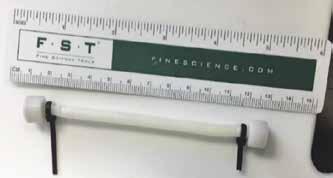
18 minute read
Mechanical characterization of silk derived vascular grafts for human arterial implantation
Patrick Iyasele a , Eoghan M. Cunnane a , Katherine L. Lorentz a , Justin S. Weinbaum a, e , and David A. Vorp a, b, c, d, f, g a Department of Bioengineering, b McGowan Institute for Regenerative Medicine, c Department of Surgery, d Center for Vascular Remodeling and Regeneration, e Department of Pathology, f Department of Chemical and Petroleum Engineering, g Department of Cardiothoracic Surgery
Patrick Iyasele
Patrick Iyasele is a Senior Bioengineer from Milwaukee, Wisconsin. He is pursuing a Ph.D. in Bioengineering with focus on cardiovascular tissue engineering. He is a Foundation board member for Pitt B.R.O.T.H.E.R.H.O.O.D and is highly active in the Pitt Excel Program.
David A. Vorp, PhD
David A. Vorp, PhD, is the Associate Dean for Research at the Swanson School of Engineering. He is also John A. Swanson Professor of Bioengineering, with secondary appointments in the Department of Cardiothoracic Surgery, the Department of Surgery, the Department of Chemical and Petroleum Engineering, and the Clinical and Translational Science Institute at the University of Pittsburgh.
Significance Statement
Occluded blood vessels are commonly treated by revascularization surgery utilizing vascular grafts. Compliance mismatch between the native coronary artery and a vein autograft often leads to restenosis and failure of roughly half of all autografts implanted during revascularization surgery [1]. Due to the limited quantity of autografts and required invasive harvesting, tissue engineered vascular grafts (TEVGs) are being researched and developed as an alternative. This paper presents preliminary data that suggests silk TEVGs may be a suitable alternative as they have similar mechanical properties to native tissue at low strain ratios.
Category: Experimental research Keywords: TEVG, Silk, Mechanical Testing, Tangential Modulus Abbreviations: TEVG- Tissue Engineered Vascular Graft
Abstract
Coronary artery disease occurs from the narrowing and blockage of the vessels supplying blood to the heart, leading to reduced blood flow and tissue damage. The preferred treatment for occluded small diameter arteries is revascularization surgery utilizing vascular autografts including the saphenous vein or internal thoracic artery. However, such autografts are limited in quantity, may be of poor quality and require invasive surgery to harvest and utilize. Additionally, compliance mismatch from the native coronary artery with a vein autograft often leads to restenosis and failure of roughly half of all autografts implanted during bypass surgery [1]. Tissue engineered vascular grafts (TEVGs) are currently being studied and developed as alternatives. A successful TEVG should mimic the mechanical properties of native vessels to reduce failure due to compliance mismatching, therefore, the objective of this study was to calculate the tangential modulus of a bombyx mori (BM) silk derived TEVG and compare it to the tangential modulus of the native vessels it is intended to replace. A BM scaffold was seeded with human cells and analyzed by uniaxial extension testing along with two explanted sheep carotid arteries. This entailed cutting the tube into ring specimens and tensile testing five replicates, recording the stress-strain curve and calculating the tangential modulus. The BM silk TEVG had a similar tangential modulus to a native sheep carotid artery at low strain ratios (1.3) but was significantly lower at high strain ratios (1.9).
1. Introduction
Cardiovascular disease is the leading cause of death worldwide, with most deaths associated with coronary heart disease, cerebrovascular disease, peripheral arterial disease, and deep vein thrombosis. These diseases often occur from the narrowing and blockage of blood vessels leading to reduced blood flow and tissue damage due to inadequate nutrient supply [1]. The preferred treatment for occluded small diameter arteries (such as the coronary arteries) is revascularization surgery utilizing vascular grafts. During this surgery, a graft is used to replace or bypass the damaged or occluded vessel. Around 400,000 coronary artery bypass grafting (CABG) procedures are performed each year in the United States alone [2]. The saphenous vein and internal thoracic artery are commonly used for autografts but those are limited in quantity, may be of poor quality and require invasive surgery to harvest and utilize [1]. Compliance mismatch from the native coronary artery and a vein autograft leads to restenosis and failure of roughly half of all autografts implanted during bypass surgery [1]. Tissue engineered vascular grafts (TEVGs) are currently being studied and developed as alternatives.
