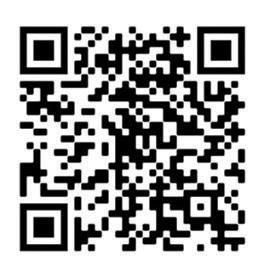
Congratulations to the 2022 CASE Scholarship winner, Taylor Drahota, from the University of Nebraska-Lincoln! View information about the scholarship and winners at acec.org under “Awards.”
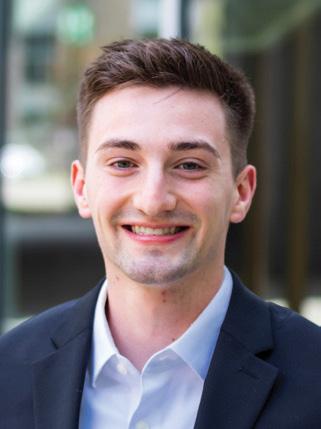
News of the Coalition of American Structural Engineers
CASE Tools and Resources
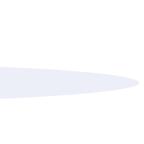
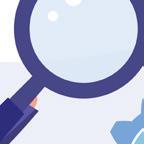
Get to Know the CASE Committees
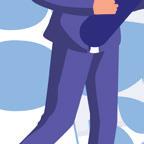
The CASE Coalition has several committees that meet regularly to develop documents that help guide engineers in their business practice. The work of these committees is an important part of what coalitions do and is one of the most significant values of CASE membership. The Toolkit Committee develops and distributes tools built around the 10 Foundations of Risk Management to reduce risk in member firms.
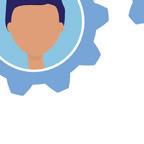
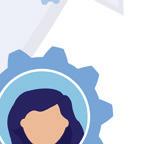
Structural engineers have the highest claims-to-revenue ratio among practitioners in the Architectural-Engineering (A/E) field. However, structural engineers do not necessarily have more claims made against them. Instead, these claims tend to be higher per claim than claims for other types of engineers or architects.
These Foundations were developed by engineers in private practice to help engineering firms focus their practice on avoiding and minimizing risk. The first five Foundations deal with the process of the engineering business, and the last five deal with project management.
This month, the Toolkit Committee met in person at the Coalitions Summer Meeting in Salt Lake City, UT. We are currently seeking new members to join the Toolkit Committee. Do you know someone in your firm looking for ways to expand and strengthen their business skillset, gain experience serving on a committee, sharpen their leadership skills, and travel to interesting places? Please consider applying for a position on the committee. Committee member commitments include a monthly virtual meeting, working on relevant documents a few hours a month, and travel to the winter and summer coalitions meetings!
To apply, your firm should:
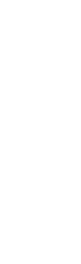
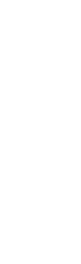
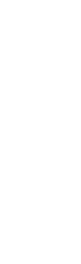
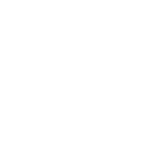
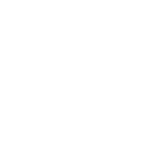
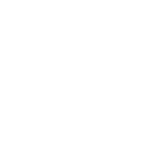
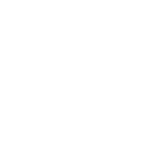
• Be a current member of ACEC
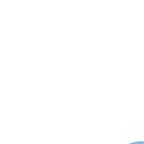
• Be a member of the Coalition of American Structural Engineers (CASE); or be willing to join the Coalition
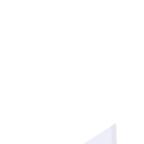
• Be able to attend the groups’ regular face-to-face meetings each year: August, February (hotel, travel partially reimbursable)
• Be available to engage with the committees via email and video/conference call
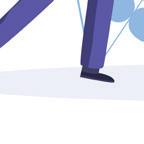
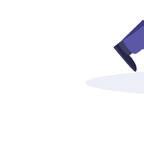
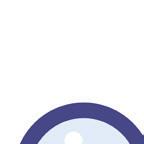
• Have some specific experience and/or expertise to contribute to the group
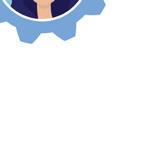
Did You Know?
CASE has tools and practice guidelines to help firms deal with a wide variety of business scenarios that structural engineering firms face daily. So whether your firm needs to establish a new Quality Assurance Program, update its risk management program, keep track of the skills their young engineers are learning at each level of experience, or need a sample contract document – CASE has the tools you need!
Check out some of the CASE Toolkit Documents:
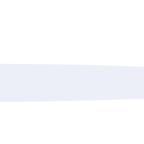
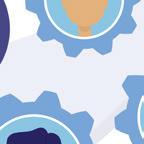
CASE Tool 1-1 – Create a Culture for Managing Risks and Preventing Claims. Initially designed for structural engineers with high claims-to-revenue ratios, this download is intended to inject a risk management culture into your firm. This is the first and most comprehensive tool offered on risk management in the engineering industry. It includes a video, a storyboard, and a role-playing guide to involve your staff in the risk management discussion. In addition, this Tool includes sample commitment statements for your firm to buy into the process. If you want to start your firm personnel on the path to sound risk management habits, this is where you start.
CASE Tool 2-1 – A Risk Evaluation Checklist. This tool provides one way for you to act in both a preventative and proactive way before starting a project in your office. The purpose is to evaluate risk by considering a variety of factors and perspectives and determining whether the risk(s) of the pursuit is a worthwhile investment of firm resources.
CASE Tool 3-2 – Staffing and Revenue Projection Tool. The Staffing and Revenue Projection Tool Firm and project planning are crucial to reducing your firm’s risk and avoiding claims. One major facet of firm planning is predicting upcoming revenue and staffing demand to ensure that your firm has the resources to service upcoming booked and potential projects adequately.