An ideal TEVG should mimic the mechanical properties of native tissues. Compliance mismatch from the native coronary artery and a vein autograft lead to restenosis and failure of roughly half of all autografts implanted during bypass surgery [1]. Tangential modulus has been a useful property to ascertain in this regard as it describes the stiffness of a material at certain mechanical strains that are experimentally tested during uniaxial
extension. Stiffness determines a vessels mechanical reaction to cardiac pressure waves. Replicating vessel stiffness of native tissue is critical to developing accurate blood vessel replacements [3]. The TEVG tested in this study is made from Bombyx mori (BM) silk. BM silk has considerable mechanical strength compared to its weight. Also, silk is resistant to digestion from most proteolytic enzymes, biocompatible, has controllable degradability, has the ability to be fabricated into different shapes, and has the ability for amino acid side chain modification [4]. The TEVG is seeded with human adipose-derived stem cells using a large-seeding device that perfuses cells in a rotating assembly that has been previously developed in our laboratory [5]. According to Issa et al., the number of cells seeded to a scaffold can change the mechanical properties of the scaffold [6]. The TEVG is seeded because seeding autologous endothelial cells onto the luminal surface of graft has been seen to improve patency rates. The absence of endothelial cells lining the lumen leads to the adherence of blood proteins and the activation of clotting mechanisms causing thrombosis [1]. The graft in this paper is seeded with adipose-derived stem cells which differentiate into smooth muscle cells and endothelial cells. Adipose-derived stem cells are used as they can be extracted in high quantities from adipose tissue aspirate and are easy to harvest [1]. The objective of this study is to quantify the tangential modulus of a BM silk derived TEVG and compare it to the tangential modulus of the native vessels it is intended to replace.
2. Methods 2.1 TEVG Fabrication
2.1.1 Graft Casting The grafts were fabricated by loading a 6% BM silk solution (by weight) into cylindrical molds. The silk solution was a generous gift from Dr. Biman Mandal, IIT-Guwahati. The mold was comprised of an outer stainless-steel tube that had a plastic straw inserted into it to provide a smooth surface to prevent damage to the graft during extraction. The mold had a 5.5 mm stainless steel rod that was inserted into the tube to provide the center alignment and stability for the tubular graft structure. This configuration was secured by two custom-designed 3D printed caps at the center of the tube that formed a watertight seal to keep the silk solution from leaking out of the mold. The filled mold was frozen at -20° C for 24 hours and then lyophilized for 12 hours. After lyophilization, the produced graft was submerged in an 80% ethanol solution. From the ethanol treatment, dense membranes of silk fibers were crystallized to the β-sheet conformation which are suitable for adherence and growth of endothelial cells [7]. The finished graft had an inner diameter of 5.5 mm, an outer diameter of 7.1 mm, and a length of 10 cm (Figure 1).
Figure 1: The finished silk TEVG. The final TEVG had an inner diameter of 5.5 mm, an outer diameter of 7.1 mm, and a length of 10 cm. An electrospun layer of silk/PCL was added around the internal structure to increase mechanical strength.
2.1.2 Addition of Electrospun Layer An electrospun layer made up of a 1:1 mixture of silk and polycaprolactone (PCL) was added to the outside of the graft to add additional mechanical strength to the graft using a custom electrospinning device [3]. The device consisted of a power drill on a linear actuator, a syringe pump, and a 12.5 kV voltage generator. The graft was put on a rod that was inserted into the drill head in order to rotate and move linearly. The voltage generator was connected to the rod on its negative terminal causing the graft to be negatively charged and the head of the syringe in the syringe pump was connected to the positive terminal giving the silk/PCL solution a positive charge. This polarization causes extruded silk/ PCL to be attracted to the graft due to electrostatic forces. The drill was set to 200 RPM and 50 linear cycles/min and the syringe pump was set at a .1 ml/min output rate. A total of 1ml of silk/ PCL was dispensed from the syringe. When the drill, pump, and generator were activated, a thin fiber of the silk/PCL mixture was stretched from the tip of the syringe pump to the graft causing the addition of a second silk layer onto the graft. 2.1.3 Vacuum Cell Seeding The scaffold was bulk seeded with 120 million human adipose-derived stem cells in order to produce a graft seeded at similar density to previous work using smaller-scale grafts [8]. This was done by using a custom vacuum rotational seeding device (Figure 2). The cultured cells were suspended and put into a syringe and pumped into the vacuum chamber. The vacuum chamber pressure was -127 mmHg. A cylindrical diffusing nozzle fabricated to match the internal diameter of the graft was used to spray the cell suspension onto the scaffold’s inner surface. The nozzle was attached to a rod that traveled the full length of the graft. The graft was rotated around the diffusion nozzle, inside an acrylic vacuum chamber. This hybrid method of rotational and vacuum seeding combined the best qualities of both methods. With the diffusion nozzle approximating very closely with the internal surface of the graft, the cells were better able to penetrate the graft. Because the graft was rotating under vacuum there was a pressure gradient to further enhance seeding while the rotation
helped to uniformly distribute the cells circumferentially. 30 ml of cell suspension was dispensed through the nozzle at 15 ml/min. The graft was rotated at 60 RPM and the linear displacement for the nozzle was 1 linear cycle /min.