You can purchase these and other Risk Management Tools at www.acec.org/bookstore
If you are a member of CASE, this tool and all publications are free to you. NCSEA and SEI members receive a discount on publications. Use discount code – NCSEASEI2022 when you check out.
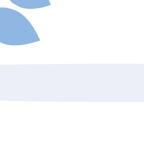
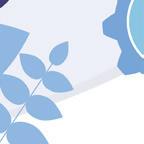
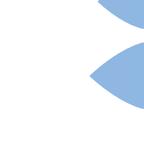
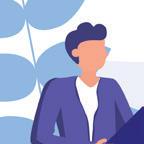
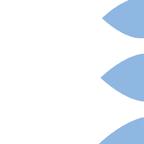
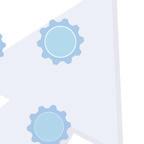
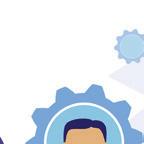
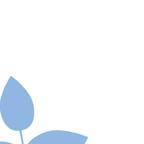
stakeholder PERSPECTIVES
The “Under-Demanded” Office Market
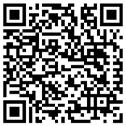
The following is a developer’s view of today’s built environment as a result of COVID and work-from-home restrictions. STRUCTURE believes it is important to visualize how these factors can influence the field we work in. This regional example is reflective of many areas around the U.S.
The swallows abandoned San Juan Capistrano in the 1990s. Their home – the historic mission – underwent a renovation; the birds lost their ancestral nests and, despite every effort to lure them back, they have yet to return in meaningful numbers. No less flighty, workers abandoned their office buildings in 2020. They, too, have migrated elsewhere. Those in real estate ask themselves when office employees will return. Others wonder if they will return. Back to that existential question in a moment.
Global Commercial Real Estate Services (CBRE) has just published the 2nd quarter results for San Francisco’s office market. Almost 29 percent of the city’s office space – about 25 million square feet – is now available. Vacancies increased by 362,000 feet in the 2nd quarter, and about 550,000 feet of brand-new office space is coming on line soon. In short, the vacancy rate is climbing toward a cloud-obscured peak. One industry executive wryly observed, “We’re not overbuilt, we’re under-demanded.”
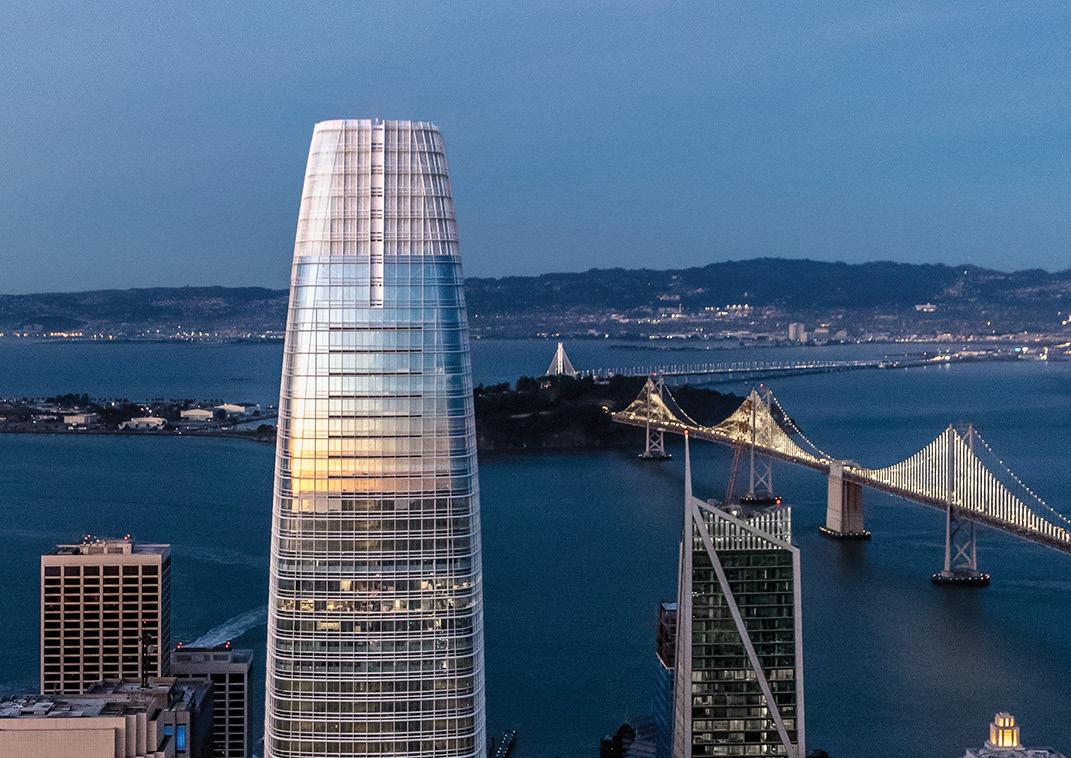
Turning this data around, 71 percent of the city’s buildings are leased. Sadly, that figure is somewhat misleading. In these “covidy” times, a building’s occupancy rate is a far more critical metric than its leased rate. Kastle Systems, a workplace security company that requires office employees to swipe entry cards, provides precise occupancy data. As of this writing, San Francisco’s overall occupancy rate – the workers who actually show up – is 39 percent.
A 39 percent occupied building may have a happy ending, but like falling in love with someone with a bad heart, you could find yourself praying in the emergency room before it is all over. Meanwhile, with the exception of the swankiest buildings – say, Sales Force Tower –rents are plummeting; tech is down 24 percent on the NASDAQ and shedding workers like winter coats in Miami.