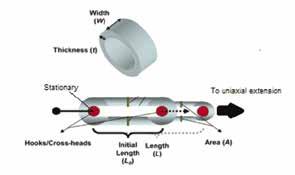
Figure 2: Custom cell vacuum rotational seeding device. A cylindrical diffusing nozzle sprays the cell suspension onto the scaffold’s inner surface inside an acrylic vacuum chamber. the cells were better able to penetrate the graft to uniformly distribute the cells circumferentially [9]. The scaffold was bulk seeded with 120 million human adipose-derived stem cells.
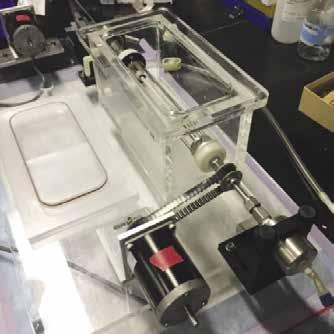
2.2 Uniaxial Tensile Testing
Two sheep carotid arteries were explanted and the TEVG were cut into ring specimens which were tested under uniaxial tension to determine their stress-strain response and tangential modulus at both low (1.3) strain ratio and high (1.9) strain ratio (Figure 3). According to Véronique Laterreur et al., data from a ring tensile test provides an accurate estimate of the failure strain and the stiffness of the graft when compared to measurements with the direct method which consists of pressurizing the graft with fluid until tissue failure [9].

b) Figure 3: Schematic for uniaxial ring testing. Ring tests are performed to obtain stress-strain curves and thereby determine the mechanical properties of the TEVG and carotid arteries. The diameter, width (W) and wall thickness (t) of each of the ring specimens were used to determine A 0 and L 0 . These parameters were used to calculate corresponding stress, strain and tangential modulus values [3].
The diameter, width and wall thickness of each of the ring specimens were measured in pixels using Image-J. A pixel to millimeter scaling factor from a metric ruler in captured images was used to convert measured pixels into millimeters. Force and extension values were measured from an Instron 5543A uniaxial extension tester and were converted into stress and strain ratio values (Figure 4). Stress was calculated by equation 1 where F is the load in Newtons (N) and A 0 is the initial cross-sectional area:
(1)
Strain ratio was calculated using equation 2 where L 0 is the initial length and λ is the extension:
(2)
Tangential modulus was calculated by using linear regression on the low and high strain ratio regions. The low strain ratio region was designated as the 1.2 - 1.4 strain ratio region of the full stress-strain curve. The high strain ratio region was designated as the 1.8 - 2.0 strain ratio region. A linear fitting was applied to each region yielding tangential modulus calculated as the slope of the fitted line [10].
c)
Figure 4: Stress-strain curves for the three samples tested. The low strain ratio region is labeled orange, and the high strain ratio region is labeled red. a) Right sheep carotid artery stress-strain curve. b) Left sheep carotid artery stress-strain curve. c) Silk TEVG stress-strain curve. The silk TEVG is a linear sigmoidal shape (Figure 4c) but the arterial stress-strain curves are a non-linear logarithmic shape.
3. Results
Five replicates for both sheep carotid arteries as well as the TEVG were tested. The tangential modulus for the TEVG was 0.11 ± 0.02 MPa in the low strain ratio and 0.15 ± 0.03 MPa in the high strain ratio region (Figure 5). The high strain ratio region tangential modulus was significantly higher for the left and right carotids compared to the TEVG (4.3 ± 1.3 MPa and 3.6 ± 0.66 MPa vs. 0.15 ±. 03 MPa, respectively; both p= 0.0079 using two tailed Mann-Whitney Test, α= 0.05). The low strain ratio region tangential modulus was not statistically different between the left and right carotid artery and the TEVG (0.07 ± 0.03 MPa and 0.12 ± 0.04 MPa vs. 0.11 ± 0.02 MPa, respectively; p=0.095 and 0.8413).