None of this is news to the office world’s big hitters, major league owners, lenders, and brokers. In fact, the country’s biggest banks have all but ceased lending on high-rises, and big equity, the kind you need to buy a $500 million building, has run for the exits. “There is almost no liquidity in the office market today,” a seasoned mortgage broker proclaimed. “No one knows where pricing will be when one of these towers finally does sell.” I asked a handful of industry leaders how much high-rises had dropped in value over the last two years. Their guesses (yes, guesses) ran anywhere from a decline of 25 to 60 percent. This broad lack of consensus is part of the problem; without consensus on value, there is no marketplace, leaving office buildings buried under ten feet of permafrost.
Why? Back to those missing workers. No one (including this writer) knows how many employees will eventually return. Assuming you are OK with deep recessions, the rosy scenario for a prodigal worker homecoming goes like this: tech’s massive lay-offs will continue, employers will regain the whip hand, and they will force their employees’ return. One pundit believes that clever workers will come back on their own once they realize that remote working sets them squarely at the lip of Mount Doom. How? If work remains remote, if employers capitulate to it, companies will stop paying $220,000 a year to some
The problem with this homecoming prediction is its underlying assumption that tech actually wants its employees back. I asked a half-dozen CEOs of small to mid-sized tech companies how efficient they were running remotely. This one had total consensus: they are all humming along, 90-100 percent as effective as they were pre-Covid. That may not be true for the FAANGs of the world (an acronym for the five most popular and best performing tech stocks in the market), and it certainly is not true for start-ups; everyone agrees that nascent companies require all hands to huddle endlessly. But between Google and a garage venture, there must be hundreds, if not thousands, of companies with no need to revisit downtown anytime soon.
The author’s essays recently insisted that, despite all the troubling economic news, real estate would cough up few good deals because of the trillions in opportunity funds desperately seeking yield. Office buildings could prove an exception. In five or six years, we may well look back at 2023-24, whack ourselves on the forehead, and swear, “How the hell did I miss that? I could have bought a Class A office for fifty cents on the dollar.”
If you are willing to place a career bet on the return of the swallows, you could possibly reap the biggest reward real estate has offered since 1992. For what it’s worth, I do think the city will right itself, the employees will return, and landlords will once again toast each other’s brilliance. I’m just glad I don’t have to bet on it.■
A graduate of the University of California at Berkeley and Hastings College of The Law, John co-founded McNellis Partners, a Northern California shopping center development firm, in 1982. John is a decades-long member of the Urban Land Institute and the International Council of Shopping Centers (ICSC), among others. Mr. McNellis writes a monthly essay for the San Francisco Business Times and is the author of Making it in Real Estate: Starting out as a Developer. All of John's essays may be viewed at McNellis.com
This essay originally appeared in The San Francisco Business Times and is reprinted with permission.
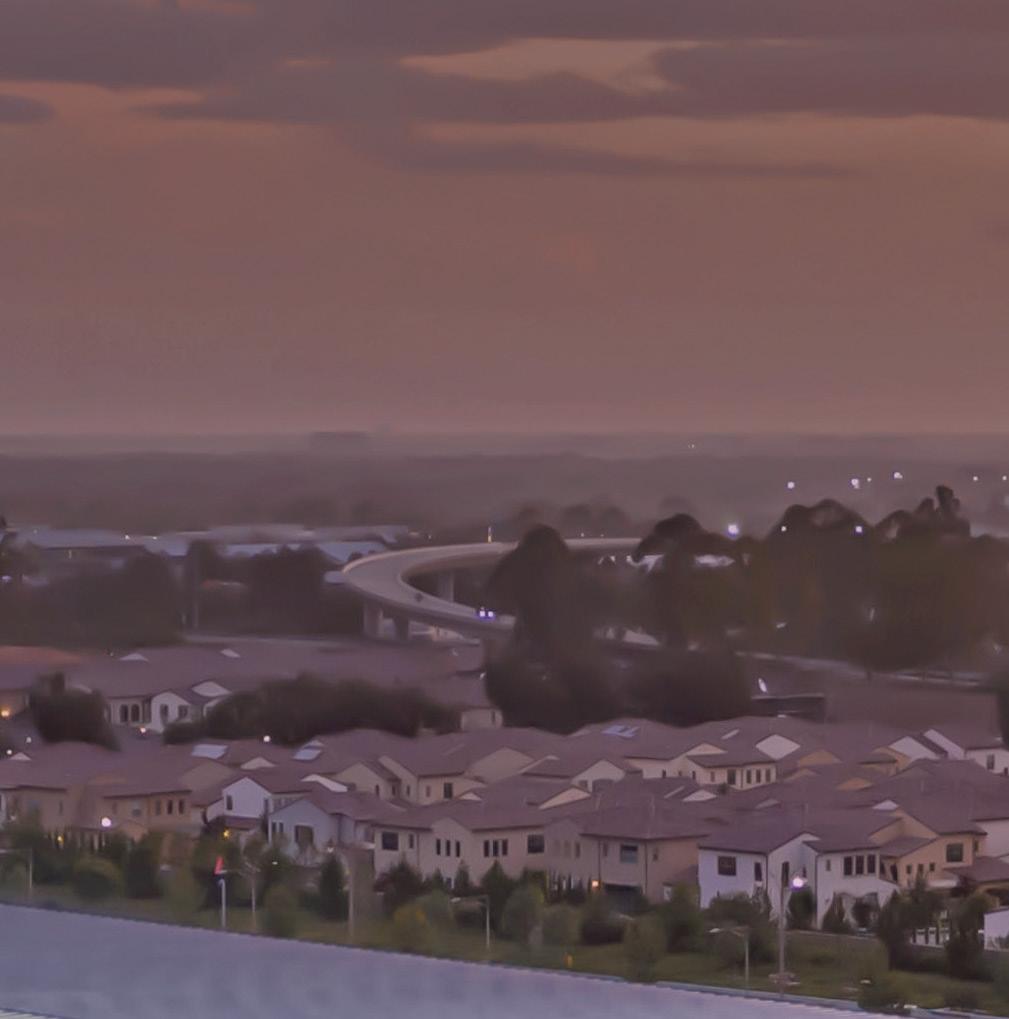
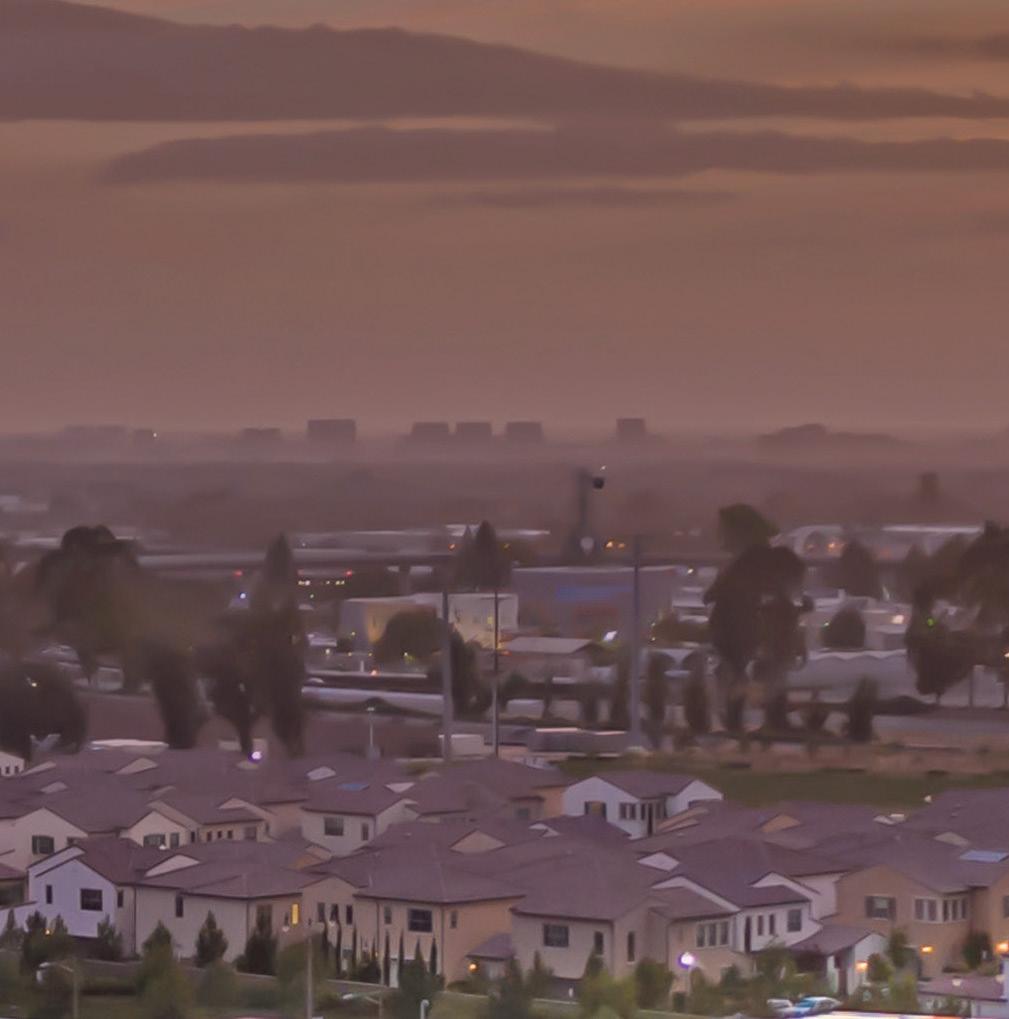
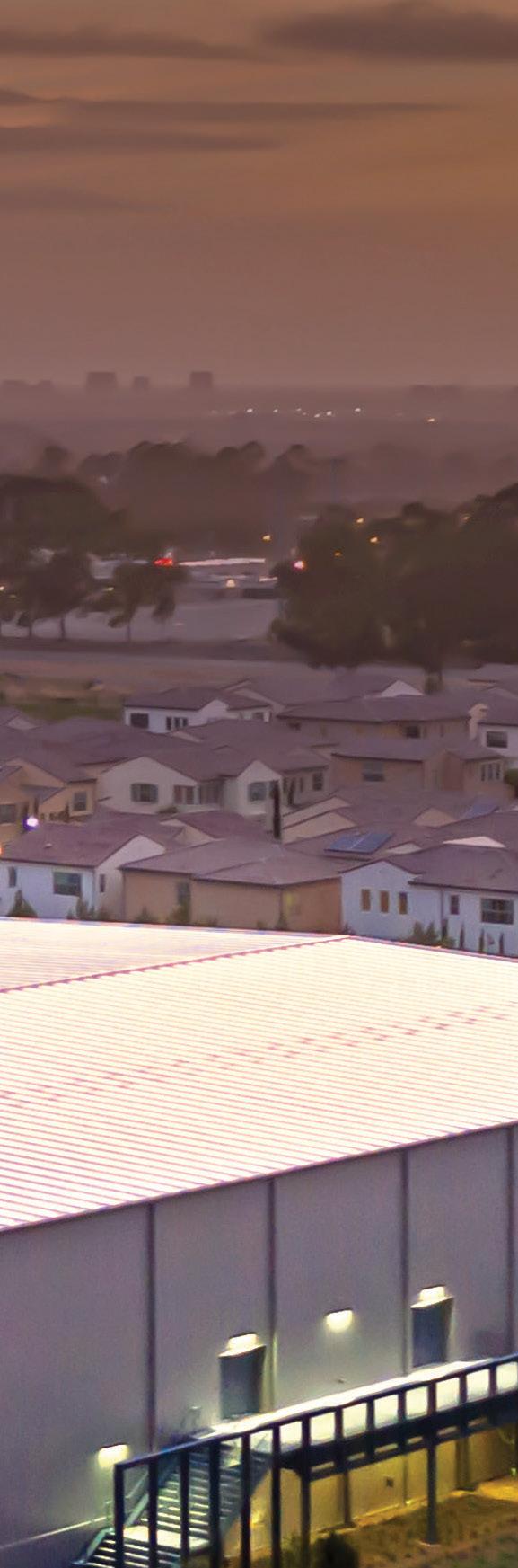
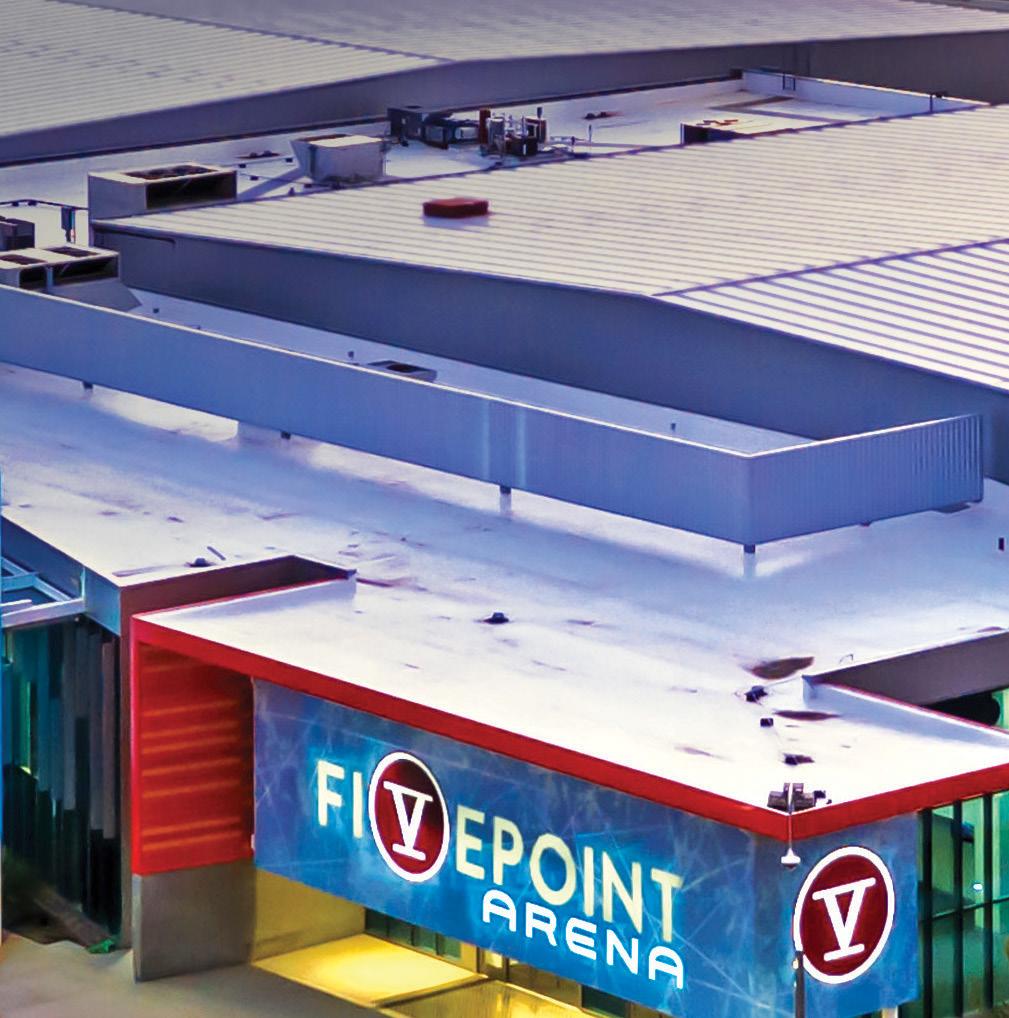
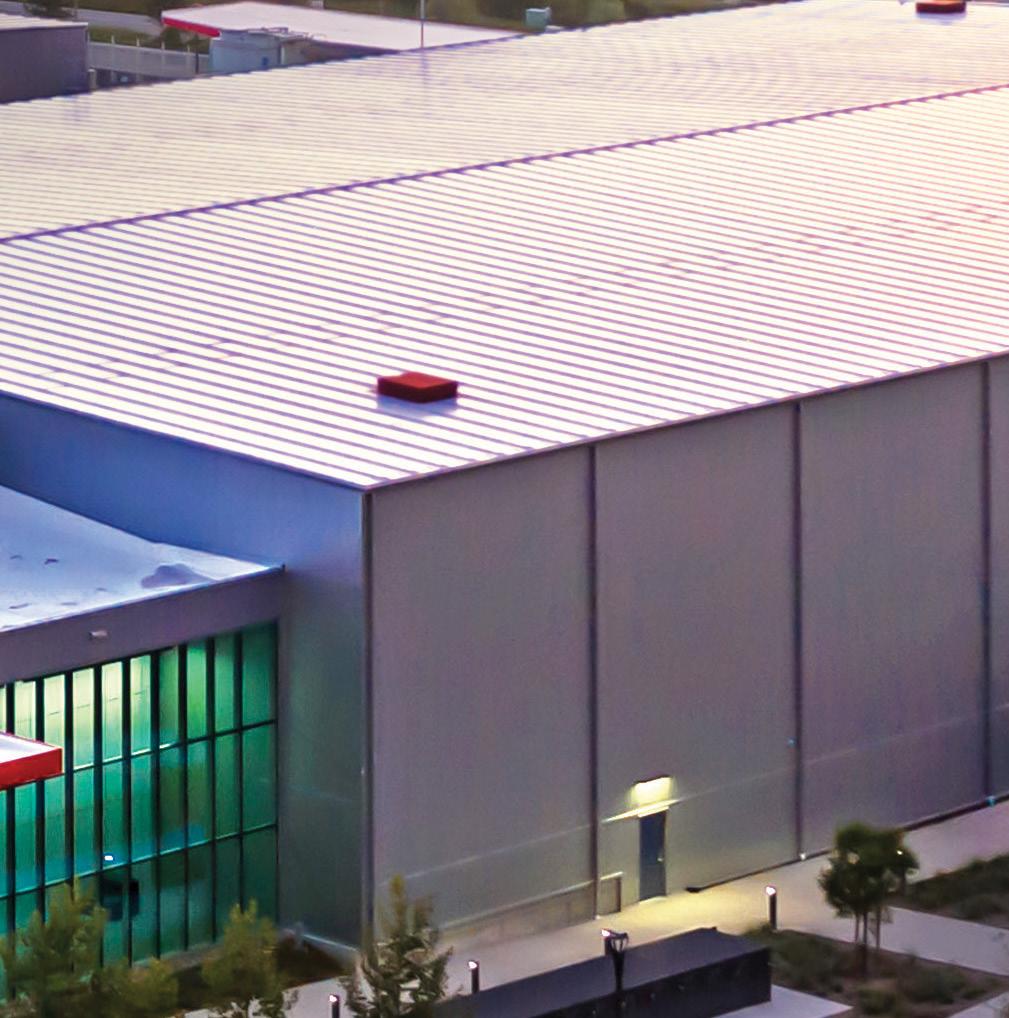
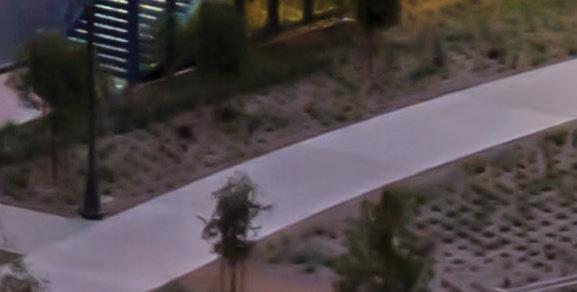
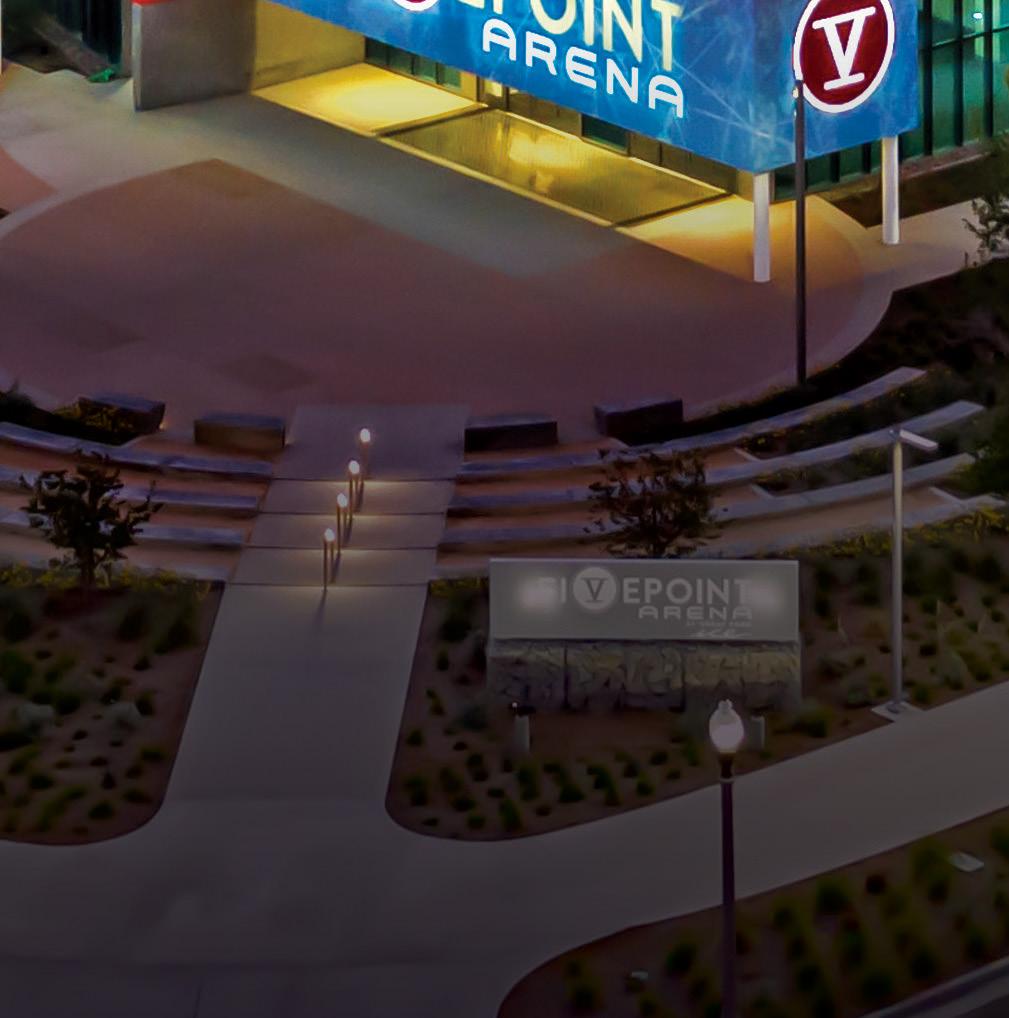
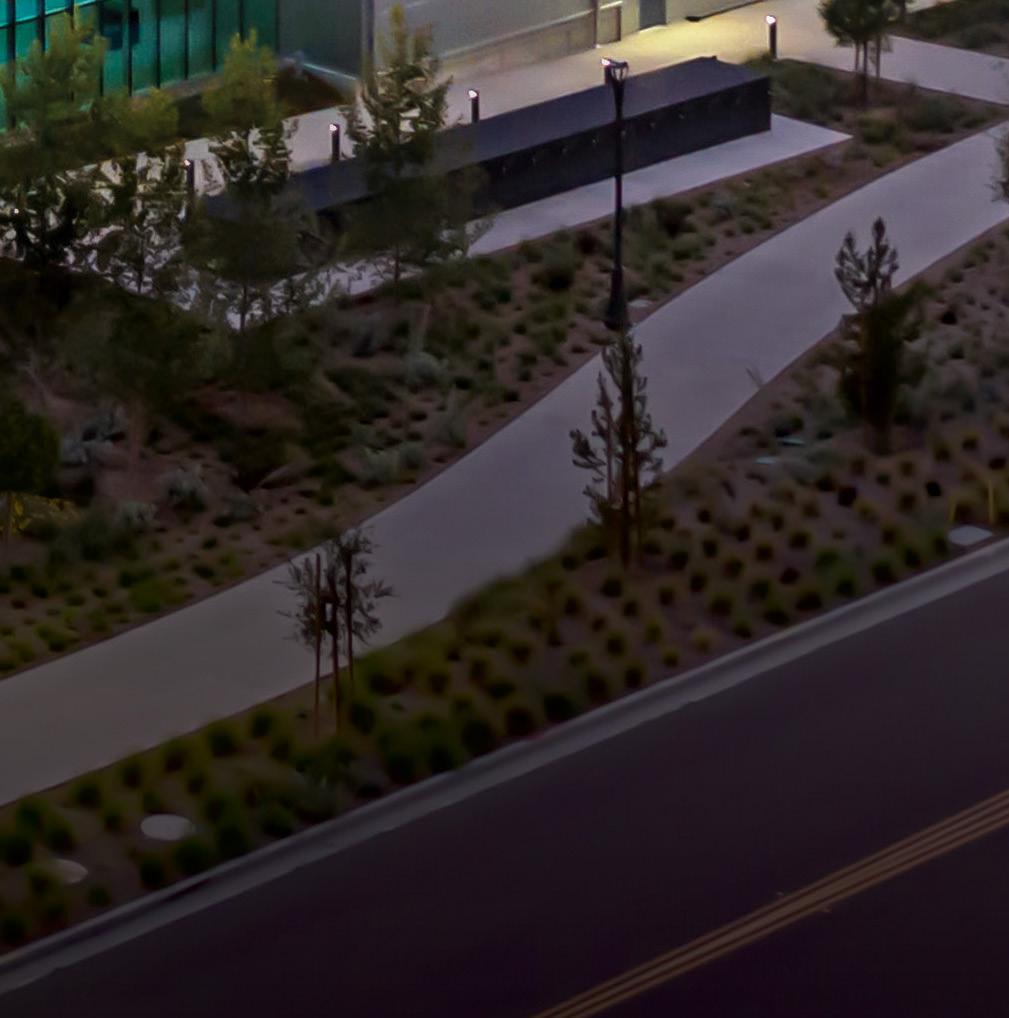
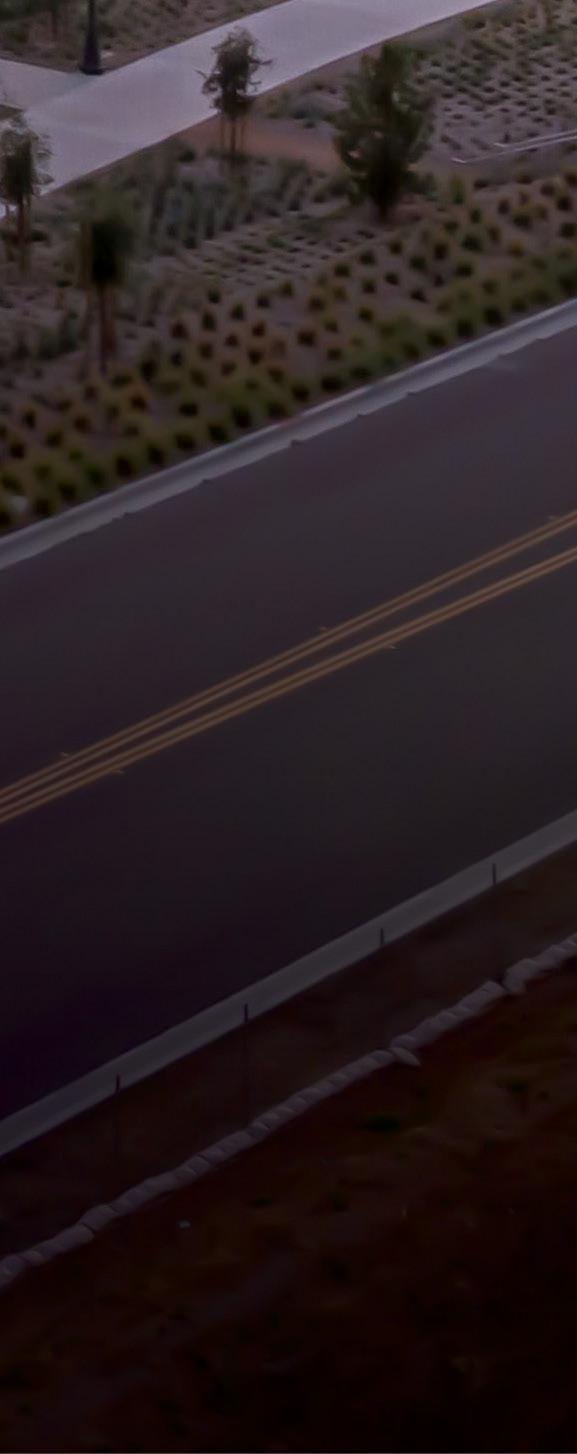
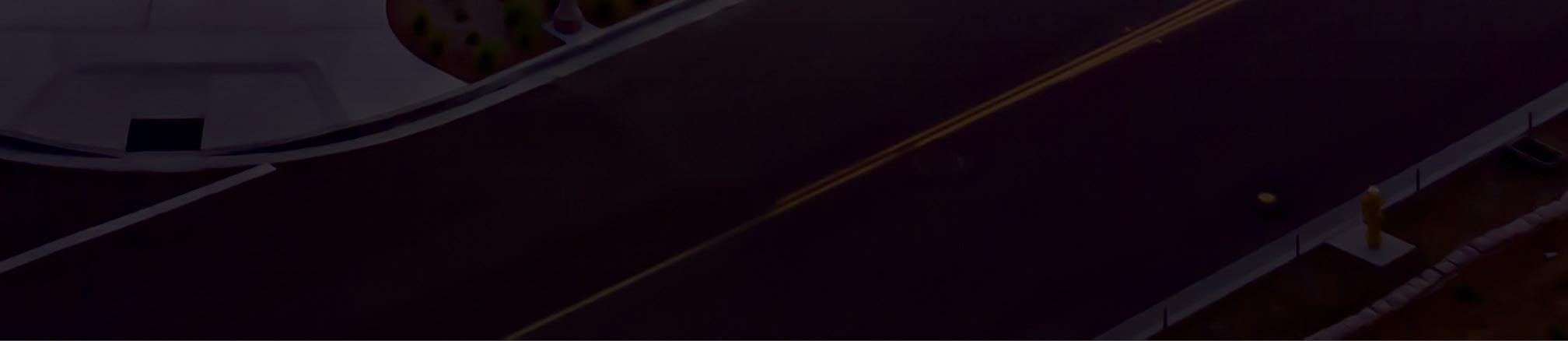
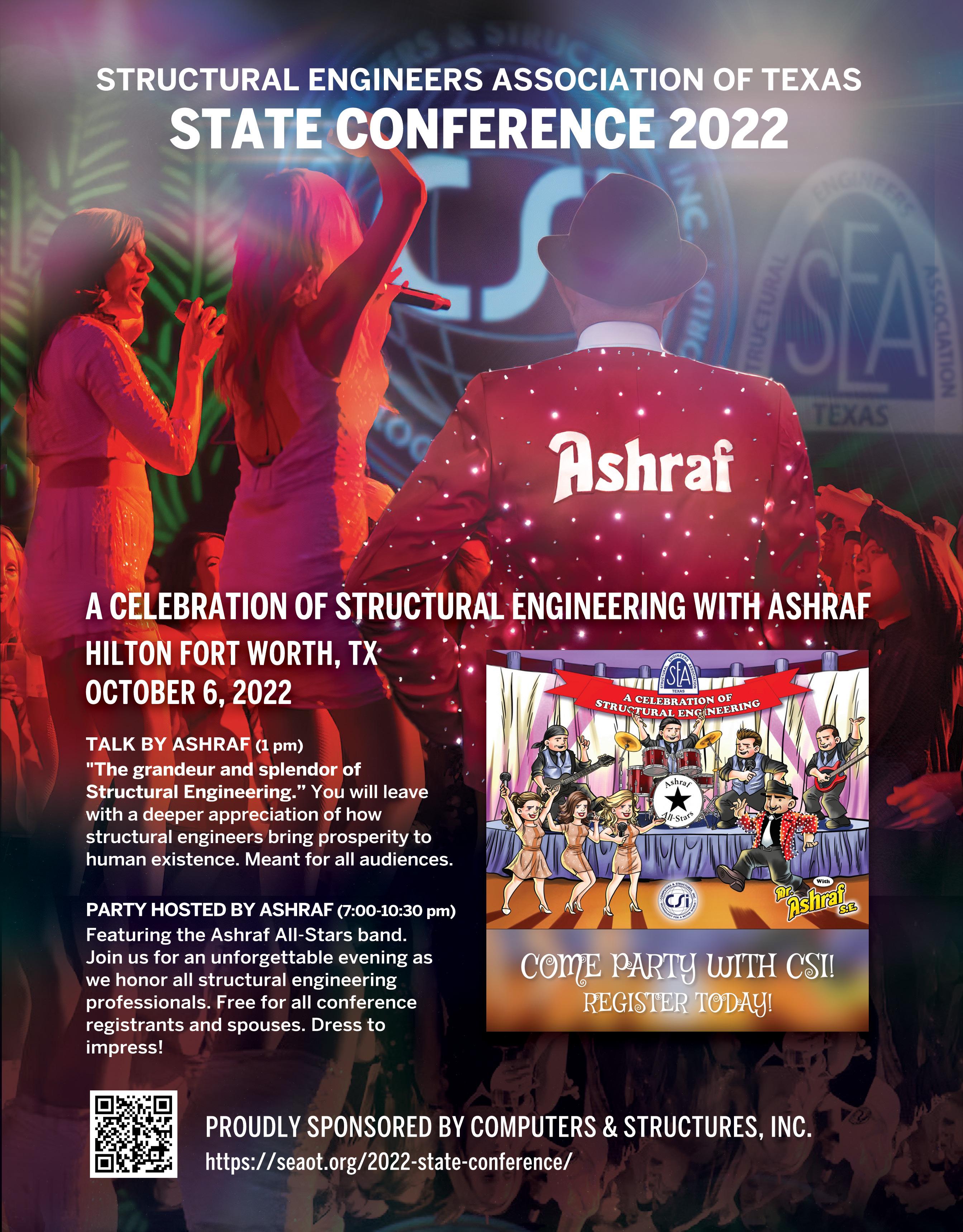