a)
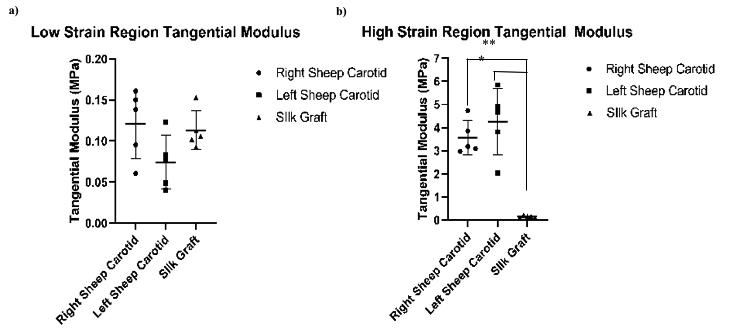
b)
Figure 5: Samples had similar modulus at low strain ratios, but the TEVG modulus was lower at high strain ratios. a) Tangential moduli between the left and right sheep carotids and the silk graft at 1.3 strain ratio region. b) Tangential moduli between the left and right sheep carotids and the silk graft at 1.9 strain ratio region. A two tailed Mann-Whitney Test indicated no significant statistical difference between any groups in the low strain region, but showed significant statistical difference in the high strain region. Asterisks specify statistically significance between groups. * p= 0.0079. **p= 0.0079
4. Discussion
The BM silk TEVG had a similar tangential modulus to a native sheep carotid artery at low strain ratios but was significantly lower at high strain ratios. The difference in tangential moduli between the high strain ratio regions of the TEVG and sheep carotids may come from the TEVG not being remodeled by macrophages and arterial cells as it would be post-implantation [11]. Vascular remodeling is an active process of structural change that involves changes in at least four cellular processes: cell growth, cell death, cell migration, and the synthesis and degradation of extracellular matrix [12]. Cells would not have added any structural proteins to the vessel to increase stiffness since they did not have an implantation or in vitro culture period. Even though the tangential modulus at low strain ratio is similar, the overall shapes of the stress-strain curves are different. The silk TEVG stress-strain curve is a linear sigmoidal shape (Figure 4c) but the arterial stress-strain curves are a non-linear logarithmic shape (Figure 4a, b).
According to Peterson et al., the strain which the arteries undergo as a result of arterial pulse pressure variations is normally between 0.01 and 0.04, i.e., between 1% and 4% change in circumference. The total strain associated with marked constriction and dilation does not usually exceed 10% [13]. Having similar tangential modulus at the 1.3 strain ratio region provides evidence that the graft would not rupture at physiological strains of 10%. It also provides evidence that at this strain region there is no compliance mismatch between the graft and native vessel which causes blood flow disturbances resulting in the development of atherosclerotic plaque. In Soffer, et al., they fabricated a silk scaffold made with an 8% silk solution and a 7.5% silk/ poly(ethylene oxide) solution for the electrospun layer [14]. They determined their graft to have a tangential modulus of 2.45 ± 0.47 MPa at the 50% strain region. Although the tangential modulus of their graft is significantly different from the graft fabricated in this paper, the tangential modulus for a human carotid artery and thoracic aorta at 50% strain is 0.04 ± 0.9 [15][16].
A limitation of this study is its use of uniaxial ring extension testing. The stationary cross heads used to pull the ring specimens may cut into the specimen instead of pulling it. This may happen due to the softness of the specimens and that the cross heads were made out of steel. Also, the scaffold was not cultured to allow for cellular remodeling. Cellular remodeling would change the mechanical properties of the graft. The change in mechanical properties of the graft due to remodeling may make it a closer match to native vessels. This would provide data on the optimum time point to implant a graft to minimize compliance mismatch. This study could be improved by increasing the sample size of all groups tested. The limited sample size hinders us from determining if this is a real effect and from determining if our fabrication technique is consistent.
This paper presents preliminary data that suggests silk TEVGs may be a suitable alternative as they have similar mechanical properties to native tissue at low strain ratios. Even with the cells seeded in the graft, the graft seems to have similar enough mechanical properties to be immediately implanted in vivo. A future study would be to repeat the scaffold seeding and extension test to obtain more replicates for the data set. If the graft has mechanical stiffness that is no different than the tissue it is meant to replace, compliance mismatch which leads to restenosis could be mitigated. The graft would prevent the need for autografts which would prevent the required invasive surgery to harvest and utilize them.
5. Conclusions
This preliminary data suggests that our silk TEVG seeded with 120 million human adipose-derived stem cells has a similar tangential modulus to a native sheep carotid artery at low strain ratios but is significantly lower at high strain ratios. At low strains, the TEVG behaves similarly to native carotid artery which would reduce compliance mismatch after bypass surgery. Even though the low strain regions are above the physiological strain of 10%, the silk TEVG grafts behave linearly while native tissue behaves non-linearly. This difference may cause damage or rupture of the graft if strain on the vessels went above physiological strain.
6. References
[1] Pashneh-Tala, Samand et al. “The Tissue-Engineered Vascular Graft-Past, Present, and Future.” Tissue engineering. Part B, Reviews vol. 22,1(2016):68-100.
[2] Go, Alan S et al. “Heart disease and stroke statistics--2013 update: a report from the American Heart Association.” Circulation vol. 127,1 (2013): e6-e245.
[3] Stankus, John J et al. “Fabrication of cell microintegrated blood vessel constructs through electrohydrodynamic atomization.” Biomaterials vol.28,17(2007):2738-46.
[4] Vepari, Charu and David L Kaplan. “Silk as a Biomaterial.” Progress in polymer science vol. 32,8-9 (2007): 991-1007. [5] Soletti, Lorenzo, et al. “A Seeding Device for Tissue Engineered Tubular Structures.” Biomaterials, vol. 27, no. 28, 2006, pp. 4863–4870., 2006.04.042.
[6] Issa, Rita I., et al. “The Effect of Cell Seeding Density on the Cellular and Mechanical Properties of a Mechanostimulated Tissue-Engineered Tendon.” Tissue Engineering Part A, vol. 17, no. 11-12, 2011, pp. 1479–1487., 2010.0484.
[7] Nogueira, Grínia M., et al. “Preparation and Characterization of Ethanol-Treated Silk Fibroin Dense Membranes for Biomaterials Application Using Waste Silk Fibers as Raw Material.” Bioresource Technology, vol. 101, no. 21, 2010, pp. 8446–8451.
[8] Krawiec, Jeffrey T et al. “In Vivo Functional Evaluation of Tissue-Engineered Vascular Grafts Fabricated Using Human Adipose-Derived Stem Cells from High Cardiovascular Risk Populations.” Tissue engineering. Part A vol. 22,9-10 (2016): 765- 75. doi:10.1089/ten.TEA.2015.0379
[9] Véronique Laterreur et al, Comparison of the direct burst pressure and the ring tensile test methods for mechanical characterization of tissue-engineered vascular substitutes, Journal of the Mechanical Behavior of Biomedical Materials, Volume 34, 2014, Pages 253-263
[10] Khalil Khanafer et al, Determination of the elastic modulus of ascending thoracic aortic aneurysm at different ranges of pressure using uniaxial tensile testing, The Journal of Thoracic and Cardiovascular Surgery, Volume 142, Issue 3, 2011, 682-686 [11] Theodoridis, Karolina et al. “Six-Year-Old Sheep as a Clinically Relevant Large Animal Model for Aortic Valve Replacement Using Tissue-Engineered Grafts Based on Decellularized Allogenic Matrix.” Tissue Engineering Part C: Methods. vol: 23 Issue 12: December 1, 2017
[12] Renna, Nicolás F et al, “Pathophysiology of Vascular Remodeling in Hypertension,” International Journal of Hypertension, vol. 2013, ArticleID 808353, 2013.
[13] Peterson, Lysle H., et al. “Mechanical Properties of Arteries in Vivo.” Circulation Research, vol. 8, no. 3, 1960, pp. 622–639., 8.3.622.
[14] Soffer, Leah et al. “Silk-based electrospun tubular scaffolds for tissue-engineered vascular grafts.” Journal of biomaterials science. Polymer edition vol. 19,5 (2008): 653-64. doi:10.1163/156856208784089607 [15] Adham M, Gournier J, Favre J, De La Roche E, Ducerf C, Baulieux J, Barral X, Pouyet M. Journal of Surgical Research. 1996;64:32.
[16] Roeder R, Wolfe J, Lianakis N, Hinson T, Geddes L, Obermiller J. Journal of Biomedical Materials Research. 1999;47:65.
7. Acknowledgements
Thanks to the Swanson School of Engineering and Dr. Vorp for funding that made this work possible. Thanks to Dr. Jonathan Vande Geest for his generous gift of sheep arteries.